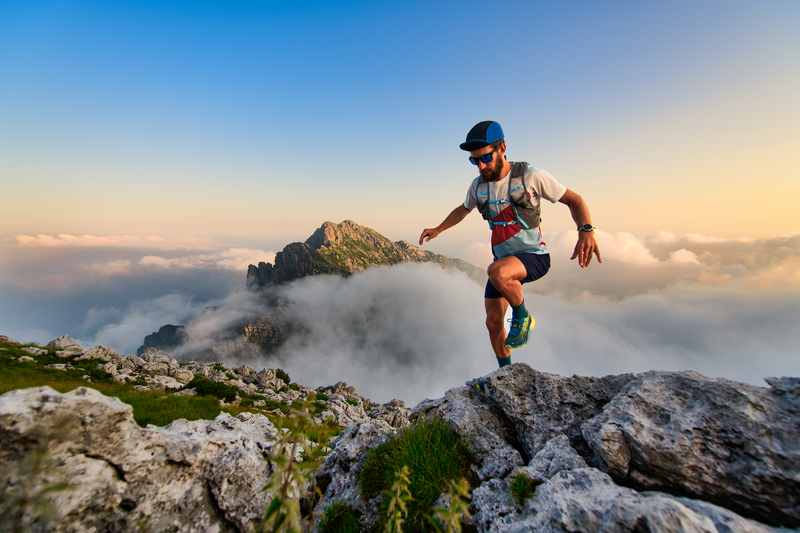
95% of researchers rate our articles as excellent or good
Learn more about the work of our research integrity team to safeguard the quality of each article we publish.
Find out more
REVIEW article
Front. Med. , 28 June 2023
Sec. Infectious Diseases: Pathogenesis and Therapy
Volume 10 - 2023 | https://doi.org/10.3389/fmed.2023.1208866
This article is part of the Research Topic Novel Targets and State of the Art Therapies in ARDS and Sepsis View all 11 articles
SARS-CoV-2 binds to ACE2 receptors, expressed within the lungs. Risk factors for hospitalization include hypertension, diabetes, ischaemic heart disease and obesity–conditions linked by the presence of endothelial pathology. Viral infection in this setting causes increased conversion of circulating Factor XII to its active form (FXIIa). This is the first step in the contact-kinin pathway, leading to synchronous activation of the intrinsic coagulation cascade and the plasma Kallikrein-Kinin system, resulting in clotting and inflammatory lung disease. Temporal trends are evident from blood results of hospitalized patients. In the first week of symptoms the activated partial thromboplastin time (APTT) is prolonged. This can occur when clotting factors are consumed as part of the contact (intrinsic) pathway. Platelet counts initially fall, reflecting their consumption in coagulation. Lymphopenia occurs after approximately 1 week, reflecting the emergence of a lymphocytic pneumonitis [COVID-19 acute respiratory distress syndrome (ARDS)]. Intrinsic coagulation also induces the contact-kinin pathway of inflammation. A major product of this pathway, bradykinin causes oedema with ground glass opacities (GGO) on imaging in early COVID-19. Bradykinin also causes release of the pleiotrophic cytokine IL-6, which causes lymphocyte recruitment. Thromobosis and lymphocytic pneumonitis are hallmark features of COVID-19 ARDS. In this review we examine the literature with particular reference to the contact-kinin pathway. Measurements of platelets, lymphocytes and APTT should be undertaken in severe infections to stratify for risk of developing ARDS.
Severe acute respiratory syndrome coronavirus 2 (SARS-CoV-2) has spread around the world rapidly. This novel beta coronavirus, responsible for coronavirus disease of 2019 (COVID-19) may result in a spectrum of illness ranging from asymptomatic infection to severe respiratory failure and death. COVID-19 is extremely contagious and is transmitted via respiratory droplets. Viral cell entry is enabled via the SARS-CoV-2 spike protein receptor binding domain (RBD) which attaches to the angiotensin-converting enzyme-2 (ACE2) receptor which is expressed in a wide variety of tissues, including pulmonary epithelial cells and the endothelium. With the help of transmembrane serine protease (TMPRSS2), the virus-receptor complex becomes internalized in cells enabling viral replication within the cell. The principal effects of initial infection are cough, fever, breathlessness, anosmia, loss of taste and infiltrates on the chest radiograph or computed tomography (CT). These infiltrates are alveolar filling defects, caused initially by oedema. A proportion of patients develop worsening symptoms and require hospitalization, often due to respiratory failure. These patients also develop coagulopathy, abnormal thrombosis, hypotension and acute renal impairment (1). Risk factors for progressive infection and mortality include age, hypertension, diabetes, ischaemic heart disease and obesity (2) suggesting that endothelial pathology may signify a unifying disease mechanism. Pre-existing endothelial dysfunction may cause excessive activation of the contact system in response to SARS-CoV-2 infection. The contact system is a key component of the innate immune response to inflammatory stimuli, tissue injury and infection. It is initiated by the conversion of the circulating zymogen Factor XII (FXII) to its active form FXIIa. This causes synchronous triggering of the intrinsic clotting pathway and the plasma Kallikrein-Kinin System (KKS). Activation of the intrinsic coagulation pathway causes clotting factor consumption (seen as raised APTT) and thrombus formation. Triggering of the KKS leads to the production of bradykinin, resulting in vascular proliferation, vasodilation, oedema, cough and IL-6 release (3). The diverse manifestations of COVID-19 infection could be due to sustained activation of these systems/pathways. Temporal changes in blood markers may reflect the actions of the contact-kinin system.
FXII is a serine protease that circulates in plasma as a single-chain zymogen. After contact with anionic surfaces such as neutrophil extracellular traps (NETs), polyphosphates, activated platelets or DNA released from damaged cells, FXII undergoes autoactivation to FXIIa which triggers the intrinsic clotting cascade via FXI cleavage to generate FXIa.
The subsequent proteolytic cleavage of factors IX and X ultimately leads to thrombin generation, fibrin formation and clotting (Figure 1). Activated partial thromboplastin time (APTT) measures the length of time taken for blood to clot via the intrinsic and common pathways. Prolonged results can indicate factor deficiencies/depletion or the presence of a competing antibody. APTT is often abnormally prolonged in COVID-19 (4). The mechanism behind this is not fully understood. Studies have identified the antiphospholipid antibody, lupus anticoagulant (LA) (5–7). Findings remain controversial as LA assays can be inaccurate in setting of raised C-reactive protein (CRP) or in patients on anticoagulant therapy (8) and these aspects were not always taken into consideration (9). Importantly, APTT prolongation is also found in LA negative COVID-19 patients (10–12). Prothrombin time (PT), fibrinogen and D-dimer levels are often abnormally elevated in COVID-19 (13, 14), and as the incidence of thromboembolism is known to be much higher than hemorrhagic events (15), APTT prolongation may therefore reflect clotting factor depletion.
Figure 1. Activators of Factor XII. Factor XII is activated upon contact with anionic surfaces leading to dual activation of the Kallikrein-Kinin system and Intrinsic Coagulation cascade.
In addition to triggering the clotting cascade, FXIIa also synchronously activates the KKS; a complex pathway that modulates inflammation, blood pressure control, coagulation and pain (Figure 2). This system is initiated when FXIIa cleaves plasma prekallikrein (PKa) to kallikrein, a serine protease which forms a feedback loop to continue the activation of FXII. Kallikrein then cleaves circulating high-molecular-weight-kininogen (HK), releasing bradykinin and subsequent derivatives. Bradykinin is broken down by ACE receptors. ACE inhibitor drugs are widely used for the treatment of hypertension, heart failure and diabetic nephropathy. Their effects are well established. Between 7 and 25% of people develop a dry cough (3, 16). A lymphocytic alveolitis can also occur (17).
Figure 2. Contact Kinin System: Kallikrein-Kinin system (Blue) FXIIa cleaves pre-kallikrein to kallikrein (positive feedback loop to continue FXII activation), kallikrein cleaves high molecular weight kininogen (HK) to produce bradykinin, further processed to Des-Arg9 bradykinin. Bradykinin and Des-Arg9 bradykinin act at receptors B2 and B1, respectively. Intrinsic coagulation cascade (Orange)–abbreviated pathway.
Bradykinin binds to cell receptor B2, a G-protein-coupled receptor which is ubiquitously expressed in most human tissues (18). Further enzymatic processing of bradykinin produces des-Arg-9 bradykinin (DABK), a ligand of receptor B1, which is present on endothelial cells and upregulated in tissue injury and inflammatory states (19). Stimulation of these receptors has a potent effect; inducing vasodilation, vascular proliferation, micro-vascular permeability, oedema and release of pro-inflammatory cytokines including IL-6 and TNF-⍺ (20–22). Nagashima et al. studied lung tissue immunoexpression of B1 and B2 in post mortem lung samples from mechanically ventilated patients with COVID-19 (n = 24) and influenza (n = 10). Notably, expression of B1 and B2 were significantly increased in both COVID-19 and influenza patients compared to uninfected controls (n = 11) (23).
Bradykinin peptides can be generated independently of FXIIa due to the actions of proteases which trigger distal elements of the KKS. Prolylcarboxypeptidase (PRCP), a regulatory protease involved in the renin-angiotensin system (RAS) can cleave PKa to kallikrein (24). However, PRCP activity has been found to be similar in COVID-19 patients and controls (25). Cleavage of HK and kinin generation also occurs by agents other than kallikrein, such as the protease neutrophil elastase (NE), tryptase, cathepsins and proteinase-3 (PR3). These not only cleave HK but liberate bradykinin-like peptides with the ability to act at B2R (26, 27). Neutrophilia occurs frequently in COVID-19 and is associated with poorer outcomes (28). Neutrophils release a variety of potent enzymes including PR3 and NE (29). PR3 is a destructive protease with microbicidal activity and is capable of extracellular matrix degradation. Furthermore, PR3 can cleave high molecular weight kininogen producing PR3-kinin, which is then processed to bradykinin and des-Arg9-bradykinin in plasma (30). Increased PR3 has been reported in severe COVID-19 (31, 32). NE acts on elastin, collagens and HK - producing E-kinin, which is then cleaved to release bradykinin (26). Compared to healthy controls, progressively increasing NE levels correlated with hospitalization, need for ICU admission and mortality (33).
Wygrecka et al. compared the levels of factor XII (FXII) and its activation products from critically ill COVID-19 patients with samples from influenza patients with severe ARDS (34). FXII was reduced, plasma kallikrein-like activity and HK cleavage products were increased in COVID-19 patients in comparison to influenza ARDS and controls. This shows the decrease in FXII is due to activation (34). A similar pattern of decreased FXII, PKa and HK was reported in a study of 66 intensive care COVID-19 patients (35). Neutrophil extracellular traps (NETS) have been implicated as triggers for the cascade in a study demonstrating the presence of FXIIa alongside NETS in lung parenchyma of COVID-19 patients (36). An increase in serum FXIIa was also seen in a separate group of COVID-19 patients (36). In a study of 128 COVID-19 and 16 healthy controls, admission FXII levels were increased in asymptomatic/ mild/ moderate disease in comparison to controls–possibly reflecting an acute phase response. However, FXII levels were significantly lower in those who progressed to severe disease (37). Importantly this finding is not limited to severely ill COVID-19 patients: reduced FXII levels have also been found in critically ill patients with sepsis (38), likely reflecting increased activation.
Pre-existing endothelial pathology may be a priming factor for contact system activation in COVID-19. The endothelium is a complex organ that controls vascular tone, angiogenesis, clotting and the recruitment of leukocytes and platelets. These functions are regulated by expression of cell adhesion molecules such as E-selectin, intracellular adhesion molecule 1 (ICAM-1), vascular cell adhesion molecule 1 (VCAM-1) and thrombomodulin, an anticoagulant glycoprotein. Endothelial cells rapid responses are aided by organelles which function as storage granules called Weibel-Palade bodies. These store P-selectin, Von-Willebrand factor (vWF), eotaxin-3, interleukin-8, tissue-plasminogen activator, angiopoetin-2 (Ang-2), osteoprotegerin and endothelin-1. These are powerful inflammatory and physiological regulators which are rapidly expelled in response to vessel injury or inflammation. Vascular tone is regulated by endothelin-1, a potent vasoconstrictor. Angiopoietin-2, a form of growth factor, can function as a pro or anti-angiogenesis agent depending on inflammatory conditions and the presence or absence of other growth factors (39). P-selectin and vWF promote platelet and leucocyte adhesion. Activated platelets release adhesion molecules, perpetuating endothelial activation and cell recruitment. Von Willebrand factor polymerizes into large multimers that are size regulated by ADAMTS-13 (A Disintegrin And Metalloprotease with ThrombSpondin Motif type 1 motif, member 13), which is reduced in severely ill COVID-19 patients (40–42). This may reflect secondary consumption due to excessive release of vWF–which has been consistently shown to be markedly raised in the infection (43, 44). In a study examining components secreted from Weibel-palade bodies, blood from 39 patients with moderate/critical/fatal COVID-19 infection had significantly increased levels of plasma vWF, Ang-2 and osteoprotegerin compared to 15 healthy controls (45). When plasma was added to human endothelial cell cultures vWF increased significantly with critical/ fatal case plasma, though not with moderate disease plasma. Furthermore, plasma from fatal cases induced higher intracellular levels, but lower secreted levels of Ang-2, while inducing features of angiogenesis.
COVID-19 infection is also associated with elevated levels of endothelial P-selectin, E-selectin, VCAM-1 and Ang-2 (43, 46–49). At an early stage in the pandemic, Goshua et al. reported a significant increase in vWF factor and P-selectin in both non-ICU and ICU COVID-19 patients compared to controls (46). Notably, disease severity and mortality directly correlated with elevated vWF (46) and this trend has subsequently been shown in further studies (44, 50). Levels of soluble thrombomodulin (sTM) were found to have a mortality correlation (46). Philippe et al. studied results from patients with COVID-19 (n = 208) and unaffected controls (n = 29) (44). Ang-2, VCAM-1 and E-selectin were included in their analysis. Patients had bloods drawn on admission and were classified into groups (outpatient, non-critical and critical) according to outcomes over next 48 h. Compared to the control group, VCAM-1 was significantly increased in both the critical group and non-critical group. Elevated Ang-2, sTM and E-selectin was only demonstrated in the critical group. None of these markers varied significantly between the non-COVID-19 control group and the outpatient cohort of COVID-19 patients. A markedly different pattern was observed with vWF, with levels significantly elevated in all COVID-19 patients compared to controls. In addition, vWF levels further increased according to disease severity. Both of these results were seen in a smaller study of 50 mild/mod/severe patients and in a study of 28 patients (40, 51).
In a separate hospitalized group, E-selectin levels were raised in severe disease only, but Von Willebrand factor was raised in all patient groups with COVID-19. In 20 of the evaluated patients with mild/mod/severe disease, vWF levels remained elevated in 57% 30 days after admission (52). A larger study of 203 COVID-19 patients found that over 80% had raised vWF levels 3 months after the onset of infection (43). A study of 215 patients investigated endothelial markers in relation to transfer factor (DLCO) 6 months after COVID-19 illness. Patients with the lowest DLCO had higher levels of ICAM-1 and angiopoetin-2 (53). The prolonged lag in normalization may reflect the severity of the endothelial damage occurring during infection. Alternatively, it is possible that patients with persistent, abnormal endothelial markers may have had baseline abnormalities which accentuated their illness.
Viral infection causes oxidative stress and excess protein production in the endoplasmic reticulum. This overwhelms the regulatory unfolded protein response and results in loss of cellular homeostasis (54), which may accentuate the vigorous systemic response to COVID-19 in patients with pre-existing endothelial dysfunction. This includes the known high risk groups of older age, hypertension, ischemic heart disease, obesity, COPD and diabetes. For example, untreated hypertension has been associated with higher levels of vWF and P-selectin (55, 56). Levels improve with treatment though may still remain higher than control results (57). Other studies of hypertensive disorders have also found raised E-selectin (56, 58). Schumacher et al. found that patients with coronary artery disease (n = 193) have elevated levels of ICAM-1, VCAM-1 and P selectin compared to healthy controls (n = 193) (59). Patients with obesity have elevated levels of chemokines IL-6, TNF⍺ and MCP-1 and increased levels of endothelial ICAM-1, E-selectin, and P-selectin compared to those with a normal BMI (60). Elevated levels of vWF, E-selectin, ICAM-1 and VCAM-1 can be seen in patients with Type II diabetes–and can also precede diagnosis (61–63). Interestingly, increased vWF has also been found in patients experiencing acute COPD exacerbations (64). Hospital admissions due to COPD exacerbations are linked to a markedly increased risk of cardiovascular events (65). Polatli et al. compared levels of vWF in a healthy control group (n = 16), patients with stable COPD (n = 33) and patients with acute COPD exacerbations (n = 26). They found that the levels were progressively higher between the groups though not reaching statistical significance between stable/acute COPD (66).
Post-mortem findings from patients with severe COVID-19 lung disease have shown marked endothelial dysfunction, lymphocytic infiltration, intussusceptive angiogenesis and widespread thrombosis with microangiopathy (67). Pathological abnormalities can also be seen in asymptomatic patients. Resected lung samples in patients who were subsequently found to have COVID-19 in the postoperative period have shown alveolar damage, oedema, proteinaceous exudates, vascular congestion, alveolar hemorrhage and interstitial inflammatory infiltrates (68–70). Histological abnormalities are not limited to the respiratory system, demonstrating the systemic nature of the infection. Lymphocytic infiltration, endotheliitis, oedema, microthrombi and vascular proliferation are found in organs such as small bowel, spleen, kidney and liver (67, 70, 71). As neurological symptoms and complications are commonly seen (confusion, delirium, headache, autonomic dysfunction, stroke and meningoencephalitis) histological examination of brain tissue is of significance in this systemic effect. There are ischemic lesions, oedema and microhaemorrhages. Lymphocytic infiltration is seen, affecting the brainstem in particular (72).
These findings are indicative of dysfunction at the epithelial/endothelial interface. Endothelial viral infection and endotheliitis in heart, lung, kidney and liver has been reported (73). A closer look at the mechanism of viral entry may provide an explanation for endothelial disruption. The ACE2 receptor serves as the binding site for SARS-CoV-2. Membrane bound ACE2 undergoes cleavage by ADAM Metallopeptidase Domain 17 (ADAM17) to form soluble ACE2, which plays a critical role in the renin-angiotensin system and the cleavage of numerous bioactive peptides including Des-Arg9-bradykinin (DABK). ACE2 is expressed in lung epithelial cells, the starting point of infection. It is known to be increased in the airways of COPD and current smokers (74). It is found throughout endothelial surfaces in veins, arteries and arterioles and also in arterial smooth muscle cells (75). However, an RNA sequencing study found that ACE2 expression on endothelial cells was low in comparison to epithelial cells from respiratory, gastrointestinal and skin sites (76). Similarly, early in the pandemic ACE2 expression was shown to be highest in small intestine, heart, kidney, adrenal glands, with blood vessels grouped among tissues with lowest expression (77). However, the endothelium is capable of mounting instant systemic responses and it is possible that low levels of expression are sufficient for damage. Bordoni et al. demonstrated that SARS-CoV-2 viral replication can occur in both airway epithelial and pulmonary endothelial cells (78). They also showed that culture of infected endothelial cells with non-infected pulmonary epithelial cells induced low levels of viral RNA in the pulmonary epithelial cells – though not vice versa. Moreover, viral infection of endothelial cells did not cause an increase in adhesion molecules (E-selectin, ICAM-1 and VCAM-1), but infection of epithelial cells resulted in increased E-selectin and VCAM-1, suggesting that the endothelial damage observed in COVID-19 is initiated by a cytotoxic effect from neighboring infected pulmonary epithelial cells.
Cells infected with SARS-CoV2 undergo pyroptosis leading to cytokines and danger associated molecular patterns (DAMPs). The response to these signals includes the release of neutrophil extracellular traps (NETS) and the activation of platelets. In addition to the damage caused by cell death, internalization of the viral/ACE2 complex leads to a relative ACE2 deficiency, which is significant, as ACE2 plays a vital role in the renin-angiotensin system by converting angiotensin II to angiotensin 1–7 (a vasodilator with anti-inflammatory, anti-thrombotic, antiproliferative and antifibrotic, activity) (79). The beneficial effects of ACE2 have been investigated in mouse models, showing that ACE2 deficient mice with acute lung injury improved following administration of recombinant ACE2 (80). Downregulation of the normal ACE2 function in COVID-19 leads to increased tissue and vessel exposure to angiotensin II, characterized by vasoconstriction, enhanced thrombosis, cell proliferation, increased tissue permeability, and cytokine production (81). Most importantly, a key agent of the contact-kinin pathway is bradykinin. ACE2 metabolizes des-Arg9-bradykinin.
Blood results in hospitalized patients with COVID-19 often follow predictable trends, correlating with stage of infection. Blood markers can also indicate severity of disease and aid in predicting outcomes. Lymphopenia is regarded as a cardinal finding, occurring in approximately two-thirds of people with COVID-19 (82). The lowest values occur after 7 days from symptom onset (83), reflecting recruitment to affected tissues. Patients with more severe disease tend to have lower lymphocyte counts at point of admission and have a more prolonged period of lymphopenia than those with mild disease. A retrospective study described admission blood results and subsequent dynamic changes for 548 inpatients (84). In severe disease, average counts were lower than in patients with mild disease. Non-survivors display very low levels on admission without appreciable lymphocyte recovery (84, 85).
Neutrophils usually rise from infection onset (85). Neutrophilia is associated with disease severity and mortality (28). Throughout the pandemic, lymphocyte and neutrophil results have been combined to form the neutrophil/lymphocyte ratio. A score of >11.75 predictive of mortality in hospitalized patients (86). Evidence has suggested that neutrophils may promote organ injury and coagulopathy via direct tissue infiltration and subsequent formation of Neutrophil Extracellular Traps (NETs) (87). NETS are networks of extracellular fibers composed of DNA and histones complexes. Structurally, they play an important role in the entrapment of microbes. They are potent activators of FXII and thus mediators of thromboinflammation. There is evidence to suggest that neutrophils in COVID-19 patients produce higher levels of NETs and also longer complexes in comparison to those from healthy controls (88).
Platelet counts are also affected by COVID-19 infection. Thrombocytopenia is present on admission in over a third of patients (89) although it is typically mild. Studies correlating platelets with day of symptoms have shown that counts begin drop from infection onset and are markedly lower at 7 days post symptom onset. This is often overlooked as the reduction may still fall within normal parameters. The sudden decrease in platelets may reflect depletion due to activation and subsequent consumption. At a later stage in infection, a rebound increase occurs (90, 91)—often meeting criteria for thrombocytosis. This pattern was also observed in the 2002 SARS infection (92). Activated platelets are likely to be a major contributing factor to the disease pathology in COVID-19. Patients with critical disease have increased levels of soluble P-selectin and CD40 ligand (circulating markers of platelet activation) (93). The use of antiplatelet agents has been explored. Anti-platelet therapy has a number of potentially beneficial mechanisms, including inhibition of platelet aggregation, reduction of platelet activation inflammation, and blocking of neutrophil extracellular traps. The RECOVERY trial investigated the use of aspirin in hospitalized COVID-19 patients and found no difference in mortality compared to standard care (94). However, there may be a role for antiplatelet agents in the prevention of disease severity. A cohort study of 984 COVID-19 patients (253 pre-admission aspirin, 751 not on aspirin) reported that that requirement for respiratory support (as defined by non-invasive or invasive ventilation) was significantly lower in the pre-hospital aspirin group: 33% vs. 49%. They also found that this group had a lower neutrophil to lymphocyte ratio on admission (p = 0.013) (95). In addition, a large, propensity matched study found there was a 2.6% absolute reduction in mortality with pre-hospital antiplatelet agents (96).
There are limited studies reporting dynamic coagulation changes according to day of symptoms as the majority of studies report findings by day of admission. In a retrospective analysis of 843 COVID-19 inpatients the APTT was significantly higher after 5 days of symptoms, but not after 10 or 13 days (97). This is confirmed by a separate group which reported raised APTT levels from day 5 of symptoms in patients with severe disease which then decreased until day 10 (98). Similarly a significantly higher APTT level was seen in the first week of symptoms compared to the 4th week (99). Studies reporting dynamic changes per day of admission have also demonstrated this trend. A meta-analysis of coagulation factors in COVID-19 reported that APTT levels decreased from point of admission, day 4 and onwards (100). A study of 1,131 hospitalized patients (36 severe) reported a slightly increasing trend in APTT and PT for the severe group and a slightly decreasing trend in the mild group within the first 10 days of admission (4). There was no temporal change in D-dimer for the mild group, but an increasing trend was observed in the severe group, a finding similar to a study of 320 hospitalized patients (101). Smadja et al. studied temporal trends of fibrin monomers, which are generated from the cleavage of fibrinogen from thrombin. They evaluated results from 246 inpatients during their first 9 days of hospitalization and demonstrated an increasing trend until approximately day 6 of admission in critically ill patients (102).
In addition to abnormal hematological parameters, numerous pro-inflammatory cytokines have been detected and associated with the disease evolution of COVID-19. Studies of COVID-19 inpatients have revealed elevated levels of TNF-α, IFN-γ, IL-6, IL-8 and IL-10 (103–105). IL-6 is one of the most widely studied cytokines, leading to the utilization of targeted therapies such as tocilizumab and sarilumab. IL-6 is implicated in multiple processes, however it has a strong lymphotrophic effect, recruiting lymphocytes to the site of inflammation. Studies have consistently shown that elevated levels are predictive of disease severity and mortality (103, 104, 106). A cross sectional observation study of 272 COVID-19 inpatients demonstrated that a value of >79.9 pg./mL was predictive of unfavorable outcomes in terms of respiratory support and mortality (107). Santa Cruz et al. demonstrated that IL-6 levels peaked between 7 and 10 days post symptom onset in hospitalized patients (108). In keeping with risk factors for severe disease, IL-6 levels are higher in men and increase with age (105). This may be the link between high levels and severe lymphopenia and severe disease. It is notable that bradykinin causes release of IL-6.
Chest imaging abnormalities are a signature feature of COVID-19 disease and may even be observed in asymptomatic individuals. Chest radiographs and CT imaging can demonstrate ground glass, oedema, consolidation and fibrotic change. For patients who require hospitalization, these changes also follow trends that can help to distinguish stage of disease. Many studies have correlated imaging findings with days of symptoms. Patients may have imaging changes without symptoms; a meta-analysis of 231 asymptomatic cases reported that 63% had findings on CT imaging–the majority were ground glass opacities (GGO) (109). Within the first 7 days from symptom onset, GGOs are the main radiographic finding (83) and are usually bilateral and favor mid/lower zones (110). After 7 days, consolidation increases and may lead to respiratory failure and ARDS in a proportion of patients. Management involves escalated therapy with ventilatory support via NIV/CPAP and may require treatment in intensive care with invasive ventilation, which is associated with a high mortality (111). A meta-analysis of risk factors for COVID-19 severity reported that male sex, hypertension, cardiovascular, cerebrovascular, diabetes and respiratory disease were significantly associated with progression to ARDS (112). Hospitalized patients have a high risk of venous thromboembolic disease. A meta-analysis of hospitalized patients reported a pooled incidence of pulmonary embolism in 14.7% of cases (113). Ackermann et al. carried out high resolution Syncroton imaging on lung samples from 31 COVID-19 patients who had died from respiratory failures, showing partial/total occlusion in sub segmental pulmonary arteries (114).
In addition to parenchymal changes and thrombosis, studies have reported enlargement/dilation of sub segmental blood vessels at an early stage of infection/ on admission to hospital (110, 115). Bianco et al. investigated this phenomenon in COVID-19 patients and compared the findings with CT scans carried out in patients with influenza pneumonia (116). They found that vascular enlargement was present in 90% (45/50) COVID-19 patients, but only seen in 24% (12/50) patients with influenza pneumonia. Poletti et al. carried out an analysis of the thoracic vasculature in COVID-19 patients (n = 279), Influenza patients (n = 159) and patients with normal CT chest imaging (n = 634). Blood vessel volume percentage (BV%) was calculated for small, medium and large vessels. In COVID-19, small vessel BV% were decreased in comparison to normal controls (14% vs. 18%), but larger vessels were significantly bigger (15% vs. 11%) (117). It is possible that these vascular changes develop due to dysregulated release of bradykinin and alterations in the RAS as a consequence of ACE2 downregulation.
The contact system is known to be regulated by several physiological inhibitors. C1-esterase inhibitor (C1-INH) is the main inhibitor of FXIIa and FXIa and also acts to inhibit plasma kallikrein and the complement classical and lectin pathways. C1 inhibitor deficiency is the main cause for hereditary episodic angioedema (HAE); a rare genetic condition characterized by bradykinin mediated episodes of oedema. Consequently, individuals with C1-INH HAE have reduced regulation of the coagulation system and are at an increased risk of thromboembolic disease (118)—a finding recently replicated in a mouse model study (119). There have been differing reports of C1-INH in COVID-19. At the start of the pandemic, a study of admission blood results from 154 COVID-19 inpatients reported elevated levels of C1-INH which correlated positively with peak CRP, LDH and ferritin (120). A smaller study also reported increased C1-INH levels in 5 patients with severe disease (121). Conversely, 27 COVID-19 positive hemodialysis (HD) patients compared with 32 COVID-19 negative HD patients had significantly lower levels of CI-INH in the positive group. However, both HD groups were elevated in comparison to healthy controls (122). Other agents that can inhibit FXIIa (to a lesser extent) include antithrombin, α2-antiplasmin and α2-macroglobulin (123). Antithrombin targets FXIIa, FXIa and plasma kallikrein. Several studies have identified lower antithrombin levels in COVID-19 non-survivors compared to surviving groups (123–126). α2-antiplasmin is the main inhibitor of plasmin and can also inhibit FXIIa. Data on α2-antiplasmin levels in COVID-19 is limited, however similar results have been found between healthy control groups and admission values for COVID-19 patients (127, 128). α2-macroglobulin regulates several proteases including kallikrein and FXIIa. De Laat-Kreemers et al. reported that α2-macroglobulin levels were within normal range in 133 COVID-19 inpatients, but significantly lower levels were noted in patients with thrombosis compared to those without (129).
C1 esterase inhibitor - inhibitor of FXIIa and FXIa and also acts to inhibit plasma kallikrein and the complement classical and lectin pathways. A small case–control study compared the outcomes of 5 COVID-19 patients who received C1-esterase inhibitor with 15 matched controls. The baseline characteristics and admission laboratory parameters were similar between groups. Eight patients (53%) in the matched control group died or required mechanical ventilation compared to only 1 (20%) in the C1-esterase inhibitor group (121). Another study reported the results of a randomized, open label trial of C1-esterase inhibitor, bradykinin B2 receptor antagonist (icatibant) or standard care alone (n = 9, 10, 9 respectively) (130). COVID-19 patients with severe infection (radiological pneumonia; SpO2 ≤ 94% in ambient air or Pa02/FiO2 ≤ 300 mmHg) were recruited and allocated to treatment arms 1:1:1 up to day 12 of symptoms. There were no significant differences between groups in terms of time to clinical improvement or mortality. There was an improvement in lung CT scores. The size of this study would require a strong signal to identify clinical improvement or mortality.
Plasma kallikrein inhibitors have been developed for use in hereditary episodic angioedema (HAE). Lanadelumab and berotralstat are licensed for the prevention of recurrent attacks. Lanadelumab is administered as a subcutaneous injection every 2–4 weeks, depending on disease stability. Trials in COVID-19 are currently underway. Berotralstat is taken orally once per day. Both medications have been shown to significantly reduce the incidence of attacks compared to placebo (131, 132). Ecallantide is a plasma kallikrein inhibitor used to treat acute HAE attacks (133). Donidalorsen is an antisense oligonucleotide treatment which results in decreased production of PK from the liver. A phase 2 study has demonstrated a reduced rate of angioedema attacks compared to placebo (p = <0.001) (134).
Garadacimab, a novel FXIIa inhibitor has been recently trialed in a phase 1 study (135). Dose-dependent increases in plasma concentration and pharmacodynamic effects in kinin and coagulation pathways were observed. Trials are currently underway in COVID-19.
Bradykinin receptor activation may cause the radiological oedema, hypotension and cytokine release seen in early COVID-19 infection. The use of a receptor antagonist may interrupt this pathway and prevent progression to secondary damage, as defined by inflammatory cell infiltrates, thrombosis and vascular injury. Icatibant is a B2 receptor antagonist that has been in use since 2008 for the treatment of oedema caused by hereditary angioedema. It has a rapid onset of action, with median time of 2–2.5 h to symptom relief following a single dose (136, 137). It has a short half-life of 1.48 +/− 0.35 h (138). Published data on the use of icatibant in COVID-19 is limited to four studies and two case reports. Importantly, there have been no safety concerns noted regarding the use of icatibant in COVID-19 in existing reports.
A case report of a single patient with severe COVID-19 infection described a favorable clinical response after icatibant (139). Giol et al. reported radiological improvement in a COVID-19 patient who received icatibant as a treatment for ACE inhibitor induced angioedema (140). Van de Veerdonk et al. described decreased oxygen requirements in COVID-19 patients (n = 9) treated with open label icatibant compared to standard care (n = 18). After 24 h, 89% of patients receiving Icatibant had a reduction of at least 3 L of oxygen compared to only 17% of controls (141). Mansour et al. carried out a randomized, open-label trial with three treatment arms; icatibant, C1 esterase inhibitor and no trial drug (“usual care”) (130). Eligible patients had hypoxia, radiological COVID-19 pneumonia and were within 12 days of symptom onset. Patients in the icatibant arm received 30 mg three times per day for 4 days. The C1 esterase group were administered a dose on days 1 and 4. The main outcomes were time to clinical improvement as defined by the WHO ordinal scale and improvement in lung CT scoring. There were no significant differences between the groups.
Malchair et al. carried out a randomized, open label trial of icatibant 30 mg three times per day for 3 days plus standard care compared to standard care alone. Patients were eligible if requiring supplemental oxygen, but not high flow oxygen or ventilation. Patients were required to have a positive PCR or antigen test within 10 days of randomization although symptom onset was not recorded. The primary outcome was clinical response (defined as category 2 or lower of the WHO ordinal scale, sustained for 48 h) by day 10 or discharge. The primary outcome was assessed for 37 and 36 patients, respectively. 73% of patients in the icatibant arm (27/37) and 53% of standard care patients (20/36) met the clinical response target, although this did not meet statistical significance. However, clinical response was maintained at day 28 by all of the patients in the icatibant arm compared to controls (p = 0.011) and icatibant patients had a significantly shorter inpatient admission, 8 days vs. 10 (p = 0.014) (142).
A platform study has recently published data for the first seven medications trialed (including icatibant). The trial recruited patients with severe/ critical disease (requiring ≥6 L of oxygen) and an average symptom history of 9–10 days. There were 96 patients enrolled in the icatibant arm. Over half of the patients required ≥15 L of oxygen and a third were on non-invasive or mechanical ventilation. The primary aim was to find a large signal in terms of improved recovery time and mortality. This was not achieved for any of the medications used (143).
Activation of the bradykinin pathway begins at infection onset. In a proportion of cases this process eventually leads to inflammatory cell infiltration and tissue damage. These features are most evident at a later stage of the illness (>7 days post symptom onset). At this point, lymphocyte counts are lowest and neutrophil counts increase. Chest radiological appearances demonstrate progression from initial ground glass appearance (oedema) to dense consolidation. It is acknowledged that widespread vaccine uptake and the emergence of other variants are associated with milder illness in COVID-19. However, patients with vascular co-morbidities remain at risk of infection progression and this may be due to accentuation of the contact-kinin system in setting of endothelial dysfunction.
The study authors are conducting a proof of concept trial of icatibant in hospitalized patients with early COVID-19 infection. The aim is to trial bradykinin blockade in early, potentially reversible disease, before the onset of secondary damage and acute respiratory distress syndrome (ARDS). Patients who have had symptoms for more than 7 days will not be included. The primary outcome is to determine the effect on the Alveolar-arterial gradient (as measured by arterial blood gas before and after treatment). If an improvement in oxygenation is found, icatibant or alterative medications targeting the KKS could be tested on a larger scale. This could potentially change the management at point of admission for early COVID-19, preventing progression to respiratory failure. There may be wider implications for lung injury, as a study of bradykinin inhibition showed an improvement in the 28-day risk-adjusted survival in patients with SIRS from gram-negative infections (144).
All authors listed have made a substantial, direct, and intellectual contribution to the work and approved it for publication.
MB is funded by the R&D office for an NIHR adopted COVID-19 study (Grant number: COM/5612/20).
The authors declare that the research was conducted in the absence of any commercial or financial relationships that could be construed as a potential conflict of interest.
All claims expressed in this article are solely those of the authors and do not necessarily represent those of their affiliated organizations, or those of the publisher, the editors and the reviewers. Any product that may be evaluated in this article, or claim that may be made by its manufacturer, is not guaranteed or endorsed by the publisher.
1. Lanzani, C, Simonini, M, Arcidiacono, T, Messaggio, E, Bucci, R, Betti, P, et al. Bio angels for COVID-BioB study group. Role of blood pressure dysregulation on kidney and mortality outcomes in COVID-19. Kidney, blood pressure and mortality in SARS-CoV-2 infection. J Nephrol. (2021) 34:305–14. doi: 10.1007/s40620-021-00997-0
2. Yang, J, Zheng, Y, Gou, X, Pu, K, Chen, Z, Guo, Q, et al. Prevalence of comorbidities and its effects in patients infected with SARS-CoV-2: a systematic review and meta-analysis. Int J Infect Dis. (2020) 94:91–5. doi: 10.1016/j.ijid.2020.03.017
3. Fox, AJ, Lalloo, UG, Belvisi, MG, Bernareggi, M, Chung, KF, and Barnes, PJ. Bradykinin-evoked sensitization of airway sensory nerves: a mechanism for ACE-inhibitor cough. Nat Med. (1996) 2:814–7. doi: 10.1038/nm0796-814
4. Xu, W, Fei, L, Huang, CL, Li, WX, Xie, XD, Li, Q, et al. Dynamic changes in coagulation parameters and correlation with disease severity and mortality in patients with COVID-19. Aging (Albany NY). (2021) 13:13393–404. doi: 10.18632/aging.203052
5. Taha, M, and Samavati, L. Antiphospholipid antibodies in COVID-19: a meta-analysis and systematic review. RMD Open. (2021) 7:e001580. doi: 10.1136/rmdopen-2021-001580
6. Siguret, V, Voicu, S, Neuwirth, M, Delrue, M, Gayat, E, Stépanian, A, et al. Are antiphospholipid antibodies associated with thrombotic complications in critically ill COVID-19 patients? Thromb Res. (2020) 195:74–6. doi: 10.1016/j.thromres.2020.07.016
7. Najim, M, Rahhal, A, Khir, F, Aljundi, AH, Abu Yousef, S, Ibrahim, F, et al. Prevalence and clinical significance of antiphospholipid antibodies in patients with coronavirus disease 2019 admitted to intensive care units: a prospective observational study. Rheumatol Int. (2021) 41:1243–52. doi: 10.1007/s00296-021-04875-7
8. Devreese, KMJ, de Groot, PG, de Laat, B, Erkan, D, Favaloro, EJ, Mackie, I, et al. Guidance from the scientific and standardization committee for lupus anticoagulant/antiphospholipid antibodies of the international society on thrombosis and haemostasis: update of the guidelines for lupus anticoagulant detection and interpretation. J Thromb Haemost. (2020) 18:2828–39. doi: 10.1111/jth.15047
9. Favaloro, EJ, Henry, BM, and Lippi, G. Is lupus anticoagulant a significant feature of COVID-19? A critical appraisal of the literature. Semin Thromb Hemost. (2022) 48:55–71. doi: 10.1055/s-0041-1729856
10. Reyes Gil, M, Barouqa, M, Szymanski, J, Gonzalez-Lugo, JD, Rahman, S, and Billett, HH. Assessment of lupus anticoagulant positivity in patients with coronavirus disease 2019 (COVID-19). JAMA Netw Open. (2020) 3:e2017539. doi: 10.1001/jamanetworkopen.2020.17539
11. Ferrari, E, Sartre, B, Squara, F, Contenti, J, Occelli, C, Lemoel, F, et al. High prevalence of acquired thrombophilia without prognosis value in patients with coronavirus disease 2019. J Am Heart Assoc. (2020) 9:e017773. doi: 10.1161/JAHA.120.017773
12. Owaidah, T, Saleh, M, Aguilos, AM, Amri, AA, Maghrabi, K, Owaidah, M, et al. Incidence of lupus anticoagulant in hospitalized covid-19 patients. Am J Blood Res. (2021) 11:317–24.
13. Zhu, J, Pang, J, Ji, P, Zhong, Z, Li, H, Li, B, et al. Coagulation dysfunction is associated with severity of COVID-19: a meta-analysis. J Med Virol. (2021) 93:962–72. doi: 10.1002/jmv.26336
14. Zhang, X, Yang, X, Jiao, H, and Liu, X. Coagulopathy in patients with COVID-19: a systematic review and meta-analysis. Aging (Albany NY). (2020) 12:24535–51. doi: 10.18632/aging.104138
15. Jiménez, D, García-Sanchez, A, Rali, P, Muriel, A, Bikdeli, B, Ruiz-Artacho, P, et al. Incidence of VTE and bleeding among hospitalized patients with coronavirus disease 2019: a systematic review and meta-analysis. Chest. (2021) 159:1182–96. doi: 10.1016/j.chest.2020.11.005
16. Simon, SR, Black, HR, Moser, M, and Berland, WE. Cough and ACE inhibitors. Arch Intern Med. (1992) 152:1698–700. doi: 10.1001/archinte.1992.00400200128023
17. Kidney, JC, O'Halloran, DJ, and FitzGerald, MX. Captopril and lymphocytic alveolitis. BMJ. (1989) 299:981. doi: 10.1136/bmj.299.6705.981
18. Ma, JX, Wang, DZ, Ward, DC, Chen, L, Dessai, T, Chao, J, et al. Structure and chromosomal localization of the gene (BDKRB2) encoding human bradykinin B2 receptor. Genomics. (1994) 23:362–9. doi: 10.1006/geno.1994.1512
19. Qadri, F, and Bader, M. Kinin B1 receptors as a therapeutic target for inflammation. Expert Opin Ther Targets. (2018) 22:31–44. doi: 10.1080/14728222.2018.1409724
20. Qin, LJ, Gu, YT, Zhang, H, and Xue, YX. Bradykinin-induced blood-tumor barrier opening is mediated by tumor necrosis factor-alpha. Neurosci Lett. (2009) 450:172–5. doi: 10.1016/j.neulet.2008.10.080
21. Morbidelli, L, Parenti, A, Giovannelli, L, Granger, HJ, Ledda, F, and Ziche, M. B1 receptor involvement in the effect of bradykinin on venular endothelial cell proliferation and potentiation of FGF-2 effects. Br J Pharmacol. (1998) 124:1286–92. doi: 10.1038/sj.bjp.0701943
22. Lee, CH, Shieh, DC, Tzeng, CY, Chen, CP, Wang, SP, Chiu, YC, et al. Bradykinininduced IL-6 expression through bradykinin B2 receptor, phospholipase C, protein kinase Cdelta and NF-kappaB pathway in human synovial fibroblasts. Mol Immunol. (2008) 45:3693–702. doi: 10.1016/j.molimm.2008.06.007
23. Nagashima, S, Dutra, AA, Arantes, MP, Zeni, RC, Klein, CK, de Oliveira, FC, et al. COVID-19 and lung mast cells: the kallikrein-kinin activation pathway. Int J Mol Sci. (2022) 23:1714. doi: 10.3390/ijms23031714
24. Schmaier, AH. The contact activation and kallikrein/kinin systems: pathophysiologic and physiologic activities. J Thromb Haemost. (2016) 14:28–39. doi: 10.1111/jth.13194
25. Bracke, A, De Hert, E, De Bruyn, M, Claesen, K, Vliegen, G, Vujkovic, A, et al. Proline-specific peptidase activities (DPP4, PRCP, FAP and PREP) in plasma of hospitalized COVID-19 patients. Clin Chim Acta. (2022) 531:4–11. doi: 10.1016/j.cca.2022.03.005
26. Imamura, T, Tanase, S, Hayashi, I, Potempa, J, Kozik, A, and Travis, J. Release of a new vascular permeability enhancing peptide from kininogens by human neutrophil elastase. Biochem Biophys Res Commun. (2002) 294:423–8. doi: 10.1016/S0006-291X(02)00490-4
27. Coffman, LG, Brown, JC, Johnson, DA, Parthasarathy, N, D'Agostino, RB, Lively, MO, et al. Cleavage of high-molecular-weight kininogen by elastase and tryptase is inhibited by ferritin. Am J Physiol Lung Cell Mol Physiol. (2008) 294:L505–15. doi: 10.1152/ajplung.00347.2007
28. Henry, BM, de Oliveira, MHS, Benoit, S, Plebani, M, and Lippi, G. Hematologic, biochemical and immune biomarker abnormalities associated with severe illness and mortality in coronavirus disease 2019 (COVID-19): a meta-analysis. Clin Chem Lab Med. (2020) 58:1021–8. doi: 10.1515/cclm-2020-0369
29. Ugonotti, J, Chatterjee, S, and Thaysen-Andersen, M. Structural and functional diversity of neutrophil glycosylation in innate immunity and related disorders. Mol Asp Med. (2021) 79:100882. doi: 10.1016/j.mam.2020.100882
30. Kahn, R, Hellmark, T, Leeb-Lundberg, LM, Akbari, N, Todiras, M, Olofsson, T, et al. Neutrophil-derived proteinase 3 induces kallikrein-independent release of a novel vasoactive kinin. J Immunol. (2009) 182:7906–15. doi: 10.4049/jimmunol.0803624
31. Huang, W, Li, M, Luo, G, Wu, X, Su, B, Zhao, L, et al. The inflammatory factors associated with disease severity to predict COVID-19 progression. J Immunol. (2021) 206:1597–608. doi: 10.4049/jimmunol.2001327
32. Akgun, E, Tuzuner, MB, Sahin, B, Kilercik, M, Kulah, C, Cakiroglu, HN, et al. Proteins associated with neutrophil degranulation are upregulated in nasopharyngeal swabs from SARS-CoV-2 patients. PLoS One. (2020) 15:e0240012. doi: 10.1371/journal.pone.0240012
33. Ng, H, Havervall, S, Rosell, A, Aguilera, K, Parv, K, von Meijenfeldt, FA, et al. Circulating markers of neutrophil extracellular traps are of prognostic value in patients with COVID-19. Arterioscler Thromb Vasc Biol. (2021) 41:988–94. doi: 10.1161/ATVBAHA.120.315267
34. Wygrecka, M, Birnhuber, A, Seeliger, B, Michalick, L, Pak, O, Schultz, AS, et al. Altered fibrin clot structure and dysregulated fibrinolysis contribute to thrombosis risk in severe COVID-19. Blood Adv. (2022) 6:1074–87. doi: 10.1182/bloodadvances.2021004816
35. Lipcsey, M, Persson, B, Eriksson, O, Blom, AM, Fromell, K, Hultström, M, et al. The outcome of critically ill COVID-19 patients is linked to Thromboinflammation dominated by the kallikrein/kinin system. Front Immunol. (2021) 12:627579. doi: 10.3389/fimmu.2021.627579
36. Englert, H, Rangaswamy, C, Deppermann, C, Sperhake, JP, Krisp, C, Schreier, D, et al. Defective NET clearance contributes to sustained FXII activation in COVID-19-associated pulmonary thrombo-inflammation. EBioMedicine. (2021) 67:103382. doi: 10.1016/j.ebiom.2021.103382
37. Ceballos, FC, Ryan, P, Blancas, R, Martin-Vicente, M, Vidal-Alcántara, EJ, Peréz-García, F, et al. Are reduced levels of coagulation proteins upon admission linked to COVID-19 severity and mortality? Front Med (Lausanne). (2021) 8:718053. doi: 10.3389/fmed.2021.718053
38. Collins, PW, Macchiavello, LI, Lewis, SJ, Macartney, NJ, Saayman, AG, Luddington, R, et al. Global tests of haemostasis in critically ill patients with severe sepsis syndrome compared to controls. Br J Haematol. (2006) 135:220–7. doi: 10.1111/j.1365-2141.2006.06281.x
39. Akwii, RG, Sajib, MS, Zahra, FT, and Mikelis, CM. Role of Angiopoietin-2 in vascular physiology and pathophysiology. Cells. (2019) 8:471. doi: 10.3390/cells8050471
40. Mancini, I, Baronciani, L, Artoni, A, Colpani, P, Biganzoli, M, Cozzi, G, et al. The ADAMTS13-von Willebrand factor axis in COVID-19 patients. J Thromb Haemost. (2021) 19:513–21. doi: 10.1111/jth.15191
41. Delrue, M, Siguret, V, Neuwirth, M, Joly, B, Beranger, N, Sène, D, et al. von Willebrand factor/ADAMTS13 axis and venous thromboembolism in moderate-to-severe COVID-19 patients. Br J Haematol. (2021) 192:1097–100. doi: 10.1111/bjh.17216
42. Leisman, DE, Mehta, A, Thompson, BT, Charland, NC, Gonye, ALK, Gushterova, I, et al. Alveolar, endothelial, and organ injury marker dynamics in severe COVID-19. Am J Respir Crit Care Med. (2022) 205:507–19. doi: 10.1164/rccm.202106-1514OC
43. Willems, LH, Nagy, M, Ten Cate, H, Spronk, HMH, Groh, LA, Leentjens, J, et al. Sustained inflammation, coagulation activation and elevated endothelin-1 levels without macrovascular dysfunction at 3 months after COVID-19. Thromb Res. (2022) 209:106–14. doi: 10.1016/j.thromres.2021.11.027
44. Philippe, A, Chocron, R, Gendron, N, Bory, O, Beauvais, A, Peron, N, et al. Circulating Von Willebrand factor and high molecular weight multimers as markers of endothelial injury predict COVID-19 in-hospital mortality. Angiogenesis. (2021) 24:505–17. doi: 10.1007/s10456-020-09762-6
45. Karampini, E, Fogarty, H, Elliott, S, Morrin, H, Bergin, C, O'Sullivan, JM, et al. Endothelial cell activation, Weibel-Palade body secretion, and enhanced angiogenesis in severe COVID-19. Res Pract Thromb Haemost. (2023) 7:100085. doi: 10.1016/j.rpth.2023.100085
46. Goshua, G, Pine, AB, Meizlish, ML, Chang, CH, Zhang, H, Bahel, P, et al. Endotheliopathy in COVID-19-associated coagulopathy: evidence from a single-Centre, cross-sectional study. Lancet Haematol. (2020) 7:e575–82. doi: 10.1016/S2352-3026(20)30216-7
47. Agrati, C, Bordoni, V, Sacchi, A, Petrosillo, N, Nicastri, E, Del Nonno, F, et al. Elevated P-selectin in severe Covid-19: considerations for therapeutic options. Mediterr J Hematol Infect Dis. (2021) 13:e2021016. doi: 10.4084/mjhid.2021.016
48. Karsli, E, Sabirli, R, Altintas, E, Canacik, O, Sabirli, GT, Kaymaz, B, et al. Soluble P-selectin as a potential diagnostic and prognostic biomarker for COVID-19 disease: a case-control study. Life Sci. (2021) 277:119634. doi: 10.1016/j.lfs.2021.119634
49. Smadja, DM, Guerin, CL, Chocron, R, Yatim, N, Boussier, J, Gendron, N, et al. Angiopoietin-2 as a marker of endothelial activation is a good predictor factor for intensive care unit admission of COVID-19 patients. Angiogenesis. (2020) 23:611–20. doi: 10.1007/s10456-020-09730-0
50. Sinkovits, G, Réti, M, Müller, V, Iványi, Z, Gál, J, Gopcsa, L, et al. Associations between the von Willebrand factor-ADAMTS13 Axis, complement activation, and COVID-19 severity and mortality. Thromb Haemost. (2022) 122:240–56. doi: 10.1055/s-0041-1740182
51. Ward, SE, Curley, GF, Lavin, M, Fogarty, H, Karampini, E, McEvoy, NL, et al. Irish COVID-19 vasculopathy study (ICVS) investigators. Von Willebrand factor propeptide in severe coronavirus disease 2019 (COVID-19): evidence of acute and sustained endothelial cell activation. Br J Haematol. (2021) 192:714–9. doi: 10.1111/bjh.17273
52. Cugno, M, Meroni, PL, Gualtierotti, R, Griffini, S, Grovetti, E, Torri, A, et al. Complement activation and endothelial perturbation parallel COVID-19 severity and activity. J Autoimmun. (2021) 116:102560. doi: 10.1016/j.jaut.2020.102560
53. Sibila, O, Perea, L, Albacar, N, Moisés, J, Cruz, T, Mendoza, N, et al. Elevated plasma levels of epithelial and endothelial cell markers in COVID-19 survivors with reduced lung diffusing capacity six months after hospital discharge. Respir Res. (2022) 23:37. doi: 10.1186/s12931-022-01955-5
54. Barabutis, N. Unfolded protein response in the COVID-19 context. Aging Health Res. (2021) 1:100001. doi: 10.1016/j.ahr.2020.100001
55. Lip, GY, Edmunds, E, Hee, FL, Blann, AD, and Beevers, DG. A cross-sectional, diurnal, and follow-up study of platelet activation and endothelial dysfunction in malignant phase hypertension. Am J Hypertens. (2001) 14:823–8. doi: 10.1016/s0895-7061(01)02045-3
56. Petrák, O, Widimský, J Jr, Zelinka, T, Kvasnicka, J, Strauch, B, Holaj, R, et al. Biochemical markers of endothelial dysfunction in patients with endocrine and essential hypertension. Physiol Res. (2006) 55:597–602. doi: 10.33549/physiolres.930912
57. Spencer, CG, Gurney, D, Blann, AD, Beevers, DG, and Lip, GY. ASCOT steering committee, Anglo-Scandinavian cardiac outcomes trial. Von Willebrand factor, soluble P-selectin, and target organ damage in hypertension: a substudy of the Anglo-Scandinavian cardiac outcomes trial (ASCOT). Hypertension. (2002) 40:61–6. doi: 10.1161/01.hyp.0000022061.12297.2e
58. de La Sierra, A, Larrousse, M, Oliveras, A, Armario, P, Hernández-Del Rey, R, Poch, E, et al. Abnormalities of vascular function in resistant hypertension. Blood Press. (2012) 21:104–9. doi: 10.3109/08037051.2011.622983
59. Schumacher, A, Seljeflot, I, Sommervoll, L, Christensen, B, Otterstad, JE, and Arnesen, H. Increased levels of markers of vascular inflammation in patients with coronary heart disease. Scand J Clin Lab Invest. (2002) 62:59–68. doi: 10.1080/003655102753517217
60. Mulhem, A, Moulla, Y, Klöting, N, Ebert, T, Tönjes, A, Fasshauer, M, et al. Circulating cell adhesion molecules in metabolically healthy obesity. Int J Obes. (2021) 45:331–6. doi: 10.1038/s41366-020-00667-4
61. Meigs, JB, O'donnell, CJ, Tofler, GH, Benjamin, EJ, Fox, CS, Lipinska, I, et al. Hemostatic markers of endothelial dysfunction and risk of incident type 2 diabetes: the Framingham offspring study. Diabetes. (2006) 55:530–7. doi: 10.2337/diabetes.55.02.06.db05-1041
62. Meigs, JB, Hu, FB, Rifai, N, and Manson, JE. Biomarkers of endothelial dysfunction and risk of type 2 diabetes mellitus. JAMA. (2004) 291:1978–86. doi: 10.1001/jama.291.16.1978
63. Hegazy, GA, Awan, Z, Hashem, E, Al-Ama, N, and Abunaji, AB. Levels of soluble cell adhesion molecules in type 2 diabetes mellitus patients with macrovascular complications. J Int Med Res. (2020) 48:030006051989385. doi: 10.1177/0300060519893858
64. Wang, M, Lin, EP, Huang, LC, Li, CY, Shyr, Y, and Lai, CH. Mortality of cardiovascular events in patients with COPD and preceding hospitalization for acute exacerbation. Chest. (2020) 158:973–85. doi: 10.1016/j.chest.2020.02.046
65. Van der Vorm, LN, Li, L, Huskens, D, Hulstein, JJJ, Roest, M, de Groot, PG, et al. Acute exacerbations of COPD are associated with a prothrombotic state through platelet-monocyte complexes, endothelial activation and increased thrombin generation. Respir Med. (2020) 171:106094. doi: 10.1016/j.rmed.2020.106094
66. Polatli, M, Cakir, A, Cildag, O, Bolaman, AZ, Yenisey, C, and Yenicerioglu, Y. Microalbuminuria, von Willebrand factor and fibrinogen levels as markers of the severity in COPD exacerbation. J Thromb Thrombolysis. (2008) 26:97–102. doi: 10.1007/s11239-007-0073-1
67. Ackermann, M, Verleden, SE, Kuehnel, M, Haverich, A, Welte, T, Laenger, F, et al. Pulmonary vascular Endothelialitis, thrombosis, and angiogenesis in Covid-19. N Engl J Med. (2020) 383:120–8. doi: 10.1056/NEJMoa2015432
68. Tian, S, Hu, W, Niu, L, Liu, H, Xu, H, and Xiao, SY. Pulmonary pathology of early-phase 2019 novel coronavirus (COVID-19) pneumonia in two patients with lung cancer. J Thorac Oncol. (2020) 15:700–4. doi: 10.1016/j.jtho.2020.02.010
69. Pernazza, A, Mancini, M, Rullo, E, Bassi, M, De Giacomo, T, Rocca, CD, et al. Early histologic findings of pulmonary SARS-CoV-2 infection detected in a surgical specimen. Virchows Arch. (2020) 477:743–8. doi: 10.1007/s00428-020-02829-1
70. Tabary, M, Khanmohammadi, S, Araghi, F, Dadkhahfar, S, and Tavangar, SM. Pathologic features of COVID-19: a concise review. Pathol Res Pract. (2020) 216:153097. doi: 10.1016/j.prp.2020.153097
71. Mikhaleva, LM, Cherniaev, AL, Samsonova, MV, Zayratyants, OV, Kakturskiy, LV, Vasyukova, OA, et al. Pathological features in 100 deceased patients with COVID-19 in correlation with clinical and laboratory data. Pathol Oncol Res. (2021) 27:1609900. doi: 10.3389/pore.2021.1609900
72. Matschke, J, Lütgehetmann, M, Hagel, C, Sperhake, JP, Schröder, AS, Edler, C, et al. Neuropathology of patients with COVID-19 in Germany: a post-mortem case series. Lancet Neurol. (2020) 19:919–29. doi: 10.1016/S1474-4422(20)30308-2
73. Varga, Z, Flammer, AJ, Steiger, P, Haberecker, M, Andermatt, R, Zinkernagel, AS, et al. Endothelial cell infection and endotheliitis in COVID-19. Lancet. (2020) 395:1417–8. doi: 10.1016/S0140-6736(20)30937-5
74. Leung, JM, Yang, CX, Tam, A, Shaipanich, T, Hackett, TL, Singhera, GK, et al. ACE-2 expression in the small airway epithelia of smokers and COPD patients: implications for COVID-19. Eur Respir J. (2020) 55:2000688. doi: 10.1183/13993003.00688-2020
75. Hamming, I, Timens, W, Bulthuis, ML, Lely, AT, Navis, G, and van Goor, H. Tissue distribution of ACE2 protein, the functional receptor for SARS coronavirus. A first step in understanding SARS pathogenesis. J Pathol. (2004) 203:631–7. doi: 10.1002/path.1570
76. McCracken, IR, Saginc, G, He, L, Huseynov, A, Daniels, A, Fletcher, S, et al. Lack of evidence of angiotensin-converting enzyme 2 expression and replicative infection by SARS-CoV-2 in human endothelial cells. Circulation. (2021) 143:865–8. doi: 10.1161/CIRCULATIONAHA.120.052824
77. Li, MY, Li, L, Zhang, Y, and Wang, XS. Expression of the SARS-CoV-2 cell receptor gene ACE2 in a wide variety of human tissues. Infect Dis Poverty. (2020) 9:45. doi: 10.1186/s40249-020-00662-x
78. Bordoni, V, Mariotti, D, Matusali, G, Colavita, F, Cimini, E, Ippolito, G, et al. SARS-CoV-2 infection of airway epithelium triggers pulmonary endothelial cell activation and senescence associated with type I IFN production. Cells. (2022) 11:2912. doi: 10.3390/cells11182912
79. Passos-Silva, DG, Verano-Braga, T, and Santos, RA. Angiotensin-(1-7): beyond the cardio-renal actions. Clin Sci (Lond). (2013) 124:443–56. doi: 10.1042/CS20120461
80. Imai, Y, Kuba, K, Rao, S, Huan, Y, Guo, F, Guan, B, et al. Angiotensin-converting enzyme 2 protects from severe acute lung failure. Nature. (2005) 436:112–6. doi: 10.1038/nature03712
81. Gan, R, Rosoman, NP, Henshaw, DJE, Noble, EP, Georgius, P, and Sommerfeld, N. COVID-19 as a viral functional ACE2 deficiency disorder with ACE2 related multi-organ disease. Med Hypotheses. (2020) 144:110024. doi: 10.1016/j.mehy.2020.110024
82. Li, LQ, Huang, T, Wang, YQ, Wang, ZP, Liang, Y, Huang, TB, et al. COVID-19 patients' clinical characteristics, discharge rate, and fatality rate of meta-analysis. J Med Virol. (2020) 92:577–83. doi: 10.1002/jmv.25757
83. Lim, AYH, Goh, JL, Chua, MCW, Heng, BH, Abisheganaden, JA, and George, PP. Temporal changes of haematological and radiological findings of the COVID-19 infection-a review of literature. BMC Pulm Med. (2021) 21:37. doi: 10.1186/s12890-020-01389-z
84. Chen, R, Sang, L, Jiang, M, Yang, Z, Jia, N, Fu, W, et al. Medical treatment expert group for COVID-19. Longitudinal hematologic and immunologic variations associated with the progression of COVID-19 patients in China. J Allergy Clin Immunol. (2020) 146:89–100. doi: 10.1016/j.jaci.2020.05.003
85. Lanini, S, Montaldo, C, Nicastri, E, Vairo, F, Agrati, C, Petrosillo, N, et al. COVID-19 disease-temporal analyses of complete blood count parameters over course of illness, and relationship to patient demographics and management outcomes in survivors and non-survivors: a longitudinal descriptive cohort study. PLoS One. (2020) 15:e0244129. doi: 10.1371/journal.pone.0244129
86. Yan, X, Li, F, Wang, X, Yan, J, Zhu, F, Tang, S, et al. Neutrophil to lymphocyte ratio as prognostic and predictive factor in patients with coronavirus disease 2019: a retrospective cross-sectional study. J Med Virol. (2020) 92:2573–81. doi: 10.1002/jmv.26061
87. Zuo, Y, Yalavarthi, S, Shi, H, Gockman, K, Zuo, M, Madison, JA, et al. Neutrophil extracellular traps in COVID-19. JCI Insight. (2020) 5:e138999. doi: 10.1172/jci.insight.138999
88. Veras, FP, Pontelli, MC, Silva, CM, Toller-Kawahisa, JE, de Lima, M, Nascimento, DC, et al. SARS-CoV-2-triggered neutrophil extracellular traps mediate COVID-19 pathology. J Exp Med. (2020) 217:e20201129. doi: 10.1084/jem.20201129
89. Guan, WJ, Ni, ZY, Hu, Y, Liang, WH, Ou, CQ, He, JX, et al. China medical treatment expert Group for Covid-19. Clinical characteristics of coronavirus disease 2019 in China. N Engl J Med. (2020) 382:1708–20. doi: 10.1056/NEJMoa2002032
90. Linssen, J, Ermens, A, Berrevoets, M, Seghezzi, M, Previtali, G, van der Sar-van der Brugge, S, et al. A novel haemocytometric COVID-19 prognostic score developed and validated in an observational multicentre European hospital-based study. Elife. (2020) 9:e63195. doi: 10.7554/eLife.63195
91. Burke, H, Freeman, A, O'Regan, P, Wysocki, O, Freitas, A, Dushianthan, A, et al. REACT COVID group. Biomarker identification using dynamic time warping analysis: a longitudinal cohort study of patients with COVID-19 in a UK tertiary hospital. BMJ Open. (2022) 12:e050331. doi: 10.1136/bmjopen-2021-050331
92. Wong, RS, Wu, A, To, KF, Lee, N, Lam, CW, Wong, CK, et al. Haematological manifestations in patients with severe acute respiratory syndrome: retrospective analysis. BMJ. (2003) 326:1358–62. doi: 10.1136/bmj.326.7403.1358
93. Philippe, A, Chocron, R, Bonnet, G, Yatim, N, Sutter, W, Hadjadj, J, et al. Platelet activation and coronavirus disease 2019 mortality: insights from coagulopathy, antiplatelet therapy and inflammation. Arch Cardiovasc Dis. (2023) 116:183–91. doi: 10.1016/j.acvd.2023.01.006
94. Recovery Collaborative Group. Aspirin in patients admitted to hospital with COVID-19 (RECOVERY): a randomised, controlled, open-label, platform trial. Lancet. (2022) 399:143–51. doi: 10.1016/S0140-6736(21)01825-0
95. Sisinni, A, Rossi, L, Battista, A, Poletti, E, Battista, F, Battista, RA, et al. Pre-admission acetylsalicylic acid therapy and impact on in-hospital outcome in COVID-19 patients: the ASA-CARE study. Int J Cardiol. (2021) 344:240–5. doi: 10.1016/j.ijcard.2021.09.058
96. Chow, JH, Yin, Y, Yamane, DP, Davison, D, Keneally, RJ, Hawkins, K, et al. Association of prehospital antiplatelet therapy with survival in patients hospitalized with COVID-19: a propensity score-matched analysis. J Thromb Haemost. (2021) 19:2814–24. doi: 10.1111/jth.15517
97. Wang, M, Zhang, J, Ye, D, Wang, Z, Liu, J, He, H, et al. Time-dependent changes in the clinical characteristics and prognosis of hospitalized COVID-19 patients in Wuhan, China: a retrospective study. Clin Chim Acta. (2020) 510:220–7. doi: 10.1016/j.cca.2020.06.051
98. Lu, H, Chen, M, Tang, S, and Yu, W. Association of coagulation disturbances with severity of COVID-19: a longitudinal study. Hematology. (2021) 26:656–62. doi: 10.1080/16078454.2021.1968648
99. Xu, J, Zhang, Y, Li, Y, Liao, K, Zeng, X, Zeng, X, et al. Dynamic changes in coagulation function in patients with pneumonia under admission and non-admission treatment. Front Med (Lausanne). (2021) 8:626384. doi: 10.3389/fmed.2021.626384
100. Xiang, G, Hao, S, Fu, C, Hu, W, Xie, L, Wu, Q, et al. The effect of coagulation factors in 2019 novel coronavirus patients: a systematic review and meta-analysis. Medicine (Baltimore). (2021) 100:e24537. doi: 10.1097/MD.0000000000024537
101. Smadja, DM, Bory, OM, Diehl, JL, Mareau, A, Gendron, N, Jannot, AS, et al. Daily monitoring of D-dimer allows outcomes prediction in COVID-19. TH Open. (2021) 6:e21–5. doi: 10.1055/a-1709-5441
102. Smadja, DM, Gendron, N, Philippe, A, Diehl, JL, Ochat, N, Bory, O, et al. Fibrin monomers evaluation during hospitalization for COVID-19 is a predictive marker of in-hospital mortality. Front Cardiovasc Med. (2023) 10:1001530. doi: 10.3389/fcvm.2023.1001530
103. Liu, Y, Tan, W, Chen, H, Zhu, Y, Wan, L, Jiang, K, et al. Dynamic changes in lymphocyte subsets and parallel cytokine levels in patients with severe and critical COVID-19. BMC Infect Dis. (2021) 21:79. doi: 10.1186/s12879-021-05792-7
104. Han, H, Ma, Q, Li, C, Liu, R, Zhao, L, Wang, W, et al. Profiling serum cytokines in COVID-19 patients reveals IL-6 and IL-10 are disease severity predictors. Emerg Microbes Infect. (2020) 9:1123–30. doi: 10.1080/22221751.2020.1770129
105. Del Valle, DM, Kim-Schulze, S, Huang, HH, Beckmann, ND, Nirenberg, S, Wang, B, et al. An inflammatory cytokine signature predicts COVID-19 severity and survival. Nat Med. (2020) 26:1636–43. doi: 10.1038/s41591-020-1051-9
106. Liu, Z, Li, J, Chen, D, Gao, R, Zeng, W, Chen, S, et al. Dynamic Interleukin-6 level changes as a prognostic indicator in patients with COVID-19. Front Pharmacol. (2020) 11:1093. doi: 10.3389/fphar.2020.01093
107. Sakthivadivel, V, Bohra, GK, Maithilikarpagaselvi, N, Khichar, S, Meena, M, Palanisamy, N, et al. Association of Inflammatory Markers with COVID-19 outcome among hospitalized patients: experience from a tertiary healthcare Center in Western India. Maedica (Bucur). (2021) 16:620–7. doi: 10.26574/maedica.2021.16.4.620
108. Santa Cruz, A, Mendes-Frias, A, Oliveira, AI, Dias, L, Matos, AR, Carvalho, A, et al. Interleukin-6 is a biomarker for the development of fatal severe acute respiratory syndrome coronavirus 2 pneumonia. Front Immunol. (2021) 12:613422. doi: 10.3389/fimmu.2021.613422
109. Tsikala Vafea, M, Atalla, E, Kalligeros, M, Mylona, EK, Shehadeh, F, and Mylonakis, E. Chest CT findings in asymptomatic cases with COVID-19: a systematic review and meta-analysis. Clin Radiol. (2020) 75:876.e33–9. doi: 10.1016/j.crad.2020.07.025
110. Larici, AR, Cicchetti, G, Marano, R, Bonomo, L, and Storto, ML. COVID-19 pneumonia: current evidence of chest imaging features, evolution and prognosis. Chin J Acad Radiol. (2021) 4:229–40. doi: 10.1007/s42058-021-00068-0
111. Bhatraju, PK, Ghassemieh, BJ, Nichols, M, Kim, R, Jerome, KR, Nalla, AK, et al. Covid-19 in critically ill patients in the Seattle region - case series. N Engl J Med. (2020) 382:2012–22. doi: 10.1056/NEJMoa2004500
112. Fang, X, Li, S, Yu, H, Wang, P, Zhang, Y, Chen, Z, et al. Epidemiological, comorbidity factors with severity and prognosis of COVID-19: a systematic review and meta-analysis. Aging (Albany NY). (2020) 12:12493–503. doi: 10.18632/aging.103579
113. Roncon, L, Zuin, M, Barco, S, Valerio, L, Zuliani, G, Zonzin, P, et al. Incidence of acute pulmonary embolism in COVID-19 patients: systematic review and meta-analysis. Eur J Intern Med. (2020) 82:29–37. doi: 10.1016/j.ejim.2020.09.006
114. Ackermann, M, Kamp, JC, Werlein, C, Walsh, CL, Stark, H, Prade, V, et al. The fatal trajectory of pulmonary COVID-19 is driven by lobular ischemia and fibrotic remodelling. EBioMedicine. (2022) 85:104296. doi: 10.1016/j.ebiom.2022.104296
115. Caruso, D, Zerunian, M, Polici, M, Pucciarelli, F, Polidori, T, Rucci, C, et al. Chest CT features of COVID-19 in Rome, Italy. Radiology. (2020) 296:E79–85. doi: 10.1148/radiol.2020201237
116. Bianco, A, Valente, T, Perrotta, F, Stellato, E, Brunese, L, Wood, BJ, et al. Study investigators. Remarkable vessel enlargement within lung consolidation in COVID-19 compared to AH1N1 pneumonia: a retrospective study in Italy. Heliyon. (2021) 7:e07112. doi: 10.1016/j.heliyon.2021.e07112
117. Poletti, J, Bach, M, Yang, S, Sexauer, R, Stieltjes, B, Rotzinger, DC, et al. Automated lung vessel segmentation reveals blood vessel volume redistribution in viral pneumonia. Eur J Radiol. (2022) 150:110259. doi: 10.1016/j.ejrad.2022.110259
118. Sundler Björkman, L, Persson, B, Aronsson, D, Skattum, L, Nordenfelt, P, and Egesten, A. Comorbidities in hereditary angioedema-a population-based cohort study. Clin Transl Allergy. (2022) 12:e12135. doi: 10.1002/clt2.12135
119. Grover, SP, Kawano, T, Wan, J, Tanratana, P, Polai, Z, Shim, YJ, et al. C1 inhibitor deficiency enhances contact pathway-mediated activation of coagulation and venous thrombosis. Blood. (2023) 141:2390–401. doi: 10.1182/blood.2022018849
120. Charitos, P, Heijnen, IAFM, Egli, A, Bassetti, S, Trendelenburg, M, and Osthoff, M. Functional activity of the complement system in hospitalized COVID-19 patients: a prospective cohort study. Front Immunol. (2021) 12:765330. doi: 10.3389/fimmu.2021.765330
121. Urwyler, P, Moser, S, Charitos, P, Heijnen, IAFM, Rudin, M, Sommer, G, et al. Treatment of COVID-19 with Conestat alfa, a regulator of the complement, contact activation and kallikrein-kinin system. Front Immunol. (2020) 11:2072. doi: 10.3389/fimmu.2020.02072
122. Medjeral-Thomas, NR, Troldborg, A, Hansen, AG, Pihl, R, Clarke, CL, Peters, JE, et al. Protease inhibitor plasma concentrations associate with COVID-19 infection. Oxf Open Immunol. (2021) 2:iqab014. doi: 10.1093/oxfimm/iqab014
123. Weidmann, H, Heikaus, L, Long, AT, Naudin, C, Schlüter, H, and Renné, T. The plasma contact system, a protease cascade at the nexus of inflammation, coagulation and immunity. Biochim Biophys Acta Mol Cell Res. (2017) 1864:2118–27. doi: 10.1016/j.bbamcr.2017.07.009
124. Joshi, D, Manohar, S, Goel, G, Saigal, S, Pakhare, AP, and Goyal, A. Adequate antithrombin III level predicts survival in severe COVID-19 pneumonia. Cureus. (2021) 13:e18538. doi: 10.7759/cureus.18538
125. Gazzaruso, C, Paolozzi, E, Valenti, C, Brocchetta, M, Naldani, D, Grignani, C, et al. Association between antithrombin and mortality in patients with COVID-19. A possible link with obesity. Nutr Metab Cardiovasc Dis. (2020) 30:1914–9. doi: 10.1016/j.numecd.2020.07.040
126. Anaklı, İ, Ergin Özcan, P, Polat, Ö, Orhun, G, Alay, GH, Tuna, V, et al. Prognostic value of antithrombin levels in COVID-19 patients and impact of fresh frozen plasma treatment: a retrospective study. Turk J Haematol. (2021) 38:15–21. doi: 10.4274/tjh.galenos.2021.2020.0695
127. Schrick, D, Tőkés-Füzesi, M, Réger, B, and Molnár, T. Plasma fibrinogen independently predicts hypofibrinolysis in severe COVID-19. Meta. (2021) 11:826. doi: 10.3390/metabo11120826
128. Hammer, S, Häberle, H, Schlensak, C, Bitzer, M, Malek, NP, Handgretinger, R, et al. Severe SARS-CoV-2 infection inhibits fibrinolysis leading to changes in viscoelastic properties of blood clot: a descriptive study of fibrinolysis in COVID-19. Thromb Haemost. (2021) 121:1417–26. doi: 10.1055/a-1400-6034
129. de Laat-Kremers, R, De Jongh, R, Ninivaggi, M, Fiolet, A, Fijnheer, R, Remijn, J, et al. Coagulation parameters predict COVID-19-related thrombosis in a neural network with a positive predictive value of 98. Front Immunol. (2022) 13:977443. doi: 10.3389/fimmu.2022.977443
130. Mansour, E, Palma, AC, Ulaf, RG, Ribeiro, LC, Bernardes, AF, Nunes, TA, et al. Safety and outcomes associated with the pharmacological inhibition of the kinin-kallikrein system in severe COVID-19. Viruses. (2021) 13:309. doi: 10.3390/v13020309
131. Banerji, A, Riedl, MA, Bernstein, JA, Cicardi, M, Longhurst, HJ, Zuraw, BL, et al. HELP investigators. Effect of Lanadelumab compared with placebo on prevention of hereditary angioedema attacks: a randomized clinical trial. JAMA. (2018) 320:2108–21. doi: 10.1001/jama.2018.16773
132. Zuraw, B, Lumry, WR, Johnston, DT, Aygören-Pürsün, E, Banerji, A, Bernstein, JA, et al. Oral once-daily berotralstat for the prevention of hereditary angioedema attacks: a randomized, double-blind, placebo-controlled phase 3 trial. J Allergy Clin Immunol. (2021) 148:164–172.e9. doi: 10.1016/j.jaci.2020.10.015
133. Levy, RJ, Lumry, WR, McNeil, DL, Li, HH, Campion, M, Horn, PT, et al. EDEMA4: a phase 3, double-blind study of subcutaneous ecallantide treatment for acute attacks of hereditary angioedema. Ann Allergy Asthma Immunol. (2010) 104:523–9. doi: 10.1016/j.anai.2010.04.012
134. Fijen, LM, Riedl, MA, Bordone, L, Bernstein, JA, Raasch, J, Tachdjian, R, et al. Inhibition of Prekallikrein for hereditary angioedema. N Engl J Med. (2022) 386:1026–33. doi: 10.1056/NEJMoa2109329
135. McKenzie, A, Roberts, A, Malandkar, S, Feuersenger, H, Panousis, C, and Pawaskar, D. A phase I, first-in-human, randomized dose-escalation study of anti-activated factor XII monoclonal antibody garadacimab. Clin Transl Sci. (2022) 15:626–37. doi: 10.1111/cts.13180
136. Lumry, WR, Farkas, H, Moldovan, D, Toubi, E, Baptista, J, Craig, T, et al. Icatibant for multiple hereditary angioedema attacks across the controlled and open-label extension phases of FAST-3. Int Arch Allergy Immunol. (2015) 168:44–55. doi: 10.1159/000441060
137. Cicardi, M, Banerji, A, Bracho, F, Malbrán, A, Rosenkranz, B, Riedl, M, et al. Icatibant, a new bradykinin-receptor antagonist, in hereditary angioedema. N Engl J Med. (2010) 363:532–41. doi: 10.1056/NEJMoa0906393
138. Leach, JK, Spencer, K, Mascelli, M, and McCauley, TG. Pharmacokinetics of single and repeat doses of icatibant. Clin Pharmacol Drug Dev. (2015) 4:105–11. doi: 10.1002/cpdd.138
139. Pecori, D, Della Siega, P, Sozio, E, Barbano, E, Mazzoran, L, Zanichelli, A, et al. Icatibant in severe acute respiratory syndrome coronavirus 2 infection: a case report. J Investig Allergol Clin Immunol. (2021) 31:451–2. doi: 10.18176/jiaci.0659
140. Giol, J, Jacob, J, Llopis, F, Lleonart, R, Ruiz Esteve, F, and Malchair, P. Exceptional treatment of COVID-19 pneumonia with icatibant. Emergencias. (2022) 34:159–60.
141. Van de Veerdonk, FL, Kouijzer, IJE, de Nooijer, AH, van der Hoeven, HG, Maas, C, Netea, MG, et al. Outcomes associated with use of a kinin B2 receptor antagonist among patients with COVID-19. JAMA Netw Open. (2020) 3:e2017708. doi: 10.1001/jamanetworkopen.2020.17708
142. Malchair, P, Giol, J, García, V, Rodríguez, O, Ruibal, JC, Zarauza, A, et al. Three-day Icatibant on top of standard Care in Patients with Coronavirus Disease 2019 pneumonia: a randomized, open-label, phase 2, Proof-of-Concept. Trial Clin Infect Dis. (2023) 76:1784–92. doi: 10.1093/cid/ciac984
143. I-SPY COVID Consortium. Report of the first seven agents in the I-SPY COVID trial: a phase 2, open label, adaptive platform randomised controlled trial. EClinicalMedicine. (2023) 58:101889. doi: 10.1016/j.eclinm.2023.101889
144. Fein, AM, Bernard, GR, Criner, GJ, Fletcher, EC, Good, JT, Knaus, WA, et al. Treatment of severe systemic inflammatory response syndrome and sepsis with a novel bradykinin antagonist, deltibant (CP-0127). Results of a randomized, double-blind, placebo-controlled trial. CP-0127 SIRS and sepsis study group. JAMA. (1997) 277:482–7. doi: 10.1001/jama.1997.03540300050033
Keywords: bradykinin, inflammation, coagulation, endothelium, COVID-19
Citation: Bailey M, Linden D, Guo-Parke H, Earley O, Peto T, McAuley DF, Taggart C and Kidney J (2023) Vascular risk factors for COVID-19 ARDS: endothelium, contact-kinin system. Front. Med. 10:1208866. doi: 10.3389/fmed.2023.1208866
Received: 19 April 2023; Accepted: 05 June 2023;
Published: 28 June 2023.
Edited by:
Daniel O’Toole, University of Galway, IrelandReviewed by:
Nektarios Barabutis, University of Louisiana at Monroe, United StatesCopyright © 2023 Bailey, Linden, Guo-Parke, Earley, Peto, McAuley, Taggart and Kidney. This is an open-access article distributed under the terms of the Creative Commons Attribution License (CC BY). The use, distribution or reproduction in other forums is permitted, provided the original author(s) and the copyright owner(s) are credited and that the original publication in this journal is cited, in accordance with accepted academic practice. No use, distribution or reproduction is permitted which does not comply with these terms.
*Correspondence: Melanie Bailey, bWVsYW5pZS5iYWlsZXlAYmVsZmFzdHRydXN0LmhzY25pLm5ldA==
Disclaimer: All claims expressed in this article are solely those of the authors and do not necessarily represent those of their affiliated organizations, or those of the publisher, the editors and the reviewers. Any product that may be evaluated in this article or claim that may be made by its manufacturer is not guaranteed or endorsed by the publisher.
Research integrity at Frontiers
Learn more about the work of our research integrity team to safeguard the quality of each article we publish.