- 1Department of Otorhinolaryngology, Longgang Otorhinolaryngology Hospital and Shenzhen Key Laboratory of Otorhinolaryngology, Shenzhen Institute of Otorhinolaryngology, Shenzhen, Guangdong, China
- 2Department of Graduate and Scientific Research, Zunyi Medical University Zhuhai Campus, Zhuhai, Guangdong, China
- 3School of Computer Science and Engineering, Yulin Normal University, Yulin, Guangxi, China
Head and neck squamous cell carcinoma (HNSCC) originates from the squamous epithelium of the oral cavity, oropharynx, larynx, and hypopharynx. HNSCC in the oral cavity and larynx is strongly associated with tobacco smoking and alcohol consumption, while oropharyngeal cancer is increasingly attributed to infection by human papillomavirus (HPV), particularly HPV-16. The tumor microenvironment (TME) is a complex network of cancer cells, immune cells, stromal cells, surrounding blood vessels, and signaling molecules, and plays a critical role in tumor cell survival, invasion, and recurrence. Therefore, it is critical to elucidate the molecular basis of the interaction between tumor cells and the TME in order to develop innovative anti-cancer therapeutic strategies.
1. Introduction
Head and neck cancer ranks as the sixth most common cancer globally, with approximately 600,000 new cases diagnosed every year. Head and neck squamous cell carcinoma (HNSCC) is the predominant type, and arises from the mucosal epithelium of the oral cavity, pharynx, and larynx (1, 2). Several risk factors of HNSCC have been identified, such as exposure to tobacco-derived carcinogens and excessive alcohol consumption (3). In addition, oncogenic viruses such as high-risk human papillomavirus (HPV), particularly HPV-16, are increasingly being recognized as common causes of HNSCC in younger patients (4). The treatment options for HNSCC include surgery, radiation therapy, chemotherapy, targeted therapy, or a combination thereof, and the suitable approach depends on the tumor location and staging, along with the age and overall health of patients (5). Nevertheless, the prognosis for HNSCC patients is often poor due to high rates of local recurrence and lymph node metastasis (6). The five-year survival rate of HNSCC patients ranges from 50 to 60%, and up to 30% will experience cancer recurrence and treatment failure (7).
The tumor microenvironment (TME) is a complex array of cellular and non-cellular components that drive tumor initiation and progression (8). The cellular components include stromal cells and immune cells, and the non-cellular components consist of extracellular matrix (ECM) proteins (9). Stromal cells include cancer-associated fibroblasts (CAFs), endothelial cells (ECs), and the blood and lymphatic vessel network, while immune cells comprise of tumor-associated macrophages (TAMs), myeloid-derived suppressor cells (MDSCs), T cells, B cells, and natural killer (NK) cells. Tumor cells rely on the TME for nutrients, intermediate metabolites, hormones, cytokines/chemokines, and growth factors crucial for their proliferation and survival. Moreover, the TME plays a pivotal role in tumor immune evasion and promoting tumor-associated inflammation (10). On the other hand, the metabolic alterations in the proliferating tumor cells can reshape the TME to create conditions favorable for tumor progression (11) (Figure 1).
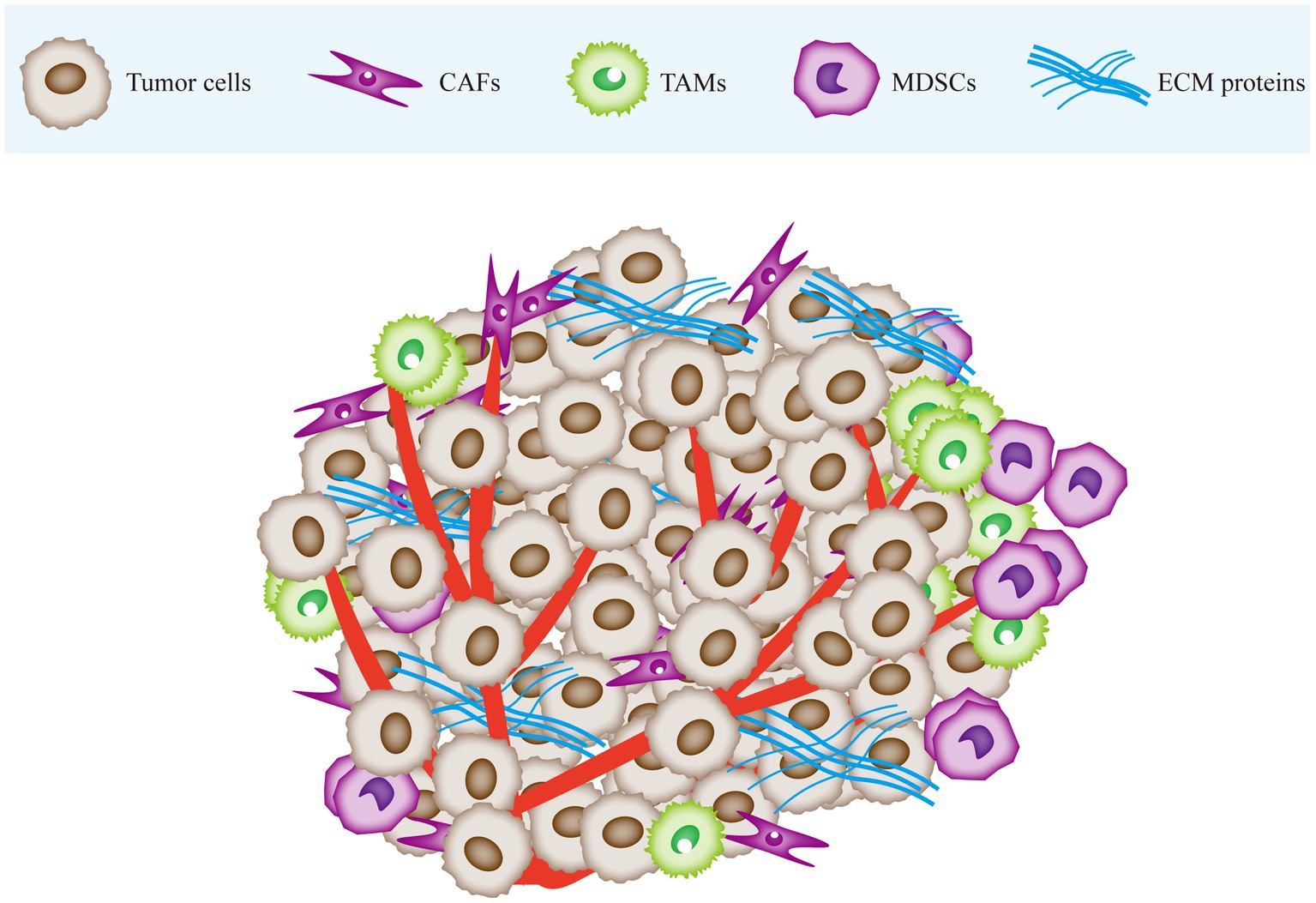
Figure 1. The tumor microenvironment is mainly composed of cancer cells, immune cells, stromal cells and extracellular matrix (ECM). Tumor tissue infiltrated by CAF and immune cells. Tumor tissue is stiffer than normal tissue due to stromal deposition and cross-linking. CAF, cancer-associated fibroblasts; TAM, tumor-associated macrophages; MDSC, myeloid-derived suppressor cells; ECM, extracellular matrix.
While surgery and radiation therapy are effective against early-stage tumors (stages I and II), many HNSCC patients are diagnosed at the advanced stage of the disease without a clinical history of precancerous lesions, which portends poor prognosis (12). Furthermore, Radiotherapy and chemotherapy often leads to severe side effects and reduces quality of life (13). Targeted therapy involves specific drugs that selectively bind to oncogenic targets within tumor cells, with minimal effects on the adjacent healthy tissues (14). Given the indispensable role of the TME in tumor progression, further research has been initiated into new therapeutic strategies that target TME for the treatment of HNSCC or other solid tumors.
2. Targeting the tumor microenvironment
Tumor microenvironment is a complex network of cellular and non-cellular components. Cancer is considered to be an evolutionary and ecological process involving continuous, dynamic and reciprocal interactions between cancer cells and TMEs (15). The TME is a key determinant of cancer prognosis and treatment outcomes (16). While the TME promotes tumor progression, the latter induces adaptations in the TME to facilitate its growth. These reciprocal interactions between tumor cells and the TME collectively shape the trajectory of the tumor (17). Furthermore, the TME becomes highly complex and heterogenous in the advanced stages of solid tumors (18). Therefore, it is crucial to elucidate the molecular interactions between tumor cells and the TME in order to identify potential therapeutic targets for cancer treatment. The key components of the TME that contribute to tumor progression, as well as the clinical studies on drugs targeting these components, have been discussed in the subsequent sections.
2.1. Targeting the extracellular matrix
The ECM is an intricate network of protein, polysaccharides, and glycoproteins that provides structural and biochemical support to the tissue. It is primarily composed of collagen, along with fibronectin, elastin, laminin, hyaluronic acid, chondroitin sulfate, keratan sulfate, and heparan sulfate (19). Dysregulation of the ECM is a hallmark feature of cancer (20). Tumor cells recruit and transform fibroblasts into CAFs, which contribute to excessive ECM deposition. CAFs are the predominant non-immune cells in the TME, and constitute up to 80% of the cells in advanced HNSCC tumors. While undifferentiated fibroblasts can suppress tumor growth, activated CAFs remodel the tumor stroma, and influence the behavior and invasiveness of HNSCC cells by producing soluble factors and ECM proteins (21, 22). Excessive collagen deposition and crosslinking of fibrillar collagen and elastin result in a dense and rigid ECM, leading to tissue stiffening (23). This protein network protects tumor cells from immune destruction and mediates treatment resistance. Moreover, the ECM promotes tumor progression by providing proliferative signals to the tumor cells, blocking growth-inhibitory factors, inducing angiogenesis, and facilitating the invasion and metastasis of tumor cells (24).
Given its critical role in HNSCC progression, the ECM represents an important therapeutic target. The TGF-β signaling pathway is involved in collagen synthesis (25), and drugs targeting TGF-β receptors have shown promising clinical effects. Fluorothiazinone (FT), a plant-derived anti-bacterial alkaloid, can inhibit collagen synthesis by inactivating the TGF-β/Smad2/3 signaling pathway (26). Wang et al. demonstrated that HF inhibited the proliferation of CAFs in oral squamous cell carcinoma (OSCC) by targeting the TGF-β/Smad2/3 pathway (27). Flumatinib (HF) has shown favorable clinical outcomes. Bintrafusp alfa, a bifunctional fusion protein targeting TGF-β and PD-L1, achieved promising clinical outcomes in a phase I trial in advanced HNSCC patients with a manageable safety profile. Darantelcept binds to activin receptor-like kinase 1 (ALK1), a TGF-β receptor expressed on activated endothelial cells, and blocks TGF-β signaling. It has demonstrated modest dose-dependent anti-cancer activity and a favorable safety profile in phase I clinical trials in patients with cisplatin-resistant, recurrent or metastatic HNSCC (RM-HNSCC), and may be tested further in combination with radiotherapy in RM-HNSCC patients (28).
CD44 is a receptor for hyaluronic acid, collagen, fibronectin and growth factors, and thus regulates signaling pathways related to cancer proliferation, invasion, metastasis, and treatment resistance (29). CD44 isoforms are overexpressed in various tumors, including HNSCC. Although targeted drugs like bivatuzumab mertansine (BIWI 1) have been explored in clinical trials, their severe skin toxic side effects have halted their development (30). The strong toxic side effects of BIWI 1 have forced the termination of research on this drug. However, CD44 plays an important role in tumor progression and has the potential to be a tumor therapeutic target, which may warrant more in-depth research in tumor therapy in the future.
In summary, dysregulation of the ECM contributes to cancer development and progression, and targeting the ECM and associated signaling pathways is a promising therapeutic strategy for HNSCC. However, further research and clinical studies are necessary to unravel the intricate interplay between tumor cells and the ECM in order to develop effective and safe targeted therapies against HNSCC.
2.2. Targeting tumor hypoxia
Tumor hypoxia (TH) is characterized by an increased demand for oxygen due to the rapid proliferation of tumor cells and is often associated with poor prognosis (31). It can be classified into acute and chronic hypoxia (32). Acute hypoxia is the result of insufficient oxygen supply to cells due to compromised blood vessels, while chronic hypoxia is primarily caused by limited oxygen diffusion into the tumor cells on account of the distance from blood vessels or restrictive geometric shapes (33). Chronic hypoxia is more common in solid tumors due to their expansive growth. For instance, the oxygen pressure within HNSCC tissue is <10 mm Hg compared to approximately 43 mm Hg in normal tissues (34).
Hypoxia exacerbates the malignant phenotype of tumor cells and inhibits apoptosis, thereby promoting tumor progression, invasion, metastasis, and treatment resistance (35, 36). Moreover, hypoxia-induced increase in glycolysis and carbon dioxide production acidifies the TME, which renders cells resistant to radiation and chemotherapy (37). Key endogenous hypoxia markers in tumors include hypoxia-inducible factor 1 (HIF-1), glucose transporter 1 (GLUT-1), carbonic anhydrase IX (CAIX), vascular endothelial growth factor (VEGF), and osteopontin (OPN) (38). HIF-1 is a heterodimeric transcription factor composed of a constitutively expressed β subunit and an oxygen-regulated α subunit. It is a major regulator of cellular oxygen homeostasis, and promotes angiogenesis in hypoxic tumor tissues by upregulating VEGF and promoting recruitment of mature endothelial cells (39). HIF-1 also induces glycolysis and GLUT-1 expression under hypoxic conditions to facilitate energy production (40, 41). OPN expression is induced under hypoxic conditions independent of the HIF pathway, and protects cells against hypoxia-triggered death (42). CAIX is a cell surface metalloenzyme that catalyzes the reversible conversion of carbon dioxide to bicarbonate (HCO3-) and H+, which maintains a favorable pH for tumor cell survival and growth. Furthermore, CAIX contributes to extracellular acidification, and promotes tumor cell migration, invasion, metastasis, and treatment resistance (43).
Hypoxia and TME acidification are contributing factors to HNSCC recurrence (44). Furthermore, hypoxic conditions promote epithelial-mesenchymal transition (EMT) of OSCC cells, leading to a significant decrease in E-cadherin mRNA levels and increased tumor cell migration (45). Clinical trials targeting HIF-1 and CAIX have been conducted extensively and have yielded some promising therapeutic results. Thus, HIF-1 and CAIX might be promising therapeutic targets for head and neck cancers. For instance, the HIF-1α inhibitor bortezomib has shown good tolerability in combination with bevacizumab in phase I trials for advanced refractory malignancies. It is effective against pre-treated advanced malignancies and inhibits tumor angiogenesis. Furthermore, clinical trials involving bortezomib in combination with docetaxel for androgen-independent prostate cancer and the combination of bortezomib and irinotecan for relapsed/refractory high-risk neuroblastoma have reported encouraging results (46, 47). Another HIF-1α inhibitor topotecan is currently being tested in clinical trials for late-stage solid tumors. After 1 week of treatment, DCE-MRI imaging demonstrated a reduction in tumor blood flow and permeability, indicating effective suppression of HIF-1α expression in late-stage solid tumors (48). The CAIX inhibitor SLC-0111 has shown a good safety profile in phase I trials for treatment-experienced patients with late-stage solid tumors, even at high doses of 1,000 mg per day. Some patients treated with SLC-0111 have exhibited prolonged stable disease (SD). In addition, SLC-0111 augmented the effects of immune checkpoint blockade in preclinical models of melanoma and breast cancer. Nevertheless, further clinical studies are warranted to explore the efficacy and safety of SLC-0111 in a larger patient population (49).
To summarize, inhibiting the HIF-1 and CAIX pathways in HNSCC and other solid tumors can disrupt the adaptive mechanisms of tumor cells to hypoxia, overcome treatment resistance, and augment the efficacy of existing therapies. However, it is crucial to fully elucidate the complex mechanisms underlying tumor hypoxia and develop effective and safe targeted therapies for HNSCC patients. In addition, combination of hypoxia-targeting agents with other treatment modalities, such as radiation and chemotherapy, should be explored to optimize treatment outcomes and improve patient survival.
2.3. Targeting tumor-promoting chronic inflammation
Inflammation is a dynamic defense mechanism that occurs in response to harmful stimuli, and involves biological, chemical, and physical factors. The primary objective of the inflammatory response is to eliminate damage and facilitate tissue regeneration (50). However, inflammation can also contribute to the progression of certain diseases by exacerbating tissue damage. Chronic inflammation in particular is characterized by prolonged cycles of tissue destruction and regeneration (51). Furthermore, inflammation plays a significant role in tumor development and progression, and tumor cells in turn can enhance inflammatory responses (52). The microenvironment of HNSCC is rich in inflammatory mediators that may promote tumorigenesis, and are therefore ideal targets for innovative cancer therapies.
2.3.1. Targeting the COX-2 pathway
Cyclooxygenase (COX) enzyme exists as COX-1 and COX-2 isoforms. It has the function of converting arachidonic acid into prostaglandins (PG) (53). COX-1 is constitutively expressed in most cells and is involved in physiological functions such as platelet aggregation. On the other hand, COX-2 is an inducible enzyme that is upregulated only in response to inflammation and other pathological stimuli. In addition, COX-2 is aberrantly expressed in pre-cancerous and cancerous lesions, and its overexpression can promote carcinogenesis (54). The oncogenic effect of COX-2 is primarily mediated through the release of the pro-inflammatory mediator PGE2 (55). Numerous studies have demonstrated the significant role of the COX-2/PGE2 pathway in the progression of HNSCC. High expression levels of COX-2 and PGE2 in HNSCC have been associated with worse prognosis, lymph node involvement, advanced histological grade, local tumor recurrence, and lower survival rate (56). COX-2 and PGE2 enhance migration of OSCC cells by upregulating intercellular adhesion molecule-1 (ICAM-1), a surface glycoprotein involved in cell-to-cell adhesion (57, 58). In addition, both COX-2 and PGE2 regulate tumor angiogenesis by modulating VEGF or directly influencing endothelial cell proliferation (59). COX-2 expression is also correlated with lymph node metastasis and disease progression in nasopharyngeal carcinoma (NPC), and co-expression of COX-2/VEGF-C in OSCC has been associated with the generation of lymphatic vessels (60, 61). Furthermore, PGE2 promotes the maturation of regulatory T cells (Tregs) and facilitates the recruitment of MDSCs to the tumor tissues, which suppresses the anti-tumor immune response and promotes tumor growth (62).
The above findings suggest that COX-2 is a promising therapeutic target in cancer (63). Indeed, the COX-2 inhibitors tested so far have demonstrated high treatment efficacy with acceptable side effects compared to traditional anti-cancer therapies (64). In addition, COX-2 inhibitors can also increase tumor sensitivity to radiation and chemotherapy. Due to the simultaneous inhibition of COX-1 and COX-2, non-selective NSAIDs not only fail to achieve the anti-inflammatory and analgesic purpose, but also cause serious adverse effects, such as gastrointestinal tract damage and platelet dysfunction. On the other hand, selective NSAIDs only inhibit COX-2 and does not affect the protective effects of COX-1-catalyzed prostaglandins on the gastrointestinal tract and platelets, thus greatly reducing the risk of gastrointestinal side effects (65). However, NSAIDs that selectively target COX-2, including celecoxib and rofecoxib, cause minimal damage to the GI, and have been widely tested in clinical trials (66). For instance, rofecoxib has been shown to reduce neo-angiogenesis in colorectal cancer patients with liver metastasis (67). In a phase II clinical trial evaluating the efficacy and safety of celecoxib in advanced cancer patients with cachexia, the body weight and tumor necrosis factor (TNF-α) levels improved following celecoxib treatment. These findings suggest that celecoxib could be an effective monotherapy for cancer-related cachexia (68).
2.3.2. Targeting tumor necrosis factor alpha
Tumor necrosis factor alpha (TNF-α) is a cytokine that plays a critical role in regulating inflammation, immunity, cellular homeostasis, and tumor progression (69). Recent studies show that TNF-α is one of the key mediators of cancer-related inflammation and acts as a tumor-promoting factor (70). It exerts its effects through TNF receptor 2 (TNFR-2) and TNF receptor 1 (TNFR-1). While TNFR-2 has higher affinity, it is mainly expressed on immune cells. On the other hand, TNFR-1 is expressed ubiquitously and initiates most of the biological activities of TNF-α (71). In addition, TNFR-1 is a dual-action receptor that relays both apoptotic and survival signals, and TNFR-1 activation also contributes to pro-inflammatory responses (72). Overexpression of TNF-α in HNSCC is associated with higher proliferative potency (73), and inhibiting TNF-α in oral cancer suppressed tumor growth. Furthermore, TNF-α is a mediator of pain perception and inflammation in oral cancer, and TNF-α blockade can potentially alleviate oral cancer-related pain (74, 75). TNF-α promotes HNSCC progression by upregulating MMP-9, which in turn enhances tumor migration and invasion by facilitating TGF-β1-induced EMT (76, 77). Moreover, TNF-α also increases the metastatic potential of HNSCC cells by upregulating CCR6 and CXCR-4 (78, 79).
Tumor necrosis factor alpha inhibitors, such as infliximab and etanercept, have been widely evaluated in clinical trials for various cancers and have shown promising results. In a phase II trial, infliximab showed therapeutic effects in renal cell carcinoma (RCC) patients. Infliximab may inhibit tumor cell proliferation by neutralizing TNF-α or inducing TNF-α-dependent apoptosis by depriving cells of the cytokine. Lower circulating levels of TNF-α can stabilize tumor growth (80). In another clinical study evaluating tolerance and biological effects in advanced cancer patients, infliximab was found to be safe and well-tolerated without dose-limiting toxic reactions. Etanercept has also demonstrated therapeutic efficacy and safety in phase II studies on recurrent ovarian cancer and metastatic breast cancer (81).
2.4. Targeting the tumor immune system
Immune cells are an important component of the TME, and exert both anti-tumorigenic and pro-tumorigenic effects. The MDSCs and TAMs are immunosuppressive cells that promote tumor growth and aid in immune evasion. The role of these cell types in HNSCC and their therapeutic potential have been discussed in greater detail below.
2.4.1. Targeting tumor-associated macrophages
Most TAMs originate from the bone marrow and infiltrate into the tumor via peripheral blood (82). Macrophages can be classified into the classically activated M1 macrophages and alternatively activated M2 macrophages. M1 macrophages exhibit enhanced antigen presentation and lysosomal activity, and promote Th1 responses. They also secrete chemokines (e.g., TNF-α, iNOS) involved in immune activation and phagocytosis to counteract tumor growth. TAMs predominantly display the M2 phenotype, and produce pro-oncogenic factors (IL-10, IL-4, TGF-β, VEGF, and MMP) that drive tumor growth, metastasis, angiogenesis, and immune evasion (83, 84).
Haque et al. (85) found that CD206+ TAMs promote proliferation of oral tumor cells through EGF signaling. In addition, TAMs also play a role in regulating the adhesion, migration, and invasion of HNSCC cells, promote metastasis by supporting the generation of blood vessels and lymphatic vessels, and contribute to tumor progression via immunosuppression (86). In laryngeal squamous cell carcinoma, M2 macrophages activate JAK/STAT signaling to produce IL-10, which upregulates the immune checkpoint PD-L1. TAMs can directly inhibit T cell activation and proliferation, and induce T cell apoptosis via PD-L1. Immune checkpoint blockade through PD-1/PD-L1 inhibitors has been highly effective in various cancers (87).
Head and neck squamous cell carcinoma cells and TAMs have a mutually synergistic relationship. While the tumor cells release CCL2 to recruit monocytes and induce their differentiation and polarization to M2 macrophages, the latter release epidermal growth factor (EGF) that upregulates CCL2 expression in tumor cells (88). Numerous TAM-targeting drugs have been developed that are currently in the clinical phase of testing. CCL2/CCR2 inhibitors, such as carlumab (CNTO 888) (89) and PF-04136309 (90), modulate macrophage recruitment and differentiation, and have been tested in clinical trials. CSF-1 receptor (CSF-1R), a transmembrane tyrosine kinase receptor, plays a crucial role in regulating TAM development, morphology, survival, and function after binding with CSF-1 (91). Several CSF-1 and CSF-1R inhibitors, such as emactuzumab (RG-7155) (92), AMG-820 (93), and pexidartinib (PLX3397) (94), are currently undergoing clinical trials. In addition, reprogramming TAMs from the pro-tumor M2 phenotype to the anti-tumor M1 phenotype is a promising therapeutic strategy. RRx-001 is an SIRP-a and CD47 inhibitor that can repolarize TAMs to the M1 phenotype, and clinical trials conducted so far on cancer patients have been encouraging (95). Inhibitors of the CCL2/CCR2 axis and CSF-1/CSF-1R signaling also modulate macrophage recruitment and differentiation, and have shown promising results in preclinical and clinical studies. Therefore, elucidating the complex interactions between TAMs and the TME will help in the development of effective therapies for HNSCC and other cancers (Table 1).
2.4.2. Targeting myeloid-derived suppressor cells
Myeloid-derived suppressor cells constitute a heterogeneous population of cells that morphologically resemble immature granulocytes, monocytes, and dendritic cells (DCs) (96). The MDSCs are normally scarce but their numbers increase significantly during early or advanced stages of cancer (97). MDSCs are primarily recruited to the TME through the CXCR2 ligand, which is overexpressed in various cancers (98). They inhibit T cell-mediated immunity through multiple mechanisms. For instance, MDSCs interfere with the supply of amino acids (such as L-arginine and L-citrulline), which is necessary for T cell proliferation and activation. In addition, the MDSCs produce high levels of reactive oxygen species (ROS), which interact with nitric oxide (NO) to generate peroxynitrite (ONOO−) radicals that inhibit T cell activation and proliferation (99). Moreover, MDSCs promote tumor angiogenesis by expressing VEGF, and the latter recruits MDSCs through the VEGF receptor (VEGFR) expressed on the cells’ surface (100).
The accumulation of MDSCs in the tumor tissues is closely associated with clinical outcomes and generally indicates poor prognosis. MDSCs are abundant in HNSCC tissues (101), and promote tumor progression in HNSCC through various mechanisms, including proliferation, apoptosis resistance, migration, invasion, EMT, and vasculogenic mimicry formation (VM). Tumor cells also induce immunosuppression by upregulating arginase 1 (ARG1) and inducible nitric oxide synthase (iNOS) in the MDSCs (102). Detection of circulating MDSCs in patients with thyroid nodules using flow cytometry is a novel approach for the evaluating cancer risk and severity, and may even serve as a useful tool for predicting the tumor stage and recurrence risk of HNSCC (103). Fugle et al. (104) demonstrated that functional inhibition of MDSCs in mice delayed the onset of oral cancer. Signal transducer and activator of transcription 1 (STAT1) is a transcription factor involved in a wide variety of immunological responses (104). Ryan et al. (105) showed that inhibiting accumulation of MDSCs in HNSCC through STAT1 promotion facilitated T cell-mediated anti-tumor immune response.
Current treatment strategies targeting MDSCs mainly focus on (1) depletion of MDSCs, (2) inducing differentiation and maturation of MDSCs, and (3) inhibition of the immunosuppressive functions of MDSCs. For instance, most colorectal cancer patients showed a decrease in MDSC numbers after first-line combination therapy with 5-fluorouracil, oxaliplatin, and bevacizumab (FOLFOX-bevacizumab), which was associated with improved survival outcomes (106). Furthermore, all-trans retinoic acid (ATRA) can induceefore decrease their numbers in circulation. The combination of ipilimumab and ATRA significantly reduced the number of circulating MDSCs in melanoma patients compared to ipilimumab monotherapy (107). Nrf2 plays a crucial role in regulating the expression of antioxidant enzymes and protects cells against free radical damage. The synthetic triterpenoid compound CCDO-Me reduced the production of ROS by MDSCs through Nrf2 upregulation, and reversed their immunosuppressive effects (108, 109). Several drugs that target the above aspects of MDSCs are currently in clinical trials. Furthermore, phosphodiesterase-5 (PDE5) inhibitors such as sildenafil, tadalafil, and vardenafil can reduce the levels of ARG1 and iNOS, thereby reversing MDSC-mediated immune suppression, reducing inflammation in the TME, and reactivating anti-tumor T cells and NK cells (110–112). In one clinical trial, tadalafil significantly reduced the number of intra-tumoral and circulating MDSCs and Tregs in HNSCC patients, and was well-tolerated. Chemotherapeutic agents can also effectively deplete MDSCs.
2.5. Targeting tumor angiogenesis
The rapid proliferation of tumor cells is accompanied by generation of new blood vessels that supply adequate nutrients, oxygen, and growth factors for sustaining tumor growth and facilitating dissemination of tumor cells (113, 114). Neo-angiogenesis involves tumor endothelial cells (TECs) and surrounding perivascular cells. TECs exhibit genetic abnormalities and are resistant to anti-angiogenic drugs (115). Naito et al. (116) showed that the recalcitrance of TECs to antiangiogenic drugs may contribute to tumor resistance. In addition, endothelial cells play a significant role in tumor progression and metastasis. The hypoxic conditions in the tumor tissue induce the production of VEGF, which initiates tumor angiogenesis and confers resistance to hypoxia. VEGF exerts its effect upon binding to its receptors (VEGFR-1, VEGFR-2, and VEGFR-3). VEGFR-1 and VEGFR-2 are expressed in the blood vessels, while VEGFR-3 is expressed in the lymphatic endothelium (117). Elevated VEGF expression in HNSCC has diagnostic and prognostic value. VEGF activates the VEGF receptors on the surface of the neighboring endothelial cells through paracrine signaling, which stimulates their migration and proliferation, and induces angiogenesis (118). During neovascular expansion, endothelial cells expressing high levels of VEGFR become tip cells and promote angiogenesis by interacting with delta-like ligand 4 (DLL4) and angiopoietin 2 (ANGPT2) (119). Sun et al. (120) demonstrated that inhibition of VEGF/VEGFR2 signaling with the flavonoid B2PB2 suppressed angiogenesis and growth in the OSCC cell line SCC-25. It also decreased the viability, invasion, migration, and EMT of the tumor cells, and promoted apoptosis (120). Under normal circumstances, endothelial cells remain quiescent and proliferate once every 150 days. However, increased expression of VEGF in response to various pathological stimuli can induce endothelial cell-mediated angiogenesis. Chen et al. and Wu et al. have shown that inhibiting VEGF expression can suppress migration and angiogenesis in NPC cells (121, 122). Anti-angiogenic drugs targeting VEGF/VEGFR, including bevacizumab (123), apatinib (124), vandetanib (125), AMG 706 (126), pazopanib (127), axitinib (128), famitinib (129), lenvatinib (130), cabozantinib (131), and regorafenib (132). Most of these drugs have shown therapeutic effects against various cancers, and could be considered for HNSCC treatment. There are others that need to be further explored because of toxicity or efficacy (Table 2).
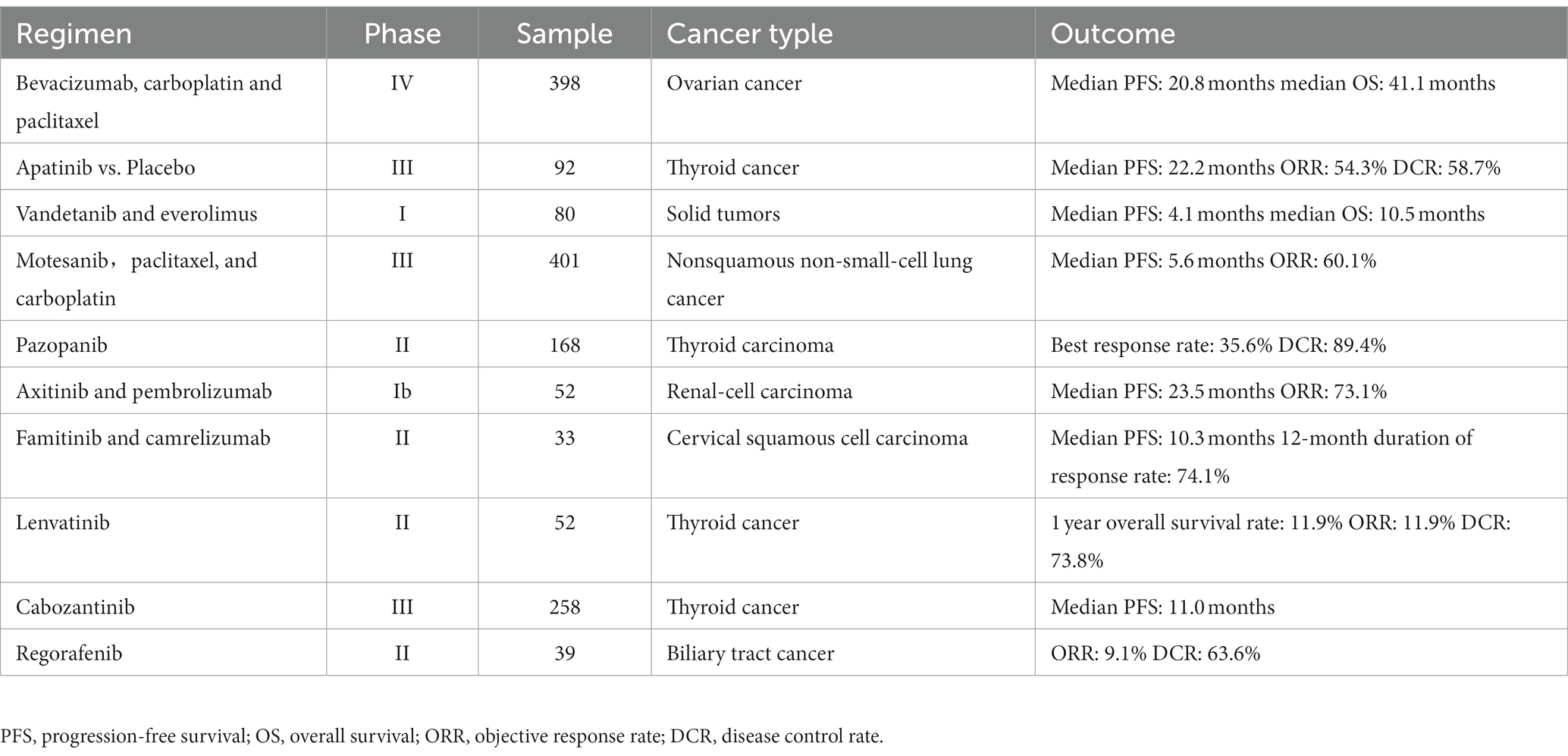
Table 2. Antiangiogenic agents targeting vascular endothelial growth factor signaling in clinical trials.
2.6. Targeting other factors in the TME
In addition to the above TME components, there are many other TME components (e.g., cancer stem cells, microorganism, and mechanical microenvironment) that have received less attention but may also be therapeutic targets for tumors. Cancer Stem Cells (CSCs) constitute a small portion of malignant cells and serve as tumor-initiation cells, propelling tumor development (133). CSCs possess a range of functions, including plasticity, quiescence, and self-renewal, enabling them to regulate tumor growth, metastasis, survival, recurrence, and resistance to cancer treatment through specific signaling pathways (134, 135). Specific molecules have been identified as markers for CSCs in HNSCC, such as Aldehyde Dehydrogenase (ALDH) and CD44. ALDH+ CD44+ cancer cells are considered CSCs in HNSCC and exhibit increased tumorigenicity through the aberrant activation of the PI3K/mTOR signaling pathway and upregulation of SOX2 expression (136). The tumor microenvironment (TME) harbors microorganisms, and the microbial communities that influence tumor progression and are associated with tumors are referred to as the tumor microbiota (137). Tumors can create more suitable conditions for microbial survival and remodeling of microbial profiles, while microbes can also contribute to tumorigenesis and progression by establishing an inflammatory milieu and influencing host immunity, and unlike normal tissues where the balance of the microbiota contributes to the defense against tissue pathology, the microbiota in the TME affects tumor progression and therapy (138). The mechanical microenvironment is also part of the TME.
3. Conclusion
In this review, we have summarized the role of hypoxia, inflammatory response, immune cells, and angiogenesis in the progression of HNSCC, and discussed novel therapeutic strategies targeting these components. In recent decades, the focus of cancer treatment has steadily shifted to the TME, and numerous clinical trials are currently underway to validate the efficacy and safety of anti-cancer agents targeting the cells and factors that comprise the TME. Several of these targeted therapies have demonstrated promising clinical outcomes. However, disrupting the interactions between tumor cells and the TME often yield suboptimal results. It has been realized that TME is a complex ecosystem, full of heterogeneity, that can affect almost every aspect of cancer biology. At the same time, the advantages of targeted drugs over conventional drugs have been deeply understood, and the efficacy of many drugs targeting the TME in tumors has brought home the potential of the TME for tumor therapy. Therefore, there is an urgent need to elucidate the relationship between HNSCC and TME in more detail, with a focus on targeting the key components that promote tumor growth within the TME, to find more targets for treating tumors, to improve and refine the drugs in current clinical trials, and to develop more effective antitumor strategies.
Author contributions
ZG: Conceptualization, Investigation, Writing – original draft. KL: Conceptualization, Investigation, Writing – original draft. PL: Conceptualization, Investigation, Writing – original draft. XZ: Writing – original draft. JL: Project administration, Supervision, Writing – review & editing. XZ: Funding acquisition, Project administration, Supervision, Writing – review & editing. PZ: Funding acquisition, Project administration, Supervision, Writing – review & editing.
Funding
The author(s) declare financial support was received for the research, authorship, and/or publication of this article.
The present study was supported in part by Guangdong Basic and Applied Basic Research Foundation (2021A1515010970); Shenzhen Innovation of Science and Technology Commission (No. JCYJ20210324132407019, LGKCYLWS2022002, LGWJ2021-118, LGKCYLWS2021000027); and Shenzhen Key Medical Discipline Construction Fund (No. SZXK039).
Conflict of interest
The authors declare that the research was conducted in the absence of any commercial or financial relationships that could be construed as a potential conflict of interest.
Publisher’s note
All claims expressed in this article are solely those of the authors and do not necessarily represent those of their affiliated organizations, or those of the publisher, the editors and the reviewers. Any product that may be evaluated in this article, or claim that may be made by its manufacturer, is not guaranteed or endorsed by the publisher.
References
1. Shibata, H, Zhou, L, Xu, N, Egloff, AM, and Uppaluri, R. Personalized cancer vaccination in head and neck cancer. Cancer Sci. (2021) 112:978–88. doi: 10.1111/cas.14784
2. Leemans, CR, Braakhuis, BJ, and Brakenhoff, RH. The molecular biology of head and neck cancer. Nat Rev Cancer. (2011) 11:9–22. doi: 10.1038/nrc2982
3. Gillison, ML. Human papillomavirus and prognosis of oropharyngeal squamous cell carcinoma: implications for clinical research in head and neck cancers. J Clin Oncol. (2006) 24:5623–5. doi: 10.1200/JCO.2006.07.1829
4. Jou, A, and Hess, J. Epidemiology and molecular biology of head and neck cancer. Oncol Res Treat. (2017) 40:328–32. doi: 10.1159/000477127
6. Fan, S, Tang, QL, Lin, YJ, Chen, WL, Li, JS, Huang, ZQ, et al. A review of clinical and histological parameters associated with contralateral neck metastases in oral squamous cell carcinoma. Int J Oral Sci. (2011) 3:180–91. doi: 10.4248/IJOS11068
7. Canning, M, Guo, G, Yu, M, Myint, C, Groves, MW, Byrd, JK, et al. Heterogeneity of the head and neck squamous cell carcinoma immune landscape and its impact on immunotherapy. Front Cell Dev Biol. (2019) 7:52. doi: 10.3389/fcell.2019.00052
8. Wu, T, and Dai, Y. Tumor microenvironment and therapeutic response. Cancer Lett. (2017) 387:61–8. doi: 10.1016/j.canlet.2016.01.043
9. Chen, F, Zhuang, X, Lin, L, Yu, P, Wang, Y, Shi, Y, et al. New horizons in tumor microenvironment biology: challenges and opportunities. BMC Med. (2015) 13:45. doi: 10.1186/s12916-015-0278-7
10. Hanahan, D. Hallmarks of cancer: new dimensions. Cancer Discov. (2022) 12:31–46. doi: 10.1158/2159-8290.CD-21-1059
11. Bilotta, MT, Antignani, A, and Fitzgerald, DJ. Managing the tme to improve the efficacy of cancer therapy. Front Immunol. (2022) 13:954992. doi: 10.3389/fimmu.2022.954992
12. Johnson, DE, Burtness, B, Leemans, CR, Lui, V, Bauman, JE, and Grandis, JR. Head and neck squamous cell carcinoma. Nat Rev Dis Primers. (2020) 6:92. doi: 10.1038/s41572-020-00224-3
13. Caponigro, F, Longo, F, Ionna, F, and Perri, F. Treatment approaches to nasopharyngeal carcinoma: a review. Anti-Cancer Drugs. (2010) 21:471–7. doi: 10.1097/CAD.0b013e328337160e
14. Kang, Y, He, W, Ren, C, Qiao, J, Guo, Q, Hu, J, et al. Advances in targeted therapy mainly based on signal pathways for nasopharyngeal carcinoma. Signal Transduct Target Ther. (2020) 5:245. doi: 10.1038/s41392-020-00340-2
15. Xiao, Y, and Yu, D. Tumor microenvironment as a therapeutic target in cancer. Pharmacol Ther. (2021) 221:107753. doi: 10.1016/j.pharmthera.2020.107753
16. Netea-Maier, RT, Smit, J, and Netea, MG. Metabolic changes in tumor cells and tumor-associated macrophages: a mutual relationship. Cancer Lett. (2018) 413:102–9. doi: 10.1016/j.canlet.2017.10.037
17. Bao, L, Xu, T, Lu, X, Huang, P, Pan, Z, and Ge, M. Metabolic reprogramming of thyroid cancer cells and crosstalk in their microenvironment. Front Oncol. (2021) 11:773028. doi: 10.3389/fonc.2021.773028
18. El-Sayes, N, Vito, A, and Mossman, K. Tumor heterogeneity: a great barrier in the age of cancer immunotherapy. Cancers. (2021) 13:806. doi: 10.3390/cancers13040806
19. Walker, C, Mojares, E, and Del, RHA. Role of extracellular matrix in development and cancer progression. Int J Mol Sci. (2018) 19:13028. doi: 10.3390/ijms19103028
20. Xu, M, Zhang, T, Xia, R, Wei, Y, and Wei, X. Targeting the tumor stroma for cancer therapy. Mol Cancer. (2022) 21:208. doi: 10.1186/s12943-022-01670-1
21. Romano, V, Belviso, I, Venuta, A, Ruocco, MR, Masone, S, Aliotta, F, et al. Influence of tumor microenvironment and fibroblast population plasticity on melanoma growth, therapy resistance and immunoescape. Int J Mol Sci. (2021) 22:5283. doi: 10.3390/ijms22105283
22. Avagliano, A, Fiume, G, Bellevicine, C, Troncone, G, Venuta, A, Acampora, V, et al. Thyroid cancer and fibroblasts. Cancers. (2022) 14:4172. doi: 10.3390/cancers14174172
23. Huang, J, Zhang, L, Wan, D, Zhou, L, Zheng, S, Lin, S, et al. Extracellular matrix and its therapeutic potential for cancer treatment. Signal Transduct Target Ther. (2021) 6:153. doi: 10.1038/s41392-021-00544-0
24. Jiang, Y, Zhang, H, Wang, J, Liu, Y, Luo, T, and Hua, H. Targeting extracellular matrix stiffness and mechanotransducers to improve cancer therapy. J Hematol Oncol. (2022) 15:34. doi: 10.1186/s13045-022-01252-0
25. Pallasch, FB, and Schumacher, U. Angiotensin inhibition, tgf-beta and emt in cancer. Cancers. (2020) 12:2785. doi: 10.3390/cancers12102785
26. Marty, P, Chatelain, B, Lihoreau, T, Tissot, M, Dirand, Z, Humbert, P, et al. Halofuginone regulates keloid fibroblast fibrotic response to tgf-beta induction. Biomed Pharmacother. (2021) 135:111182. doi: 10.1016/j.biopha.2020.111182
27. Wang, D, Tian, M, Fu, Y, Sun, Y, Ding, L, Zhang, X, et al. Halofuginone inhibits tumor migration and invasion by affecting cancer-associated fibroblasts in oral squamous cell carcinoma. Front Pharmacol. (2022) 13:1056337. doi: 10.3389/fphar.2022.1056337
28. Cho, BC, Daste, A, Ravaud, A, Salas, S, Isambert, N, McClay, E, et al. Bintrafusp alfa, a bifunctional fusion protein targeting tgf-beta and pd-l1, in advanced squamous cell carcinoma of the head and neck: results from a phase i cohort. J Immunother Cancer. (2020) 8:e000664. doi: 10.1136/jitc-2020-000664
29. Xu, H, Niu, M, Yuan, X, Wu, K, and Liu, A. Cd44 as a tumor biomarker and therapeutic target. Exp Hematol Oncol. (2020) 9:36. doi: 10.1186/s40164-020-00192-0
30. Stroomer, JW, Roos, JC, Sproll, M, Quak, JJ, Heider, KH, Wilhelm, BJ, et al. Safety and biodistribution of 99mtechnetium-labeled anti-cd44v6 monoclonal antibody biwa 1 in head and neck cancer patients. Clin Cancer Res. (2000) 6:3046–55.
31. Nordsmark, M, Bentzen, SM, Rudat, V, Brizel, D, Lartigau, E, Stadler, P, et al. Prognostic value of tumor oxygenation in 397 head and neck tumors after primary radiation therapy. Radiother Oncol. (2005) 77:18–24. doi: 10.1016/j.radonc.2005.06.038
32. Vaupel, P, and Mayer, A. Hypoxia in cancer: significance and impact on clinical outcome. Cancer Metastasis Rev. (2007) 26:225–39. doi: 10.1007/s10555-007-9055-1
33. Hockel, M, and Vaupel, P. Tumor hypoxia: definitions and current clinical, biologic, and molecular aspects. J Natl Cancer Inst. (2001) 93:266–76. doi: 10.1093/jnci/93.4.266
34. Lartigau, E, Le Ridant, AM, Lambin, P, Weeger, P, Martin, L, Sigal, R, et al. Oxygenation of head and neck tumors. Cancer. (1993) 71:2319–25. doi: 10.1002/1097-0142(19930401)71:7<2319::AID-CNCR2820710724>3.0.CO;2-C
35. Karar, J, and Maity, A. Modulating the tumor microenvironment to increase radiation responsiveness. Cancer Biol Ther. (2009) 8:1994–2001. doi: 10.4161/cbt.8.21.9988
36. Petrova, V, Annicchiarico-Petruzzelli, M, Melino, G, and Amelio, I. The hypoxic tumor microenvironment. Oncogene. (2018) 7:10. doi: 10.1038/s41389-017-0011-9
37. Vaupel, P. Tumor microenvironmental physiology and its implications for radiation oncology. Semin Radiat Oncol. (2004) 14:198–206. doi: 10.1016/j.semradonc.2004.04.008
38. Gottgens, EL, Ostheimer, C, Span, PN, Bussink, J, and Hammond, EM. Hpv, hypoxia and radiation response in head and neck cancer. Br J Radiol. (2019) 92:20180047. doi: 10.1259/bjr.20180047
39. Dery, MA, Michaud, MD, and Richard, DE. Hypoxia-inducible factor 1: regulation by hypoxic and non-hypoxic activators. Int J Biochem Cell Biol. (2005) 37:535–40. doi: 10.1016/j.biocel.2004.08.012
40. Hewitson, KS, and Schofield, CJ. The hif pathway as a therapeutic target. Drug Discov Today. (2004) 9:704–11. doi: 10.1016/S1359-6446(04)03202-7
41. Vaupel, P. The role of hypoxia-induced factors in tumor progression. Oncologist. (2004) 9:10–7. doi: 10.1634/theoncologist.9-90005-10
42. Bandopadhyay, M, Bulbule, A, Butti, R, Chakraborty, G, Ghorpade, P, Ghosh, P, et al. Osteopontin as a therapeutic target for cancer. Expert Opin Ther Targets. (2014) 18:883–95. doi: 10.1517/14728222.2014.925447
43. Pastorekova, S, and Gillies, RJ. The role of carbonic anhydrase ix in cancer development: links to hypoxia, acidosis, and beyond. Cancer Metastasis Rev. (2019) 38:65–77. doi: 10.1007/s10555-019-09799-0
44. Curtis, KK, Wong, WW, and Ross, HJ. Past approaches and future directions for targeting tumor hypoxia in squamous cell carcinomas of the head and neck. Crit Rev Oncol Hematol. (2016) 103:86–98. doi: 10.1016/j.critrevonc.2016.05.005
45. Joseph, JP, Harishankar, MK, Pillai, AA, and Devi, A. Hypoxia induced emt: a review on the mechanism of tumor progression and metastasis in oscc. Oral Oncol. (2018) 80:23–32. doi: 10.1016/j.oraloncology.2018.03.004
46. Dreicer, R, Petrylak, D, Agus, D, Webb, I, and Roth, B. Phase i/ii study of bortezomib plus docetaxel in patients with advanced androgen-independent prostate cancer. Clin Cancer Res. (2007) 13:1208–15. doi: 10.1158/1078-0432.CCR-06-2046
47. Falchook, GS, Wheler, JJ, Naing, A, Jackson, EF, Janku, F, Hong, D, et al. Targeting hypoxia-inducible factor-1alpha (hif-1alpha) in combination with antiangiogenic therapy: a phase i trial of bortezomib plus bevacizumab. Oncotarget. (2014) 5:10280–92. doi: 10.18632/oncotarget.2163
48. Kummar, S, Raffeld, M, Juwara, L, Horneffer, Y, Strassberger, A, Allen, D, et al. Multihistology, target-driven pilot trial of oral topotecan as an inhibitor of hypoxia-inducible factor-1alpha in advanced solid tumors. Clin Cancer Res. (2011) 17:5123–31. doi: 10.1158/1078-0432.CCR-11-0682
49. McDonald, PC, Chia, S, Bedard, PL, Chu, Q, Lyle, M, Tang, L, et al. A phase 1 study of slc-0111, a novel inhibitor of carbonic anhydrase ix, in patients with advanced solid tumors. Am J Clin Oncol. (2020) 43:484–90. doi: 10.1097/COC.0000000000000691
50. Leibovici, D, Grossman, HB, Dinney, CP, Millikan, RE, Lerner, S, Wang, Y, et al. Polymorphisms in inflammation genes and bladder cancer: from initiation to recurrence, progression, and survival. J Clin Oncol. (2005) 23:5746–56. doi: 10.1200/JCO.2005.01.598
51. Karin, M, and Clevers, H. Reparative inflammation takes charge of tissue regeneration. Nature. (2016) 529:307–15. doi: 10.1038/nature17039
52. Wang, K, and Karin, M. Tumor-elicited inflammation and colorectal cancer. Adv Cancer Res. (2015) 128:173–96. doi: 10.1016/bs.acr.2015.04.014
53. Mendes, RA, Carvalho, JF, and Waal, I. An overview on the expression of cyclooxygenase-2 in tumors of the head and neck. Oral Oncol. (2009) 45:e124–8. doi: 10.1016/j.oraloncology.2009.03.016
54. Hamakawa, H, Nakashiro, K, Sumida, T, Shintani, S, Myers, JN, Takes, RP, et al. Basic evidence of molecular targeted therapy for oral cancer and salivary gland cancer. Head Neck. (2008) 30:800–9. doi: 10.1002/hed.20830
55. Yang, CC, and Chang, KW. Eicosanoids and hb-egf/egfr in cancer. Cancer Metastasis Rev. (2018) 37:385–95. doi: 10.1007/s10555-018-9746-9
56. Byatnal, AA, Byatnal, A, Sen, S, Guddattu, V, and Solomon, MC. Cyclooxygenase-2--an imperative prognostic biomarker in oral squamous cell carcinoma--an immunohistochemical study. Pathol Oncol Res. (2015) 21:1123–31. doi: 10.1007/s12253-015-9940-9
57. Lawson, C, and Wolf, S. Icam-1 signaling in endothelial cells. Pharmacol Rep. (2009) 61:22–32. doi: 10.1016/S1734-1140(09)70004-0
58. Yang, SF, Chen, MK, Hsieh, YS, Chung, TT, Hsieh, YH, Lin, CW, et al. Prostaglandin e2/ep1 signaling pathway enhances intercellular adhesion molecule 1 (icam-1) expression and cell motility in oral cancer cells. J Biol Chem. (2010) 285:29808–16. doi: 10.1074/jbc.M110.108183
59. Gately, S, and Li, WW. Multiple roles of cox-2 in tumor angiogenesis: a target for antiangiogenic therapy. Semin Oncol. (2004) 31:2–11. doi: 10.1053/j.seminoncol.2004.03.040
60. Morita, Y, Morita, N, Hata, K, Nakanishi, M, Kimoto, N, Omata, T, et al. Cyclooxygenase-2 expression is associated with vascular endothelial growth factor-c and lymph node metastasis in human oral tongue cancer. Oral Surg Oral Med Oral Pathol Oral Radiol. (2014) 117:502–10. doi: 10.1016/j.oooo.2013.12.410
61. Yang, G, Deng, Q, Fan, W, Zhang, Z, Xu, P, Tang, S, et al. Cyclooxygenase-2 expression is positively associated with lymph node metastasis in nasopharyngeal carcinoma. PLoS One. (2017) 12:e173641. doi: 10.1371/journal.pone.0173641
62. Liu, B, Qu, L, and Yan, S. Cyclooxygenase-2 promotes tumor growth and suppresses tumor immunity. Cancer Cell Int. (2015) 15:106. doi: 10.1186/s12935-015-0260-7
63. Lin, DT, Subbaramaiah, K, Shah, JP, Dannenberg, AJ, and Boyle, JO. Cyclooxygenase-2: a novel molecular target for the prevention and treatment of head and neck cancer. Head Neck. (2002) 24:792–9. doi: 10.1002/hed.10108
64. Nasry, W, Rodriguez-Lecompte, JC, and Martin, CK. Role of cox-2/pge2 mediated inflammation in oral squamous cell carcinoma. Cancers. (2018) 10:348. doi: 10.3390/cancers10100348
65. Ju, Z, Li, M, Xu, J, Howell, DC, Li, Z, and Chen, FE. Recent development on cox-2 inhibitors as promising anti-inflammatory agents: the past 10 years. Acta Pharm Sin B. (2022) 12:2790–807. doi: 10.1016/j.apsb.2022.01.002
66. Dannenberg, AJ, Lippman, SM, Mann, JR, Subbaramaiah, K, and DuBois, RN. Cyclooxygenase-2 and epidermal growth factor receptor: pharmacologic targets for chemoprevention. J Clin Oncol. (2005) 23:254–66. doi: 10.1200/JCO.2005.09.112
67. Fenwick, SW, Toogood, GJ, Lodge, JP, and Hull, MA. The effect of the selective cyclooxygenase-2 inhibitor rofecoxib on human colorectal cancer liver metastases. Gastroenterology. (2003) 125:716–29. doi: 10.1016/S0016-5085(03)01061-8
68. Mantovani, G, Maccio, A, Madeddu, C, Serpe, R, Antoni, G, Massa, E, et al. Phase ii nonrandomized study of the efficacy and safety of cox-2 inhibitor celecoxib on patients with cancer cachexia. J Mol Med. (2010) 88:85–92. doi: 10.1007/s00109-009-0547-z
69. Croft, M, Benedict, CA, and Ware, CF. Clinical targeting of the tnf and tnfr superfamilies. Nat Rev Drug Discov. (2013) 12:147–68. doi: 10.1038/nrd3930
70. Mantovani, A, Allavena, P, Sica, A, and Balkwill, F. Cancer-related inflammation. Nature. (2008) 454:436–44. doi: 10.1038/nature07205
71. Al-Lamki, RS, Sadler, TJ, Wang, J, Reid, MJ, Warren, AY, Movassagh, M, et al. Tumor necrosis factor receptor expression and signaling in renal cell carcinoma. Am J Pathol. (2010) 177:943–54. doi: 10.2353/ajpath.2010.091218
72. Mercogliano, MF, Bruni, S, Mauro, F, Elizalde, PV, and Schillaci, R. Harnessing tumor necrosis factor alpha to achieve effective cancer immunotherapy. Cancers. (2021) 13:564. doi: 10.3390/cancers13030564
73. Si, H, Lu, H, Yang, X, Mattox, A, Jang, M, Bian, Y, et al. Tnf-alpha modulates genome-wide redistribution of deltanp63alpha/tap73 and nf-kappab crel interactive binding on tp53 and ap-1 motifs to promote an oncogenic gene program in squamous cancer. Oncogene. (2016) 35:5781–94. doi: 10.1038/onc.2016.112
74. Qiu, YF, Wang, MX, Meng, LN, Zhang, R, and Wang, W. Mir-21 regulates proliferation and apoptosis of oral cancer cells through tnf-alpha. Eur Rev Med Pharmacol Sci. (2018) 22:7735–41. doi: 10.26355/eurrev_201811_16395
75. Salvo, E, Tu, NH, Scheff, NN, Dubeykovskaya, ZA, Chavan, SA, Aouizerat, BE, et al. Tnfalpha promotes oral cancer growth, pain, and schwann cell activation. Sci Rep. (2021) 11:1840. doi: 10.1038/s41598-021-81500-4
76. Li, Y, He, J, Wang, F, Wang, X, Yang, F, Zhao, C, et al. Role of mmp-9 in epithelial-mesenchymal transition of thyroid cancer. World J Surg Oncol. (2020) 18:181. doi: 10.1186/s12957-020-01958-w
77. Hohberger, L, Wuertz, BR, Xie, H, Griffin, T, and Ondrey, F. Tnf-alpha drives matrix metalloproteinase-9 in squamous oral carcinogenesis. Laryngoscope. (2008) 118:1395–9. doi: 10.1097/MLG.0b013e318174e09b
78. Han, N, Li, X, Wang, Y, Wang, L, Zhang, C, Zhang, Z, et al. Increased tumor-infiltrating plasmacytoid dendritic cells promote cancer cell proliferation and invasion via tnf-alpha/nf-kappab/cxcr-4 pathway in oral squamous cell carcinoma. J Cancer. (2021) 12:3045–56. doi: 10.7150/jca.55580
79. Coperchini, F, Pignatti, P, Carbone, A, Bongianino, R, Di Buduo, CA, Leporati, P, et al. Tnf-alpha increases the membrane expression of the chemokine receptor ccr6 in thyroid tumor cells, but not in normal thyrocytes: potential role in the metastatic spread of thyroid cancer. Tumour Biol. (2016) 37:5569–75. doi: 10.1007/s13277-015-4418-7
80. Harrison, ML, Obermueller, E, Maisey, NR, Hoare, S, Edmonds, K, Li, NF, et al. Tumor necrosis factor alpha as a new target for renal cell carcinoma: two sequential phase ii trials of infliximab at standard and high dose. J Clin Oncol. (2007) 25:4542–9. doi: 10.1200/JCO.2007.11.2136
81. Madhusudan, S, Foster, M, Muthuramalingam, SR, Braybrooke, JP, Wilner, S, Kaur, K, et al. A phase ii study of etanercept (enbrel), a tumor necrosis factor alpha inhibitor in patients with metastatic breast cancer. Clin Cancer Res. (2004) 10:6528–34. doi: 10.1158/1078-0432.CCR-04-0730
82. Vitale, I, Manic, G, Coussens, LM, Kroemer, G, and Galluzzi, L. Macrophages and metabolism in the tumor microenvironment. Cell Metab. (2019) 30:36–50. doi: 10.1016/j.cmet.2019.06.001
83. Goswami, KK, Ghosh, T, Ghosh, S, Sarkar, M, Bose, A, and Baral, R. Tumor promoting role of anti-tumor macrophages in tumor microenvironment. Cell Immunol. (2017) 316:1–10. doi: 10.1016/j.cellimm.2017.04.005
84. Quatromoni, JG, and Eruslanov, E. Tumor-associated macrophages: function, phenotype, and link to prognosis in human lung cancer. Am J Transl Res. (2012) 4:376–89.
85. Haque, A, Moriyama, M, Kubota, K, Ishiguro, N, Sakamoto, M, Chinju, A, et al. Cd206(+) tumor-associated macrophages promote proliferation and invasion in oral squamous cell carcinoma via egf production. Sci Rep. (2019) 9:14611. doi: 10.1038/s41598-019-51149-1
86. Udeabor, SE, Adisa, AO, Orlowska, A, Sader, RA, and Ghanaati, S. Tumor-associated macrophages, angiogenesis, and tumor cell migration in oral squamous cell carcinoma. Ann Afr Med. (2017) 16:181–5. doi: 10.4103/aam.aam_8_17
87. Zhang, P, Zhang, Y, Wang, L, and Lou, W. Tumor-regulated macrophage type 2 differentiation promotes immunosuppression in laryngeal squamous cell carcinoma. Life Sci. (2021) 267:118798. doi: 10.1016/j.lfs.2020.118798
88. Gao, L, Wang, FQ, Li, HM, Yang, JG, Ren, JG, He, KF, et al. Ccl2/egf positive feedback loop between cancer cells and macrophages promotes cell migration and invasion in head and neck squamous cell carcinoma. Oncotarget. (2016) 7:87037–51. doi: 10.18632/oncotarget.13523
89. Pienta, KJ, Machiels, JP, Schrijvers, D, Alekseev, B, Shkolnik, M, Crabb, SJ, et al. Phase 2 study of carlumab (cnto 888), a human monoclonal antibody against cc-chemokine ligand 2 (ccl2), in metastatic castration-resistant prostate cancer. Investig New Drugs. (2013) 31:760–8. doi: 10.1007/s10637-012-9869-8
90. Nywening, TM, Wang-Gillam, A, Sanford, DE, Belt, BA, Panni, RZ, Cusworth, BM, et al. Targeting tumour-associated macrophages with ccr2 inhibition in combination with folfirinox in patients with borderline resectable and locally advanced pancreatic cancer: a single-Centre, open-label, dose-finding, non-randomised, phase 1b trial. Lancet Oncol. (2016) 17:651–62. doi: 10.1016/S1470-2045(16)00078-4
91. Hume, DA, and Mac Donald, KP. Therapeutic applications of macrophage colony-stimulating factor-1 (csf-1) and antagonists of csf-1 receptor (csf-1r) signaling. Blood. (2012) 119:1810–20. doi: 10.1182/blood-2011-09-379214
92. Gomez-Roca, CA, Italiano, A, Le Tourneau, C, Cassier, PA, Toulmonde, M, D'Angelo, SP, et al. Phase i study of emactuzumab single agent or in combination with paclitaxel in patients with advanced/metastatic solid tumors reveals depletion of immunosuppressive m2-like macrophages. Ann Oncol. (2019) 30:1381–92. doi: 10.1093/annonc/mdz163
93. Papadopoulos, KP, Gluck, L, Martin, LP, Olszanski, AJ, Tolcher, AW, Ngarmchamnanrith, G, et al. First-in-human study of amg 820, a monoclonal anti-colony-stimulating factor 1 receptor antibody, in patients with advanced solid tumors. Clin Cancer Res. (2017) 23:5703–10. doi: 10.1158/1078-0432.CCR-16-3261
94. Tap, WD, Gelderblom, H, Palmerini, E, Desai, J, Bauer, S, Blay, JY, et al. Pexidartinib versus placebo for advanced tenosynovial giant cell tumour (enliven): a randomised phase 3 trial. Lancet. (2019) 394:478–87. doi: 10.1016/S0140-6736(19)30764-0
95. Reid, TR, Abrouk, N, Caroen, S, Oronsky, B, Stirn, M, Larson, C, et al. Rocket: phase ii randomized, active-controlled, multicenter trial to assess the safety and efficacy of rrx-001 + irinotecan vs. single-agent regorafenib in third/fourth line colorectal cancer. Clin Colorectal Cancer. (2023) 22:92–9. doi: 10.1016/j.clcc.2022.11.003
96. Gabrilovich, D. Mechanisms and functional significance of tumour-induced dendritic-cell defects. Nat Rev Immunol. (2004) 4:941–52. doi: 10.1038/nri1498
97. Diaz-Montero, CM, Salem, ML, Nishimura, MI, Garrett-Mayer, E, Cole, DJ, and Montero, AJ. Increased circulating myeloid-derived suppressor cells correlate with clinical cancer stage, metastatic tumor burden, and doxorubicin-cyclophosphamide chemotherapy. Cancer Immunol Immunother. (2009) 58:49–59. doi: 10.1007/s00262-008-0523-4
98. Taki, M, Abiko, K, Baba, T, Hamanishi, J, Yamaguchi, K, Murakami, R, et al. Snail promotes ovarian cancer progression by recruiting myeloid-derived suppressor cells via cxcr2 ligand upregulation. Nat Commun. (2018) 9:1685. doi: 10.1038/s41467-018-03966-7
99. Nagaraj, S, Gupta, K, Pisarev, V, Kinarsky, L, Sherman, S, Kang, L, et al. Altered recognition of antigen is a mechanism of cd8+ t cell tolerance in cancer. Nat Med. (2007) 13:828–35. doi: 10.1038/nm1609
100. Rivera, LB, and Bergers, G. Intertwined regulation of angiogenesis and immunity by myeloid cells. Trends Immunol. (2015) 36:240–9. doi: 10.1016/j.it.2015.02.005
101. Chen, WC, Lai, CH, Chuang, HC, Lin, PY, and Chen, MF. Inflammation-induced myeloid-derived suppressor cells associated with squamous cell carcinoma of the head and neck. Head Neck. (2017) 39:347–55. doi: 10.1002/hed.24595
102. Pang, X, Fan, HY, Tang, YL, Wang, SS, Cao, MX, Wang, HF, et al. Myeloid derived suppressor cells contribute to the malignant progression of oral squamous cell carcinoma. PLoS One. (2020) 15:e229089. doi: 10.1371/journal.pone.0229089
103. Angell, TE, Lechner, MG, Smith, AM, Martin, SE, Groshen, SG, Maceri, DR, et al. Circulating myeloid-derived suppressor cells predict differentiated thyroid cancer diagnosis and extent. Thyroid. (2016) 26:381–9. doi: 10.1089/thy.2015.0289
104. Fugle, CW, Zhang, Y, Hong, F, Sun, S, Westwater, C, Rachidi, S, et al. Cd24 blunts oral squamous cancer development and dampens the functional expansion of myeloid-derived suppressor cells. Onco Targets Ther. (2016) 5:e1226719. doi: 10.1080/2162402X.2016.1226719
105. Ryan, N, Anderson, K, Volpedo, G, Hamza, O, Varikuti, S, Satoskar, AR, et al. Stat1 inhibits t-cell exhaustion and myeloid derived suppressor cell accumulation to promote antitumor immune responses in head and neck squamous cell carcinoma. Int J Cancer. (2020) 146:1717–29. doi: 10.1002/ijc.32781
106. Weed, DT, Vella, JL, Reis, IM, De la Fuente, AC, Gomez, C, Sargi, Z, et al. Tadalafil reduces myeloid-derived suppressor cells and regulatory t cells and promotes tumor immunity in patients with head and neck squamous cell carcinoma. Clin Cancer Res. (2015) 21:39–48. doi: 10.1158/1078-0432.CCR-14-1711
107. Tobin, RP, Jordan, KR, Robinson, WA, Davis, D, Borges, VF, Gonzalez, R, et al. Targeting myeloid-derived suppressor cells using all-trans retinoic acid in melanoma patients treated with ipilimumab. Int Immunopharmacol. (2018) 63:282–91. doi: 10.1016/j.intimp.2018.08.007
108. Nagaraj, S, Youn, JI, Weber, H, Iclozan, C, Lu, L, Cotter, MJ, et al. Anti-inflammatory triterpenoid blocks immune suppressive function of mdscs and improves immune response in cancer. Clin Cancer Res. (2010) 16:1812–23. doi: 10.1158/1078-0432.CCR-09-3272
109. Wang, YY, Yang, YX, Zhe, H, He, ZX, and Zhou, SF. Bardoxolone methyl (cddo-me) as a therapeutic agent: an update on its pharmacokinetic and pharmacodynamic properties. Drug Des Devel Ther. (2014) 8:2075–88. doi: 10.2147/DDDT.S68872
110. Serafini, P, Meckel, K, Kelso, M, Noonan, K, Califano, J, Koch, W, et al. Phosphodiesterase-5 inhibition augments endogenous antitumor immunity by reducing myeloid-derived suppressor cell function. J Exp Med. (2006) 203:2691–702. doi: 10.1084/jem.20061104
111. Tai, LH, Alkayyal, AA, Leslie, AL, Sahi, S, Bennett, S, Tanese, DSC, et al. Phosphodiesterase-5 inhibition reduces postoperative metastatic disease by targeting surgery-induced myeloid derived suppressor cell-dependent inhibition of natural killer cell cytotoxicity. Onco Targets Ther. (2018) 7:e1431082. doi: 10.1080/2162402X.2018.1431082
112. Noonan, KA, Ghosh, N, Rudraraju, L, Bui, M, and Borrello, I. Targeting immune suppression with pde5 inhibition in end-stage multiple myeloma. Cancer Immunol Res. (2014) 2:725–31. doi: 10.1158/2326-6066.CIR-13-0213
113. Folkman, J. Angiogenesis in cancer, vascular, rheumatoid and other disease. Nat Med. (1995) 1:27–31. doi: 10.1038/nm0195-27
114. Hanahan, D, and Folkman, J. Patterns and emerging mechanisms of the angiogenic switch during tumorigenesis. Cells. (1996) 86:353–64. doi: 10.1016/S0092-8674(00)80108-7
115. Akino, T, Hida, K, Hida, Y, Tsuchiya, K, Freedman, D, Muraki, C, et al. Cytogenetic abnormalities of tumor-associated endothelial cells in human malignant tumors. Am J Pathol. (2009) 175:2657–67. doi: 10.2353/ajpath.2009.090202
116. Naito, H, Wakabayashi, T, Kidoya, H, Muramatsu, F, Takara, K, Eino, D, et al. Endothelial side population cells contribute to tumor angiogenesis and antiangiogenic drug resistance. Cancer Res. (2016) 76:3200–10. doi: 10.1158/0008-5472.CAN-15-2998
117. Ferrara, N, Gerber, HP, and LeCouter, J. The biology of vegf and its receptors. Nat Med. (2003) 9:669–76. doi: 10.1038/nm0603-669
118. Klein, D. The tumor vascular endothelium as decision maker in cancer therapy. Front Oncol. (2018) 8:367. doi: 10.3389/fonc.2018.00367
119. Viallard, C, and Larrivee, B. Tumor angiogenesis and vascular normalization: alternative therapeutic targets. Angiogenesis. (2017) 20:409–26. doi: 10.1007/s10456-017-9562-9
120. Sun, Q, Zhang, T, Xiao, Q, Mei, B, and Zhang, X. Procyanidin b2 inhibits angiogenesis and cell growth in oral squamous cell carcinoma cells through the vascular endothelial growth factor (vegf)/vegf receptor 2 (vegfr2) pathway. Bioengineered. (2022) 13:6500–8. doi: 10.1080/21655979.2022.2033013
121. Wu, A, Luo, N, Xu, Y, Du, N, Li, L, and Liu, Q. Exosomal lbh inhibits epithelial-mesenchymal transition and angiogenesis in nasopharyngeal carcinoma via downregulating vegfa signaling. Int J Biol Sci. (2022) 18:242–60. doi: 10.7150/ijbs.66506
122. Chen, L, Lin, G, Chen, K, Liang, R, Wan, F, Zhang, C, et al. Vegf promotes migration and invasion by regulating emt and mmps in nasopharyngeal carcinoma. J Cancer. (2020) 11:7291–301. doi: 10.7150/jca.46429
123. Daniele, G, Raspagliesi, F, Scambia, G, Pisano, C, Colombo, N, Frezzini, S, et al. Bevacizumab, carboplatin, and paclitaxel in the first line treatment of advanced ovarian cancer patients: the phase iv Mito-16a/mango-ov2a study. Int J Gynecol Cancer. (2021) 31:875–82. doi: 10.1136/ijgc-2021-002434
124. Lin, Y, Qin, S, Li, Z, Yang, H, Fu, W, Li, S, et al. Apatinib vs placebo in patients with locally advanced or metastatic, radioactive iodine-refractory differentiated thyroid cancer: the reality randomized clinical trial. JAMA Oncol. (2022) 8:242–50. doi: 10.1001/jamaoncol.2021.6268
125. Cascone, T, Sacks, RL, Subbiah, IM, Drobnitzky, N, Piha-Paul, SA, Hong, DS, et al. Safety and activity of vandetanib in combination with everolimus in patients with advanced solid tumors: a phase i study. Esmo Open. (2021) 6:100079. doi: 10.1016/j.esmoop.2021.100079
126. Kubota, K, Yoshioka, H, Oshita, F, Hida, T, Yoh, K, Hayashi, H, et al. Phase iii, randomized, placebo-controlled, double-blind trial of motesanib (amg-706) in combination with paclitaxel and carboplatin in east asian patients with advanced nonsquamous non-small-cell lung cancer. J Clin Oncol. (2017) 35:3662–70. doi: 10.1200/JCO.2017.72.7297
127. de la Fouchardiere, C, Godbert, Y, Dalban, C, Illouz, F, Wassermann, J, Do, CC, et al. Intermittent versus continuous administration of pazopanib in progressive radioiodine refractory thyroid carcinoma: final results of the randomised, multicenter, open-label phase ii trial pazothyr. Eur J Cancer. (2021) 157:153–64. doi: 10.1016/j.ejca.2021.07.029
128. Atkins, MB, Plimack, ER, Puzanov, I, Fishman, MN, McDermott, DF, Cho, DC, et al. Axitinib plus pembrolizumab in patients with advanced renal-cell carcinoma: long-term efficacy and safety from a phase ib trial. Eur J Cancer. (2021) 145:1–10. doi: 10.1016/j.ejca.2020.12.009
129. Xia, L, Zhou, Q, Gao, Y, Hu, W, Lou, G, Sun, H, et al. A multicenter phase 2 trial of camrelizumab plus famitinib for women with recurrent or metastatic cervical squamous cell carcinoma. Nat Commun. (2022) 13:7581. doi: 10.1038/s41467-022-35133-4
130. Higashiyama, T, Sugino, K, Hara, H, Ito, KI, Nakashima, N, Onoda, N, et al. Phase ii study of the efficacy and safety of lenvatinib for anaplastic thyroid cancer (hope). Eur J Cancer. (2022) 173:210–8. doi: 10.1016/j.ejca.2022.06.044
131. Brose, MS, Robinson, BG, Sherman, SI, Jarzab, B, Lin, CC, Vaisman, F, et al. Cabozantinib for previously treated radioiodine-refractory differentiated thyroid cancer: updated results from the phase 3 cosmic-311 trial. Cancer. (2022) 128:4203–12. doi: 10.1002/cncr.34493
132. Kim, RD, Sanoff, HK, Poklepovic, AS, Soares, H, Kim, J, Lyu, J, et al. A multi-institutional phase 2 trial of regorafenib in refractory advanced biliary tract cancer. Cancer. (2020) 126:3464–70. doi: 10.1002/cncr.32964
133. Boumahdi, S, Driessens, G, Lapouge, G, Rorive, S, Nassar, D, Le Mercier, M, et al. Sox2 controls tumour initiation and cancer stem-cell functions in squamous-cell carcinoma. Nature. (2014) 511:246–50. doi: 10.1038/nature13305
134. Valent, P, Bonnet, D, De Maria, R, Lapidot, T, Copland, M, Melo, JV, et al. Cancer stem cell definitions and terminology: the devil is in the details. Nat Rev Cancer. (2012) 12:767–75. doi: 10.1038/nrc3368
135. Qian, X, Nie, X, Yao, W, Klinghammer, K, Sudhoff, H, Kaufmann, AM, et al. Reactive oxygen species in cancer stem cells of head and neck squamous cancer. Semin Cancer Biol. (2018) 53:248–57. doi: 10.1016/j.semcancer.2018.06.001
136. Keysar, SB, Le, PN, Miller, B, Jackson, BC, Eagles, JR, Nieto, C, et al. Regulation of head and neck squamous cancer stem cells by pi3k and sox2. J Natl Cancer Inst. (2017) 109:djw189. doi: 10.1093/jnci/djw189
137. Zheng, H, Chen, X, Li, Q, Liu, Y, and Cai, J. Effects of chemotherapy and immunotherapy on microbial diversity in tme and engineered bacterial-mediated tumor therapy. Front Immunol. (2023) 14:1084926. doi: 10.3389/fimmu.2023.1084926
Keywords: HNSCC, microenvironment, targeted therapy, tumor progression, inflammation
Citation: Guo Z, Li K, Liu P, Zhang X, Lv J, Zeng X and Zhang P (2023) Targeted therapy for head and neck squamous cell carcinoma microenvironment. Front. Med. 10:1257898. doi: 10.3389/fmed.2023.1257898
Edited by:
Zhaoqi Yang, Jiangnan University, ChinaReviewed by:
Yan Lu, Nanjing General Hospital of Nanjing Military Command, ChinaLianhe Chu, Karolinska Institutet (KI), Sweden
Xin Hua, Chinese Academy of Agricultural Sciences, China
Copyright © 2023 Guo, Li, Liu, Zhang, Lv, Zeng and Zhang. This is an open-access article distributed under the terms of the Creative Commons Attribution License (CC BY). The use, distribution or reproduction in other forums is permitted, provided the original author(s) and the copyright owner(s) are credited and that the original publication in this journal is cited, in accordance with accepted academic practice. No use, distribution or reproduction is permitted which does not comply with these terms.
*Correspondence: Jie Lv, bHZqaWVAeWx1LmVkdS5jbg==; Xianhai Zeng, enhoa2x3eEAxNjMuY29t; Peng Zhang, emhhbmdwZW5nMjYwMEAxNjMuY29t
†These authors have contributed equally to this work