- 1Center for Global Safe Water, Sanitation, and Hygiene, Rollins School of Public Health, Emory University, Atlanta, GA, United States
- 2Hope Clinic of the Emory Vaccine Center, Division of Infectious Diseases, Department of Medicine, School of Medicine, Emory University, Atlanta, GA, United States
- 3Division of Infectious Diseases, Emory University School of Medicine, Atlanta, GA, United States
Since the coronavirus disease 2019 (COVID-19) pandemic, wastewater-based epidemiology (WBE) has been widely applied in many countries and regions for monitoring COVID-19 transmission in the population through testing severe acute respiratory syndrome coronavirus 2 (SARS-CoV-2) in wastewater. However, the amount of virus shed by individuals over time based on the stage of infection and accurate number of infections in the community creates challenges in predicting COVID-19 prevalence in the population and interpreting WBE results. In this study, we measured SARS-CoV-2, pepper mild mottle virus (PMMoV), and human mitochondrial DNA (mtDNA) in longitudinal fecal samples collected from 42 COVID-19 patients for up to 42 days after diagnosis. SARS-CoV-2 RNA was detected in 73.1% (19/26) of inpatient study participants in at least one of the collected fecal specimens during the sampling period. Most participants shed the virus within 3 weeks after diagnosis, but five inpatient participants still shed the virus between 20 and 60 days after diagnosis. The median concentration of SARS-CoV-2 in positive fecal samples was 1.08 × 105 genome copies (GC)/gram dry fecal material. PMMoV and mtDNA were detected in 99.4% (154/155) and 100% (155/155) of all fecal samples, respectively. The median concentrations of PMMoV RNA and mtDNA in fecal samples were 1.73 × 107 and 2.49 × 108 GC/dry gram, respectively. These results provide important information about the dynamics of fecal shedding of SARS-CoV-2 and two human fecal indicators in COVID-19 patients. mtDNA showed higher positive rates, higher concentrations, and less variability between and within individuals than PMMoV, suggesting that mtDNA could be a better normalization factor for WBE results than PMMoV.
1 Introduction
COVID-19 (coronavirus disease 2019) is caused by SARS-CoV-2 (severe acute respiratory coronavirus 2), a positive-sense single-stranded RNA virus. Although COVID-19 is a respiratory disease and patients predominantly manifest respiratory symptoms, systemic and respiratory manifestations are often accompanied by gastrointestinal symptoms and fecal shedding of viral RNA. Meta-analysis of studies that focus on gastrointestinal (GI) infection and fecal shedding in patients with COVID-19 report the prevalence of GI symptoms to be around 17.6% (1) and 28.5% (2) in patients with severe COVID-19 during the acute infection phase. In COVID-19 patients, the prevalence of positive RNA in stool samples was about 50% (1, 3, 4) within the first week of diagnosis. Interestingly, several studies that have collected paired longitudinal respiratory and fecal samples demonstrated prolonged fecal shedding and higher viral load in feces compared to the paired respiratory samples collected at the same time period (3, 5). This suggests that SARS-CoV-2 infection of the GI tract persists longer than in the respiratory tract and the GI tract excretes more viruses than the respiratory tract. SARS-CoV-2 concentration in stool was reported to be between 102 to 107 GC/mL in an early study (6) for the prototype SARS-CoV-2, but there is a lack of this information for the recent variants.
While the presence and prevalence of SARS-CoV-2 RNA in the GI tract are well established, much less is known about the duration and the amount of SARS-CoV-2 fecal shedding in patients with COVID-19. Natarajan et al. (3) reported that 12.7% of subjects still shed SARS-CoV-2 at 4 months and 3.8% of patients shed SARS-CoV-2 at 7 months post diagnosis. Whereas there was no evidence of ongoing oropharyngeal virus shedding at this time. Additionally, one study suggested that fecal SARS-CoV-2 RNA shedding may start 3–4 days before the onset of the symptoms (6), indicating that the duration of SARS-CoV-2 fecal shedding may be even longer.
Studies have also shown that individuals with no clinical symptoms (asymptomatic infection) can shed high concentrations of SARS-CoV-2 RNA in their feces (4, 7–9), contributing to the overall viral load in wastewater. There seems no statistically significant difference in the viral load between symptomatic and asymptomatic patient fecal samples (8, 9).
Many studies have observed significant correlations between the SARS-CoV-2 RNA concentration in wastewater and the number of confirmed COVID-19 cases reported in the corresponding wastewater catchment areas (10–19), which highlights the application of WBE to monitor the burden of viral infection in the population. Other studies have attempted to use SARS-CoV-2 fecal shedding levels as parameters in models to predict the prevalence or incidence of COVID-19 in communities (12, 20, 21). Predicting prevalence or incidence in the population is critical for public health decision-making. This application has been explored via several statistical modeling approaches (21–23); however, using modeling to accurately predict COVID-19 prevalence or incidence in populations from SARS-CoV-2 concentrations in wastewater requires longitudinal and quantitative SARS-CoV-2 fecal shedding data. To date, there have been several reports on SARS-CoV-2 fecal shedding, but many studies lack longitudinal samples, quantitative SARS-CoV-2 concentrations (report Ct values rather than absolute quantification) in stool, accurate timing of when the samples were collected after the onset of symptoms, specific amount of fecal material used for viral quantification, and the limit of detection of the assay used for viral quantification, etc. (4, 24). This missing information in fecal shedding data limits the appropriate application of the models and the interpretation of WBE modeling results (25).
Several fecal indicators, including human ribonuclease P (26), PMMoV (27), Bacteroides HF183 (28); F-specific RNA bacteriophages (29), human 18S rRNA (30), cross-assembly phage (CrAssphage) (31), have been proposed. Pepper mild mottle virus (PMMoV) and human mitochondrial DNA (mtDNA) are two human fecal indicators which are often analyzed along with SARS-CoV-2 quantification in wastewater samples, and both are used for normalization of SARS-CoV-2 concentrations in wastewater (32–36). PMMoV, a plant RNA virus that causes infection in pepper crops, is excreted in high concentrations in human fecal material if people consume food products containing infected peppers. Up to 109 virion particles of PMMoV have been reported per gram of human feces by dry weight (37). Because of its abundance in human stool, persistence in the environment, and multiple exposure pathways to humans, PMMoV is frequently reported as a human fecal indicator in WBE studies (34, 38). Human mtDNA is exclusively human in origin and highly abundant in human feces. However, it is less frequently reported (36, 39) than PMMoV and is potentially a new, reliable human fecal indicator. This marker has been detected in high copy numbers in 100% of human fecal specimens across multiple geographic regions (40) and in 92% of sewage samples (41).
The objective of this study was to examine the fecal shedding dynamics of SARS-CoV-2 RNA, PMMoV, and mtDNA in longitudinal fecal samples collected from confirmed COVID-19 inpatient and outpatient participants. The results can be used to better predict COVID-19 prevalence or incidence in communities using mathematical modeling and guide interpretation of SARS-CoV-2 RNA levels in wastewater samples using WBE approaches.
2 Materials and methods
2.1 Study description and stool sample collection
Study participants were recruited from the inpatient clinic at Emory University Hospital between March 21, 2021 and September 21, 2021 and the outpatient clinic by the Hope Clinic of Emory Vaccine Center between September 22, 2021 and July 28 2022. All participants were required to sign an informed consent. In addition, information on demographics, clinical symptoms, and COVID-19 vaccination was also collected. COVID-19 patients were enrolled as study participants if an in-house real-time RT-PCR test detected SARS-CoV-2 RNA in their nasopharyngeal swab samples or other commercial assays (e.g., antigen test) showed SARS-CoV-2 antigen in their saliva specimens within 7 days after the onset of COVID-19 symptoms. Participants with any behavioral, cognitive, or psychiatric condition were excluded from the enrollment. The day with SARS-CoV-2 positive detection was considered the COVID-19 confirmation date in this study. The first stool sample was collected after the participant was enrolled, usually within 7 days after the confirmation date. The date of the first stool sample collection was defined as Day 1, and subsequent samples were collected on Days 3, 7, 14, 28, and 42 from the first stool sample for each subject. The sampling day was converted to the day of COVID-19 confirmation for data analysis and visualization purposes. Stool collection kits were provided to study participants. For inpatients, stool samples were picked up from the hospital room and there was usually no delay. For outpatients, stool collection kits were shipped to the home address, and study participants were asked to ship samples back to the Emory study lab using prepaid mailers. These samples were usually delayed for shipment and may not have been kept all the way in the cold chain. Once received by the study lab, the samples were immediately stored at −80°C prior to nucleic acid extraction and RT-qPCR detection.
2.2 Fecal sample processing and nucleic acid extraction
Stool samples were processed by the physical disruption method of bead lysing followed by total nucleic acid extraction with an automated purification platform, Qiagen EZ1 Advanced XL (Qiagen, #9001875). First, fecal specimens were thawed on ice from −80°C. Each specimen was precisely weighed on an analytical balance, ranging between 27.0 mg to 33.1 mg, into a 2.0 mL pre-filled 0.7 mm garnet bead tube (Omni International, #19-624). Then, 600 μL of Qiagen PowerBead solution (Qiagen, #12955-4-BS) was added to each wet sample. Specimens were immediately mixed on a Bead Genie (Scientific Industries Inc., #SI-B100) at 3,000 rpm for 2 min, which introduces physical agitation resulting in the lysing of the sample matrix and homogenizing the sample. A 200 μL aliquot of lysate was added to 5,000 genome copies (10 μL) of Bovine Respiratory Syncytial Virus (BRSV) (INFORCE 3, Zoetis, Parsippany, NJ) and processed by the Qiagen EZ1 Advanced XL with the Qiagen EZ1 DSP virus kit (Qiagen, # 62724) and Qiagen EZ1 Advanced DSP Virus Card (Qiagen, #9018306). BRSV served as an internal processing control during the concentration and extraction procedures. Total nucleic acids were extracted by a Zymo Research OneStep™ PCR Inhibitor Removal kit (Zymo Research, #D6030). A Zymo-Spin™ III-HRC Column (Zyme Research, #C1005) was used for each sample as per the manufacturer’s instructions. We inserted the column into a collection tube and added 600 μL of Prep-Solution. Then, we centrifuged the column at 8,000 × g for 3 min. The prepared column was transferred to a clean 1.5 mL microcentrifuge tube, and 60 μL of RNA was added to the Zymo-Spin™ III-HRC Column. The sample was then centrifuged at 16,000 × g for 3 min. Total nucleic acid was collected and stored at 80°C.To estimate the dry weight, each fecal sample was weighed, placed onto an aluminum weigh boat, incubated at 105–110°C for 24 h, and re-weighed after incubation.
The dry weight percent for the calculation of SARS-CoV-2 concentrations per mass of dry weight was calculated using the following formula:
2.3 Quantification of SARS-CoV-2, PMMoV, and mtDNA in stool samples using dPCR
Digital PCR was performed using the QIAcuity Digital PCR System and QIAcuity OneStep Advanced Probe Kit (Qiagen, #250132) following the manufacturer’s protocol. The Qiagen QIAcuity instrument was programmed using the following parameters. Reverse transcription was set at 50°C for 40 min for 1 cycle followed by PCR initial heat activation at 95°C for 2 min. The PCR was repeated for 45 cycles at 95°C for 5 s, and at 55°C–59°C for 30 s depended on targets. One triplex PCR was used to detect and quantify PMMoV, BRSV, and N1 (nucleocapsid gene) of SARS-CoV-2, while mtDNA was quantified in a singleplex assay. SARS-CoV-2 N1 and BRSV genes were detected using the primers/probe described by Liu et al. (42, 43). PMMoV primers/probe was described by Marlene et al. (44). For the triplex PCR assay, each 40 μL reaction contained 10 μL of 4× One-step Advanced Probe Master Mix, 0.4 μL of 100× OneStep RT Mix, 2 μL of each 16× primer-probe mix of PMMoV, BRSV, and N1, 5 μL of nucleic acid extract, and 18.6 μL of RNase-free water. For the mtDNA singleplex master mix, each 40 μL reaction contained 10 μL of 4× One-step Advanced Probe Master Mix, 0.4 μL of 100× OneStep RT Mix, 2 μL of 16× primer-probe mix of mtDNA, 5 μL of 1:100 diluted nucleic acid extract, and 22.6 μL of RNase-free water. mtDNA primers/probe was described by Zhu et al. (45). The master mix was pipetted into a QIAGEN QIAcuity 24 well 26 k partition nanoplate.
2.4 Data analysis and statistics
We performed data analysis using R program (version 4.0.1). A logistic regression model was used to study the association between SARS-CoV-2 positivity and other variables including inpatient, outpatient, days after COVID-19 was confirmed, PMMoV, and mtDNA concentration. The Pearson correlation was used to estimate the association between log10-transformed PMMoV and mtDNA concentrations.
3 Results
3.1 Participant description
A total of 42 subjects with confirmed COVID-19 were enrolled in this study, and 155 fecal samples were collected throughout the 42 days follow-up period. Most of these subjects, 61.9% (26/42), were inpatients, and 38.1% (16/42) were outpatients. Most participants manifested severe clinical symptoms if hospitalized (inpatients), while outpatient participants usually had mild symptoms. The self-reported COVID-19 vaccination status indicated that 66.7% (28/42) were vaccinated (received at least one dose of COVID-19 vaccine and had a breakthrough infection) and 33.3% (14/42) were unvaccinated. Among these subjects, only 14.3% (6/42) were ≥65 years old (Table 1).
3.2 COVID-19 clinical symptoms
To compare the clinical course of infections of vaccinated and unvaccinated participants, clinical symptoms relevant to COVID-19 infection were assessed. Cough, diarrhea, fever, loss of smell, and shortness of breath were more frequently observed in unvaccinated subjects than in vaccinated subjects. Frequencies of headache and myalgia fatigue were higher in vaccinated and in unvaccinated groups. When inpatients and outpatients were compared, inpatients were much more likely to report cough, diarrhea, and fever than outpatients while outpatients tended to have more symptoms of headache, loss of smell, myalgia and fatigue. These differences may be caused by the small number of subjects in both groups (Table 2).
3.3 Longitudinal SARS-CoV-2 fecal shedding
The SARS-CoV-2 fecal shedding patterns for each inpatient and outpatient study subject are shown in Figure 1. SARS-CoV-2 RNA was detected in at least one of the fecal samples collected during the sampling period for 73.1% (19/26) of inpatient participants. Most SARS-CoV-2 RNA-positive samples were collected within two weeks of COVID-19 diagnosis. Five inpatients still shed the virus in their stools after 2 weeks, and one inpatient had detectable SARS-CoV-2 RNA in a stool sample at 59 days after COVID-19 diagnosis. For the 71 fecal samples collected from inpatients, 47.9% (34/71) had SARS-CoV-2 N1 gene measurements above the limit of detection of our PCR assay. In contrast, outpatients showed a different fecal shedding pattern. Only 7.1% of 84 fecal samples collected from 16 outpatients were SARS-CoV-2 RNA positive, and all of these came from four study subjects. The low proportion of SARS-CoV-2 positive stool samples from outpatients may be due to delays in sample collection. Interestingly, all four outpatient subjects with positive SARS-CoV-2 stool samples were intermittent shedders and were excreting the virus at three or more weeks after infection was confirmed.
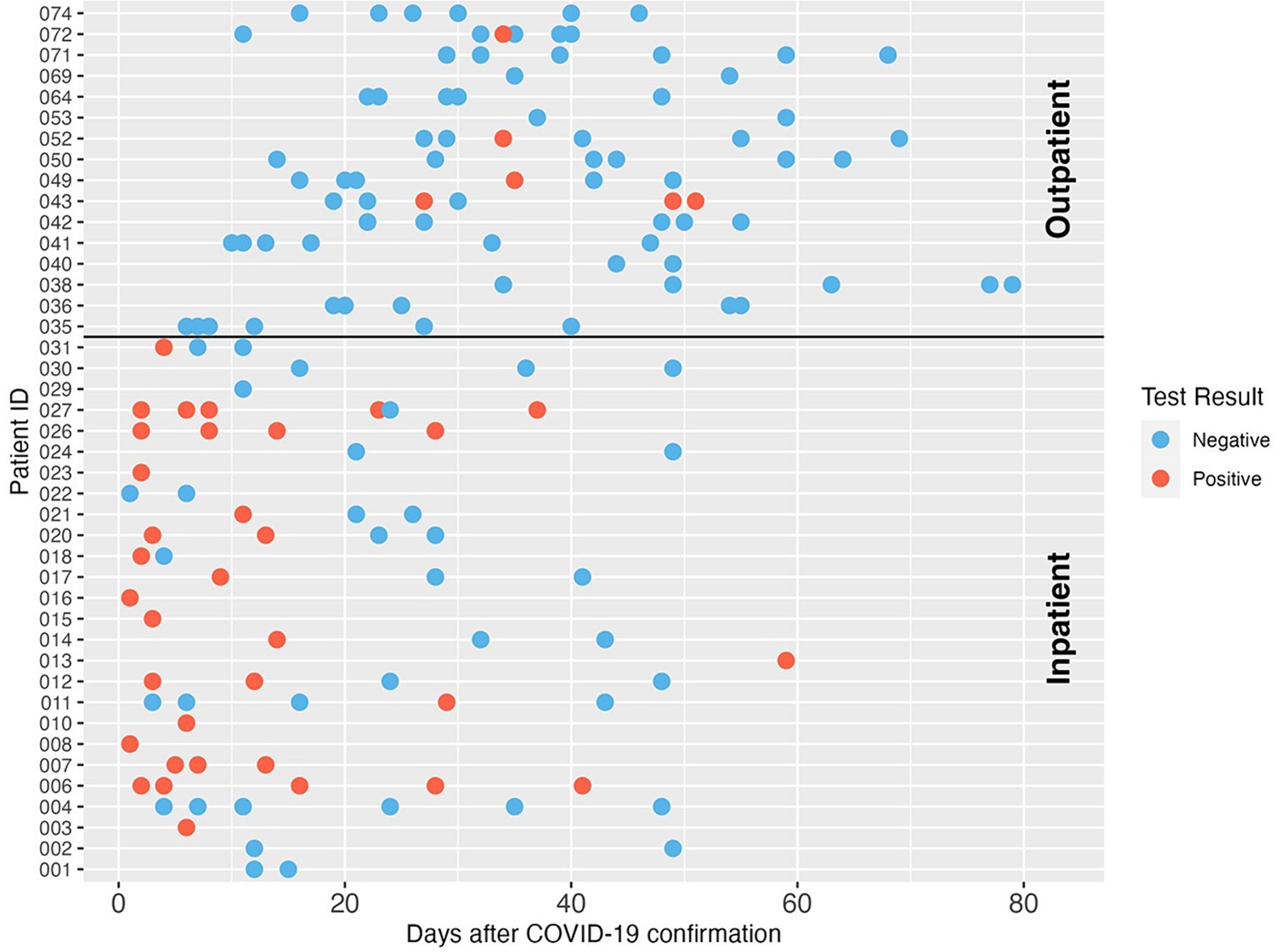
Figure 1. SARS-CoV-2 RNA longitudinal shedding dynamics in stool samples of COVID-19 patients in this study. The patient IDs are shown on the y-axis. The x-axis indicates days after COVID-19 diagnosis was confirmed. Patients 001-031 were enrolled as inpatients, and patients 035-074 were enrolled as outpatients. Three patients with unclear confirmation dates were excluded from this figure. Red circles indicate positive SARS-CoV-2 detection, and blue circles indicate the sample was negative for SARS-CoV-2 RNA by dPCR.
3.4 SARS-CoV-2 RNA concentrations in fecal specimens
Concentrations of SARS-CoV-2 RNA in stool samples ranged from 4.5 × 103 genome copies (GC)/dry gram to 1.19 × 109 GC/dry gram with a median of 1.08 × 105 GC/dry gram. For the 34 fecal samples from inpatients that tested positive for SARS-CoV-2 RNA, the median concentration of SARS-CoV-2 RNA was 1.35 × 105 GC/dry gram. For the six fecal samples from outpatient participants that tested positive for SARS-CoV-2 RNA, the median concentration of SARS-CoV-2 RNA was 9.11 × 103 GC/dry gram (Figure 2, bottom left). Both the SARS-CoV-2 detection rates in stool samples and the mean virus RNA log10 concentrations in stool were significantly different in samples from inpatients versus outpatients (p < 0.001 and p = 0.003, respectively).
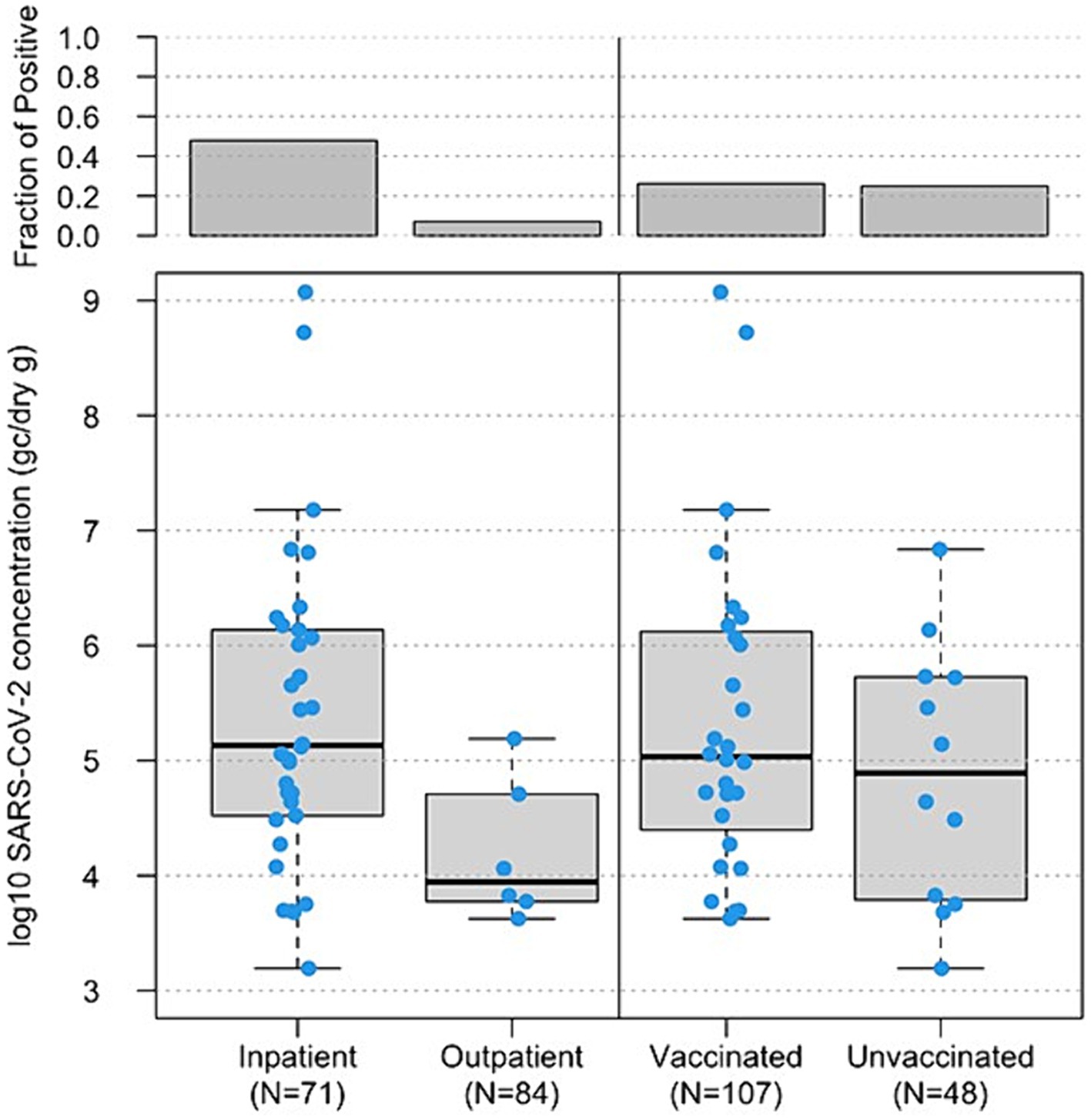
Figure 2. Boxplot of log10-transformed SARS-CoV-2 RNA concentrations (genome copies/dry gram, y-axis) in 155 fecal samples from inpatients vs. outpatients, based on status when enrolled, (bottom left, x-axis) and 146 fecal samples from vaccinated vs. unvaccinated study subjects (bottom right, x-axis). The horizontal lines in boxes denote the 50th percentiles of SARS-CoV-2 RNA concentrations. The top boxes represent the fraction of SARS-CoV-2 RNA positive samples in each group.
3.5 Fecal shedding among vaccinated (breakthrough) and unvaccinated participants
There was little difference in the detection rates and concentrations of SARS-CoV-2 RNA in fecal samples from study subjects with different COVID-19 vaccination status. For the 28 vaccinated participants, 53.6% (15/28) of vaccinated participants shed SARS-CoV-2 in at least one of the fecal specimens collected during the study period, and 26.2% (28/107) fecal samples from vaccinated participants were SARS-CoV-2 RNA positive with a median concentration of 1.08 × 105 GC/dry gram. There were 14 participants who reported that they were unvaccinated, and 8 of them (57.1%) had evidence of SARS-CoV-2 shedding in at least one of the collected fecal samples. Of the 48 fecal samples collected from unvaccinated participants, 25.0% (12/48) fecal samples from unvaccinated participants were positive for SARS-CoV-2 RNA and the median concentration was 9.14 × 104 GC/dry gram (Figure 2, bottom right).
3.6 SARS-CoV-2 variants
In this study, we could not determine the SARS-CoV-2 variants in positive fecal samples. Instead, reported COVID-19 clinical cases (Figure 3A) in Fulton County, Georgia, United States, where we collected wastewater samples were obtained from https://ga-covid19.ondemand.sas.com/docs/ga_covid_data.zip. In addition, the percent of SARS-CoV-2 cases by variant in the Department of Human and Health Services (HHS) region 4 (Figure 3B) were obtained from https://healthdata.gov/dataset/SARS-CoV-2-Variant-Proportions/44cr-xzjd/about_data. This variant information during the fecal sample collection time period in the HHS region 4 represents SARS-CoV-2 variants in our positive fecal samples.
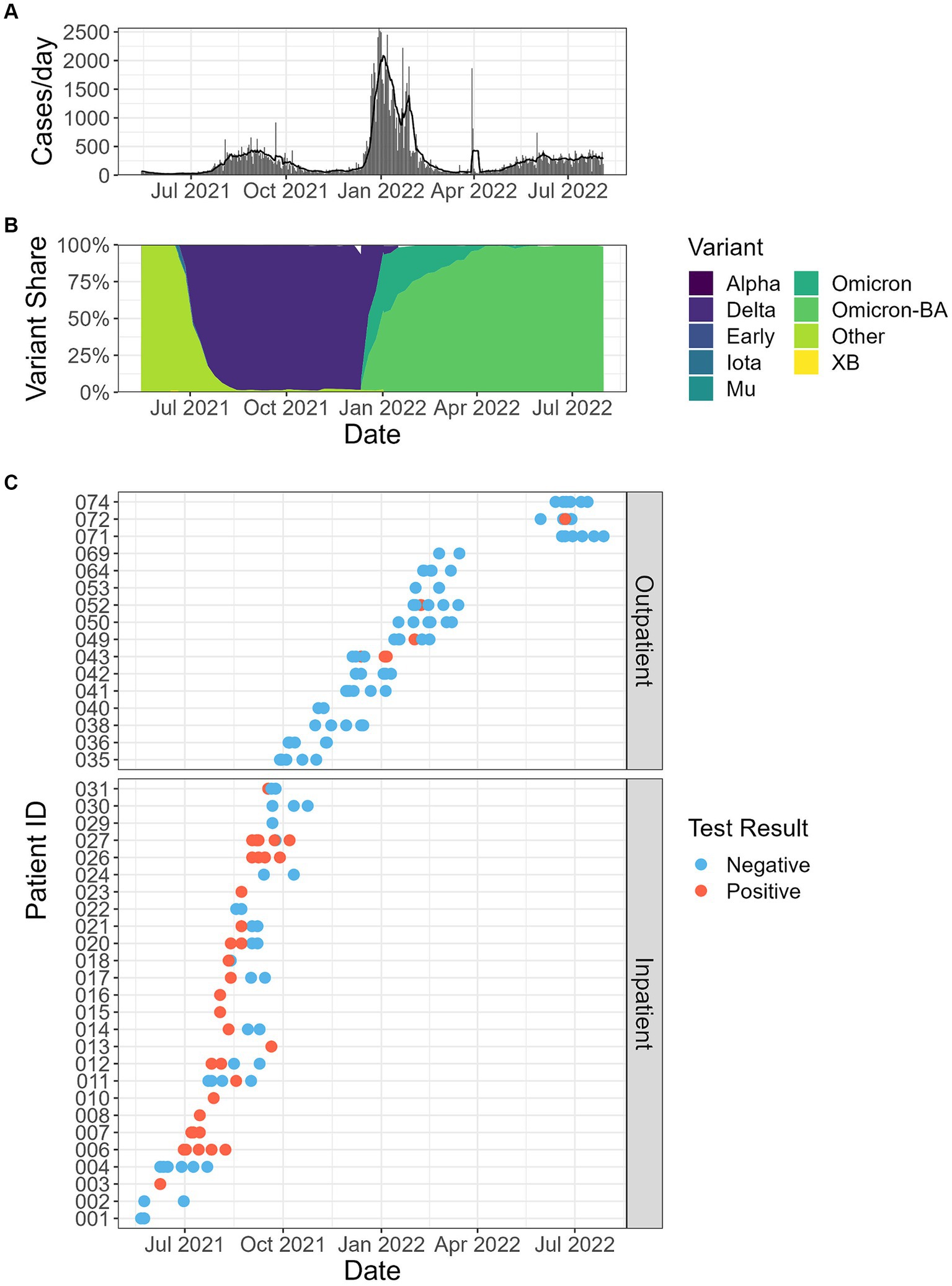
Figure 3. (A) Reported clinical cases in Fulton County, Georgia, USA, where the wastewater samples were collected in this study. Bars represent total counts from each day, and the line reports the 5 days average. (B) The percentage of SARS-CoV-2 variants in the population of HHS (Department of Human and Health Services) region 4. (C) Fecal shedding samples by date and patient, and SARS-CoV-2 RT-qPCR results.
3.7 PMMoV detection in fecal samples
PMMoV was analyzed in each fecal sample by dPCR on the same day as the SARS-CoV-2 dPCR to observe the biological variability of this fecal indicator within and between individuals over the sampling period. Almost all (99.4%, 154/155) of the fecal samples in this study had detectable PMMoV. The median concentration of PMMoV RNA was 1.73 × 107 GC/dry gram. PMMoV RNA concentrations were highly variable over the sampling period within and between the individual study subjects. Some individuals showed relatively consistent concentrations of PMMoV RNA in their longitudinal samples; while for others the PMMoV RNA concentrations varied across several orders of magnitude among all the samples from an individual. The lowest PMMoV concentration was 790 GC/dry gram, and the highest concentration was 3.51 × 109 GC/dry gram (Figure 4).
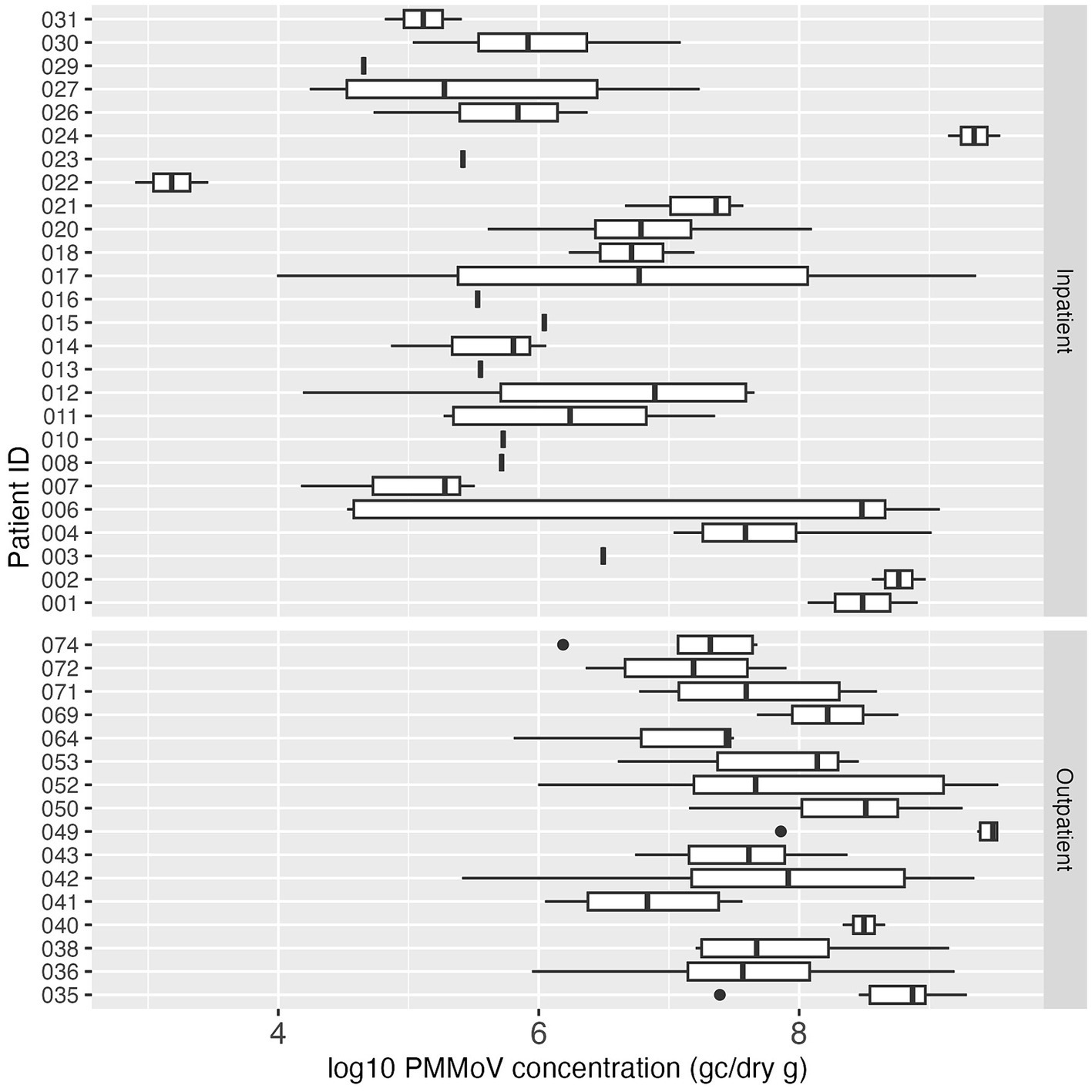
Figure 4. Boxplot of log10-transformed PMMoV concentration (genome copies/dry gram) in inpatient (top) and outpatient (bottom) study subjects. Each box summarizes the range of PMMoV concentrations in multiple fecal samples from an individual, and the vertical line in the box represents the median PMMoV concentration. The vertical line without the box indicates only one sample was tested for PMMoV for that individual.
3.8 Human mtDNA detection in fecal samples
Human mtDNA, another fecal indicator, was analyzed in all the collected fecal samples. All 155 samples (100%) tested positive for mtDNA, and the median concentration of mtDNA in all positive samples was 2.49 × 108 GC/dry gram. The concentration of mtDNA ranged between 8.22 × 106 GC/dry gram and 3.88 × 1010 GC/dry gram. In contrast to PMMoV, the mtDNA concentration in stool was more consistent both between and within the study subjects over the study period (Figure 5).
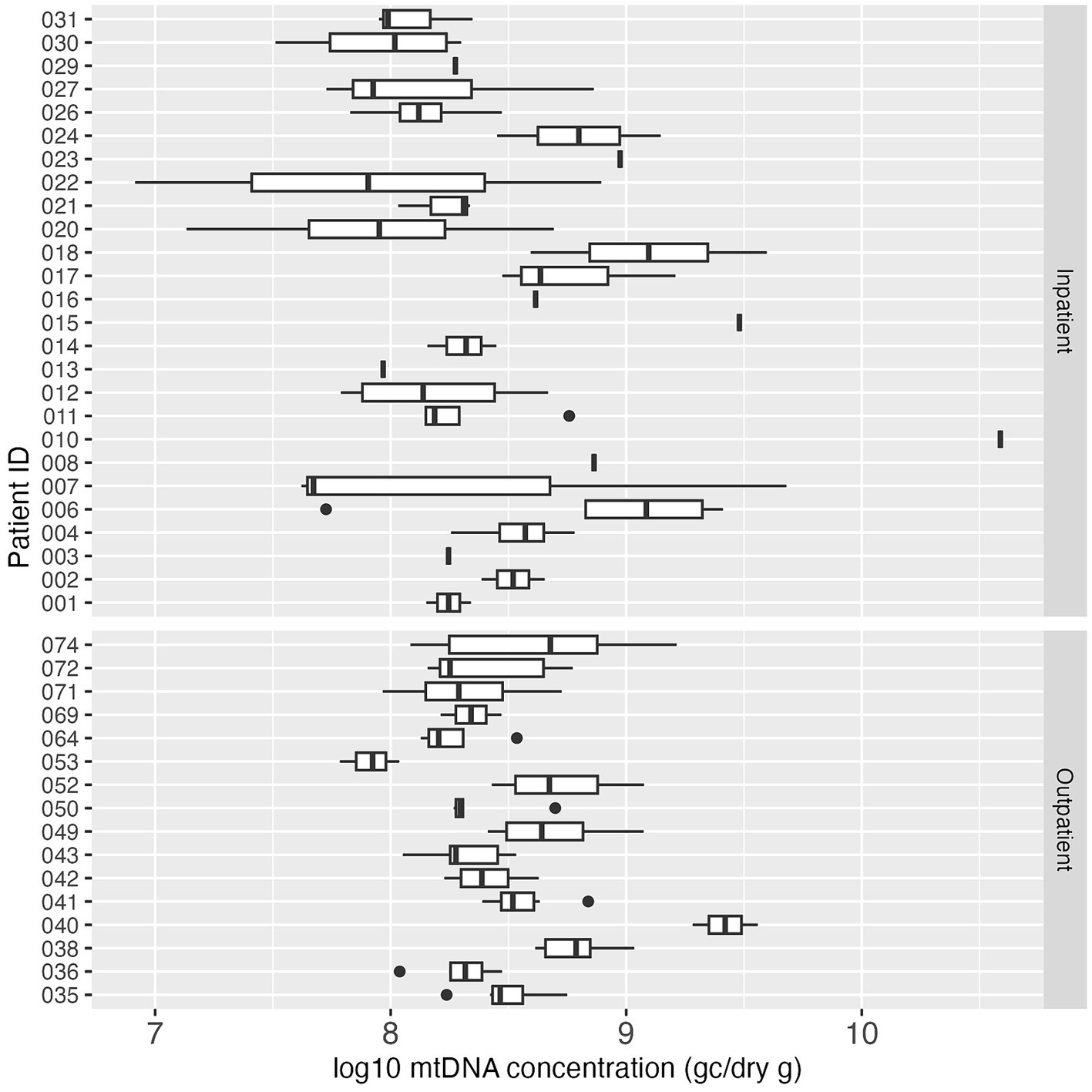
Figure 5. Boxplot of log10-transformed mtDNA concentration (GC/dry gram) in inpatient (top) and outpatient (bottom) study subjects. Each box summarizes the range of mtDNA concentrations in multiple fecal samples from an individual, and the vertical line in the box represents the median mtDNA concentration. The vertical line without the box indicates only one sample was tested for mtDNA for that individual.
3.9 Correlation between PMMoV and mtDNA
Pearson correlation analysis was performed to examine the association between PMMoV and mtDNA concentrations, the two human fecal markers measured in the fecal samples in this study. Figure 6 indicated a weak correlation (p = 0.16) between the concentrations of these two markers. Interestingly, mean log10 PMMoV concentration was significantly higher (p < 0.001) in fecal specimens from outpatients compared to those from inpatients.
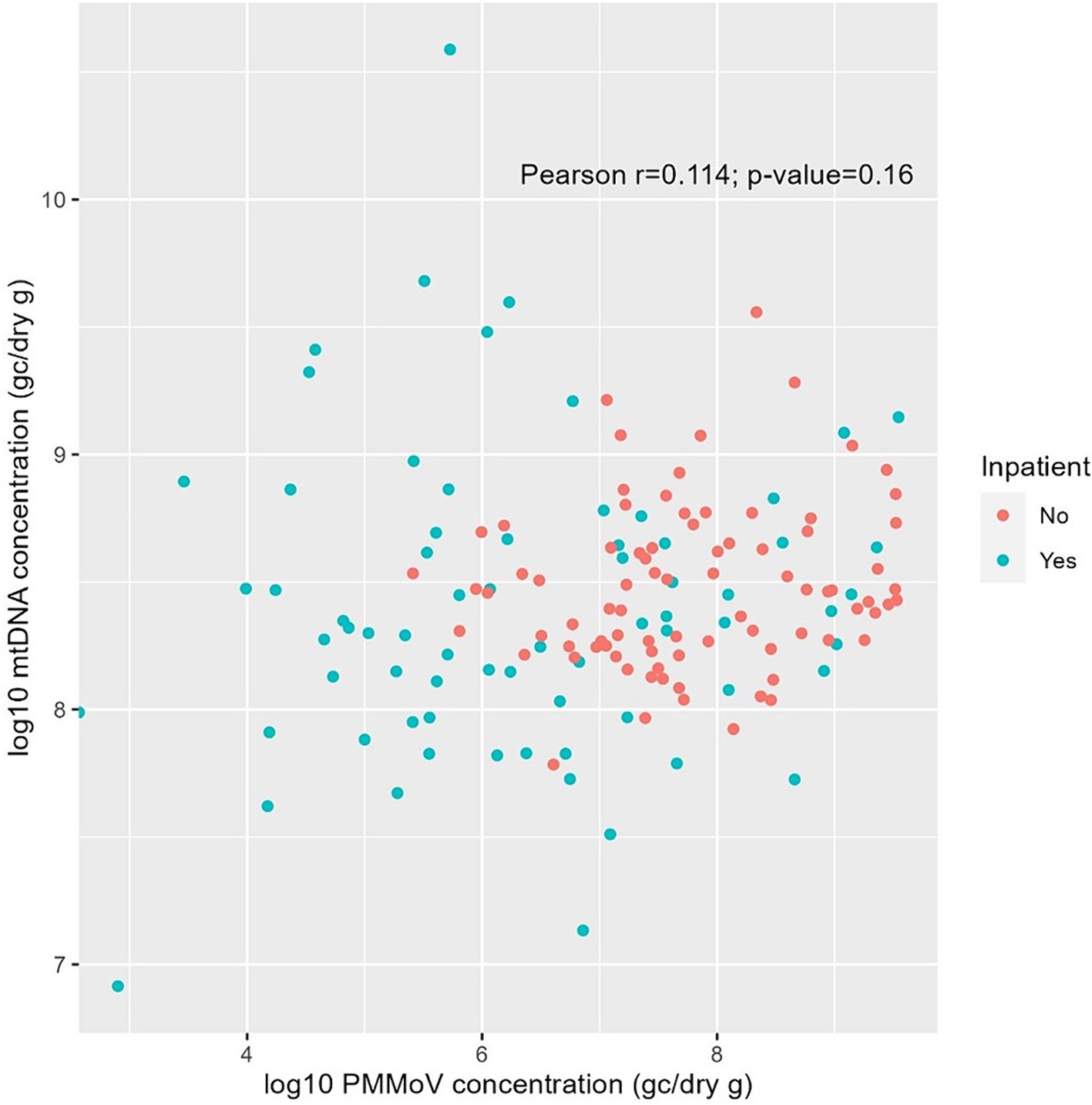
Figure 6. Pearson correlation between log10-transformed PMMoV RNA and mtDNA concentrations (GC/dry gram) from fecal samples of inpatients (green) and outpatients (red) in this study.
4 Discussion
In this study, we describe frequency, duration, and concentration of SARS-CoV-2, PMMoV, and mtDNA shedding in fecal specimens from inpatient and outpatient study subjects with COVID-19 over a 42 days period after the confirmation of SARS-CoV-2 infection. Consistent with other reports (46–48), study subjects who had been vaccinated but had breakthrough infections were less likely to exhibit some clinical symptoms, such as cough, diarrhea, fever, loss of smell, loss of taste, and shortness of breath, compared to unvaccinated study subjects. Inpatient participants were more likely to be shedding SARS-CoV-2 RNA in their fecal specimens and had higher concentrations of the virus in their stool compared to outpatient participants. These results provide important information about the dynamics of the fecal shedding of SARS-CoV-2 and two human fecal indicators. Understanding the presence, magnitude, and duration of these targets of interest is critical for the broad application of WBE and interpretation of WBE results.
Although several cross-sectional studies have reported SARS-CoV-2 fecal shedding in patients with COVID-19 at the early stage of infection, little is known about longitudinal SARS-CoV-2 shedding. In this study, we observed that 73.1% (19/26) inpatients shed SARS-CoV-2 up to three weeks and 19.2% (5/26) inpatients continued to shed viruses beyond day 20; however only 25.0% (4/16) outpatients shed viruses after three weeks of diagnosis. In contrast, the study led by Natarajan et al. (3) included a total of 113 individuals diagnosed with COVID-19 who were followed for up to 10 months. They reported that 49.2% of fecal specimens tested positive for SARS-CoV-2 during the week following diagnosis using PCR-based methods, with the positivity rate declining to 12.7% at 4 months and 3.8% at 7 months after diagnosis. Another study reported the fecal shedding results from 48 SARS-CoV-2 infected individuals and observed that approximately 80% of the fecal samples collected within the first 5 days were positive for SARS-CoV-2, and this positivity rate dropped to 10% at 28 days after symptom onset. A median concentration of three orders GC of magnitude/mg dry weight was reported in positive fecal samples (49), which is one order GC of magnitude higher compared to this study.
SARS-CoV-2 fecal shedding is influenced by viral characteristics, e.g., variants, and host immunity such as pre-existing immunity (vaccination and/or previous infection). Available data indicated that infection with different SARS-CoV-2 variants led to highly distinct infectious viral loads. Jones et al. reported that patients infected with Alpha variant led to approximately 10-fold higher RNA load compared with the ancestral virus (50) while Delta demonstrated elevated infectious virus titer than Alpha (51). However, Omicron was shown to be lower viral loads in infected patients than those infected with Delta (52). Overall, previous vaccination or infection with SARS-CoV-2 has been found to reduce viral loads among vaccinated vs. unvaccinated or infected vs. uninfected individuals (53–56). Vaccination with mRNA or adenoviral vector vaccines led to a reduction of RNA viral load in breakthrough infection with Alpha variant (57). There are limited data on the effect of previous infection on concurrent viral shedding. A prospective study among young adults with seropositive and seronegative IgG against ancestral SARS-CoV-2 demonstrated lower RNA viral load in those previous seropositive individuals than those seronegative individuals against subsequent SARS-CoV-2 infection (55).
Normalizing SARS-CoV-2 concentrations measured in wastewater with a human fecal indicator is a common practice in order to adjust for factors that may contribute to the variability in SARS-CoV-2 concentrations from distinct catchment areas and the recovery of the virus from wastewater with different methods. Among these recommended fecal indicators, PMMoV is widely used. PMMoV is a plant RNA virus associated with pepper products and human diet (27, 37). The presence and magnitude of PMMoV RNA in biological and environmental samples are varied due to the geographic and dietary variations between populations and individuals. These variations may complicate the application of PMMoV as a normalizing indicator in some situations, such as countries with less consumption of plants and pepper products. Although PMMoV has been consistently detected in wastewater and has been proposed as a normalization indicator, there are few reports characterizing PMMoV detection in human fecal samples. Additionally, high concentrations of PMMoV have been detected in non-human fecal samples, such as chickens and seagulls (58, 59), indicating that PMMoV is not human-specific and detection of PMMoV in wastewater may originate from other non-human sources, e.g., livestock and wildlife. Therefore, accurate characterization of the frequency of detection and quantification of PMMoV in human fecal samples provides critical information for better application of this marker as a normalization control in WBE. In this study, PMMoV was detected in 99.4% fecal samples, with a median concentration of 1.73 × 107 GC/dry gram. The PMMoV concentrations measured in this study are similar to those reported publications that one recent study also quantified PMMoV in human stool samples using digital PCR and reported dry mass concentrations (49). In concordance with their results, we observed significant variations of PMMoV concentrations between and within individuals throughout the sampling period. This suggests that PMMoV levels in human fecal materials may be affected by an individual’s daily diet and lifestyle.
Human mtDNA is a human-specific intrinsic genetic marker for fecal source tracking. This marker is abundant in human feces and sewage which makes it a useful indicator of human fecal contamination for environmental microbial research and risk assessment applications (40, 41, 45, 60). There are limited reports on the use of mtDNA as a normalization control in SARS-CoV-2 wastewater studies. In this study, mtDNA was detected in 100% fecal samples, with a median concentration of 2.49 × 108 GC/dry gram. Our mtDNA concentrations are within the range of mtDNA concentrations in human feces reported by other studies (45). Compared to PMMoV detection in human feces, mtDNA was detected in all the fecal specimens, was present at ten-fold higher concentrations, and exhibited little variability between and within individuals. Furthermore, there are no non-human sources of this marker. These characteristics suggest that mtDNA may be better suited for use as a normalization factor for WBE results than PMMoV.
There are several limitations in this study. First, the sample size was small, with 42 confirmed COVID-19 patients enrolled and 155 fecal samples collected. This population was not large enough to allow for stratification for further examination of the effects of some demographic variables on the fecal shedding of SARS-CoV-2. Second, the recruited subjects were from a limited geographic area, basically within the metro Atlanta area of Georgia. Therefore, the sample may not be representative of the US population, and the conclusions may not be generalizable. Third, the fecal samples were collected between March 21, 2021 and July 28, 2022, when the Delta and Omicron variants were prevalent. We do not know the shedding dynamics of other SARS-CoV-2 variants, and the small sample size did not allow us to compare the shedding dynamics of the Delta versus Omicron variants. Fourth, we did not collect the diet information from the patients who might have quite different diet at home compared to the blend food in the hospital, which it is difficult to interpret the PMMoV difference between the inpatients and the outpatients. Fifth, outpatient participants had much lower proportion of SARS-CoV-2 positive fecal specimens than inpatient participants in this study, and surprisingly, all the fecal samples from outpatient study subjects were negative for SARS-CoV-2 within the first three weeks of COVID-19 diagnosis. The low SARS-CoV-2 detection rate in fecal specimens from outpatient participants may be due to the delays between subject enrollment and stool sample collection and possible mishandling during storage and shipment from the participants’ homes to the research laboratory. In contrast, fecal specimens from inpatient participants were collected earlier in the course of infection and stored under optimum conditions until analysis. Despite these limitations, the quantitative measurements of SARS-CoV-2, PMMoV, and mtDNA in longitudinal fecal samples from confirmed COVID-19 patients have significant relevance to our understanding of COVID-19 epidemiology, and the SARS-CoV-2 shedding information addresses a critical knowledge gap for the advancement of WBE and the use of wastewater monitoring data for SARS-CoV-2 to estimate COVID-19 prevalence and incidence in specific catchment populations.
Data availability statement
The raw data supporting the conclusions of this article will be made available by the authors, without undue reservation. The data described in this article are openly available in the Shedding Hub (https://github.com/shedding-hub/shedding-hub), an Open Science Platform.
Ethics statement
The studies involving humans were approved by Emory Institutional Research Board. The studies were conducted in accordance with the local legislation and institutional requirements. The human samples used in this study were acquired from primarily isolated as part of your previous study for which ethical approval was obtained. Written informed consent for participation was not required from the participants or the participants’ legal guardians/next of kin in accordance with the national legislation and institutional requirements.
Author contributions
PL: Conceptualization, Investigation, Methodology, Validation, Writing – original draft, Writing – review & editing. OS: Methodology, Writing – original draft, Data curation. YW: Data curation, Methodology, Writing – original draft. SH: Data curation, Methodology, Writing – original draft, Writing – review & editing. LK: Methodology, Writing – original draft, Writing – review & editing. JI: Methodology, Writing – review & editing. JT: Methodology, Writing – review & editing. SE: Methodology, Writing – review & editing. GA: Methodology, Writing – review & editing. AN: Methodology, Writing – review & editing. EM: Methodology, Writing – review & editing. MW: Methodology, Writing – review & editing. NR: Methodology, Writing – review & editing. CK: Methodology, Writing – review & editing. CM: Methodology, Writing – review & editing.
Funding
The author(s) declare that financial support was received for the research, authorship, and/or publication of this article. This study was supported by the NIH Rapid Acceleration of Diagnostics (RADx) initiative (contract no. 75N92021C00012 to Ceres Nanosciences, Inc.) through a contract from Ceres Nanosciences to Emory University.
Acknowledgments
We are grateful to Salvins J. Strods, Shawn Mulvaney, Chipo Chemoyo J. Baker Afamefuna, and Jenica Patterson with the NIH RADx Initiative, and Ross Dunlap, Robbie Barbero, and Ben Lepene from Ceres Nanosciences, Inc. We are grateful for Peter Teunis in Netherlands for his insights of fecal data analysis.
Conflict of interest
The authors declare that the research was conducted in the absence of any commercial or financial relationships that could be construed as a potential conflict of interest.
Publisher’s note
All claims expressed in this article are solely those of the authors and do not necessarily represent those of their affiliated organizations, or those of the publisher, the editors and the reviewers. Any product that may be evaluated in this article, or claim that may be made by its manufacturer, is not guaranteed or endorsed by the publisher.
References
1. Cheung, KS, Hung, IFN, Chan, PPY, Lung, KC, Tso, E, Liu, R, et al. Gastrointestinal manifestations of SARS-CoV-2 infection and virus load in fecal samples from a Hong Kong cohort: systematic review and Meta-analysis. Gastroenterology. (2020) 159:81–95. doi: 10.1053/j.gastro.2020.03.065
2. Zhou, JQ, Liu, GX, Huang, XL, and Gan, HT. The importance of fecal nucleic acid detection in patients with coronavirus disease (COVID-19): a systematic review and meta-analysis. J Med Virol. (2022) 94:2317–30. doi: 10.1002/jmv.27652
3. Natarajan, A, Zlitni, S, Brooks, EF, Vance, SE, Dahlen, A, Hedlin, H, et al. Gastrointestinal symptoms and fecal shedding of SARS-CoV-2 RNA suggest prolonged gastrointestinal infection. Med. (2022) 3:e9:371–387.e9. doi: 10.1016/j.medj.2022.04.001
4. Khemiri, H, Gdoura, M, Ben Halima, S, Krichen, H, Camma, C, Lorusso, A, et al. SARS-CoV-2 excretion kinetics in nasopharyngeal and stool samples from the pediatric population. Front Med (Lausanne). (2023) 10:1226207. doi: 10.3389/fmed.2023.1226207
5. Yuan, C, Wang, H, Li, K, Tang, A, Dai, Y, Wu, B, et al. SARS-CoV-2 viral shedding characteristics and potential evidence for the priority for faecal specimen testing in diagnosis. PLoS One. (2021) 16:e0247367. doi: 10.1371/journal.pone.0247367
6. Jones, DL, Baluja, MQ, Graham, DW, Corbishley, A, McDonald, JE, Malham, SK, et al. Shedding of SARS-CoV-2 in feces and urine and its potential role in person-to-person transmission and the environment-based spread of COVID-19. Sci Total Environ. (2020) 749:141364. doi: 10.1016/j.scitotenv.2020.141364
7. Cai, J, Wang, X, Zhao, J, Ge, Y, Xu, J, Tian, H, et al. Comparison of clinical and epidemiological characteristics of asymptomatic and symptomatic SARS-CoV-2 infection in children. Virol Sin. (2020) 35:803–10. doi: 10.1007/s12250-020-00312-4
8. Lavezzo, E, Franchin, E, Ciavarella, C, Cuomo-Dannenburg, G, Barzon, L, Del Vecchio, C, et al. Suppression of a SARS-CoV-2 outbreak in the Italian municipality of Vo. Nature. (2020) 584:425–9. doi: 10.1038/s41586-020-2488-1
9. Arons, MM, Hatfield, KM, Reddy, SC, Kimball, A, James, A, Jacobs, JR, et al. Presymptomatic SARS-CoV-2 infections and transmission in a skilled nursing facility. N Engl J Med. (2020) 382:2081–90. doi: 10.1056/NEJMoa2008457
10. Nemudryi, A, Nemudraia, A, Wiegand, T, Surya, K, Buyukyoruk, M, Cicha, C, et al. Temporal detection and phylogenetic assessment of SARS-CoV-2 in municipal wastewater. Cell Rep Med. (2020) 1:100098. doi: 10.1016/j.xcrm.2020.100098
11. Roka, E, Khayer, B, Kis, Z, Kovacs, LB, Schuler, E, Magyar, N, et al. Ahead of the second wave: early warning for COVID-19 by wastewater surveillance in Hungary. Sci Total Environ. (2021) 786:147398. doi: 10.1016/j.scitotenv.2021.147398
12. Li, X, Zhang, S, Sherchan, S, Orive, G, Lertxundi, U, Haramoto, E, et al. Correlation between SARS-CoV-2 RNA concentration in wastewater and COVID-19 cases in community: a systematic review and meta-analysis. J Hazard Mater. (2023) 441:129848. doi: 10.1016/j.jhazmat.2022.129848
13. Lu, E, Ai, Y, Davis, A, Straathof, J, Halloran, K, Hull, N, et al. Wastewater surveillance of SARS-CoV-2 in dormitories as a part of comprehensive university campus COVID-19 monitoring. Environ Res. (2022) 212:113580. doi: 10.1016/j.envres.2022.113580
14. Brooks, YM, Gryskwicz, B, Sidaway, E, Shelley, B, Coroi, L, Downing, M, et al. A case study of a community-organized wastewater surveillance in a small community: correlating weekly reported COVID-19 cases with SARS-CoV-2 RNA concentrations during fall 2020 to summer 2021 in Yarmouth, ME. J Water Health. (2023) 21:329–42. doi: 10.2166/wh.2023.238
15. Acosta, N, Bautista, MA, Hollman, J, McCalder, J, Beaudet, AB, Man, L, et al. A multicenter study investigating SARS-CoV-2 in tertiary-care hospital wastewater. Viral burden correlates with increasing hospitalized cases as well as hospital-associated transmissions and outbreaks. Water Res. (2021) 201:117369. doi: 10.1016/j.watres.2021.117369
16. Ai, Y, Davis, A, Jones, D, Lemeshow, S, Tu, H, He, F, et al. Wastewater SARS-CoV-2 monitoring as a community-level COVID-19 trend tracker and variants in Ohio. United States Sci Total Environ. (2021) 801:149757. doi: 10.1016/j.scitotenv.2021.149757
17. Cluzel, N, Courbariaux, M, Wang, S, Moulin, L, Wurtzer, S, Bertrand, I, et al. A nationwide indicator to smooth and normalize heterogeneous SARS-CoV-2 RNA data in wastewater. Environ Int. (2022) 158:106998. doi: 10.1016/j.envint.2021.106998
18. Wurtzer, S, Levert, M, Dhenain, E, Accrombessi, H, Manco, S, Fagour, N, et al. From alpha to omicron BA.2: new digital RT-PCR approach and challenges for SARS-CoV-2 VOC monitoring and normalization of variant dynamics in wastewater. Sci Total Environ. (2022) 848:157740. doi: 10.1016/j.scitotenv.2022.157740
19. Wurtzer, S, Marechal, V, Mouchel, JM, Maday, Y, Teyssou, R, Richard, E, et al. Evaluation of lockdown effect on SARS-CoV-2 dynamics through viral genome quantification in waste water, greater Paris, France, 5 march to 23 April 2020. Euro Surveill. (2020) 25:e776. doi: 10.2807/1560-7917.ES.2020.25.50.2000776
20. McMahan, CS, Lewis, D, Deaver, JA, Dean, D, Rennert, L, Kalbaugh, CA, et al. Predicting COVID-19 infected individuals in a defined population from wastewater RNA data. ACS ES T Water. (2022) 2:2225–32. doi: 10.1021/acsestwater.2c00105
21. Joseph-Duran, B, Serra-Compte, A, Sarrias, M, Gonzalez, S, Lopez, D, Prats, C, et al. Assessing wastewater-based epidemiology for the prediction of SARS-CoV-2 incidence in Catalonia. Sci Rep. (2022) 12:15073. doi: 10.1038/s41598-022-18518-9
22. McMahan, CS, Self, S, Rennert, L, Kalbaugh, C, Kriebel, D, Graves, D, et al. COVID-19 wastewater epidemiology: a model to estimate infected populations. Lancet Planet Health. (2021) 5:e874–81. doi: 10.1016/S2542-5196(21)00230-8
23. Proverbio, D, Kemp, F, Magni, S, Ogorzaly, L, Cauchie, HM, Goncalves, J, et al. Model-based assessment of COVID-19 epidemic dynamics by wastewater analysis. Sci Total Environ. (2022) 827:154235. doi: 10.1016/j.scitotenv.2022.154235
24. Aninagyei, E, Ayivor-Djanie, R, Gyamfi, J, Owuani, T, Ameke, SL, Kpeli, GS, et al. Faecal shedding of SARS-CoV-2 from patients with asymptomatic and mild COVID-19 without gastrointestinal symptoms in Ghana. BMC Res Notes. (2024) 17:130. doi: 10.1186/s13104-024-06790-z
25. Tiwari, A, Radu, E, Kreuzinger, N, Ahmed, W, and Pitkanen, T. Key considerations for pathogen surveillance in wastewater. Sci Total Environ. (2024) 945:173862. doi: 10.1016/j.scitotenv.2024.173862
26. Holm, RH, Nagarkar, M, Yeager, RA, Talley, D, Chaney, AC, Rai, JP, et al. Surveillance of RNase P, PMMoV, and CrAssphage in wastewater as indicators of human fecal concentration across urban sewer neighborhoods, Kentucky. FEMS Microbes. (2022) 3:1–12. doi: 10.1093/femsmc/xtac003
27. Hamza, H, Rizk, NM, Gad, MA, and Hamza, IA. Pepper mild mottle virus in wastewater in Egypt: a potential indicator of wastewater pollution and the efficiency of the treatment process. Arch Virol. (2019) 164:2707–13. doi: 10.1007/s00705-019-04383-x
28. Chettleburgh, C, Ma, SX, Swinwood-Sky, M, McDougall, H, Kireina, D, Taggar, G, et al. Evaluation of four human-associated fecal biomarkers in wastewater in southern Ontario. Sci Total Environ. (2023) 904:166542. doi: 10.1016/j.scitotenv.2023.166542
29. He, Z, Dong, L, Zhu, P, Zhang, Z, Xu, T, Zhang, D, et al. Nano-scale analysis of uranium release behavior from river sediment in the Ili basin. Water Res. (2022) 227:119321. doi: 10.1016/j.watres.2022.119321
30. Greenwald, HD, Kennedy, LC, Hinkle, A, Whitney, ON, Fan, VB, Crits-Christoph, A, et al. Tools for interpretation of wastewater SARS-CoV-2 temporal and spatial trends demonstrated with data collected in the San Francisco Bay Area. Water Res X. (2021) 12:100111. doi: 10.1016/j.wroa.2021.100111
31. Crank, K, Li, X, North, D, Ferraro, GB, Iaconelli, M, Mancini, P, et al. CrAssphage abundance and correlation with molecular viral markers in Italian wastewater. Water Res. (2020) 184:116161. doi: 10.1016/j.watres.2020.116161
32. Barrantes, K, Chacon-Jimenez, L, Rivera-Montero, L, Segura-Villalta, A, Badilla-Aguilar, A, Alfaro-Arrieta, E, et al. Challenges detecting SARS-CoV-2 in Costa Rican domestic wastewater and river water. Sci Total Environ. (2023) 897:165393. doi: 10.1016/j.scitotenv.2023.165393
33. Li, Y, Ash, KT, Joyner, DC, Williams, DE, Alamilla, I, McKay, PJ, et al. Evaluating various composite sampling modes for detecting pathogenic SARS-CoV-2 virus in raw sewage. Front Microbiol. (2023) 14:1305967. doi: 10.3389/fmicb.2023.1305967
34. Ahmed, W, Bivins, A, Payyappat, S, Cassidy, M, Harrison, N, and Besley, C. Distribution of human fecal marker genes and their association with pathogenic viruses in untreated wastewater determined using quantitative PCR. Water Res. (2022) 226:119093. doi: 10.1016/j.watres.2022.119093
35. Monteiro, S, Machado-Moreira, B, Linke, R, Blanch, AR, Balleste, E, Mendez, J, et al. Performance of bacterial and mitochondrial qPCR source tracking methods: a European multi-center study. Int J Hyg Environ Health. (2023) 253:114241. doi: 10.1016/j.ijheh.2023.114241
36. Ragot, R, and Villemur, R. Influence of temperature and water quality on the persistence of human mitochondrial DNA, human Hf183 bacteroidales, fecal coliforms and enterococci in surface water in human fecal source tracking context. Sci Total Environ. (2022) 838:156025. doi: 10.1016/j.scitotenv.2022.156025
37. Zhang, T, Breitbart, M, Lee, WH, Run, JQ, Wei, CL, Soh, SW, et al. RNA viral community in human feces: prevalence of plant pathogenic viruses. PLoS Biol. (2006) 4:e3. doi: 10.1371/journal.pbio.0040003
38. Maal-Bared, R, Qiu, Y, Li, Q, Gao, T, Hrudey, SE, Bhavanam, S, et al. Does normalization of SARS-CoV-2 concentrations by pepper mild mottle virus improve correlations and lead time between wastewater surveillance and clinical data in Alberta (Canada): comparing twelve SARS-CoV-2 normalization approaches. Sci Total Environ. (2023) 856:158964. doi: 10.1016/j.scitotenv.2022.158964
39. Hajj-Mohamad, M, Hachad, M, Deschamps, G, Sauve, S, Villemur, R, Blais, MA, et al. Fecal contamination of storm sewers: evaluating wastewater micropollutants, human-specific bacteroides 16S rRNA, and mitochondrial DNA genetic markers as alternative indicators of sewer cross connections. Sci Total Environ. (2019) 659:548–60. doi: 10.1016/j.scitotenv.2018.12.378
40. Zhu, K, Suttner, B, Pickering, A, Konstantinidis, KT, and Brown, J. A novel droplet digital PCR human mtDNA assay for fecal source tracking. Water Res. (2020) 183:116085. doi: 10.1016/j.watres.2020.116085
41. Tanvir Pasha, ABM, Hinojosa, J, Phan, D, Lopez, A, and Kapoor, V. Detection of human fecal pollution in environmental waters using human mitochondrial DNA and correlation with general and human-associated fecal genetic markers. J Water Health. (2020) 18:8–18. doi: 10.2166/wh.2019.197
42. Liu, P, Ibaraki, M, VanTassell, J, Geith, K, Cavallo, M, Kann, R, et al. A sensitive, simple, and low-cost method for COVID-19 wastewater surveillance at an institutional level. Sci Total Environ. (2022) 807:151047. doi: 10.1016/j.scitotenv.2021.151047
43. Boxus, M, Letellier, C, and Kerkhofs, P. Real time RT-PCR for the detection and quantitation of bovine respiratory syncytial virus. J Virol Methods. (2005) 125:125–30. doi: 10.1016/j.jviromet.2005.01.008
44. Simpson, A, Topol, A, White, BJ, Wolfe, MK, Wigginton, KR, and Boehm, AB. Effect of storage conditions on SARS-CoV-2 RNA quantification in wastewater solids. PeerJ. (2021) 9:e11933. doi: 10.7717/peerj.11933.eCollection2021
45. Zhu, KJ, Suttner, B, Knee, J, Capone, D, Moe, CL, Stauber, CE, et al. Elevated fecal mitochondrial DNA from symptomatic norovirus infections suggests potential health relevance of human mitochondrial DNA in fecal source tracking. Environ Sci Technol Lett. (2022) 9:543–50. doi: 10.1021/acs.estlett.2c00140
46. Bajpai, J, Kant, S, Verma, A, Patwa, AK, Atam, V, Chaudhary, SC, et al. The severity of COVID 19 pneumonia in vaccinated vs. non-vaccinated patients in the second wave: an experience from a tertiary Care Center in India. Cureus. (2022) 14:e25378. doi: 10.7759/cureus.25378
47. Nakakubo, S, Kishida, N, Okuda, K, Kamada, K, Iwama, M, Suzuki, M, et al. Associations of COVID-19 symptoms with omicron subvariants BA.2 and BA.5, host status, and clinical outcomes in Japan: a registry-based observational study. Lancet Infect Dis. (2023) 23:1244–56. doi: 10.1016/S1473-3099(23)00271-2
48. Tian, W, Ren, X, Han, M, Zhang, Y, Gao, X, Chen, Z, et al. Epidemiological and clinical characteristics of vaccinated COVID-19 patients: a meta-analysis and systematic review. Int J Immunopathol Pharmacol. (2022) 36:3946320221141802. doi: 10.1177/03946320221141802
49. Arts, PJ, Kelly, JD, Midgley, CM, Anglin, K, Lu, S, Abedi, GR, et al. Longitudinal and quantitative fecal shedding dynamics of SARS-CoV-2, pepper mild mottle virus, and crAssphage. mSphere. (2023) 8:e0013223. doi: 10.1128/msphere.00132-23
50. Jones, TC, Biele, G, Muhlemann, B, Veith, T, Schneider, J, Beheim-Schwarzbach, J, et al. Estimating infectiousness throughout SARS-CoV-2 infection course. Science. (2021) 373:5273. doi: 10.1126/science.abi5273
51. Despres, HW, Mills, MG, Shirley, DJ, Schmidt, MM, Huang, ML, Roychoudhury, P, et al. Measuring infectious SARS-CoV-2 in clinical samples reveals a higher viral titer:RNA ratio for Delta and epsilon vs. alpha variants. Proc Natl Acad Sci. (2022) 119:518119. doi: 10.1073/pnas.2116518119
52. Puhach, O, Adea, K, Hulo, N, Sattonnet, P, Genecand, C, Iten, A, et al. Infectious viral load in unvaccinated and vaccinated individuals infected with ancestral, Delta or omicron SARS-CoV-2. Nat Med. (2022) 28:1491–500. doi: 10.1038/s41591-022-01816-0
53. Pouwels, KB, Pritchard, E, Matthews, PC, Stoesser, N, Eyre, DW, Vihta, KD, et al. Effect of Delta variant on viral burden and vaccine effectiveness against new SARS-CoV-2 infections in the UK. Nat Med. (2021) 27:2127–35. doi: 10.1038/s41591-021-01548-7
54. Singanayagam, A, Hakki, S, Dunning, J, Madon, KJ, Crone, MA, Koycheva, A, et al. Community transmission and viral load kinetics of the SARS-CoV-2 delta (B.1.617.2) variant in vaccinated and unvaccinated individuals in the UK: a prospective, longitudinal, cohort study. Lancet Infect Dis. (2022) 22:183–95. doi: 10.1016/S1473-3099(21)00648-4
55. Letizia, AG, Ge, Y, Vangeti, S, Goforth, C, Weir, DL, Kuzmina, NA, et al. SARS-CoV-2 seropositivity and subsequent infection risk in healthy young adults: a prospective cohort study. Lancet Respir Med. (2021) 9:712–20. doi: 10.1016/S2213-2600(21)00158-2
56. Armas, F, Chandra, F, Lee, WL, Gu, X, Chen, H, Xiao, A, et al. Contextualizing wastewater-based surveillance in the COVID-19 vaccination era. Environ Int. (2023) 171:107718. doi: 10.1016/j.envint.2022.107718
57. Emary, KRW, Golubchik, T, Aley, PK, Ariani, CV, Angus, B, Bibi, S, et al. Efficacy of ChAdOx1 nCoV-19 (AZD1222) vaccine against SARS-CoV-2 variant of concern 202012/01 (B.1.1.7): an exploratory analysis of a randomised controlled trial. Lancet. (2021) 397:1351–62. doi: 10.1016/S0140-6736(21)00628-0
58. Malla, B, Makise, K, Nakaya, K, Mochizuki, T, Yamada, T, and Haramoto, E. Evaluation of human- and animal-specific viral markers and application of CrAssphage, pepper mild mottle virus, and tobacco mosaic virus as potential fecal pollution markers to river water in Japan. Food Environ Virol. (2019) 11:446–52. doi: 10.1007/s12560-019-09398-w
59. Rosario, K, Symonds, EM, Sinigalliano, C, Stewart, J, and Breitbart, M. Pepper mild mottle virus as an indicator of fecal pollution. Appl Environ Microbiol. (2009) 75:7261–7. doi: 10.1128/AEM.00410-09
Keywords: SARS-CoV-2, COVID-19, longitudinal, fecal shedding, PMMoV, mtDNA
Citation: Liu P, Sablon O, Wang Y, Hilton SP, Khalil L, Ingersoll JM, Truell J, Edupuganti S, Alaaeddine G, Naji A, Monarrez E, Wolfe M, Rouphael N, Kraft C and Moe CL (2024) Longitudinal fecal shedding of SARS-CoV-2, pepper mild mottle virus, and human mitochondrial DNA in COVID-19 patients. Front. Med. 11:1417967. doi: 10.3389/fmed.2024.1417967
Edited by:
Matthew D. Moore, University of Massachusetts Amherst, United StatesReviewed by:
Mats Leifels, Karl Landsteiner University of Health Sciences, AustriaAnanda Tiwari, University of Helsinki, Finland
Copyright © 2024 Liu, Sablon, Wang, Hilton, Khalil, Ingersoll, Truell, Edupuganti, Alaaeddine, Naji, Monarrez, Wolfe, Rouphael, Kraft and Moe. This is an open-access article distributed under the terms of the Creative Commons Attribution License (CC BY). The use, distribution or reproduction in other forums is permitted, provided the original author(s) and the copyright owner(s) are credited and that the original publication in this journal is cited, in accordance with accepted academic practice. No use, distribution or reproduction is permitted which does not comply with these terms.
*Correspondence: Pengbo Liu, cGxpdTVAZW1vcnkuZWR1; Christine L. Moe, Y2xtb2VAZW1vcnkuZWR1
†These authors share senior authorship