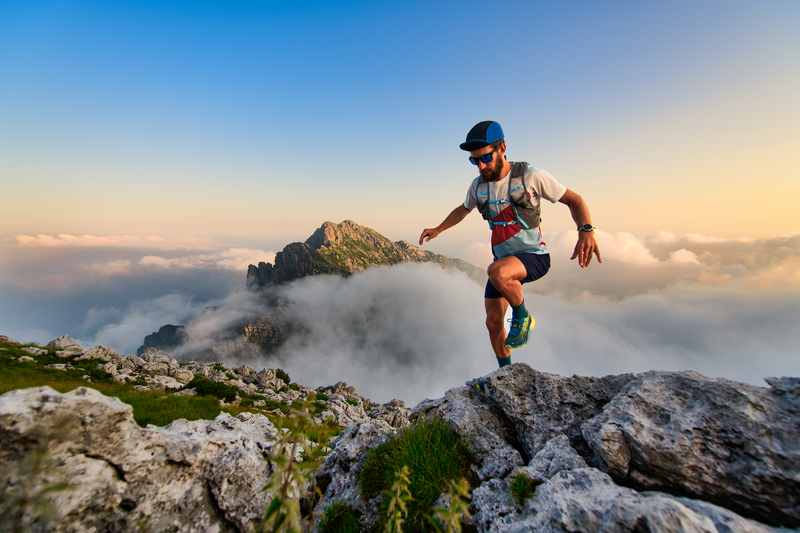
95% of researchers rate our articles as excellent or good
Learn more about the work of our research integrity team to safeguard the quality of each article we publish.
Find out more
MINI REVIEW article
Front. Med. , 16 April 2024
Sec. Gene and Cell Therapy
Volume 11 - 2024 | https://doi.org/10.3389/fmed.2024.1386683
This article is part of the Research Topic The Future of Modern Medicine: Cells, Scaffolds, and Biofactors View all 6 articles
Neuro bone tissue engineering is a multidisciplinary field that combines both principles of neurobiology and bone tissue engineering to develop innovative strategies for repairing and regenerating injured bone tissues. Despite the fact that regeneration and development are considered two distinct biological processes, yet regeneration can be considered the reactivation of development in later life stages to restore missing tissues. It is noteworthy that the regeneration capabilities are distinct and vary from one organism to another (teleost fishes, hydra, humans), or even in the same organism can vary dependent on the injured tissue itself (Human central nervous system vs. peripheral nervous system). The skeletal tissue is highly innervated, peripheral nervous system plays a role in conveying the signals and connecting the central nervous system with the peripheral organs, moreover it has been shown that they play an important role in tissue regeneration. Their regeneration role is conveyed by the different cells' resident in it and in its endoneurium (fibroblasts, microphages, vasculature associated cells, and Schwann cells) these cells secrete various growth factors (NGF, BDNF, GDNF, NT-3, and bFGF) that contribute to the regenerative phenotype. The peripheral nervous system and central nervous system synchronize together in regulating bone homeostasis and regeneration through neurogenic factors and neural circuits. Receptors of important central nervous system peptides such as Serotonin, Leptin, Semaphorins, and BDNF are expressed in bone tissue playing a role in bone homeostasis, metabolism and regeneration. This review will highlight the crosstalk between peripheral nerves and bone in the developmental stages as well as in regeneration and different neuro-bone tissue engineering strategies for repairing severe bone injuries.
Bone is a remarkable tissue that plays a vital role in the human body. It provides protection, support, and movement facility. Structurally bone consists of two main components: organic matrix (e.g., collagen), and inorganic minerals (e.g., calcium and phosphate) (1). There is a rich innervation of sensory and sympathetic nerve fibers in conjunction with the bone vascular system. Peripheral nerve fiber subtypes have a specific function within the bone (2). However, it has recently begun to be in the spotlight on the regulatory role of the bone and nervous system, which has been examined for many years (3–6).
Tissue engineering is a rapidly advancing field that holds great promise for treating various diseases and injuries (7). One area in which tissue engineering has shown significant potential is bone-nerve regeneration. Bone-nerve defects resulting from trauma, disease, or congenital abnormalities can be debilitating and difficult to treat using conventional methods. Due to that, with the advent of tissue engineering techniques, new possibilities have emerged for bone-nerve repair and regeneration. Crosstalk between bone and nerve systems has attracted the researcher's attention in different fields, such as clinical medicine, basic medicine, and biomaterials. While much progress has been made in developing biomaterials and scaffolds that mimic the structure of bone, one crucial aspect that cannot be overlooked is the role of nerves in this process (8, 9).
Nerves play a vital role in bone regeneration by providing sensory input, controlling blood flow, and facilitating cells communication. They are responsible for transmitting signals that regulate cell proliferation, differentiation, and migration. Without proper innervation, tissue-engineered bones may lack functionality and fail to integrate with surrounding tissues (4, 10). In addition, nerves also contribute to pain perception during the healing process (11). Understanding how nerves interact with tissue-engineered constructs can help researchers develop new strategies to minimize pain and enhance patient comfort during recovery. To achieve successful nerve integration into tissue-engineered bones, scientists have explored various approaches such as incorporating nerve growth factors into scaffolds or utilizing stem cells capable of differentiating into both neuronal and osteogenic lineages. Therefore, this study summarizes the relationship between bone-nerve factors and functions, and their applications in bone-neuro tissue engineering (Figure 1).
Previously the function of the highly innervations in bones was just limited to pain perception, studies on denervated limbs that showed impaired bone development has proven the role of nervous system in bone development (12).
Structurally long bones are divided into three parts: the diaphysis, the metaphysis and the epiphysis, they are ensheathed by a thin connective tissue known as the periosteum, within which the majority of blood vessels and nerve fibers exist. Innervations are found in the bone marrow and the traverse nutrient canals in mineralized bone matrix as well (13).
Early studies of peripheral innervation to bones showed that nerve fibers start being visible at early embryonic stages, in the perichondrium of long bones, TrkA+ sensory fibers are visible at E14.5 in mouse embryo (14), while by E21 PGP9.5+ and GAP43+ nerve are visible in rat embryo (15). In the periosteum and bone marrow of the diaphysis CGRP+ sensory afferent fibers are visible at P3 (15). Autonomic fibers first appear in long bone postnatally. Sympathetic NPY+ nerve fibers are first visible in the bone marrow of the mouse femur and tibia at P6. Both NPY+ and TH+ fibers are evident in perichondrium of the epiphysis and the cartilage canals at P10 (12, 14).
The nervous system is involved in bone growth and limb development where sensory and autonomic innervation play a crucial role in bone formation, a study by Tomlinson has shown that NGF expression in the developing endochondral bone coincides with vascularization and osteochondral progenitor cell expansion. Inhibition of TrkA signaling or deletion of NGF in perichondral osteochondral precursor cells over this time frame disrupts ossification of the primary and secondary ossification centers and impairs postnatal bone mass and length, thus proofing that NGF is essential for bone progenitor cell differentiation and mineralization (16). Another study showed that the sensory neuropeptide calcitonin gene-related peptide (CGRP) act at earlier stages in osteoclast development and inhibits osteoclastic bone resorption and enhances osteoblastic bone formation (17). Neuropeptide Y (NPY) is involved in the regulations of bone metabolism and homeostasis, it can stimulate proliferation, migration, promote osteoblastic differentiation and play crucial roles in the bone development (18). Indeed, in a study with global NPY deletion male mice had shown a smaller femoral cortical cross-sectional area (−12%) and reduced bone strength (−18%) while female mice had shown no alterations in bone mass, suggesting a sex-specific effect (19).
A study by Kong et al. uncovered a novel brain-skeleton axis involving NSCs in the mammalian brain, demonstrating the role of bone regeneration through perilipin 5 (PLIN5)-driven lipid metabolism modulation. This study found that the homocysteine (Hcy) negatively affected the osteogenic and angiogenic processes, while the NSC-Exo restored these impairments. Furthermore, the mecobalamin (a neurotrophic drug) enhanced the protective effects of NSC-Exo by increasing the PLIN5 expression. On the other hand, mechanistically, the NSC-derived PLIN5 reversed Hcy-induced lipid metabolism imbalance and aberrant lipid droplet accumulation through lipophagy-dependent intracellular lipolysis. This effects of PLIN5-deriven endocrine play a role on new bone formation and vascular reconstruction in models with elevated homocysteine and high-fat diet through intracerebroventricular administration of mecobalamin and/or AAV-shPlin5 (20).
Skeletal interoception refers to the perception and interpretation of internal signals that are arising from skeletal system. This sensory mechanism involves the mechanical changes, biochemical signals, and proprioceptive information within bones and joints (21, 22). However, it was known that the interoception was associated with visceral process, and recent research has showed the importance of skeletal interoception in understanding the dynamic interactions between bones and nervous system (23).
Bone regeneration is a complex process involving different molecular mechanisms with numerous complicated interactions through stem cell regulation, cytokines, peptide, hormones, and environmental factors (4, 24, 25). Among these many regulatory factors, the neural regulation of bone regeneration is attracting many interests. Previous studies have been described the rich nerve network that distributed in all types of bone. The nervous system regulates bone regeneration by targeting two basic functional cell types, osteoblast, and osteoblasts. These cells are responsible in skeletal model changes, bone growth and remodeling (26). The nerve fibers that are spread ion the bone can transmit nerve signals to muscle and tendon tissue which can influence the compression or stretching of the bone through different factors such as mechanical environment (27). To achieve successful nerve growth towered regenerated bones, cells, scaffolds, and biofactors with specific properties are required.
The interactions process between nerve and bone cells involves series of cellular properties that work in harmony to ensure successful bone-nerve regeneration. These properties include cell adhesion, cell signaling, and cell migration. Cell adhesion allows cells to stick together and form cohesive structure. In the context of nerve growth toward regenerated bones, cell adhesion plays a vital role in guiding nerves along the desired path. By adhering to each other and forming bridges, cells create a scaffold for nerve fibers to grow upon (28). In cell signaling during nerve regeneration, specific signaling molecules are released at the site of bone regeneration. These molecules act as guidance for growing nerves directing them toward the regenerating bone tissue (10, 29). In addition, certain type of cells migrates toward the regenerating bone tissue and align themselves along its surface. This alignment provides physical guidance for growing nerves and helps them navigate their way toward the desired destination (28, 30).
Stem cell, especially mesenchymal stem cell (MSC) can be isolated from a variety of tissues such as bone marrow, adipose tissue, and the synovial fluid. MSC has a unique feature due to its ability to differentiate into different cell types such as osteoblast and chondrocyte (31).
Scaffolds with specific structures can provide support and guidance for cell growth and tissue regeneration. They can be made from various materials, such as metals, polymers, or ceramics, which possess specific properties to promote bone-nerve growth (7, 32). For instance, the scaffold's surface topography can be engineered to mimic the natural extracellular matrix, providing cues for bone-nerve cells to align and extend along desired pathways. There are specific requirements the scaffolds need to meet for successful bone-nerve regeneration: (1) Good biocompatibility that facilities the bone and nerve adhesion and growth, (2) suitable mechanical properties where the scaffold should have adequate stiffness to withstand physiological loads and prevent collapse while allowing for cellular infiltration and nutrient diffusion, (3) appropriate elasticity to mimic the surrounding tissues' mechanical properties and facilitating proper integration with the host bone, (4) good bone or nerve conductivity and inductivity, which help bone or nerve to deposit on the material surface and helps recruit stem cells to induce the osteogenic and neurogenic differentiation, and (5) control the release of bioactive factors (32–34).
Various types of scaffolds have been developed with different properties and advantages. One commonly used of scaffold is the natural scaffolds that is derived from biological sources such as collagen or decellularized extracellular matrix (ECM). These types of scaffolds closely mimic the natural composition, which making them highly compatible. However, the mechanical properties of natural scaffolds may not be optimal foe load-bearing applications. On the other hand, the synthetic scaffolds are fabricated using synthetic materials such as metals, ceramic, or polymers. These materials can be engineered to possess specific mechanical properties, that allowing for better control over their degradation rate and strength. However, they may lack the necessary bioactivity for promoting cell adhesion and differentiation (7, 35, 36).
Hybrid scaffold is a combination of both natural and synthetic materials to harness the advantages of both types. By reinforcing natural scaffolds with synthetic materials, or incorporating bioactive components into synthetic scaffold, the hybrid scaffolds can provide an optimal microenvironment for tissue engineering (36, 37).
Biofactors such as growth factors play a crucial role in guiding nerve growth toward regenerated bones. Nerve regeneration is a complex process that requires the coordination of various cellular and molecular events (38). The interaction between nerves and bones is essential for proper bone healing and regeneration. During nerve regeneration, growth factors attract nerve cells toward the site of injury or bone defect. This migration is essential for establishing connections between nerves and bones, allowing for good communication and function (39). Furthermore, growth factors stimulate the proliferation of nerve cells. By promoting cell division, these factors increase the number of nerve cells available for regeneration which enhances the chances of successful nerve regrowth toward the regenerated bones (10). In addition, growth factors also have a role in promoting angiogenesis, which is the formation of new blood vessels. Blood vessels are essential for delivering oxygen and nutrients to regenerating tissues, including nerves and bones. By stimulating angiogenesis, growth factors ensure an adequate blood supply to support nerve growth toward regenerated bones (40, 41). Moreover, growth factors can modulate the expression of specific genes involved in nerve regeneration processes. They can upregulating genes responsible for axonal guidance and outgrowth while downregulating genes that inhibit nerve regrowth (42).
One of the most important factors for bone regeneration is transforming growth factor beta (TGF-β). TGF-β is able to stimulate the MSCs differentiation into osteoblast that are responsible for bone formation. It also plays a role on stimulating the ECM proteins production that are necessary for bone repair Transforming growth factor-β in stem cells and tissue homeostasis (43). Another significant growth factors are bone morphogenic proteins (BMPs), which are a group of GFs that induce osteogenesis and stimulate bone formation. BMPs play a crucial role in initiating the early stages of bone repair by attracting MSCs to differentiate into osteoblasts (44). Insulin-like growth factor (IGF) is another important GF that enhances cell proliferation and differentiation. It stimulates osteoblast activity which leads to increase bone formation during bone healing process (45). Vascular endothelial growth factor (VEGF) is essential for angiogenesis, the new blood vessels formation. Adequate blood supply is essential for delivering oxygen and nutrients to the site of injury to promote bone healing (46) (Table 1).
Nerve regeneration is a complex process in which several morphological and molecular changes occurs in the proximal and distal nerve stumps, in response to nerve injury various neurotrophins, neurotransmitters and neuropeptides are released that synergize together and play different roles in nerve and bone regeneration (Table 1), they exert their action by modulating several pathways including Wnt, Erk1/2 and AKT pathways Nerve Flex modulation by exercise training and mechanical loading positively affect nerve and bone regeneration by increasing the expression of NGF and Wnt signaling activation (72).
Nerve Growth Factor (NGF) is a member of neurotrophins family playing an important role in growth and survival, other members are brain derived neurotrophic factor (BDNF), neurotrophin-3 (NT-3), and neurotrophin 4/5 (NT-4/5). NGF was first discovered by Rita Levi-Montalcini in the 1950s, and this work eventually led to the Nobel Prize in Physiology or Medicine in 1986 (73). NGF biological action is mediated by binding and activating receptor tyrosine kinase trkA or a transmembrane glycoprotein pan-neurotrophin receptor p75NTR (74). Although these factors were discovered because of their actions during development, where a study by Smeyne et al. has demonstrated that mice embryos with homozygous deletion of the Trk gene, suffer from neuronal cell loss in trigeminal, sympathetic, and dorsal root ganglia (75), now they are known as well for their roles in regeneration. NGF has demonstrated to play an important role in nerve regeneration. NGF has low expression levels in healthy nerves, it is upregulated in response to peripheral nerve injury which proves its role in nerve regeneration (61), indeed several studies have been carried out on animal models where exogenous NGF administration had shown promoted peripheral nerve growth and reestablishment of the functional activities of peripheral nerve fibers and damaged neurons. Repairing a transected nerve with silicone chambers filled with 1 mg/ml NGF resulted in an increased number of myelinated axons and 58% increased myelin sheath thickness compared to the control saline group (76). In another study shows that NGF is crucial to nerve regeneration by activating autophagy in Schwann cells and accelerating myelin debris clearance, a crucial prerequisite for a successful Wallerian degeneration and subsequent nerve regeneration, thus NGF promote axon and myelin regeneration at early stage of PNI (77). It has been shown that NGF plays a role in bone development as well, where NGF-TrkA signaling in sensory nerves is essential for early innervation, normal vascularization, and formation of both primary and secondary ossification centers of endochondral bone during late embryogenesis thus maintaining a normal femoral length and volume (16). While most studies are focused on NGF exerting its bone regenerating role via TrkA receptor, another study have shed the light on the NGF role binding to its low affinity receptor p75, in a cranial bone injury model it was identified the role of p75 signaling pathway in coordinating and stimulating skeletal cell migration during early bone repair where mice lacking NGF in myeloid cells or p75 in osteoblasts demonstrated reduced migration of osteogenic precursors to the injury site with consequently delayed bone healing (78). The influx of NGF expressing macrophages following cranial bone injury play a role in bone repair by inducing skeletal sensory nerve ingrowth that positively regulate bone repair, Genetic disrupt in NGF expressing macrophages or locoregional deletion of NGF delayed reinnervation and blunted the repair process, similarly delayed reinnervation and repair was induced by the inhibition of TrkA catalytic activity (79). NGF can be therapeutically used in accelerating bone healing in a tibial fracture model where a local injection of β-NGF during the endochondral/cartilaginous phase promoted cartilage to bone conversion (62). Tissue engineered NGF carrying hydrogels have shown a positive effect on promoting bone regeneration. In a rabbit mandibular distraction osteogenesis model, the enrichment of collagen/nano-hydroxyapatite/alginate hydrogel with NGF allowed its delayed release, prevention from inactivation and a prolonged in vivo NGF concentration, the group receiving the NGF enriched hydrogel has shown a superior bone histology where new bone formation was observed compared to saline control group (63). These results were in line with a previous study that has used a hydrogel of collagen/hydroxyapatite as a NGF delivery system in a model of rat calvarial defect, the treated group has demonstrated an increase in bone ingrowth and mass compared to control group (64). Due to NGF poor pharmacokinetic properties another study tested a highly selective TrkA agonist, Gambogic amide (GA), the results show that GA can improve bone repair by increasing the mRNA expression of osteoblastic and osteolytic differentiation markers, thus proofing that GA, like NGF, may promote fracture healing through promoting both osteoprogenitor differentiation and mineralization (65).
Brain-derived neurotrophic factor (BDNF) is neurotrophin family, it is known to exert its action by binding to one of its two receptors, tropomyosin receptor kinase B (TrkB) or the common neurotrophin receptor, p75NTR. BDNF has been extensively studied for its roles in neural development, BDNF ko mice are embryonically lethal, proofing that its essential during developmental stages (80). BDNF play a wide role in the central nervous system mainly in long term potentiation (LTP) thus enhancing the synaptic plasticity, learning and memory (80). In the peripheral nervous system it was shown that BDNF plays a role in peripheral nerve regeneration as it stimulates the actin deposition and polymerization in the regenerating nerve growth cone (81). It was found that BDNF expression is upregulated in response to peripheral nerve injury, in a model of sciatic nerve lesion which was deprived from BDNF by antibody treatment resulted in a dramatic reduction both in the number of myelinated axons distal to the lesion and the elongation of regenerating axons which led to a significant retardation of nerve regeneration and remyelination of injured sciatic nerves. Thus, we conclude that endogenous BDNF is required for regeneration and remyelination of the peripheral nerve after injury (82). BDNF has shown to play a role in bone fracture healing, in a study that analyzed the expression pattern of BDNF and its receptor from fracture gaps samples obtained from during different phases of bone healing. BDNF and TrkB expression were determined in early fracture hematomas expressed by hematopoietic cells, and in later stages of fracture healing by active osteoblasts, while its expression is totally absent in healthy mature bone tissue (66). Its action can be mediated indirectly by improving vascularization and innervation of the injured bone, as was shown in an in vivo study using a tissue engineered Tricalcium phosphate scaffold enriched with BDNF enhanced the multipotency of the seeded Bone mesenchymal stem cells (BMSCs) leading to the generation of a better innervated and vascularized bone tissue, thus promoting neurogenesis and osteogenesis (83). In vitro studies stimulating MC3T3-E1 cells (osteoblast lineage cell line) with BDNF has shown to induce osteogenesis by upregulating the expression of osteoblast differentiation marker osteocalcin (84).
Bone-derived modulators are showing potential roles in neurological disorders. For instance, Osteopontin (OPN) is highlighted with dual roles, suggesting that low levels may inhibit wound healing, while high levels may induce excessive tissue injury in Parkinson's disease (67, 85). Furthermore, an unexpected finding indicates that bone-derived Osteocalcin (OCN) is crucial for young blood for rejuvenation as it may contain molecules with rejuvenating effects on the brain (86). Lipocalin-2 (LCN2), another bone-derived hormone, was found to play a role in activating the anorexigenic pathway by binding to the melanocortin-4 receptor of the hypothalamus after crossing the blood-brain barriers (BBB) (87). Furthermore, previous studies have emphasized that bone marrow-derived mesenchymal stem cells (BMMSCs) play a significant role in various neurological disease treatments, such as Alzheimer's and ischemic (88). A review paper by Chen et al. provided a comprehensive overview of the functions of bone-derived modulators, including OCN, OPN, LCN2, BMMSCs, hematopoietic stem cells, and microglia-like cells and their implications on the pathogenesis of neurological disorders (85, 89, 90). However, the roles of these modulators in the brain still need to be completed, which requires further investigations to understand their full therapeutic potential to develop a particular tool for neurological disorders.
Norepinephrine is a member of catecholamine family of tyrosine-derived neurotransmitters in the sympathetic nervous system exerting their action by binding the adrenergic receptor (AR) alpha and beta isoforms, which are present on both osteoblasts and osteoclasts (68). α-AR signaling in osteoblastic cells can upregulate proteins that are related to osteogenesis, thus promoting bone formation (91). NE plays a role in chondrocyte metabolism, in an in vitro study where Primary costal chondrocytes were isolated and stimulated with NE, it was found that the apoptosis rate of chondrocytes was decreased, and NE can regulate the proliferation of osteoblasts, osteoblast-like cells, and mesenchymal stem cell lines (68). β-AR are expressed in osteoblasts and osteoclasts it is mainly involved in bone metabolism regulation (92).
Calcitonin gene related peptide is a members of the calcitonin family, mainly it is a nociceptive neurotransmitter acting on one of two receptors: calcitonin gene-related peptide receptor (CLR) and receptor activity modifying protein 1 (RAMP1) (93). CGRP plays a crucial role in bone fracture healing, in a study that collected plasma from patients with fracture neck of femur it was found to be highly elevated after 24 h compared to healthy controls (69). In an in vivo study on CGRP-deficient mice following femoral osteotomy, CGPR was found to be elevated in WT mice serum, CGRP deficient mice showed highly impaired bone regeneration and a reduction in the number of osteoblasts, genome wide expression analysis has shown that CGRP induces the expression of specific ossification linked genes (94). These results were in line with a previous study performed on selectively abolished CGRP production mouse model, CGRP-deficient mice displayed a low bone mass phenotype, reduced bone formation rate and developing osteopenia (95). In contrast targeted overexpression of CGRP in mice increased bone formation rate which resulted in an increased bone volume and density (96). CGRP stimulates osteogenesis and upregulate different osteoporotic differentiation-related genes, CGRP in vitro stimulation of bone marrow-derived mesenchymal stem cells (BMSCs) extracted from an osteoporotic female rats induced proliferation at 7 days cultures, at longer cultures more than 14 days it promoted BMSCs differentiation into osteoblasts that formed calcified nodules after long-term culture (70). CGRP maintain the same osteogenic differentiation capacity in BMSCs derived from aged rats (71). CGRP also protects bones by inhibiting the osteoclastogenesis a process of bone breaking down (97). CGRP as well acts as a bone metabolism modulator through osteoblast and osteoclast associated mechanisms (98). Local CGRP administration in a distraction osteogenesis model have demonstrated enhanced angiogenesis, increased endothelial progenitor cells (EPCs) population and promoted blood vessel formation and bone regeneration (99).
Substance P (SP) is a member of the mammalian tachykinin family, mainly present in sensory nervous system playing an important role in numerous biological processes related to pain transmission. SP is often co-released with CGRP and it mediates its biological effects through binding to neurokinin-receptor 1 (NK-R1) also known as tachykinin 1 receptor (TACR1) (100, 101). SP positive nerve fibers are widely distributed in various bone tissues, especially in the metabolically active parts, such as the periosteum. Similar to CGRP, SP as well plays a crucial role in bone fracture healing, with highly elevated levels in fractured bone patients (69). Nk-R1 is expressed by both osteoblasts and osteoclast precursors, the balance between osteoclastic bone resorption and osteogenic bone formation is the key factor in maintaining normal bone mass (101). Indeed, in an in vitro study the concentration-dependent effects of SP Signaling on primary osteoblast and osteoclast progenitor cells throughout the period of cell differentiation was investigated, SP stimulates BMSC proliferation and mineralization at higher concentrations, while lower SP concentrations increase BMSC osteogenesis at later stages of osteoblastic differentiation. (102).
Acetylcholine (Ach) is the first neurotransmitter to be discovered, an essential mediator in central and peripheral nervous system. It is synthesized by choline acetyltransferase (ChAT) enzyme and degraded by Acetylcholinesterase (AChE), and acts by binding to two types of receptors ACh acts on two main types of receptors: nicotinic and muscarinic acetylcholine receptors. The cholinergic system is widely distributed in many non-neural tissues as well (103). Ach receptors and the Acetylcholine enzymes (ChAT and AChE) were found to be expressed in both osteoblasts (104) and osteoclasts (105). The expression level of AChE changes during embryonic and postnatal bone developmental stages suggesting that it is involved in bone development and remodeling (103).
Ach could play a role in osteoblasts proliferation and differentiation (106).
Ach plays a gender specific role in bone remodeling following peak bone mass acquisition, in a study on choline transporter (ChT) heterozygous mice it was found that a reduced central Ach synthesis leads to a decrease in bone mass just in young female mice. This effect was rescued by galantamine a blood brain barrier permeable acetylcholinesterase inhibitor (AChEI) that caused an increase in brain Ach levels (107). The gender specific effect of Ach was demonstrated in another study concerning bone mechano-adaptation, osteocyte targeted conditional knockout mice with a deletion in the cholinergic receptor subunit α1 showed sexually dimorphic differences in bone formation rates and bone structure between experimental group and control, reductions in female bone geometry could be rescued by anabolic loading, not in male (108).
Neuropeptide Y (NPY) is a highly conserved neuropeptide that is important for various physiological processes expressed in both nerve system and bone tissue, it acts by binding to its receptors Y1, Y2, Y4, Y5, and Y6, Y1 and Y2 are mainly expressed in osteoblasts, osteocytes and bones tissues (109). They play a major role in osteoblast lineage differentiation (110) as well as bone homeostasis, during aging it is secreted by osteocytes, they differentiate BMSCs into adipogenic cells, NPY deletion form osteocytes results in an increase in the bone mass (111).
Contrary NPY have a positive effect on bone fracture healing, in response to a femoral injury it was found that the transcriptional and protein levels of NPY and its receptors are upregulated during bone repair (112), while the use of NPY inhibitors inhibit fracture healing (113). It was also demonstrated that NPY promotes neuroprotection and NPY- deficient mice show an impaired regeneration (114).
Table 2 summarizes some examples of the impact of cells, scaffolds, and biofactors on bone-nerve regenerations.
Nerve and bone injuries are common in the modern days, both are limiting factors that negatively affect the quality of patient's life and is considered an economical burden on the society. While peripheral nervous system can retain a limited regeneration capacity based on the severity of the injury. Bone fractures usually need surgical intervention. Our bodies are complicated systems which in need of a proper communication between its different systems, the functioning of one system is dependent on the proper functioning of another. The nervous and skeletal system is a perfect example demonstrating this interaction and functional cooperation. Cross-talks between the nervous and the skeletal system ensure metabolic and functional homeostasis. Developmental signals from the nervous system ensure a healthy functioning skeletal system. This interaction makes neurotrophins, neurotransmitters and neuropeptide enrichment of bone scaffolds a promising strategy for severe bone fractures repair.
LD: Writing – review & editing. ME: Writing – review & editing.
The author(s) declare that no financial support was received for the research, authorship, and/or publication of this article.
The authors declare that the research was conducted in the absence of any commercial or financial relationships that could be construed as a potential conflict of interest.
All claims expressed in this article are solely those of the authors and do not necessarily represent those of their affiliated organizations, or those of the publisher, the editors and the reviewers. Any product that may be evaluated in this article, or claim that may be made by its manufacturer, is not guaranteed or endorsed by the publisher.
1. Florencio-Silva R, Rodrigues G, Sasso S, Sasso-Cerri E, Simões JM, Cerri SP. Biology of bone tissue: structure, function, and factors that influence bone cells. Biomed Res Int. (2015) 2015:421746. doi: 10.1155/2015/421746
2. Chartier SR, Mitchell AST, Majuta LA, Mantyh WP. The changing sensory and sympathetic innervation of the young, adult and aging mouse femur. Neuroscience. (2018) 387:178–90. doi: 10.1016/j.neuroscience.2018.01.047
3. Sun W, Ye B, Chen S, Zeng L, Lu H, Wan Y, et al. Neuro-bone tissue engineering: emerging mechanisms, potential strategies, and current challenges. Bone Res. (2023) 11:1–26. doi: 10.1038/s41413-023-00302-8
4. Zhang Z, Hao Z, Xian C, Fang Y, Cheng B, Wu J, et al. Neuro-bone tissue engineering: multiple potential translational strategies between nerve and bone. Acta Biomater. (2022) 153:1–12. doi: 10.1016/j.actbio.2022.09.023
5. Huang S, Li Z, Liu Y, Gao D, Zhang X, Hao J, et al. Neural regulation of bone remodeling: identifying novel neural molecules and pathways between brain and bone. J Cell Physiol. (2019) 234:5466–77. doi: 10.1002/jcp.26502
6. Dimitri P, Rosen C. The central nervous system and bone metabolism: an evolving story. Calcif Tissue Int. (2017) 100:476–85. doi: 10.1007/s00223-016-0179-6
7. Damiati AL, El-Messeiry S. An overview of RNA-based scaffolds for osteogenesis. Front Mol Biosci. (2021) 8:682581. doi: 10.3389/fmolb.2021.682581
8. Wan QQ, Qin PP, Shen MJ, Ma, YX, Li B, Liu SY, et al. Simultaneous regeneration of bone and nerves through materials and architectural design: are we there yet? Adv Funct Mater. (2020) 30:2003542. doi: 10.1002/adfm.202003542
9. Sung YK, Kim SW. Recent advances in polymeric drug delivery systems. Biomater. Res. (2020) 24:12. doi: 10.1186/s40824-020-00190-7
10. Tao R, Mi B, Hu Y, Lin S, Xiong Y, Lu X, et al. Hallmarks of peripheral nerve function in bone regeneration. Bone Res. (2023) 11:6. doi: 10.1038/s41413-022-00240-x
11. Zhu G, Zhang T, Chen M, Yao K, Huang X, Zhang B, et al. Bone physiological microenvironment and healing mechanism: basis for future bone-tissue engineering scaffolds. Bioact Mater. (2021) 6:4110–40. doi: 10.1016/j.bioactmat.2021.03.043
12. Rajpar I, Tomlinson RE. Function of peripheral nerves in the development and healing of tendon and bone. Semin Cell Dev Biol. (2022) 123:48–56. doi: 10.1016/j.semcdb.2021.05.001
13. Marrella A, Lee TY, Lee DH, Karuthedom S, Syla D, Chawla A, et al. Engineering vascularized and innervated bone biomaterials for improved skeletal tissue regeneration. Mater Today. (2018) 21:362–76. doi: 10.1016/j.mattod.2017.10.005
14. Sisask G, Silfverswärd CJ, Bjurholm A, Nilsson O. Ontogeny of sensory and autonomic nerves in the developing mouse skeleton. Auton Neurosci Basic Clin. (2013) 177:237–43. doi: 10.1016/j.autneu.2013.05.005
15. Gajda M, Litwin JA, Cichocki T, Timmermans JP, Adriaensen D. Development of sensory innervation in rat tibia: co-localization of CGRP and substance P with growth-associated protein 43 (GAP-43). Anat, J. (2005) 207:135–44. doi: 10.1111/j.1469-7580.2005.00434.x
16. Tomlinson RE, Li Z, Zhang Q, Goh BC, Li Z, Thorek DLJ, et al. NGF-TrkA signaling by sensory nerves coordinates the vascularization and ossification of developing endochondral bone. Cell Rep. (2016) 16:2723–35. doi: 10.1016/j.celrep.2016.08.002
17. Cornish J, Callon KE, Bava U, Kamona SA, Cooper GSJ, Reid IR. Effects of calcitonin, amylin, and calcitonin gene-related peptide on osteoclast development. Bone. (2001) 29:162–8. doi: 10.1016/S8756-3282(01)00494-X
18. Wu JQ, Jiang N, Yu B. Mechanisms of action of neuropeptide Y on stem cells and its potential applications in orthopaedic disorders. World J Stem Cells. (2020) 12:986–1000. doi: 10.4252/wjsc.v12.i9.986
19. Wee NKY, Sinder BP, Novak S, Wang X, Stoddard C, Matthews BG, et al. Skeletal phenotype of the neuropeptide Y knockout mouse. Neuropeptides. (2019) 73:88. doi: 10.1016/j.npep.2018.11.009
20. Kong L, Zhao H, Wang F, Zhang R, Yao X, Zuo R, et al. Endocrine modulation of brain-skeleton axis driven by neural stem cell-derived perilipin 5 in the lipid metabolism homeostasis for bone regeneration. Mol Ther. (2023) 31:1293–312. doi: 10.1016/j.ymthe.2023.02.004
21. Xiao Y, Han C, Wang Y, Zhang X, Bao R, Li Y, et al. Interoceptive regulation of skeletal tissue homeostasis and repair. Bone Res. (2023) 11:48. doi: 10.1038/s41413-023-00285-6
22. Lv X, Gao F, Li TP, Xue X, Wang X, Wan M, et al. Skeleton interoception regulates bone and fat metabolism through hypothalamic neuroendocrine NPY. eLife. (2021) 10:70324. doi: 10.7554/eLife.70324
23. Lv X, Gao F, Cao X. Skeletal interoception in bone homeostasis and pain. Cell Metab. (2022) 34:1914–31. doi: 10.1016/j.cmet.2022.09.025
24. Oryan A, Kamali A, Moshiri A, Baghaban Eslaminejad M. Role of mesenchymal stem cells in bone regenerative medicine: what is the evidence? Cells Tissues Organs. (2017) 204:59–83. doi: 10.1159/000469704
25. Lopes D, Martins-Cruz C, Oliveira MB, Mano JF. Bone physiology as inspiration for tissue regenerative therapies. Biomaterials. (2018) 185:240–75. doi: 10.1016/j.biomaterials.2018.09.028
26. Gkiatas I, Papadopoulos D, Pakos EE, Kostas-Agnantis I, Gelalis I, Vekris M, et al. The multifactorial role of peripheral nervous system in bone growth. Front Phys. (2017) 5:44. doi: 10.3389/fphy.2017.00044
27. Mach DB, Rogers SD, Sabino MC, Luger NM, Schwei MJ, Pomonis JD, et al. Origins of skeletal pain: sensory and sympathetic innervation of the mouse femur. Neuroscience. (2002) 113:155–66. doi: 10.1016/S0306-4522(02)00165-3
28. Yu LMY, Leipzig ND, Shoichet MS. Promoting neuron adhesion and growth. Mater Today. (2008) 11:36–43. doi: 10.1016/S1369-7021(08)70088-9
29. Li Y, Ma Z, Ren Y, Lu D, Li T, Li W, et al. Tissue engineering strategies for peripheral nerve regeneration. Front Neurol. (2021) 12:768267. doi: 10.3389/fneur.2021.768267
30. Berns EJ, Sur S, Pan L, Goldberger JE, Suresh S, Zhang S, et al. Aligned neurite outgrowth and directed cell migration in self-assembled monodomain gels. Biomaterials. (2014) 35:185–95. doi: 10.1016/j.biomaterials.2013.09.077
31. Han Y, Li X, Zhang Y, Han Y, Chang F, Ding J. Mesenchymal stem cells for regenerative medicine. Cells. (2019) 8:80886. doi: 10.3390/cells8080886
32. Damiati L, Eales MG, Nobbs AH, Su B, Tsimbouri PM, Salmeron-Sanchez M, et al. Impact of surface topography and coating on osteogenesis and bacterial attachment on titanium implants. J Tissue Eng. (2018) 9:204173141879069. doi: 10.1177/2041731418790694
33. Agarwal S, Curtin J, Duffy B, Jaiswal S. Biodegradable magnesium alloys for orthopaedic applications: a review on corrosion, biocompatibility and surface modifications. Mater Sci Eng C. (2016) 68:948–63. doi: 10.1016/j.msec.2016.06.020
34. Guan S, Zhang Z, Wu J. Non-coding RNA delivery for bone tissue engineering: Progress, challenges, and potential solutions. iScience. (2022) 25:104807. doi: 10.1016/j.isci.2022.104807
35. Damiati LA, El-Yaagoubi M, Damiati SA, Kodzius R, Sefat F, Damiati S. Role of polymers in microfluidic devices. Polymers. (2022) 14:235132. doi: 10.3390/polym14235132
36. Lee SS, Du X, Kim I, Ferguson SJ. Scaffolds for bone-tissue engineering. Matter. (2022) 5:2722–59. doi: 10.1016/j.matt.2022.06.003
37. Wasyłeczko M, Sikorska W, Chwojnowski A. Review of synthetic and hybrid scaffolds in cartilage tissue engineering. Membranes. (2020) 10:110348. doi: 10.3390/membranes10110348
38. Xiong Y, Mi B-B, Lin Z, Hu Y-Q, Yu L, Zha K-K, et al. The role of the immune microenvironment in bone, cartilage, and soft tissue regeneration: from mechanism to therapeutic opportunity. Mil Med Res. (2022) 9:65. doi: 10.1186/s40779-022-00426-8
39. Xu J, Zhang Z, Zhao J, Meyers CA, Lee S, Qin Q, et al. Interaction between the nervous and skeletal systems. Front Cell Dev Biol. (2022) 10:976736. doi: 10.3389/fcell.2022.976736
40. Emanueli C, Salis MB, Pinna A, Graiani G, Manni L, Madeddu P. Nerve growth factor promotes angiogenesis and arteriogenesis in ischemic hindlimbs. Circulation. (2002) 106:2257–62. doi: 10.1161/01.CIR.0000033971.56802.C5
41. Diomede F, Marconi GD, Fonticoli L, Pizzicanella J, Merciaro I, Bramanti P, et al. Functional relationship between osteogenesis and angiogenesis in tissue regeneration. Int J Mol Sci. (2020) 21:93242. doi: 10.3390/ijms21093242
42. Wintzer M, Mladinic M, Lazarevic D, Casseler C, Cattaneo A, Nicholls J. Strategies for identifying genes that play a role in spinal cord regeneration. Anat, J. (2004) 204:3–11. doi: 10.1111/j.1469-7580.2004.00258.x
43. Xu X, Zheng L, Yuan Q, Zhen G, Crane JL, Zhou X, et al. Transforming growth factor-β in stem cells and tissue homeostasis. Bone Res. (2018) 6:2. doi: 10.1038/s41413-017-0005-4
44. Stamnitz S, Klimczak A. Mesenchymal stem cells, bioactive factors, and scaffolds in bone repair: from research perspectives to clinical practice. Cells. (2021) 10:81925. doi: 10.3390/cells10081925
45. Dixit M, Poudel SB, Yakar S. Effects of GH/IGF axis on bone and cartilage. Mol Cell Endocrinol. (2021) 519:111052. doi: 10.1016/j.mce.2020.111052
46. Beamer B, Hettrich C, Lane J. Vascular endothelial growth factor: an essential component of angiogenesis and fracture healing. HSS J. (2010) 6:85–94. doi: 10.1007/s11420-009-9129-4
47. Martino MM, Brkic S, Bovo E, Burger M, Schaefer DJ, Wolff T, et al. Extracellular matrix and growth factor engineering for controlled angiogenesis in regenerative medicine. Bioeng Biotechnol. (2015) 3:1–8. doi: 10.3389/fbioe.2015.00045
48. Daluiski A, Engstrand T, Bahamonde ME, Gamer LW, Agius E, Stevenson SL, et al. Bone morphogenetic protein-3 is a negative regulator of bone density. Nat Genet. (2001) 27:83810. doi: 10.1038/83810
49. Chen G, Deng C, Li YP. TGF-β and BMP signaling in osteoblast differentiation and bone formation. Int Biol, J Sci. (2012) 8:272–88. doi: 10.7150/ijbs.2929
50. Tsuji K, Bandyopadhyay A, Harfe BD, Cox K, Kakar S, Gerstenfeld L, et al. BMP2 activity, although dispensable for bone formation, is required for the initiation of fracture healing. Nat Genet. (2006) 38:1424–9. doi: 10.1038/ng1916
51. Luu HH, Song WX, Luo X, Manning D, Luo J, Deng ZL, et al. Distinct roles of bone morphogenetic proteins in osteogenic differentiation of mesenchymal stem cells. Orthop J Res. (2007) 25:665–77. doi: 10.1002/jor.20359
52. Lian JB, Stein GS, Javed A, van Wijnen AJ, Stein JL, Montecino M, et al. Networks and hubs for the transcriptional control of osteoblastogenesis. Rev Endocr Metab Disord. (2006) 7:1–16. doi: 10.1007/s11154-006-9001-5
53. Jing D, Tong S, Zhai M, Li X, Cai J, Wu Y, et al. Effect of low-level mechanical vibration on osteogenesis and osseointegration of porous titanium implants in the repair of long bone defects. Sci Rep. (2015) 5:1–13. doi: 10.1038/srep17134
54. Al-Jarsha M, Moulisová V, Leal-Egaña A, Connell A, Naudi KB, Ayoub AF, et al. Engineered coatings for titanium implants to present ultralow doses of BMP-7 ACS. Biomater Sci Eng. (2018) 4:1812–9. doi: 10.1021/acsbiomaterials.7b01037
55. Guillot R, Pignot-paintrand I, Lavaud J, Decambron A, Bourgeois E, Josserand V. Acta Biomaterialia Assessment of a polyelectrolyte multilayer film coating loaded with BMP-2 on titanium and PEEK implants in the rabbit femoral condyle. Acta Biomater. (2016) 36:310–22. doi: 10.1016/j.actbio.2016.03.010
56. Aenlle KK, Curtis KM, Roos BA, Howard GA. Hepatocyte growth factor and p38 promote osteogenic differentiation of human mesenchymal stem cells. Mol Endocrinol. (2014) 28:722–30. doi: 10.1210/me.2013-1286
57. Gersbach CA. Chapter 8—engineered proteins for controlling gene expression A2—Atala, Anthony. In:Lanza R, Thomson JA, RBTP RBTP of RM, editors. Nerem. 2nd ed. San Diego, CA: Academic Press (2011). p. 159–76.
58. Caplan AI. Chapter 14—MSCs in regenerative medicine A2—Atala, Anthony. In:Lanza R, Thomson JA, RBTP RBTP of RM, editors. Nerem. 2nd ed. San Diego, CA: Academic Press (2011). p. 253–62.
59. Jeng L, Ng kee Kwong F, Spector M. Chapter 42—articular cartilage A2—Atala, Anthony. In:Lanza R, Thomson JA, RBTP of RM, editors. Nerem. 2nd ed. San Diego, CA: Academic Press (2011). p. 761–77.
60. Nyberg E, Holmes C, Witham T, Grayson WL. Growth factor-eluting technologies for bone tissue engineering. Drug Deliv Transl Res. (2015) 6:184–94. doi: 10.1007/s13346-015-0233-3
61. Menorca RMG, Fussell TS, Elfar JG. Peripheral nerve trauma: mechanisms of injury and recovery. Hand Clin. (2013) 29:317–30. doi: 10.1016/j.hcl.2013.04.002
62. Rivera KO, Russo F, Boileau RM, Tomlinson RE, Miclau T, Marcucio RS, et al. Local injections of β-NGF accelerates endochondral fracture repair by promoting cartilage to bone conversion. Sci Rep. (2020) 10:1–15. doi: 10.1038/s41598-020-78983-y
63. Cao J, Wang L, Lei DL, Liu YP, Du ZJ, Cui FZ. Local injection of nerve growth factor via a hydrogel enhances bone formation during mandibular distraction osteogenesis. Oral Surg Oral Med Oral Pathol Oral Radiol. (2012) 113:48–53. doi: 10.1016/j.tripleo.2011.01.021
64. Letic-Gavrilovic A, Piattelli A, Abe K. Nerve growth factor β(NGF β) delivery via a collagen/hydroxyapatite (Col/HAp) composite and its effects on new bone ingrowth. J Mater Sci Mater Med. (2003) 14:95–102. doi: 10.1023/A:1022099208535
65. Johnstone MR, Brady RD, Schuijers JA, Church JE, Orr D, Quinn JMW, et al. The selective TrkA agonist, gambogic amide, promotes osteoblastic differentiation and improves fracture healing in mice. J Musculoskelet Neuronal Interact. (2019) 19:94–103.
66. Kilian O, Hartmann S, Dongowski N, Karnati S, Baumgart-Vogt E, Härtel FV, et al. BDNF and its TrkB receptor in human fracture healing. Ann Anat. (2014) 196:286–95. doi: 10.1016/j.aanat.2014.06.001
67. Iczkiewicz J, Jackson MJ, Smith LA, Rose S, Jenner P. Osteopontin expression in substantia nigra in MPTP-treated primates and in Parkinson's disease. Brain Res. (2006) 1118:239–50. doi: 10.1016/j.brainres.2006.08.036
68. Opolka A, Straub RH, Pasoldt A, Grifka J, Grässel S. Substance P and norepinephrine modulate murine chondrocyte proliferation and apoptosis. Arthritis Rheum. (2012) 64:729–39. doi: 10.1002/art.33449
69. Onuoha GN, Alpar EK. Elevation of plasma CGRP and SP levels in orthopedic patients with fracture neck of femur. Neuropeptides. (2000) 34:116–20. doi: 10.1054/npep.2000.0803
70. Liang W, Zhuo X, Tang Z, Wei X, Li B. Calcitonin gene-related peptide stimulates proliferation and osteogenic differentiation of osteoporotic rat-derived bone mesenchymal stem cells. Mol Cell Biochem. (2015) 402:101–10. doi: 10.1007/s11010-014-2318-6
71. Li H, Qu J, Zhu H, Wang J, He H, Xie X, et al. CGRP regulates the age-related switch between osteoblast and adipocyte differentiation. Front Cell Dev Biol. (2021) 9:1–11. doi: 10.3389/fcell.2021.675503
72. Negri S, Samuel TJ, Lee S. The potential role of exercise training and mechanical loading on bone-associated skeletal nerves. J Bone Metab. (2021) 28:267–77. doi: 10.11005/jbm.2021.28.4.267
73. Aloe L. Rita Levi-Montalcini: the discovery of nerve growth factor and modern neurobiology. Trends Cell Biol. (2004) 14:395–9. doi: 10.1016/j.tcb.2004.05.011
74. Chan JR, Watkins TA, Cosgaya JM, Zhang C, Chen L, Reichardt LF, et al. NGF controls axonal receptivity to myelination by Schwann cells or oligodendrocytes in which myelination by both Schwann cells and oligo-dendrocytes is controlled by common axonal signals (Colello and Pott, 1997). Direct evaluation of this model. Neuron. (2004) 43:183–91. doi: 10.1016/j.neuron.2004.06.024
75. Smeyne RJ, Klein R, Schnapp A, Long LK, Bryant S, Lewin A, et al. Severe sensory and sympathetic neuropathies in mice carrying a disrupted Trk/NGF receptor gene. Nature. (1994) 368:20. doi: 10.1038/368246a0
76. Rich KM, Alexander TD, Pryor JC, Hollowell JG. Nerve growth factor enhances regeneration through silicone chambers. Exp Neurol. (1989) 105:162–70. doi: 10.1016/0014-4886(89)90115-5
77. Li R, Li D, Wu C, Ye L, Wu Y, Yuan Y, et al. Nerve growth factor activates autophagy in Schwann cells to enhance myelin debris clearance and to expedite nerve regeneration. Theranostics. (2020) 10:1649–77. doi: 10.7150/thno.40919
78. Xu J, Li Z, Tower RJ, Negri S, Wang Y, Meyers CA, et al. NGF-p75 signaling coordinates skeletal cell migration during bone repair. Sci Adv. (2022) 8:abl5716. doi: 10.1126/sciadv.abl5716
79. Meyers CA, Lee S, Sono T, Xu J, Negri S, Tian Y, et al. A neurotrophic mechanism directs sensory nerve transit in cranial bone. Cell Rep. (2020) 31:107696. doi: 10.1016/j.celrep.2020.107696
80. McGregor CE, English AW. The role of BDNF in peripheral nerve regeneration: activity-dependent treatments and Val66Met. Front Cell Neurosci. (2019) 12:522. doi: 10.3389/fncel.2018.00522
81. Yuan X-b, Jin M, Xu X, Song Y-q, Wu C-p, Poo M-m, et al. Signalling and crosstalk of Rho GTPases in mediating axon guidance. Nat Cell Biol. (2003) 5:38–45. doi: 10.1038/ncb895
82. Zhang JY, Luo XG, Xian CJ, Liu ZH, Zhou XF. Endogenous BDNF is required for myelination and regeneration of injured sciatic nerve in rodents. J Eur Neurosci. (2000) 12:4171–80. doi: 10.1046/j.1460-9568.2000.01312.x
83. Liu Q, Lei L, Yu T, Jiang T, Kang Y. Effect of brain-derived neurotrophic factor on the neurogenesis and osteogenesis in bone engineering. Tissue Eng A. (2018) 16:1283–92. doi: 10.1089/ten.tea.2017.0462
84. Ida-Yonemochi H, Yamada Y, Yoshikawa H, Seo K. Locally produced BDNF promotes sclerotic change in alveolar bone after nerve injury. PLoS ONE. (2017) 12:1–18. doi: 10.1371/journal.pone.0169201
85. Chen H, Shang D, Wen Y, Liang C. Bone-derived modulators that regulate brain function: emerging therapeutic targets for neurological disorders. Front Cell Dev Biol. (2021) 9:1–13. doi: 10.3389/fcell.2021.683457
86. Khrimian L, Obri A, Ramos-Brossier M, Rousseaud A, Moriceau S, Nicot AS, et al. Gpr158 mediates osteocalcin's regulation of cognition. J Exp Med. (2017) 214:2859–73. doi: 10.1084/jem.20171320
87. Mosialou I, Shikhel S, Liu JM, Maurizi A, Luo N, He Z, et al. MC4R-dependent suppression of appetite by bone-derived lipocalin 2. Nature. (2017) 543:385–90. doi: 10.1038/nature21697
88. Bao X, Feng M, Wei J, Han Q, Zhao H, Li G, et al. Transplantation of Flk-1+ human bone marrow-derived mesenchymal stem cells promotes angiogenesis and neurogenesis after cerebral ischemia in rats. J Eur Neurosci. (2011) 34:87–98. doi: 10.1111/j.1460-9568.2011.07733.x
89. Jahromi GP, Pirsaraei AS, Sadr SS, Kaka G, Jafari M, Seidi S, et al. Multipotent bone marrow stromal cell therapy promotes endogenous cell proliferation following ischemic stroke. Clin Exp Pharmacol Physiol. (2015) 42:1158–67. doi: 10.1111/1440-1681.12466
90. Jarocha D, Milczarek O, Wedrychowicz A, Kwiatkowski S, Majka M. Continuous improvement after multiple mesenchymal stem cell transplantations in a patient with complete spinal cord injury. Cell Transplant. (2015) 24:661–72. doi: 10.3727/096368915X687796
91. Wan QQ, Qin WP, Ma YX, Shen MJ, Li J, Zhang ZB, et al. Crosstalk between bone and nerves within bone. Adv Sci. (2021) 8:3390. doi: 10.1002/advs.202003390
92. Khosla S, Drake MT, Volkman TL, Thicke BS, Achenbach SJ, Atkinson EJ, et al. Sympathetic β1-adrenergic signaling contributes to regulation of human bone metabolism. J Clin Invest. (2018) 128:4832–42. doi: 10.1172/JCI122151
93. Wang Q, Qin H, Deng J, Xu H, Liu S, Weng J, et al. Research progress in calcitonin gene-related peptide and bone repair. Biomolecules. (2023) 13:1–18. doi: 10.3390/biom13050838
94. Appelt J, Baranowsky A, Jahn D, Yorgan T, Köhli P, Otto E, et al. The neuropeptide calcitonin gene-related peptide alpha is essential for bone healing. EBioMedicine. (2020) 59:102970. doi: 10.1016/j.ebiom.2020.102970
95. Schinke T, Liese S, Priemel M, Haberland M, Schilling AF, Catala-Lehnen P, et al. Decreased bone formation and osteopenia in mice lacking α-calcitonin gene-related peptide. J Bone Miner Res. (2004) 19:2049–56. doi: 10.1359/jbmr.040915
96. Ballica R, Valentijn K, Khachatryan A, Guerder S, Kapadia S, Gundberg C, et al. Targeted expression of calcitonin gene-related peptide to osteoblasts increases bone density in mice. J Bone Miner Res. (1999) 14:1067–74. doi: 10.1359/jbmr.1999.14.7.1067
97. Ishizuka K, Hirukawa K, Nakamura H, Togari A. Inhibitory effect of CGRP on osteoclast formation by mouse bone marrow cells treated with isoproterenol. Neurosci Lett. (2005) 379:47–51. doi: 10.1016/j.neulet.2004.12.046
98. He H, Chai J, Zhang S, Ding L, Yan P, Du W, et al. CGRP may regulate bone metabolism through stimulating osteoblast differentiation and inhibiting osteoclast formation. Mol Med Rep. (2016) 13:3977–84. doi: 10.3892/mmr.2016.5023
99. Mi J, Xu J, Yao H, Li X, Tong W, Li Y, et al. Calcitonin gene-related peptide enhances distraction osteogenesis by increasing angiogenesis. Tissue Eng A. (2021) 27:9. doi: 10.1089/ten.tea.2020.0009
100. Brazill JM, Beeve AT, Craft CS, Ivanusic JJ, Scheller EL. Nerves in bone: evolving concepts in pain and anabolism. J Bone Miner Res. (2019) 34:1393–406. doi: 10.1002/jbmr.3822
101. Li FXZ, Xu F, Lin X, Wu F, Zhong JY, Wang Y, et al. The role of substance P in the regulation of bone and cartilage metabolic activity. Front Endocrinol. (2020) 11:77. doi: 10.3389/fendo.2020.00077
102. Wang L, Zhao R, Shi X, Wei T, Halloran BP, Clark DJ, et al. Substance P stimulates bone marrow stromal cell osteogenic activity, osteoclast differentiation, and resorption activity in vitro. Bone. (2009) 45:309–20. doi: 10.1016/j.bone.2009.04.203
103. Luo X, Lauwers M, Layer PG, Wen C. Non-neuronal role of acetylcholinesterase in bone development and degeneration. Front Cell Dev Biol. (2021) 8:620543. doi: 10.3389/fcell.2020.620543
104. Inkson CA, Brabbs AC, Grewal TS, Skerry TM, Genever PG. Characterization of acetylcholinesterase expression and secretion during osteoblast differentiation. Bone. (2004) 35:819–27. doi: 10.1016/j.bone.2004.05.026
105. Ternes S, Trinkaus K, Bergen I, Knaack S, Gelinsky M, Kilian O, et al. Impact of acetylcholine and nicotine on human osteoclastogenesis in vitro. Int Immunopharmacol. (2015) 29:215–21. doi: 10.1016/j.intimp.2015.09.013
106. Sato T, Abe T, Chida D, Nakamoto N, Hori N, Kokabu S, et al. Functional role of acetylcholine and the expression of cholinergic receptors and components in osteoblasts. FEBS Lett. (2010) 584:817–24. doi: 10.1016/j.febslet.2010.01.001
107. Ma Y, Elefteriou F. Brain-derived acetylcholine maintains peak bone mass in adult female mice. J Bone Miner Res. (2020) 35:1562–71. doi: 10.1002/jbmr.4024
108. Mora-Antoinette M, Obaji M, Saffari A, Lewis KL. Nicotinic acetylcholine receptors on osteocytes impact bone mechano adaptation in a sexually dimorphic manner. BioRxiv. (2023) 10:1–13. doi: 10.1101/2023.10.01.556129
109. Chen Q, Zhang Y. The role of NPY in the regulation of bone. Metabolism. (2022) 13:1–7. doi: 10.3389/fendo.2022.833485
110. Igwe CJC, Jiang X, Paic F, Ma L, Adams DJ, Baldock PA, et al. Neuropeptide Y is expressed by osteocytes and can inhibit osteoblastic activity. J Cell Biochem. (2009) 108:621–30. doi: 10.1002/jcb.22294
111. Zhang Y, Chen C-Y, Liu Y-W, Rao S-S, Tan Y-J, Qian Y-X, et al. Neuronal induction of bone-fat imbalance through osteocyte neuropeptide. Adv, Y Sci. (2021) 8:1–20. doi: 10.1002/advs.202170165
112. Alves CJ, Alencastre IS, Neto E, Ribas J, Ferreira S, Vasconcelos DM, et al. Bone injury and repair trigger central and peripheral NPY neuronal pathways. PLoS ONE. (2016) 11:1–17. doi: 10.1371/journal.pone.0165465
113. Tang P, Duan C, Wang Z, Wang C, Meng G, Lin K, et al. NPY and CGRP inhibitor influence on ERK pathway and macrophage aggregation during fracture healing. Cell Physiol Biochem. (2017) 41:1457–67. doi: 10.1159/000468405
114. Park MH, Jin HK, Min WK, Lee WW, Lee JE, Akiyama H, et al. Neuropeptide Y regulates the hematopoietic stem cell microenvironment and prevents nerve injury in the bone marrow. EMBO J. (2015) 34:1648–60. doi: 10.15252/embj.201490174
115. Lei L, Liu Z, Yuan P, Jin R, Wang X, Jiang T, et al. Injectable colloidal hydrogel with mesoporous silica nanoparticles for sustained co-release of microRNA-222 and aspirin to achieve innervated bone regeneration in rat mandibular defects. J Mater Chem B. (2019) 7:2722–35. doi: 10.1039/C9TB00025A
116. Jin P, Yin F, Huang L, Zheng L, Zhao J, Zhang X. Guangxi cobra venom-derived NGF promotes the osteogenic and therapeutic effects of porous BCP ceramic. Exp Mol Med. (2017) 49:e312. doi: 10.1038/emm.2016.173
117. Chen WH, Mao CQ, Zhuo LL, Ong JL. Beta-nerve growth factor promotes neurogenesis and angiogenesis during the repair of bone defects. Neural Regen Res. (2015) 10:1159–65. doi: 10.4103/1673-5374.160114
118. Dhand C, Ong ST, Dwivedi N, Diaz SM, Venugopal JR, Navaneethan B, et al. Bio-inspired in situ crosslinking and mineralization of electrospun collagen scaffolds for bone tissue engineering. Biomaterials. (2016) 104:323–38. doi: 10.1016/j.biomaterials.2016.07.007
119. Eap S, Bécavin T, Keller L, Kökten T, Fioretti F, Weickert J-L, et al. Nanofibers implant functionalized by neural growth factor as a strategy to innervate a bioengineered tooth. Adv Healthc Mater. (2014) 3:386–91. doi: 10.1002/adhm.201300281
120. Wang Z, Chen T, Wu Z, Jiang X, Hou Q, Miao S, et al. The dual-effects of PLGA@MT electrospun nanofiber coatings on promoting osteogenesis at the titanium-bone interface under diabetic conditions. J Mater Chem B. (2022) 10:4020–30. doi: 10.1039/D2TB00120A
121. Lotz EM, Berger MB, Boyan BD, Schwartz Z. Regulation of mesenchymal stem cell differentiation on microstructured titanium surfaces by semaphorin 3A. Bone. (2020) 134:115260. doi: 10.1016/j.bone.2020.115260
122. Wu Y, Jing D, Ouyang H, Li L, Zhai M, Li Y, et al. Pre-implanted sensory nerve could enhance the neurotization in tissue-engineered bone graft. Tissue Eng A. (2015) 21:2241–9. doi: 10.1089/ten.tea.2014.0688
123. Fitzpatrick V, Martín-Moldes Z, Deck A, Torres-Sanchez R, Valat A, Cairns D, et al. Functionalized 3D-printed silk-hydroxyapatite scaffolds for enhanced bone regeneration with innervation and vascularization. Biomaterials. (2021) 276:120995. doi: 10.1016/j.biomaterials.2021.120995
124. Li J-Y, Wang T-T, Li C, Wang Z-F, Li S, Ma L, et al. Semaphorin 3A-hypoxia inducible factor 1 subunit alpha co-overexpression enhances the osteogenic differentiation of induced pluripotent stem cells-derived mesenchymal stem cells in vitro. Chin Med J. (2020) 133:301–9. doi: 10.1097/CM9.0000000000000612
125. Ma Y-X, Jiao K, Wan Q-Q, Li J, Liu M-Y, Zhang Z-B, et al. Silicified collagen scaffold induces semaphorin 3A secretion by sensory nerves to improve in-situ bone regeneration. Bioact Mater. (2022) 9:475–90. doi: 10.1016/j.bioactmat.2021.07.016
126. Liu X, Tan N, Zhou Y, Zhou X, Chen H, Wei H, et al. Semaphorin 3A shifts adipose mesenchymal stem cells towards osteogenic phenotype and promotes bone regeneration in vivo. Stem Cells Int. (2016) 2016:2545214. doi: 10.1155/2016/2545214
127. Kauschke V, Schneider M, Jauch A, Schumacher M, Kampschulte M, Rohnke M, et al. Effects of a pasty bone cement containing brain-derived neurotrophic factor-functionalized mesoporous bioactive glass particles on metaphyseal healing in a new murine osteoporotic fracture model. J Int Mol Sci. (2018) 19:113531. doi: 10.3390/ijms19113531
128. Lan Y, Xie H, Jin Q, Zhao X, Shi Y, Zhou Y, et al. Extracellular vesicles derived from neural EGFL-Like 1-modified mesenchymal stem cells improve acellular bone regeneration via the miR-25-5p-SMAD2 signaling axis. Bioact Mater. (2022) 17:457–70. doi: 10.1016/j.bioactmat.2022.01.019
129. Li W, Miao W, Liu Y, Wang T, Zhang Y, Wang W, et al. Bioprinted constructs that mimic the ossification center microenvironment for targeted innervation in bone regeneration. Adv Funct Mater. (2022) 32:2109871. doi: 10.1002/adfm.202109871
Keywords: bone, peripheral nerve, tissue engineering, scaffolds, biofactors, growth factors
Citation: Damiati LA and El Soury M (2024) Bone-nerve crosstalk: a new state for neuralizing bone tissue engineering—A mini review. Front. Med. 11:1386683. doi: 10.3389/fmed.2024.1386683
Received: 15 February 2024; Accepted: 18 March 2024;
Published: 16 April 2024.
Edited by:
Ian James Martins, University of Western Australia, AustraliaReviewed by:
Lingchi Kong, Shanghai Jiao Tong University, ChinaCopyright © 2024 Damiati and El Soury. This is an open-access article distributed under the terms of the Creative Commons Attribution License (CC BY). The use, distribution or reproduction in other forums is permitted, provided the original author(s) and the copyright owner(s) are credited and that the original publication in this journal is cited, in accordance with accepted academic practice. No use, distribution or reproduction is permitted which does not comply with these terms.
*Correspondence: Laila A. Damiati, bGFkYW1pYXRpQHVqLmVkdS5zYQ==
†These authors have contributed equally to this work
‡ORCID: Laila A. Damiati orcid.org/0000-0002-4746-0915
Disclaimer: All claims expressed in this article are solely those of the authors and do not necessarily represent those of their affiliated organizations, or those of the publisher, the editors and the reviewers. Any product that may be evaluated in this article or claim that may be made by its manufacturer is not guaranteed or endorsed by the publisher.
Research integrity at Frontiers
Learn more about the work of our research integrity team to safeguard the quality of each article we publish.