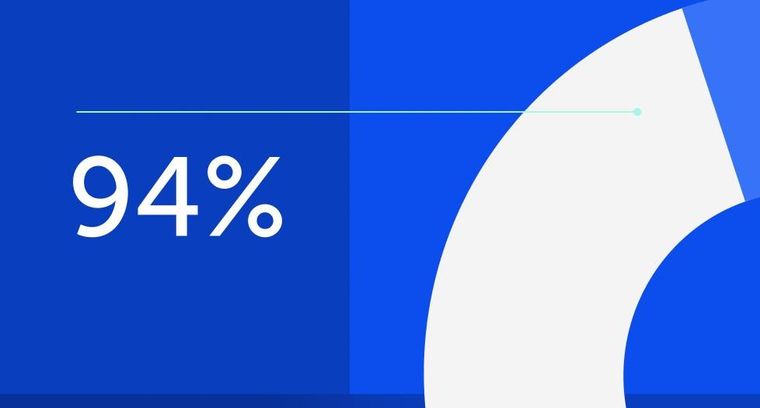
94% of researchers rate our articles as excellent or good
Learn more about the work of our research integrity team to safeguard the quality of each article we publish.
Find out more
PERSPECTIVE article
Front. Med., 19 July 2023
Sec. Translational Medicine
Volume 10 - 2023 | https://doi.org/10.3389/fmed.2023.1226531
Aerospace research has a long history of developing technologies with industry-changing applications and recent history is no exception. The expansion of commercial spaceflight and the upcoming exploration-class missions to the Moon and Mars are expected to accelerate this process even more. The resulting portable, wearable, contactless, and regenerable medical technologies are not only the future of healthcare in deep space but also the future of healthcare here on Earth. These multi-dimensional and integrative technologies are non-invasive, easily-deployable, low-footprint devices that have the ability to facilitate rapid detection, diagnosis, monitoring, and treatment of a variety of conditions, and to provide decision-making and performance support. Therefore, they are primed for applications in low-resource and remote environments, facilitating the extension of quality care delivery to all patients in all communities and empowering non-specialists to intervene early and safely in order to optimize patient-centered outcomes. Additionally, these technologies have the potential to advance care delivery in tertiary care centers by improving transitions of care, providing holistic patient data, and supporting clinician wellness and performance. The requirements of space exploration have created a number of paradigm-altering medical technologies that are primed to revitalize and elevate our standard of care here on Earth.
Technological development that supports space exploration often translates to novel and cutting-edge tools for Earth-based use. The National Aeronautics and Space Administration (NASA) is interested in mitigating identified Risks (1) associated with living in space, and, while some are space-specific, many have readily-identifiable terrestrial counterparts. The requirements of space exploration have created a number of paradigm-altering medical technologies that can elevate our standard of care here on Earth across all populations and care delivery models.
In this Perspective, we delineate the complexities of medical management during spaceflight that motivate such extensive innovation in medical technologies. Then we discuss the overlapping needs between space exploration and terrestrial environments that allow for prime translation of these technologies across the two seemingly disparate settings, including historical examples of successful technological cross-pollination. Subsequently, we explore broad categories of innovative aerospace-derived medical technologies, with representative examples. Finally, we propose high-priority terrestrial clinical applications for these technologies across in- and out-of-hospital settings, including in under-resourced areas; we also address critical touchpoints for enhancing clinician wellness and performance with these technologies. Overall, we provide a roadmap to spur clinician-leaders in spearheading collaborations with aerospace medical technology groups so that they may leverage their respective expertise to enhance patient, clinician, and astronaut health.
Long-duration spaceflight results in significant changes to 1 g-dependent human physiology. While these changes are covered in detail elsewhere (2, 3), they encompass all organ systems and have variable trajectories, plateaus, degrees of reversibility, and long-term health effects. Their complex, incompletely-characterized nature has catalyzed extensive medical technology development for both research and medical care provision during spaceflight. For context, we present a very brief, generalized overview here (Supplementary Figure S1).
Spaceflight-associated neuro-ocular syndrome (SANS) is an important example: it is a syndrome never seen on Earth that affects astronauts’ vision. It is thought to be related to cephalad fluid shifts occurring in the absence of gravity, which produces alterations in brain and eye structure and function (4). Airway edema, headache, and sinus fullness occur secondary to cephalad fluid shifts too. Cognition and mental health, while not overtly affected by microgravity per se, are highly dependent on stress, sleep, and social–emotional factors, all of which can be disrupted by spaceflight. Additionally, radiation exposure is known to accelerate cataract formation and increases malignancy risk (5). Space-related motion sickness arises due to mismatched sensory inputs disrupting normal vestibular function (6).
From the cardiovascular perspective, cardiac output and stroke volume initially increase upon exposure to microgravity, but subsequently decline, along with cardiac muscle mass (7, 8). Peripheral vasodilation predominates and is exacerbated by changes in autonomic receptor sensitivities, endothelial changes, and vascular remodeling (9, 10). Grossly, pulmonary mechanics improve (11). Cellular and humoral immunity becomes compromised (12). This is compounded by radiation-induced genetic changes that increase microbial virulence (13). Finally, even despite vigorous exercise, bone density and muscle mass decline. Increased urinary calcium from bone resorption coupled with decreased diuresis increases the incidence of renal stones (14). Ultimately, there is still much to be learned about human physiology in this extreme environment.
The challenging nature of spaceflight makes it an ideal catalyst for innovation in care delivery for remote terrestrial environments (Table 1). These sites frequently coincide with care delivery for patients requiring active monitoring and rapid diagnosis and therapy for potentially life-threatening conditions, like traumatic injuries and toxin exposures. Furthermore, clinicians operating in these conditions are often fatigued and under duress, as might be expected in deep space. However, even in well-resourced environments, monitoring, diagnosis, and therapeutics for serious conditions could be improved by facile, assistive technologies, especially in emergency, perioperative, and critical care areas.
Table 1. Health risks of space exploration, their terrestrial counterparts, and technological countermeasures.
Many medical devices that are standard of care trace their origins to technologies pioneered or refined by aerospace experts. For example, the very concept of telemetric monitoring was prompted by the desire to send astronaut health information from space to ground control during Project Mercury (72) and has since resulted in ubiquitous terrestrial use, not the least of which is routine intensive care unit (ICU) monitoring. Other recent examples include improved LASIK® eye surgery precision due to innovative optics developed for the Webb telescope (73); materials engineering advances for Martian sample collection that led to biocompatible surgical suture materials (74); and an endoscopic robotic surgery arm inspired by robotic repair arms on the International Space Station (ISS) (75). Clearly, humankind’s extraterrestrial aspirations have stimulated numerous advances across many sectors; here, we focus on medical technologies.
Early and accurate diagnosis and treatment of health conditions arising during spaceflight can potentially halt or even reverse their negative effects, without requiring a costly, mission-compromising return to Earth or delayed post-flight medical care. As such, easily-deployable and non-invasive diagnostics and therapeutics are prioritized. In this section, we survey recent space-related diagnostic (Supplementary Table S1) and therapeutic (Supplementary Table S2) technologies.
Imaging and blood analysis comprise two fundamental categories of diagnostic testing. Ultrasound is the primary imaging modality on the ISS due to its versatility, portability, and limited hazards. As a result, its already-numerous applications have been exponentially expanded and include the ability to characterize renal stones (26), vascular flow alterations (27), and bone architecture disorders (28, 29). While the brain remains relatively inaccessible to ultrasound, retinal imaging has been proposed as an alternative to the traditional, and currently space-impractical, CT and MRI modalities for diagnosing stroke (30). The ever-growing library of diagnostic images has concurrently resulted in image-based clinical decision support (CDS) systems too (64). The use of blood component analysis in diagnosis is pervasive, but standard laboratory analyte platforms are unworkable in microgravity, for many reasons including changes in fluid behavior. As a result, techniques that preserve accuracy and facilitate component miniaturization have prevailed, including for CBC (31), WBC differential (32), and multiple electrolyte analysis (33–37).
Unlike portable diagnostics used for intermittent testing, wearable and contactless sensors allow for continuous monitoring. These sensors can be light-, chemical-, current-, or motion-based, implying that the spaces to which they could foreseeably be adapted are numerous. For example, near-infrared spectroscopy (NIRS) relies on analysis of absorption and scatter of specific wavelengths to infer various parameters, such as hemoglobin oxygenation and deoxygenation, blood flow, and presence of water. Transcranial NIRS via headband-like devices can record cerebral hemodynamics during daily activity (15), can identify intracranial edema (16), and can be used in self-deployable polysomnography systems (15, 16). Transdermal NIRS can measure metabolic rate, stroke volume, peripheral hematocrit, and oxygen saturation (38, 39). Other skin-based sensors can detect nutrients, metabolites, and hormones in perspiration (40) or protease activity changes related to muscle atrophy, HIV, and even certain cancers (41). The versatility of these sensors lend them to easy incorporation into mobile monitoring garments, for astronauts and patients alike. Comfortable, washable, wearable, waterproof device-integrated clothing is already available (42–44).
The targets of contactless sensors are largely cognitive and can detect aspects of team cohesion, mental health, sleep deprivation, stress, fatigue, and performance. These devices use a variety of techniques, allowing them to blend into home or work environments, including optical computer facial recognition algorithms (17), radio wave sensors (18), and acoustical analysis (19, 20). Non-invasive biomechanical sensors can also record physical exertion (45), in order to mitigate injury risk during task performance. Overall, environmental enhancements that unobtrusively extract physiologic information from subjects provide accessible, compliance-independent data that can inform decision-making on many scales.
While the ability to improve early diagnosis of physical and cognitive decrements during long-duration spaceflight is useful, the inability to return to Earth in a reasonable treatment time frame requires the development of portable, easily-deployable therapeutics. In order to minimize payload and maximize multi-use, many of the devices used in diagnosis have also been studied as therapies. Nevertheless, some therapies are consumable and will need to be resynthesized during long-duration missions – a complex problem also addressable with innovative technologies.
Given its portability and extensive diagnostic capabilities, ultrasound technology has also been studied for therapeutic applications, including renal stone propulsion (46), targeted hemostasis (47), and bone remineralization (48). Additionally, wearable ultrasound can treat back pain (49) and potentially provide functional neuromodulation (23) for neurologic and psychiatric disorders. Improvement of sleep and performance have also been trialed using sound stimulation (50–52), light stimulation (53), transdermal vagus nerve stimulation (24), and transcranial NIRS-based photobiomodulation (25).
While these technology-based therapies may supplant standard treatment for some conditions, pharmaceuticals remain critical to the therapeutic arsenal. Radiation sickness and radiation-induced cellular damage is of utmost concern for long-duration extraplanetary missions, resulting in accelerated development of pharmacologic treatment options, including synthetic genistein (65–67) and melanin-based radioprotectants (68–71). However, in addition to human exposure concerns, cosmic radiation also risks degrading pharmaceutical stores (76) and, with the prospect of very limited and very expensive resupply missions, the need to regenerate pharmaceutical compounds is paramount. In-situ resource utilization (ISRU) using modified terrestrial biological catalysts along with compounds available on extraplanetary missions to synthesize pharmaceuticals is likely feasible (54–56). Other consumable but life-supporting therapies include intravenous resuscitative fluids, such as crystalloid solutions and blood products. Generation of sterile normal saline from potable water (59) and reconstitution of desiccated, shelf-stable hemoglobin-based oxygen carriers (HBOC) (60) are reasonable solutions for resuscitation in under-resourced conditions.
Finally, integration of CDS with these diagnostic and therapeutic technologies, to streamline and optimize care delivery, is also being tackled by aerospace groups. Integration of structured documentation with mobile critical care modules can ease user burden and improve care (61). Just-in-time training (JITT) with (57) and without telementoring (58) can also improve diagnosis by non-medical personnel. Mixed reality-guided checklist-based software for medical training and real-time management (62, 63) and semi-autonomous and autonomous intelligent agents can facilitate CDS, care coordination, and care delivery (21, 22). Overall, safe advanced care delivery in the remoteness of deep space is approaching a possible reality.
Given the breadth of biological targets and the versatility of the medical technologies developed for space exploration, finding direct terrestrial applications is not difficult. Integration of these technologies across the patient care spectrum is immediately feasible and is critical to launch medicine into an era of unprecedented access to patient-centered health optimization.
Management of health, wellness, and chronic illness in the outpatient setting is multi-faceted and is (too) frequently escalated beyond primary care services. Aerospace medical technologies have the potential to keep patients healthier at home and decrease preventable hospitalizations. Integration of these technologies in the home and clinic (Figure 1A) can hasten the decentralization of medical care without sacrificing safety or quality, empowering patients to control their health outcomes and their quality of life.
Figure 1. Integration of space-adapted medical technologies in a healthcare workflow model. Two patient care scenarios are presented – an outpatient with chronic illness and an acute surgical emergency. While they ultimately require different management, the integration of space-adapted medical technologies not only improves the efficacy and efficiency of patient care, thereby improving outcomes, quality of life, and patient satisfaction; they can also decrease facility-related length of stay, and thus decrease costs and complication rates. (A) Chronic Medical Management. Patient-centered health and wellness can be supported with various contactless and wearable devices that can intelligently monitor a variety of physiologic parameters and provide feedback to the patient and their local provider, empowering them to regulate their health outcomes and intervene early and effectively at home and in their local clinic. Local providers armed with novel technologies to diagnose and treat conditions typically requiring escalation of care can be supported virtually to further facilitate de-centralized, high-quality, and accessible healthcare and wellness optimization. (B) Surgical Emergency. An acute event occurs. The standard cycle of care is illustrated and captioned, including a number of gaps and delays that contribute to additional patient morbidity. Widespread integration of space-adapted medical technologies across the care cycle could dramatically improve patient outcomes, quality of life, and costs in a variety of ways including: (1) providing baseline health information and last known well, (2) contributing to timely escalation of care, (3) decreasing time to diagnosis and targeted treatment, (4) improving care coordination by decreasing and streamlining transfers of care, (5) providing multi-dimensional continuous monitoring supported by CDS to rapidly and effectively correct deviations, (6) optimizing clinician performance through non-invasive behavioral and cognitive support technologies, (7) leveraging non-pharmacologic interventions to more effectively prevent and treat in-hospital delirium, (8) facilitating early mobility and safe care de-escalation with unobtrusive patient-centered monitoring including of medical conditions and physical loading, and (9) moving patient care out of facilities and into the home and local clinics so patients can get back to their lives sooner, safely, and in better health.
“Smart” devices and homes are prevalent and ever-growing, but aerospace-derived technologies can fine-tune this growth by expanding biological targets of monitoring, liberating users from traditional workflow constraints, and facilitating care coordination. These intrinsic advantages of aerospace technologies stem from their highly stringent requirements favoring multi-functionality, unobtrusiveness, and integration. Examples of unobtrusive multi-functionality include concurrent, non-invasive monitoring of vital signs, sleep parameters, gait analysis and certain behaviors, like medication compliance and fall detection, to provide valuable feedback for behavioral modification and wellness optimization. Additionally, non-invasive, continuous monitoring of a various patient-specific parameters – such as biomarkers of anxiety and depression, sleep disorders, renal disease, endocrine disorders, and hematologic and oncologic disorders – would limit the need for repeated visits to clinics for invasive, expensive bloodwork. This can also provide unbiased, compliance-independent, and high-density information about disease states, including the efficacy of prescribed interventions and relevant outcomes of clinical trials. Portable and resilient point-of-care bloodwork devices can facilitate testing in clinics or at home, supporting medication titration and side-effect management without needing to travel to the office. Finally, in addition to providing readily available, structured medical and mental health support at home, semi-autonomous conversational intelligent agents can also escalate and coordinate care resources as needed, resulting in timely intervention.
Aerospace technologies are also primed for expanding clinical access by empowering non-expert providers to safely, confidently care for numerous conditions with assistance from AI-CDS, JITT, and specialist telementoring, in a model similar to non-physician astronauts caring for their fellow spacefarers. Expansion of remotely-guided and AI-assisted ultrasound-based diagnostics and therapeutics is a game-changing possibility for care delivery in under-resourced areas (77). These technologies can decrease hospitalizations by allowing earlier detection of disease and earlier intervention, even (and especially) by non-specialists. Treatment of certain ailments that previously required care escalation could initially be attempted in the home or office, such as ultrasound-based renal stone management or hemorrhage control. Similarly, infusion of shelf-stable reconstituted HBOCs could obviate the need to travel long distances for patients requiring transfusion. In cases of extreme remoteness or other travel impediment, such as in pandemics or wars, ISRU and synthetic pharmacy could restore stocks of basic medical supplies.
Furthermore, these technologies not only have the potential to improve baseline health, they can also improve specific chronic conditions ahead of elective surgery, decreasing perioperative morbidity. At-home data collection could also inform preoperative and risk evaluation, providing patients and clinicians with individualized risk–benefit assessments. “Pre-habilitation” programs for at-risk patients could include biometric and metabolic/hormone monitoring to support targeted systemic disease optimization and NIRS-based photobiomodulation to shore up cognitive and psychological reserve ahead of surgery.
Last, but not least, device detection of deviations from known baseline and semi-autonomous contacting of a clinician or emergency services can limit delays in care, especially for time-sensitive conditions like stroke or myocardial infarction. Emergency services equipped with portable or wearable blood component or perspiration analysis devices, advanced diagnostic and therapeutic ultrasound, retinal imaging, and AI-CDS could more rapidly diagnose specific critical conditions, initiate earlier targeted treatment en route to a hospital, and redirect transport to a specialty hospital based on tentative technology-assisted diagnoses, limiting the need for late transfers of care. Overall, aerospace medical technology can rapidly bring 21st century medicine to all patients.
Translation of space-adapted medical technologies is primed for high-acuity hospital areas (Figure 1B). Faster, more accurate diagnostics in the Emergency Department (ED), especially for critically ill or unstable patients would undoubtedly aid decision-making and treatment. Integration of the above portable blood testing platforms and ultrasound-based diagnostics to the standard arsenal should be prioritized. Furthermore, use of ultrasound-based therapeutics in this setting, such as for hemostasis or kidney stone propulsion, should be considered.
ICU care could be augmented by space-adapted technologies. For example, patients could be non-invasively and continuously monitored for muscle degradation (from being bed-bound) or improvement (from physical therapy), drug metabolites (especially of sedatives or narcotics), and cancer progression (given treatment is paused for ICU admission), without multiple painful, costly blood draws. Stroke could be identified using retinal imaging without needing urgent and unwieldy transport to the CT scanner. Nutritional supplementation decisions could be supported with NIRS-based measurements of metabolic rate. Stroke volume and hematocrit could also be monitored by NIRS. Circadian rhythm disorders could be averted or treated with non-pharmaceutical sleep entrainment, reducing delirium and sedative use. Seamless multi-modal physiologic monitoring, including brain monitoring, could assist with mental health and delirium assessment, sleep monitoring, drug dosing, or detection of disease state or acute changes; their portable nature can mitigate the cumbersomeness of patient transport to procedures and imaging, and can improve periprocedural care by limiting the need to connect and disconnect monitoring multiple times.
Unobtrusive physiologic monitoring can also facilitate patient care transfers and patient mobility within the hospital. Sensor-equipped patient gowns or wearables could allow continuous, yet comfortable, monitoring; coupling these continuous inputs with CDS could track patient clinical status longitudinally and determine transfers to different levels of care. CDS can also integrate the multi-modal data to better direct which procedures and interventions are offered and reduce unrecognized bias in care delivery (78). Wearables deployed during acute changes in status would facilitate monitoring of the patient during resuscitation, triage, and transport. Finally, sensor-equipped gowns could improve patient mobility during hospitalization while maintaining a safety net of monitoring, improving physical and mental health rehabilitation and patient satisfaction.
Ultimately, the timely and safe de-escalation of care acuity can be facilitated with these technologies, including transitions to non-hospital facilities. For example, non-invasive sensors of metabolic rate, skeletal muscle mass, and cardiac parameters could be of great utility in monitoring physical therapy progress as well as physiologic parameters that would limit the extent of therapy in at-risk patients. Wearable unobtrusive monitors could provide a virtual safety net supported by telementoring and consultancy medicine, keeping patients healthier and out of the hospitals as much as possible.
Finally, space-adapted medical technologies have the potential to improve clinician performance and wellness. Application of biomedical sensors to the patient care environment have the potential to record physical loading and torque experienced by staff such as nurses, physical and occupational therapists, or patient care assistants during routine physical tasks required for patient care; this can help inform and improve workplace safety measures to prevent injury as well as improve patient safety. Other “smart” workplace design possibilities would include incorporation of contactless monitoring – optical, acoustical, radio wave detection – of cognitive or mental health decrements in high-stakes, stressful environments, such as the ED, OR, or ICU. Real-time unbiased feedback on patient safety and team dynamics can speed the quality improvement cycle.
Much like astronauts, clinicians can experience cognitive decrements resulting from heightened stress and decreases in sleep quantity or quality. In particular, clinicians working in complex environments like the ED, the OR, or the ICU, where heightened vigilance is required and emergency action is not uncommon, are at risk for suboptimal attention and performance despite maximal effort, especially during overnight or 24-h shifts. The same could be said for care delivery personnel operating in remote or under-resourced locations. Enhancing cognition and performance during these shifts, and enhancing recovery after these shifts, may improve clinician well-being, reduce burnout, and improve outcomes. Routine availability or application of non-pharmaceutical sleep-improving or performance-boosting technologies for medical practitioners either in the workplace (e.g., while on call) or at home is worth exploring.
Disruptive medical technologies, like those required to support extraplanetary human existence, have an extensive array of potential applications across all terrestrial care delivery settings, for both patients and practitioners. Rapid and efficient clinical validation of these technologies and their integration into standard clinical workflow must be a priority and requires the involvement of clinicians to spearhead the charge. The future of democratized, safe, quality healthcare delivery is already here.
The original contributions presented in the study are included in the article/Supplementary material, further inquiries can be directed to the corresponding author.
JS wrote the first draft of the manuscript. All authors contributed to the scope and content of the manuscript, read, edited, and approved the final submitted version of the manuscript.
SP is employed by company Fluidity Technologies, Inc. SP is employed by Operator Solutions and Voyager Space Holdings as a board member.
The remaining authors declare that the research was conducted in the absence of any commercial or financial relationships that could be construed as a potential conflict of interest.
All claims expressed in this article are solely those of the authors and do not necessarily represent those of their affiliated organizations, or those of the publisher, the editors and the reviewers. Any product that may be evaluated in this article, or claim that may be made by its manufacturer, is not guaranteed or endorsed by the publisher.
The Supplementary material for this article can be found online at: https://www.frontiersin.org/articles/10.3389/fmed.2023.1226531/full#supplementary-material
AI, Artificial Intelligence; AMRA, Autonomous Medical Response Agent; CBC, Complete Blood Count; CDS, Clinical Decision Support; CMO, Crew Medical Officer; CT, Computed Tomography; ECG, Electrocardiography; ED, Emergency Department; EEG, Electroencephalography; HBOC, Hemoglobin-Based Oxygen Carriers; ICU, Intensive Care Unit; ISS, International Space Station; ISRU, In-Situ Resource Utilization; IV, Intravenous; JITT, Just-In-Time Training; MRI, Magnetic Resonance Imaging; NASA, National Aeronautics and Space Administration; NIRS, Near-Infrared Spectroscopy; PACU, Post-Anesthesia Care Unit; SANS, Spaceflight-Associated Neuro-ocular Syndrome; WBC, White Blood Cell.
1. “A risk reduction strategy for human space exploration”. NASA Human Research Roadmap, Available at: https://humanresearchroadmap.nasa.gov/.
2. Nicogossian, AE, Williams, RS, Huntoon, CL, Doarn, CR, Polk, JD, and Schneider, VS. Space physiology and medicine: from evidence to practice. 4th ed. New York: Springer (2016).
3. Stepanek, J, Blue, RS, and Parazynski, SE. Space medicine in the era of civilian spaceflight. N Engl J Med. (2019) 380:1053–60. doi: 10.1056/NEJMra1609012
4. Ong, J, Tarver, W, Brunstetter, T, Mader, TH, Gibson, CR, Mason, SS, et al. Spaceflight associated neuro-ocular syndrome: proposed pathogenesis, terrestrial analogues, and emerging countermeasures. Br J Ophthalmol. (2023) 107:895–900. doi: 10.1136/bjo-2022-322892
5. Jones, JA, and Karouia, F. Radiation disorders In: MR Barratt, ES Baker, and SL Pool, editors. Principles of clinical medicine for space flight. New York: Springer (2019). 475–519.
6. Carriot, J, Mackrous, I, and Cullen, KE. Challenges to the vestibular system in space: how the brain responds and adapts to microgravity. Front Neural Circ. (2021) 15:760313. doi: 10.3389/fncir.2021.760313
7. Baker, ES, Barratt, MR, Sams, CF, and Wear, ML. Human response to spaceflight In: MR Barratt, ES Baker, and SL Pool, editors. Principles of clinical medicine for space flight. New York: Springer (2019). 367–411. doi: 10.1007/978-1-4939-9889-0_12
8. Sy, MR, Keefe, JA, Sutton, JP, and Wehrens, XHT. Cardiac function, structural, and electrical remodeling by microgravity exposure. Am J Physiol Heart Circ Physiol. (2023) 324:H1–H13. doi: 10.1152/ajpheart.00611.2022
9. Norsk, P, Asmar, A, Damgaard, M, and Christensen, NJ. Fluid shifts, vasodilatation and ambulatory blood pressure reduction during long duration spaceflight. J Physiol. (2014) 593:573–84. doi: 10.1113/jphysiol.2014.284869
10. Vernice, NA, Meydan, C, Afshinnekoo, E, and Mason, CE. Long-term spaceflight and the cardiovascular system. Precis Clin Med. (2020) 3:284–91. doi: 10.1093/pcmedi/pbaa022
12. Sams, CF, Crucian, BE, and Pierson, DL. Immunology and microbiology In: MR Barratt, ES Baker, and SL Pool, editors. Principles of clinical medicine for space flight. New York: Springer (2019). 659–71.
13. Cervantes, JL, and Hong, BY. Dysbiosis and immune dysregulation in outer space. Int Rev Immunol. (2016) 35:67–82. doi: 10.3109/08830185.2015.1027821
14. Smith, SM, Heer, M, Shackelford, LC, Sibonga, JD, Spatz, J, Pietrzyk, RA, et al. Bone metabolism and renal stone risk during international Space Station missions. Bone. (2015) 81:712–20. doi: 10.1016/j.bone.2015.10.002
15. Zhang, Q, Ivkovic, V, Hu, G, and Strangman, GE. Twenty-four-hour ambulatory recording of cerebral hemodynamics, systemic hemodynamics, electrocardiography, and actigraphy during people's daily activities. J Biomed Opt. (2014) 19:47003. doi: 10.1117/1.JBO.19.4.047003
16. Strangman, GE, Ivkovic, V, and Zhang, Q. Wearable brain imaging with multi-modal physiological recording. J Appl Physiol. (2018) 124:564–72. doi: 10.1152/japplphysiol.00297.2017
17. Yu, X, Huang, J, Zhang, S, and Metaxas, DN. Face landmark fitting via optimized part mixtures and cascaded deformable model. IEEE Trans Pattern Anal Mach Intell. (2016) 38:2212–26. doi: 10.1109/TPAMI.2015.2509999
18. Non-Contact Sleep, Vitals, and Behavior Sensing. National Aeronautics and Space Administration TechPort. Available at: https://techport.nasa.gov/view/96043 (Accessed March 27, 2023).
19. Lieberman, P, Morey, A, Hochstadt, J, Larson, M, and Mather, S. Mount Everest: a space analogue for speech monitoring of cognitive deficits and stress. Aviat Space Environ Med. (2005) 76:B198–207.
20. Driskell, T, Salas, E, Burke, CS, and Driskell, JE. A lexical approach to assessing stress: development and proof-of-concept. Hum Factors. (2021) 187208211045167:001872082110451. doi: 10.1177/00187208211045167
21. Conversational Intelligent Agents for Astronaut Behavioral Health and Performance. National Aeronautics and Space Administration TechPort. Available at: https://taskbook.nasaprs.com/tbp/index.cfm?action=public_query_taskbook_content&TASKID=14096 (Accessed November 02, 2022).
22. Autonomous Medical Response Agent for Prolonged Field Care in Space, Phase II. National Aeronautics and Space Administration TechPort. Available at: https://techport.nasa.gov/view/113111 (Accessed March 27, 2023).
23. Kim, HC, Lee, W, Kowsari, K, Weisholtz, DS, and Yoo, SS. Effects of focused ultrasound pulse duration on stimulating cortical and subcortical motor circuits in awake sheep. PLoS One. (2022) 17:e0278865. doi: 10.1371/journal.pone.0278865
24. McIntire, LK, McKinley, RA, Goodyear, C, McIntire, JP, and Brown, RD. Cervical transcutaneous vagal nerve stimulation (ctVNS) improves human cognitive performance under sleep deprivation stress. Commun Biol. (2021) 4:634. doi: 10.1038/s42003-021-02145-7
25. Dmochowski, GM, Shereen, AD, Berisha, D, and Dmochowski, JP. Near-infrared light increases functional connectivity with a non-thermal mechanism. Cereb Cortex Commun. (2020) 1:tgaa004. doi: 10.1093/texcom/tgaa004
26. Sorensen, MD, Harper, JD, Hsi, RS, Shah, AR, Dighe, MK, Carter, SJ, et al. B-mode ultrasound versus color Doppler twinkling artifact in detecting kidney stones. J Endourol. (2013) 27:149–53. doi: 10.1089/end.2012.0430
27. Marshall-Goebel, K, Laurie, SS, Alferova, IV, Arbeille, P, Aunon-Chancellor-SM,, Ebert, DJ, et al. Assessment of jugular venous blood flow stasis and thrombosis during spaceflight. JAMA Netw Open. (2019) 2:e1915011. doi: 10.1001/jamanetworkopen.2019.15011
28. Lin, W, Xia, Y, and Qin, YX. Characterization of the trabecular bone structure using frequency modulated ultrasound pulse. J Acoust Soc Am. (2009) 125:4071–7. doi: 10.1121/1.3126993
29. Wideband Single Crystal Transducer for Bone Characterization. TRS Ceramics Inc. Small Business Innovation Research. Available at: https://www.sbir.gov/sbirsearch/detail/388158 (Accessed March 22, 2023).
30. Pachade, S, Coronado, I, Abdelkhaleq, R, Yan, J, Salazar-Marioni, S, Jagolino, A, et al. Detection of stroke with retinal microvascular density and self-supervised learning using OCT-A and fundus imaging. J Clin Med. (2022) 11:7408. doi: 10.3390/jcm11247408
31. The CytoTracker: Point-of-Care Complete Blood Count. National Aeronautics and Space Administration TechPort. Available at: https://techport.nasa.gov/view/96051 (Accessed March 27, 2023).
32. In-Flight Blood Analysis Technology for Astronaut Health Monitoring. National Aeronautics and Space Administration TechPort. Available at: https://techport.nasa.gov/view/23235 (Accessed March 27, 2023).
33. Cell Phone-Based Lateral Flow Assay for Blood Biomarker Detection, Phase II. National Aeronautics and Space Administration TechPort. Available at: https://techport.nasa.gov/view/16779 (Accessed March 27, 2023)
34. Reusable Handheld Electrolytes and Lab Technology for Humans (rHEALTH Sensor), Phase 2E. National Aeronautics and Space Administration TechPort. Available at: https://techport.nasa.gov/view/103021 (Accessed March 27, 2023).
35. Smart Phone Fluorescent Chem8, Phase I. National Aeronautics and Space Administration TechPort. Available at: https://techport.nasa.gov/view/9886 (Accessed March 27, 2023).
36. Room Temperature Stable Basic Metabolic Panel. National Aeronautics and Space Administration TechPort. Available at: https://techport.nasa.gov/view/96048 (Accessed March 27, 2023).
37. InnaMed TeleLab Platform. National Aeronautics and Space Administration TechPort. Available at: https://techport.nasa.gov/view/96040 (Accessed March 27, 2023).
38. Soller, BR, Zou, F, Ryan, KL, Rickards, CA, Ward, K, and Convertino, VA. Lightweight noninvasive trauma monitor for early indication of central hypovolemia and tissue acidosis: a review. J Trauma Acute Care Surg. (2012) 73:S106–11. doi: 10.1097/TA.0b013e318260a928
39. Noninvasive Biosensor Algorithms for Continuous Metabolic Rate Determination. National Aeronautics and Space Administration TechPort. Available at: https://techport.nasa.gov/view/23210 (Accessed March 27, 2023).
40. Wang, M, Yang, Y, Min, J, Song, Y, Tu, J, Mukasa, D, et al. A wearable electrochemical biosensor for the monitoring of metabolites and nutrients. Nat Biomed Eng. (2022) 6:1225–35. doi: 10.1038/s41551-022-00916-z
41. Anderson, MJ, Song, Y, Fan, H, Wright, JG, Ren, Z, Hua, DH, et al. Simultaneous, multiplex quantification of protease activities using a gold microelectrode array. Biosens Bioelectron. (2020) 165:112330. doi: 10.1016/j.bios.2020.112330
42. Wearable Health Monitoring Systems, Phase II. National Aeronautics and Space Administration TechPort. Available at: https://techport.nasa.gov/view/6426 (Accessed March 27, 2023).
43. Lightweight, Wearable Metal Rubber Textile-Sensor for In-Situ Lunar Autonomous Health Monitoring, Phase II. National Aeronautics and Space Administration TechPort. Available at: https://techport.nasa.gov/view/6447 (Accessed March 27, 2023).
44. Lunar Health Monitor, Phase II. National Aeronautics and Space Administration TechPort. Available at: https://techport.nasa.gov/view/6434 (Accessed March 27, 2023).
45. Vitali, RV, Miller, MJ, and Stirling, LA. Methodology for the scientific physical and operations characterization (SPOC) of terrestrial fieldwork. 50th International Conference on Environmental Systems 2020. Available at: https://hdl.handle.net/2346/86391 (Accessed July 31, 2020).
46. May, PC, Bailey, MR, and Harper, JD. Ultrasonic propulsion of kidney stones. Curr Opin Urol. (2016) 26:264–70. doi: 10.1097/MOU.0000000000000276
47. Zderic, V, Keshavarzi, A, Noble, ML, Paun, M, Sharar, SR, Crum, LA, et al. Hemorrhage control in arteries using high-intensity focused ultrasound: a survival study. Ultrasonics. (2006) 44:46–53. doi: 10.1016/j.ultras.2005.08.002
48. Uddin, SMZ, Cheng, J, Lin, W, and Qin, YX. Low-intensity amplitude modulated ultrasound increases osteoblastic mineralization. Cell Mol Bioeng. (2011) 4:81–90. doi: 10.1007/s12195-010-0153-8
49. Wearable, sustained acoustic medicine for Back pain National Aeronautics and Space Administration TechPort. Available at: https://techport.nasa.gov/view/87682. (Accessed May 2, 2023).
50. Tononi, G, Riedner, B, Hulse, B, Ferrarelli, F, and Sarasso, S. Enhancing sleep slow waves with natural stimuli. Medicamundi. (2010) 54:82–8.
51. OASIS: Optimizing Auditory Stimulation to Improve Cognitive Performance Using SmartSleep. National Aeronautics and Space Administration TechPort. Available at: https://techport.nasa.gov/view/96044 (Accessed May 2, 2023).
52. Evaluation of SmartSleep Technology for improving the efficiency and restorative quality of sleep in healthy adults in order to mitigate cognitive performance deficits due to sleep restriction and emergency awakening. National Aeronautics and Space Administration TechPort. Available at: https://techport.nasa.gov/view/96038 (Accessed May 2, 2023).
53. LumosTech SmartSleep Mask for Circadian Realignment in Space and on Earth. National Aeronautics and Space Administration TechPort. Available at: https://techport.nasa.gov/view/93296 (Accessed March 22, 2023).
54. Menezes, AA, Cumbers, J, Hogan, JA, and Arkin, AP. Towards synthetic biological approaches to resource utilization on space missions. J R Soc Interface. (2015) 12:20140715. doi: 10.1098/rsif.2014.0715
55. Menezes, AA, Montague, MG, Cumbers, J, Hogan, JA, and Arkin, AP. Grand challenges in space synthetic biology. J R Soc Interface. (2015) 12:20150803. doi: 10.1098/rsif.2015.0803
56. McNulty, MJ, Berliner, AJ, Negulescu, PG, McKee, L, Hart, O, Yates, K, et al. Evaluating the cost of pharmaceutical purification for a long-duration space exploration medical foundry. Front Microbiol. (2021) 12:700863. doi: 10.3389/fmicb.2021.700863
57. Foale, CM, Kaleri, AY, Sargsyan, AE, Hamilton, DR, Melton, S, Martin, D, et al. Diagnostic instrumentation aboard ISS: just-in-time training for non-physician crewmembers. Aviat Space Environ Med. (2005) 76:594–8.
58. Hurst, VW, Peterson, S, Garcia, K, Ebert, D, Ham, D, Amponsah, A, et al. Concept of operations evaluation for using remote-guidance ultrasound for exploration spaceflight. Aerosp Med Hum Perform. (2015) 86:1034–8. doi: 10.3357/AMHP.3244.2015
59. McQuillen, JB, McKay, TL, Griffin, DW, Brown, DF, and Zoldak, JT. Final report for intravenous fluid generation (IVGEN) spaceflight equipment. Washington, DC: National Aeronautics and Space Administration (2011).
60. Erythromer: Nanoscale Bio-synthetic Red Blood Cell Substitute. Kalocyte In: Small Business Innovation Research. Available at: https://www.sbir.gov/sbirsearch/detail/1568385 (Accessed March 27, 2023).
61. Integration of iRevive with the Lightweight Trauma Module. National Aeronautics and Space Administration TechPort. Available at: https://techport.nasa.gov/view/23271 (Accessed March 23, 2023).
62. Burian, BK, Ebnali, M, Robertson, JM, Musson, D, Pozner, CN, Doyle, T, et al. Using extended reality (XR) for medical training and real-time clinical support during deep space missions. Appl Ergon. (2023) 2023:103902. doi: 10.1016/j.apergo.2022.103902
63. Ebnali, M, Paladugu, P, Miccile, C, Park, SH, Burian, B, Yule, S, et al. Extended reality applications for space health. Aerosp Med Hum Perform. (2023) 94:122–30. doi: 10.3357/AMHP.6131.2023
64. VisualDx Clinical Decision Support for Ultrasound. National Aeronautics and Space Administration TechPort. Available at: https://techport.nasa.gov/view/96055 (Accessed March 27, 2023).
65. Singh, VK, Serebrenik, AA, Fatanmi, OO, Wise, SY, Carpenter, AD, Janocha, BL, et al. The radioprotectant, BIO 300, protects the lungs from total-body irradiation injury in C57L/J mice. Radiat Res. (2023) 199:294–300. doi: 10.1667/RADE-22-00142.1
66. Li, Y, Girgis, M, Jayatilake, M, Serebrenik, A, Cheema, AK, Kaytor, MD, et al. Pharmacokinetic and metabolomic studies with a BIO 300 oral powder formulation in nonhuman primates. Sci Rep. (2022) 12:13475. doi: 10.1038/s41598-022-17807-7
67. Salem, AM, Jackson, IL, Gibbs, A, Poirier, Y, Newman, D, Zodda, A, et al. Interspecies comparison and radiation effect on pharmacokinetics of BIO 300, a Nanosuspension of genistein, after different routes of administration in Mice and non-Human Primates. Radiat Res. (2022) 197:447–58. doi: 10.1667/RADE-21-00114.1
68. Revskaya, E, Chu, P, Howell, RC, Schweitzer, AD, Bryan, RA, Harris, M, et al. Compton scattering by internal shields based on melanin-containing mushrooms provides protection of gastrointestinal tract from ionizing radiation. Cancer Biother Radiopharm. (2012) 27:570–6. doi: 10.1089/cbr.2012.1318
69. Malo, ME, Frank, C, Khokhoev, E, Gorbunov, A, Dontsov, A, Garg, R, et al. Mitigating effects of sublethal and lethal whole-body gamma irradiation in a mouse model with soluble melanin. J Radiol Prot. (2022) 42:011508. doi: 10.1088/1361-6498/ac3dcf
70. Kunwar, A, Adhikary, B, Jayakumar, S, Barik, A, Chattopadhyay, S, Raghukumar, S, et al. Melanin, a promising radioprotector: mechanisms of actions in a mice model. Toxicol Appl Pharmacol. (2012) 264:202–11. doi: 10.1016/j.taap.2012.08.002
71. Schweitzer, AD, Revskaya, E, Chu, P, Pazo, V, Friedman, M, Nosanchuk, J, et al. Melanin-covered nanoparticles for protection of bone marrow during radiation therapy of cancer. Int J Radiat Oncol Biol Phys. (2010) 78:1494–502. doi: 10.1016/j.ijrobp.2010.02.020
72. Link, MM. Space medicine in project mercury. Washington, DC: National Aeronautics and Space Administration Special Publication (1965).
73. Sooy, P. NASA’s Webb telescope tech improves patients’ vision. National Aeronautics and Space Administration, (2019). Available at: https://www.nasa.gov/feature/goddard/2019/nasa-s-webb-telescope-tech-improves-patients-vision (Accessed March 22, 2023).
74. Material for Mars Makes Life-Saving Sutures. National Aeronautics and Space Administration Spinoffs. (2019) Available at: https://spinoff.nasa.gov/Spinoff2019/hm_2.html (Accessed March 22, 2023).
75. Robotic Surgery. National Aeronautics and Space Administration Spinoffs. (2000) Available at: https://spinoff.nasa.gov/spinoff2000/hm1.htm (Accessed March 22, 2023).
76. Du, B, Daniels, VR, Vaksman, Z, Boyd, JL, Crady, C, and Putcha, L. Evaluation of physical and chemical changes in pharmaceuticals flown on space missions. AAPS J. (2011) 13:299–308. doi: 10.1208/s12248-011-9270-0
77. Kirkpatrick, AW, Blaivas, M, Sargsyan, AE, McBeth, PB, Patel, C, Xiao, Z, et al. Enabling the mission through trans-Atlantic remote mentored musculoskeletal ultrasound: case report of a portable hand-carried tele-ultrasound system for medical relief mission. Telemed J E Health. (2013) 19:530–4. doi: 10.1089/tmj.2012.0243
78. Binkley, CE, Kemp, DS, and Braud, SB. Should we rely on AI to help avoid bias in patient selection for major surgery? AMA J Ethics. (2022) 24:E773–80. doi: 10.1001/amajethics.2022.773
Keywords: aerospace, healthcare delivery, innovation, medical technology, performance optimization, remote healthcare
Citation: Scarpa J, Parazynski S and Strangman G (2023) Space exploration as a catalyst for medical innovations. Front. Med. 10:1226531. doi: 10.3389/fmed.2023.1226531
Received: 21 May 2023; Accepted: 30 June 2023;
Published: 19 July 2023.
Edited by:
Enrico Capobianco, Jackson Laboratory, United StatesReviewed by:
Ekaterina Dadachova, University of Saskatchewan, CanadaCopyright © 2023 Scarpa, Parazynski and Strangman. This is an open-access article distributed under the terms of the Creative Commons Attribution License (CC BY). The use, distribution or reproduction in other forums is permitted, provided the original author(s) and the copyright owner(s) are credited and that the original publication in this journal is cited, in accordance with accepted academic practice. No use, distribution or reproduction is permitted which does not comply with these terms.
*Correspondence: Julia Scarpa, anVzOTE0M0BtZWQuY29ybmVsbC5lZHU=
Disclaimer: All claims expressed in this article are solely those of the authors and do not necessarily represent those of their affiliated organizations, or those of the publisher, the editors and the reviewers. Any product that may be evaluated in this article or claim that may be made by its manufacturer is not guaranteed or endorsed by the publisher.
Research integrity at Frontiers
Learn more about the work of our research integrity team to safeguard the quality of each article we publish.