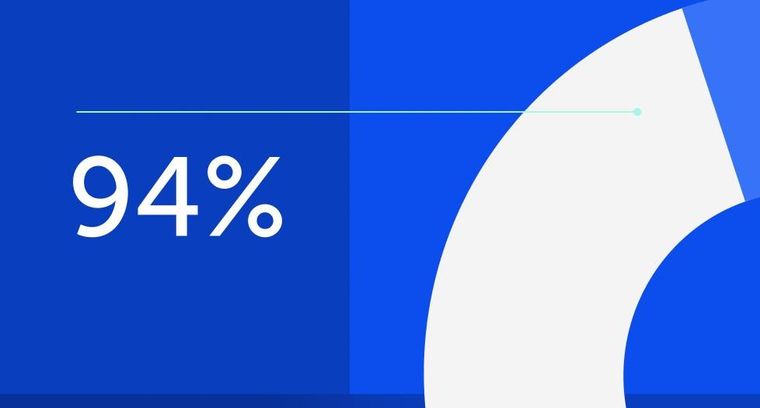
94% of researchers rate our articles as excellent or good
Learn more about the work of our research integrity team to safeguard the quality of each article we publish.
Find out more
REVIEW article
Front. Med., 22 August 2023
Sec. Precision Medicine
Volume 10 - 2023 | https://doi.org/10.3389/fmed.2023.1206071
This article is part of the Research TopicExtending Precision Medicine to Cancer SurvivorshipView all 6 articles
With advancements in cancer treatment and supportive care, there is a growing population of childhood cancer survivors who experience a substantial burden of comorbidities related to having received cancer treatment at a young age. Despite an overall reduction in the incidence of most chronic health conditions in childhood cancer survivors over the past several decades, the cumulative incidence of certain late effects, in particular diabetes mellitus (DM), has increased. The implications are significant, because DM is a key risk factor for cardiovascular disease, a leading cause of premature death in childhood cancer survivors. The underlying pathophysiology of DM in cancer survivors is multifactorial. DM develops at younger ages in survivors compared to controls, which may reflect an “accelerated aging” phenotype in these individuals. The treatment-related exposures (i.e., chemotherapy, radiation) that increase risk for DM in childhood cancer survivors may be more than additive with established DM risk factors (e.g., older age, obesity, race, and ethnicity). Emerging research also points to parallels in cellular processes implicated in aging- and cancer treatment-related DM. Still, there remains marked inter-individual variability regarding risk of DM that is not explained by demographic and therapeutic risk factors alone. Recent studies have highlighted the role of germline genetic risk factors and epigenetic modifications that are associated with risk of DM in both the general and oncology populations. This review summarizes our current understanding of recognized risk factors for DM in childhood cancer survivors to help inform targeted approaches for disease screening, prevention, and treatment. Furthermore, it highlights the existing scientific gaps in understanding the relative contributions of individual therapeutic exposures and the mechanisms by which they exert their effects that uniquely predispose this population to DM following cancer treatment.
For most common pediatric malignancies, five-year survival rates exceed 90% (1); as a result, currently, there are an estimated 500,000 childhood cancer survivors (CCS) in the U.S. alone (2). Despite this success, the growing population of long-term CCS faces a significantly worse quality of health compared to the non-oncology population due to cancer diagnosis and treatment exposures during key developmental periods. Approximately 20 years after cancer diagnosis, more than 25% of CCS have a chronic health condition (e.g., musculoskeletal deficits, cardiovascular disease, endocrinopathies) (3, 4). While advances in treatment and supportive care approaches have reduced the overall burden over time, the risk for specific chronic health conditions such as diabetes mellitus (DM) has increased (3). Among 5-year survivors, the cumulative incidence of self-reported severe, life-threatening, or fatal DM 15 years after primary cancer diagnosis more than doubled in patients diagnosed in the 1990s compared to the 1970s (3). Similar to the general population, in addition to producing immediate adverse health outcomes, DM is also a critical risk factor for subsequent cardiovascular disease, which is a leading cause of death in CCS (5, 6). Compared to the general population, CCS are seven times more likely to die from cardiovascular disease and related morbidities, largely due to treatment exposures such as anthracycline chemotherapy and chest radiation (7). Survivors of adult-onset cancer with DM have a higher risk for cardiovascular disease than do their non-oncology counterparts with DM (8) Thus, prevention and timely management of DM not only leads to a decrease in indirect complications of DM, but also has the potential opportunity to mitigate premature mortality in CCS related to cardiovascular disease.
In addition to premature mortality, the financial burden from DM is substantial. The approximate cumulative cost of medical expenditure, missed work, and decreased productivity from DM in all individuals (i.e., oncology and non-oncology populations) is estimated at 300 billion dollars annually (9). Individuals with DM experience excess financial hardship, financial distress, cost-related medication nonadherence, and food insecurity (10). As CCS have a higher burden of chronic health conditions and spend more of their income on medical care compared to siblings (3, 11), they are particularly vulnerable to the burden of financial toxicity associated with late effects and conditions such as DM.
DM typically develops through progression from normal glucose regulation, to glucose intolerance (prediabetes), and ultimately to glucose dysregulation or overt DM. Each year, approximately 10% of the general population with recognized prediabetes progresses to DM; in contrast, those with prediabetes or insulin resistance who achieve reversion to normal glucose regulation/homeostasis through intervention demonstrate a 56% reduced risk of progression to DM (12). Therefore, early diagnosis and intervention during the prediabetic phase represent important potential opportunities to reduce progression to diabetes. Survivors of childhood cancer have unique risk factors leading to the development of DM. Different mechanistic pathways may be implicated depending on their individual treatment exposures. In this review, we summarize the current understanding of recognized risk factors for DM in CCS to help inform targeted approaches for disease screening, prevention, and treatment. Additionally, this review highlights the existing scientific gaps in understanding the relative contributions of individual therapeutic exposures and the mechanisms by which they exert their effects that uniquely predispose this population to DM following cancer treatment.
Prediabetes is characterized by insulin resistance and DM is characterized by hyperglycemia due to reduced insulin action and/or relative deficiency in pancreatic insulin secretion with or without decreased insulin sensitivity in target organs. In the general population, obesity coupled with insulin resistance is the strongest risk factor for developing DM; individuals with obesity have an approximately 7-fold risk of developing DM compared to those who are normal weight (13, 14). Other identified risk factors include decreased physical activity and race/ethnicity (Hispanic, Native American, Black) (14).
The inverse relationship between body mass index (BMI) and age at DM diagnosis is well recognized (15). Although BMI is a readily available indirect measure of obesity, it fails to account for age- and sex-specific differences in body composition and amounts of specific fat depots such as visceral vs. subcutaneous adipose tissue. Studies assessing body composition in CCS have shown an increased risk for DM independent of BMI (16–18). The increased visceral adiposity seen in survivors is strongly associated with metabolic abnormalities, including insulin resistance, inflammation, and adipokine-associated inflammation, likely compounding the existent risk for DM associated with obesity (18). Visceral adipose tissue (VAT) is the immediate storage site for diet-derived fat. It exhibits high lipid turnover and is the primary source of free fatty acid (FFA) release after an overnight fast (19). Most FFA delivered to the liver originates from visceral fat, particularly in individuals with more VAT, and this effect appears to be greater in women than in men (20, 21).
Sarcopenic obesity, characterized by the concurrent loss of skeletal muscle mass and increase in fat mass, specifically VAT, is an important late effect of cancer treatment that is associated with decreased insulin sensitivity (22–24). Excess adiposity leads to an imbalance in the production of adipocytokines, including an increase in pro-inflammatory cytokines and decrease in adiponectin which results in worsening dyslipidemia and insulin resistance (25). In individuals with obesity, adipocytes become resistant to insulin-stimulated inhibition of lipolysis. This results in increased FFA flux, inflammation, and lipid deposition in skeletal muscle and liver tissue (25). Skeletal muscle is responsible for a majority of the body’s insulin-stimulated glucose disposal, thus this lipid deposition negatively affects glucose uptake in peripheral tissues (25). Importantly, pediatric allogeneic hematopoietic cell transplantation (HCT) survivors have been found to have significantly higher fat mass, higher VAT and subcutaneous adipose tissue, and significantly lower muscle density and lean mass compared to a robust, healthy age-, sex-, and race-matched reference population. These body composition differences were present despite no significant differences in BMI Z-scores between HCT survivors and the reference population (17, 18). The body composition abnormalities further highlight the failure of BMI as a measure to adequately capture discrete disease effects on lean mass and fat mass in HCT survivors. Furthermore, similar body composition abnormalities with normal BMI have also been reported in a longitudinal cohort of adult survivors of allogeneic HCT (26). The observed alterations in body composition likely contribute to treatment-related morbidity and mortality associated with premature atherosclerotic cardiovascular disease, metabolic syndrome, and poor bone health.
Normal insulin signaling typically suppresses hepatic glucose production (27). However, hepatic insulin signaling becomes impaired due to accumulated triglyceride and FFA levels in the liver, leading to increased hepatic gluconeogenesis (15). Excess hepatic glucose production, together with impaired skeletal muscle glucose uptake, further exacerbates hyperglycemia (28). Additionally, FFAs stimulate hepatic inflammatory and pro-thrombotic states, characterized by endothelial activation, which increase cardiovascular risk (29, 30). Nutritional imbalance (e.g., excess fat and carbohydrate consumption relative to needs) can induce an increase in reactive oxygen species (ROS) burden and oxidative stress. Excess ROS in turn lead to increased inflammation and additional downstream effects including mitochondrial dysfunction and epigenetic changes which can persist even after restoration of normoglycemia (15, 31, 32).
Among individuals with insulin resistance, increased lipid deposition within muscle cells (intramyocellular lipids, IMCL) is associated with poor fatty acid oxidation, and decreased insulin sensitivity, independent of BMI (33–35). In oncology patients undergoing potentially curative surgery, higher IMCL is positively correlated with weight loss from pre-illness weight (36). Furthermore, a study of pediatric HCT survivors demonstrated higher fat infiltration of muscle compared to matched controls (18). The location of IMCL also affects insulin sensitivity. A study investigating differences in lipid deposition between endurance-trained athletes and individuals with DM who had similar IMCL levels found that those with DM store lipids in larger droplets in type II muscle fibers in the subsarcolemmal region, where the IMCL can potentially interfere with insulin signaling. In contrast, the trained athletes stored IMCL in smaller lipid droplets in type I muscle fibers in the intermyofibrillar space where they can subsequently be utilized to fuel oxidation (37). This evidence suggests that, in addition to the increased VAT generally seen with sarcopenic obesity, the imbalance of energy intake vs. expenditure and lipid distribution in adipose and muscle depots are potentially important drivers for the development of DM that require further characterization in CCS. Furthermore, the prevalence of adverse body composition, its relative contribution to the development of DM in the context of cancer treatment exposures, and how this risk compares to the general population, have not been well-defined.
As shown in Figure 1, there are multiple complex and interrelated treatment-associated mechanisms underlying the development of DM in this patient population. This includes effects of radiation and chemotherapy on the pancreas, liver, pituitary, and adipose and muscle tissues, ultimately resulting in a cycle of hyperglycemia and insulin resistance with or without impaired insulin production. Radiation is the strongest treatment-related risk factor associated with the development of subsequent DM in CCS. While cancer treatment has changed across eras, with directed effort to reduce radiation exposure for certain cancers (e.g., chest radiation in patients with Hodgkin lymphoma), radiation remains integral in the upfront treatment for many childhood malignancies such as Wilms tumor, high-risk neuroblastoma, Ewing sarcoma, and a majority of CNS tumors. Total body irradiation (TBI) is still a key component of many conditioning regimens prior to HCT, particularly in patients with relapsed acute lymphoblastic leukemia (ALL) (38, 39).
Figure 1. Mechanistic pathways involved in the development of insulin resistance in cancer survivors. Radiation and chemotherapy can have a multitude of complex, interrelated effects on the pancreas, liver, pituitary, and adipose and muscle tissues. Direct effects to the pancreas can lead to beta-cell dysfunction, subsequently resulting in decreased insulin secretion. Direct and indirect effects of treatment negatively affect muscle health (reduced muscle mass and mitochondrial respiration), which is compounded by the fatty infiltration of muscle that is seen with an increase in whole body fat mass. Together, these changes worsen peripheral tissue insulin resistance, leading to increased gluconeogenesis. This creates a cycle of hyperglycemia and decreased insulin sensitivity, with or without adequate insulin production. GH, growth hormone; IMCL, intramyocellular lipids. Created with BioRender.com.
Specifically, TBI and abdominal radiation are the two most recognized radiation treatment exposures in survivors that subsequently lead to DM. In a study of over 8,500 survivors, after adjusting for relevant clinical and treatment-related factors, survivors treated with TBI demonstrated a 7-fold increased risk (OR 7.2, 95% CI 3.4–15.0) and those with abdominal radiation, a 3-fold increased risk (OR 2.7, 95% CI 1.9–3.8) of DM compared to survivors without these respective exposures (16). Compared to siblings, and after adjusting for BMI, survivors with TBI, abdominal radiation, and cranial radiation were 12.6, 3.4, and 1.6 times as likely to develop DM, respectively (16). The increased risk of DM with TBI exposure is multifactorial, and includes direct effects on pancreatic and adipose tissues as well as the hypothalamic–pituitary axis (40). Childhood cancer survivors treated with TBI have more adipose tissue, less muscle mass, and worse muscle function compared to controls (41). Increased fat mass, in particular visceral adiposity, in TBI-treated survivors is associated with less insulin sensitivity (18). This “ectopic fat” profile, when observed along with marked hypertriglyceridemia, implicates adipocyte dysfunction and disordered fatty acid metabolism, reminiscent of lipodystrophy, in the pathogenesis of marked insulin resistance (42, 43).
The risk associated with abdominal radiation, used in the treatment of many solid tumors and certain lymphomas, is particularly increased in individuals with diagnosis and treatment at younger ages, older current age, higher BMI, and receipt of higher doses of radiation to the pancreatic tail (44). Radiation therapy to the pancreas leads to islet cell degranulation, mitochondrial destruction, and impaired insulin secretion (16). Human studies demonstrate smaller pancreatic volume on MRI after abdominal radiation, and a linear dose-dependent increased risk for developing DM with radiation to the tail of the pancreas (44–46). However, a recent study of two-year CCS with history of abdominal radiation found no difference in fasting insulin levels between those with normal vs. abnormal glucose tolerance (47). In contrast, in a study of young adult survivors of childhood ALL, those who received TBI as part HCT conditioning had a higher prevalence of abnormal glucose tolerance and lower pancreatic beta-cell reserve, less insulin secretion, and decreased pancreatic volume adjusted for body surface area, even years after TBI exposure compared to those with history of ALL who did not receive TBI and HCT (45). Together, these studies suggest that in the early survivorship period, pancreatic beta-cell damage, if present, is not clinically overt and does not account for the increased risk of DM in CCS. With time, the beta-cell damage can become more pronounced and plays a more important role in the pathogenesis of DM. Another contributary mechanism to developing DM after abdominal radiation is the effect of abdominal radiation on adipose tissue (48). Radiation is associated with decreased subcutaneous adipose tissue, which is thought to protect against metabolic dysregulation, and increased visceral adipose tissue, which increases metabolic dysregulation including insulin resistance (49, 50). Radiation-induced adipose tissue damage leads to inflammation and mitochondrial injury, both of which are also associated with the development of DM (51, 52). The extent to which radiation to the pancreas affects beta-cell function, and the timing of these effects relative to the radiation exposure deserve further delineation in future studies.
Cranial radiation is also associated with development of DM, though to a lesser degree than TBI and abdominal radiation (53). In a study of over 700 CCS with a history of cranial radiation, nearly 50% developed growth hormone deficiency (54). Untreated growth hormone deficiency is associated with alterations in body composition, including increased waist-to-height ratio and low muscle mass, that can contribute to insulin resistance (54). Cranial radiation may also impact hypothalamic responsiveness to leptin, leading to an increase in adipose tissue and its production of leptin (51). Impaired responsiveness to leptin would be expected to lead to increased caloric intake and subsequent weight gain, further increasing insulin resistance (55). Thus, both radiation exposure and increased adiposity can exacerbate similar tissue-specific inflammatory responses and mitochondrial dysfunction leading to insulin resistance. Hypothalamic-pituitary dysfunction with similar endocrine alterations as seen with cranial radiation, may also develop following neurosurgical tumor intervention (56).
Corticosteroids, commonly used in the treatment of ALL and many lymphomas, are associated with increased risk of treatment-induced DM. The posited mechanisms include steroid-induced inhibition of glucose uptake in peripheral tissues, increased hepatic gluconeogenesis, and excess lipid deposition in skeletal muscle (51, 57, 58). While not all individuals who receive corticosteroids develop DM after completion of therapy, survivors who develop treatment-induced DM during steroid therapy are at increased risk of subsequently developing DM even when off steroids (59). There is also a well-described relationship between alkylator exposure and hypogonadism (60, 61). Hypogonadism can lead to alterations in body composition including excess adiposity, thereby increasing the risk of cardiometabolic disease (62). L-asparaginase increases the risk for developing DM in the acute treatment setting by decreasing insulin secretion in response to hyperglycemia (57, 58, 63, 64). However L-asparaginase is not associated with the development of DM following completion of treatment (16).
DM has historically been considered an aging-related disease, and older age is an established risk factor for developing DM (14). In the general population, age-related changes, such as frailty (the most widely recognized phenotype of aging), mitochondrial dysfunction, and increased IMCL, can drive the development of DM (65, 66). CCS exhibit signs typically associated with premature or accelerated aging related to having received cancer treatments at a young age (67). At a mean age of 33 years, CCS demonstrate a prevalence of frailty similar to adults who are much older (≥65 years) (67). Compared to their siblings, CCS demonstrate a 6-fold higher cumulative incidence of severe or life-threatening chronic health conditions, such as hypertension, dyslipidemia, and DM, which are typically seen in older individuals (3, 68). Emerging research points to parallels in cellular processes implicated in aging- and cancer treatment-related DM. Mitochondrial dysfunction is a potential cellular mechanism underlying this accelerated aging phenotype, and studies show a decrease in mitochondrial content and respiration with aging (69–71). These alterations occur following exposure to common chemotherapeutic agents used in historical and contemporary regimens to treat pediatric cancers, such as alkylators and anthracyclines (58), or radiation therapy (72). For example, doxorubicin decreases skeletal muscle mitochondrial respiration and increases mitochondrial ROS production (73). The effects of radiation include impaired skeletal muscle fatty acid oxidation and mitochondrial oxidative capacity (72). Reduced mitochondrial quantity or function in individuals with DM has been observed in a number of studies (74–76). Specifically, individuals with DM have decreased respiration through the electron transport chain (75, 76). The decreased mitochondrial oxidative capacity may lead to lipid accumulation in skeletal muscle and thereby further impede insulin signaling (77). Thus, alterations of skeletal muscle metabolic processes due to cancer treatment are similar to the changes that occur in patients with diabetes. Additional investigations are needed to specifically elucidate the mechanisms underlying accelerated aging as they relate to the increased risk of DM in survivors of childhood cancer.
Genetic and gene-environment interactions also play an important role in the inter-individual variation in the risk of developing DM. Numerous germline genetic loci have been associated with DM both in oncology and non-oncology populations (78, 79). A recent study of CCS found an association between a novel germline genetic locus, rs55849673-A, that is associated with decreased expression of ERCC6L2, an excision repair protein involved in deoxyribonucleic acid (DNA) damage repair and mitochondrial function, and diabetes (78). The association between rs55949673 and DM was stronger in survivors not treated with abdominal radiation than in those treated with abdominal radiation. This highlights that, in the absence of abdominal radiation, genetic variations that alter mitochondrial function and response to DNA damage affect the risk of developing DM differentially in cancer survivors in the context of their treatment exposures than in the general population. It also suggests that the magnitude of DM risk attributable to radiation is far greater than the risk associated with previously identified genetic factors (78). Epigenetic processes are altered by environmental influences. Studies of epigenetic alterations like DNA methylation due to aging, changes in body composition, and stressors such as inflammation are also underway (80). Identification of such genetic and epigenetic risk factors across individuals with varied treatment exposures may help to better understand which individuals are at highest risk of developing comorbidities such as DM. Emerging high through sequencing technologies, genetic/epigenetic screening and personalized medicine can facilitate early detection for more rapid intervention to prevent progression. This has the potential to inform approaches to screening guidelines and development of interventions that are truly tailored to each individual’s unique risk factors for DM.
Guidelines in the general population recommend testing for DM in any children, adolescents, or adults who are overweight or obese by BMI and have at least one risk factor, and in all adults at age 35 (81, 82). Fasting blood glucose, HbA1c, or oral glucose tolerance test are considered appropriate for screening. As described above, there are limitations to using BMI as a key criterion for DM screening, particularly in CCS. Fasting blood glucose and HbA1c can lead to over- or underestimation of IGT, and have known limitations in certain populations (83, 84). Additionally, the younger age of DM onset in CCS may warrant earlier survivor-specific screening.
The current Children’s Oncology Group Long-Term Follow-Up Guidelines recommend screening for impaired glucose metabolism with a fasting blood glucose or HbA1c every 2 years in those with history of TBI or abdominal radiation (85). The guidelines do not currently include specific screening for impaired glucose metabolism in those with history of cranial radiation, corticosteroid exposure, or neurosurgery that may affect the hypothalamic–pituitary axis. Additional studies and quantification of this risk in CCS will inform screening guidelines following these treatment exposures.
Advancements in survivorship research have highlighted the substantial burden of DM in the growing population of CCS, as well as the complexity of DM pathogenesis. DM is a markedly heterogeneous disease, and an emerging paradigm in the non-oncology population is the identification of groups or clusters of individuals with phenotypically distinct DM (e.g., insulin-resistant, insulin-deficient, age-related, and obesity-related) (86, 87). This has not been formally studied in CCS. However, the multimodal nature of the therapeutic protocols utilized to treat pediatric cancers additionally contributes to the substantial variation in clinical phenotype and further complicates efforts to understand the specific drivers and mechanisms underlying the development of DM. The current body of literature suggests a time-dependent combination of impaired insulin secretion and muscle and adipose tissue insulin resistance which may derive from changes in body composition, including increases in VAT, adipose dysfunction and skeletal muscle IMCL. A key question for future studies is how the mechanistic etiology of these changes differs among survivors, dependent on their individual characteristics and treatment exposures. Future studies are necessary to further delineate the knowledge gap regarding body composition profiles and their role in the development of DM in CCS compared to the general population, considering additional established risk factors, and to identify strategies to promote normal growth and development in CCS. Large-scale genomic studies are also needed in CCS to identify relevant inter-individual genetic variations among different racial and ethnic groups. Addressing these questions is critical to determine which survivors are at highest risk of developing DM. This information will aid in identifying phenotypically distinct DM subgroups specifically within the context of cancer survivors. Furthermore, it will help establish clinically relevant biomarkers, risk prediction models, and risk-based screening guidelines to identify individuals most vulnerable to developing DM. Lifestyle modifications and pharmacologic interventions may then be tailored to these different diabetic phenotypes, targeting specific underlying pathways (e.g., increasing skeletal muscle mass, hepatic lipid mobilization, decreasing inflammation) and optimal times during which to intervene. For example, certain patients may derive benefit from insulin secretagogues such as sulfonylureas and others from insulin sensitizers such as metformin and thiazolidinediones. Information learned from these initial observational and interventional mechanistic studies will form the foundation for larger trials of individualized approaches aimed at early treatment and prevention of DM in at-risk childhood cancer survivors.
In summary, CCS have an increased burden of DM, an important contributor to additional morbidity and mortality, largely due to alterations resulting from their cancer treatment. Future studies aimed at characterizing the mechanisms underlying the development of DM, specifically in this patient population, will inform targeted prevention and treatment approaches that are necessary to improve the lives of cancer survivors.
RB and SM-M: conceptualization and manuscript preparation. SA, SM, and RN: manuscript preparation. All authors contributed to the article and approved the submitted version.
This work was supported, in part, by grants from the NIH [K12CA001727(RB), R01DK065073 (RN)] and Schaeffer Endowment Funds (RN). The content is solely the responsibility of the authors and does not necessarily represent the official views of the National Institutes of Health.
The authors declare that the research was conducted in the absence of any commercial or financial relationships that could be construed as a potential conflict of interest.
The handling editor NS declared a past co-authorship with the author SA.
All claims expressed in this article are solely those of the authors and do not necessarily represent those of their affiliated organizations, or those of the publisher, the editors and the reviewers. Any product that may be evaluated in this article, or claim that may be made by its manufacturer, is not guaranteed or endorsed by the publisher.
1. Howlader, N, Noone, AM, Krapcho, M, Miller, D, Brest, A, Yu, M, et al. (eds.) SEER Cancer statistics review, 1975–2017. Bethesda, MD: National Cancer Institute. Based on November 2019 SEER data submission, posted to the SEER web site, April 2020. Available at: https://seer.cancer.gov/csr/1975_2017/
2. Robison, LL, and Hudson, MM. Survivors of childhood and adolescent cancer: life-long risks and responsibilities. Nat Rev Cancer. (2014) 14:61–70. doi: 10.1038/nrc3634
3. Gibson, TM, Mostoufi-Moab, S, Stratton, KL, Leisenring, WM, Barnea, D, Chow, EJ, et al. Temporal patterns in the risk of chronic health conditions in survivors of childhood cancer diagnosed 1970-99: a report from the childhood Cancer survivor study cohort. Lancet Oncol. (2018) 19:1590–601. doi: 10.1016/S1470-2045(18)30537-0
4. van Atteveld, JE, Mulder, RL, van den Heuvel-Eibrink, MM, Hudson, MM, Kremer, LCM, Skinner, R, et al. Bone mineral density surveillance for childhood, adolescent, and young adult cancer survivors: evidence-based recommendations from the international late effects of childhood Cancer guideline harmonization group. Lancet Diabetes Endocrinol. (2021) 9:622–37. doi: 10.1016/S2213-8587(21)00173-X
5. Armenian, SH, Sun, CL, Vase, T, Ness, KK, Blum, E, Francisco, L, et al. Cardiovascular risk factors in hematopoietic cell transplantation survivors: role in development of subsequent cardiovascular disease. Blood. (2012) 120:4505–12. doi: 10.1182/blood-2012-06-437178
6. Dixon, SB, Liu, Q, Chow, EJ, Oeffinger, KC, Nathan, PC, Howell, RM, et al. Specific causes of excess late mortality and association with modifiable risk factors among survivors of childhood cancer: a report from the childhood Cancer survivor study cohort. Lancet. (2023) 401:1447–57. doi: 10.1016/S0140-6736(22)02471-0
7. Armenian, SH, Armstrong, GT, Aune, G, Chow, EJ, Ehrhardt, MJ, Ky, B, et al. Cardiovascular disease in survivors of childhood Cancer: insights into epidemiology, pathophysiology, and prevention. J Clin Oncol. (2018) 36:2135–44. doi: 10.1200/JCO.2017.76.3920
8. Oh, S, Lee, J, Hong, YS, and Kim, K. Increased risk of cardiovascular disease associated with diabetes among adult cancer survivors: a population-based matched cohort study. Eur J Prev Cardiol. (2023) 30:670–9. doi: 10.1093/eurjpc/zwad046
9. American, DA. Economic costs of diabetes in the U.S. in 2017. Diabetes Care. (2018) 41:917–28. doi: 10.2337/dci18-0007
10. Caraballo, C, Valero-Elizondo, J, Khera, R, Mahajan, S, Grandhi, GR, Virani, SS, et al. Burden and consequences of financial hardship from medical bills among nonelderly adults with diabetes mellitus in the United States. Circ Cardiovasc Qual Outcomes. (2020) 13:e006139. doi: 10.1161/CIRCOUTCOMES.119.006139
11. Nipp, RD, Kirchhoff, AC, Fair, D, Rabin, J, Hyland, KA, Kuhlthau, K, et al. Financial burden in survivors of childhood Cancer: a report from the childhood Cancer survivor study. J Clin Oncol. (2017) 35:3474–81. doi: 10.1200/JCO.2016.71.7066
12. Perreault, L, Pan, Q, Mather, KJ, Watson, KE, Hamman, RF, Kahn, SE, et al. Effect of regression from prediabetes to normal glucose regulation on long-term reduction in diabetes risk: results from the diabetes prevention program outcomes study. Lancet. (2012) 379:2243–51. doi: 10.1016/S0140-6736(12)60525-X
13. Abdullah, A, Peeters, A, de Courten, M, and Stoelwinder, J. The magnitude of association between overweight and obesity and the risk of diabetes: a meta-analysis of prospective cohort studies. Diabetes Res Clin Pract. (2010) 89:309–19. doi: 10.1016/j.diabres.2010.04.012
14. Force, USPST, Davidson, KW, Barry, MJ, Mangione, CM, Cabana, M, Caughey, AB, et al. Screening for prediabetes and type 2 diabetes: US preventive services task Force recommendation statement. JAMA. (2021) 326:736–43. doi: 10.1001/jama.2021.12531
15. Galicia-Garcia, U, Benito-Vicente, A, Jebari, S, Larrea-Sebal, A, Siddiqi, H, Uribe, KB, et al. Pathophysiology of type 2 diabetes mellitus. Int J Mol Sci. (2020) 21:6275. doi: 10.3390/ijms21176275
16. Meacham, LR, Sklar, CA, Li, S, Liu, Q, Gimpel, N, Yasui, Y, et al. Diabetes mellitus in long-term survivors of childhood cancer. Increased risk associated with radiation therapy: a report for the childhood cancer survivor study. Arch Intern Med. (2009) 169:1381–8. doi: 10.1001/archinternmed.2009.209
17. Mostoufi-Moab, S, Ginsberg, JP, Bunin, N, Zemel, BS, Shults, J, Thayu, M, et al. Body composition abnormalities in long-term survivors of pediatric hematopoietic stem cell transplantation. J Pediatr. (2012) 160:122–8. doi: 10.1016/j.jpeds.2011.06.041
18. Mostoufi-Moab, S, Magland, J, Isaacoff, EJ, Sun, W, Rajapakse, CS, Zemel, B, et al. Adverse fat depots and marrow adiposity are associated with skeletal deficits and insulin resistance in Long-term survivors of pediatric hematopoietic stem cell transplantation. J Bone Miner Res. (2015) 30:1657–66. doi: 10.1002/jbmr.2512
19. Karpe, F, and Pinnick, KE. Biology of upper-body and lower-body adipose tissue—link to whole-body phenotypes. Nat Rev Endocrinol. (2015) 11:90–100. doi: 10.1038/nrendo.2014.185
20. Martin, ML, and Jensen, MD. Effects of body fat distribution on regional lipolysis in obesity. J Clin Invest. (1991) 88:609–13. doi: 10.1172/JCI115345
21. Nielsen, S, Guo, Z, Johnson, CM, Hensrud, DD, and Jensen, MD. Splanchnic lipolysis in human obesity. J Clin Invest. (2004) 113:1582–8. doi: 10.1172/JCI21047
22. Marriott, CJC, Beaumont, LF, Farncombe, TH, Cranston, AN, Athale, UH, Yakemchuk, VN, et al. Body composition in long-term survivors of acute lymphoblastic leukemia diagnosed in childhood and adolescence: a focus on sarcopenic obesity. Cancer. (2018) 124:1225–31. doi: 10.1002/cncr.31191
23. Wei, C, Thyagiarajan, MS, Hunt, LP, Shield, JP, Stevens, MC, and Crowne, EC. Reduced insulin sensitivity in childhood survivors of haematopoietic stem cell transplantation is associated with lipodystropic and sarcopenic phenotypes. Pediatr Blood Cancer. (2015) 62:1992–9. doi: 10.1002/pbc.25601
24. Bhandari, R, Teh, JB, He, T, Peng, K, Iukuridze, A, Atencio, L, et al. Association between body composition and development of glucose intolerance after allogeneic hematopoietic cell transplantation. Cancer Epidemiol Biomark Prev. (2022) 31:2004–10. doi: 10.1158/1055-9965.EPI-21-1449
25. Valaiyapathi, B, Gower, B, and Ashraf, AP. Pathophysiology of type 2 diabetes in children and adolescents. Curr Diabetes Rev. (2020) 16:220–9. doi: 10.2174/1573399814666180608074510
26. Kyle, UG, Chalandon, Y, Miralbell, R, Karsegard, VL, Hans, D, Trombetti, A, et al. Longitudinal follow-up of body composition in hematopoietic stem cell transplant patients. Bone Marrow Transplant. (2005) 35:1171–7. doi: 10.1038/sj.bmt.1704996
27. Yee, LD, Mortimer, JE, Natarajan, R, Dietze, EC, and Seewaldt, VL. Metabolic health, insulin, and breast Cancer: why oncologists should care about insulin. Front Endocrinol (Lausanne). (2020) 11:58. doi: 10.3389/fendo.2020.00058
28. Titchenell, PM, Lazar, MA, and Birnbaum, MJ. Unraveling the regulation of hepatic metabolism by insulin. Trends Endocrinol Metab. (2017) 28:497–505. doi: 10.1016/j.tem.2017.03.003
29. Boden, G, She, P, Mozzoli, M, Cheung, P, Gumireddy, K, Reddy, P, et al. Free fatty acids produce insulin resistance and activate the proinflammatory nuclear factor-kappaB pathway in rat liver. Diabetes. (2005) 54:3458–65. doi: 10.2337/diabetes.54.12.3458
30. Mathew, M, Tay, E, and Cusi, K. Elevated plasma free fatty acids increase cardiovascular risk by inducing plasma biomarkers of endothelial activation, myeloperoxidase and PAI-1 in healthy subjects. Cardiovasc Diabetol. (2010) 9:9. doi: 10.1186/1475-2840-9-9
31. Kato, M, and Natarajan, R. Epigenetics and epigenomics in diabetic kidney disease and metabolic memory. Nat Rev Nephrol. (2019) 15:327–45. doi: 10.1038/s41581-019-0135-6
32. Reddy, MA, Zhang, E, and Natarajan, R. Epigenetic mechanisms in diabetic complications and metabolic memory. Diabetologia. (2015) 58:443–55. doi: 10.1007/s00125-014-3462-y
33. Machann, J, Haring, H, Schick, F, and Stumvoll, M. Intramyocellular lipids and insulin resistance. Diabetes Obes Metab. (2004) 6:239–48. doi: 10.1111/j.1462-8902.2004.00339.x
34. Sucharita, S, Pranathi, R, Correa, M, Keerthana, P, Ramesh, LJ, Bantwal, G, et al. Evidence of higher intramyocellular fat among normal and overweight Indians with prediabetes. Eur J Clin Nutr. (2019) 73:1373–81. doi: 10.1038/s41430-019-0402-4
35. Gavin, TP, Ernst, JM, Kwak, HB, Caudill, SE, Reed, MA, Garner, RT, et al. High incomplete skeletal muscle fatty acid oxidation explains low muscle insulin sensitivity in poorly controlled T2D. J Clin Endocrinol Metab. (2018) 103:882–9. doi: 10.1210/jc.2017-01727
36. Stephens, NA, Skipworth, RJ, Macdonald, AJ, Greig, CA, Ross, JA, and Fearon, KC. Intramyocellular lipid droplets increase with progression of cachexia in cancer patients. J Cachexia Sarcopenia Muscle. (2011) 2:111–7. doi: 10.1007/s13539-011-0030-x
37. Daemen, S, Gemmink, A, Brouwers, B, Meex, RCR, Huntjens, PR, Schaart, G, et al. Distinct lipid droplet characteristics and distribution unmask the apparent contradiction of the athlete’s paradox. Mol Metab. (2018) 17:71–81. doi: 10.1016/j.molmet.2018.08.004
38. National Academies of Sciences, Engineering, and Medicine. Childhood cancer and functional impacts across the care continuum. (2021). Washington, DC: The National Academies Press. doi: 10.17226/25944
39. Armstrong, GT, Chen, Y, Yasui, Y, Leisenring, W, Gibson, TM, Mertens, AC, et al. Reduction in late mortality among 5-year survivors of childhood Cancer. N Engl J Med. (2016) 374:833–42. doi: 10.1056/NEJMoa1510795
40. Rose, SR, Horne, VE, Howell, J, Lawson, SA, Rutter, MM, Trotman, GE, et al. Late endocrine effects of childhood cancer. Nat Rev Endocrinol. (2016) 12:319–36. doi: 10.1038/nrendo.2016.45
41. Lorenc, A, Hamilton-Shield, J, Perry, R, Stevens, M, and Adipose, CH. Muscle late effects working G. body composition after allogeneic haematopoietic cell transplantation/total body irradiation in children and young people: a restricted systematic review. J Cancer Surviv. (2020) 14:624–42. doi: 10.1007/s11764-020-00871-1
42. Ahima, RS. Adipose tissue as an endocrine organ. Obesity (Silver Spring). (2006) 14:242S–9S. doi: 10.1038/oby.2006.317
43. Tan, GD, Savage, DB, Fielding, BA, Collins, J, Hodson, L, Humphreys, SM, et al. Fatty acid metabolism in patients with PPARgamma mutations. J Clin Endocrinol Metab. (2008) 93:4462–70. doi: 10.1210/jc.2007-2356
44. Friedman, DN, Moskowitz, CS, Hilden, P, Howell, RM, Weathers, RE, Smith, SA, et al. Radiation dose and volume to the pancreas and subsequent risk of diabetes mellitus: a report from the childhood Cancer survivor study. J Natl Cancer Inst. (2020) 112:525–32. doi: 10.1093/jnci/djz152
45. Wei, C, Thyagiarajan, M, Hunt, L, Cox, R, Bradley, K, Elson, R, et al. Reduced beta-cell reserve and pancreatic volume in survivors of childhood acute lymphoblastic leukaemia treated with bone marrow transplantation and total body irradiation. Clin Endocrinol. (2015) 82:59–67. doi: 10.1111/cen.12575
46. de Vathaire, F, El-Fayech, C, Ben Ayed, FF, Haddy, N, Guibout, C, Winter, D, et al. Radiation dose to the pancreas and risk of diabetes mellitus in childhood cancer survivors: a retrospective cohort study. Lancet Oncol. (2012) 13:1002–10. doi: 10.1016/S1470-2045(12)70323-6
47. Friedman, DN, Hilden, P, Moskowitz, CS, Wolden, SL, Tonorezos, ES, Antal, Z, et al. Insulin and glucose homeostasis in childhood cancer survivors treated with abdominal radiation: a pilot study. Pediatr Blood Cancer. (2018) 65:e27304. doi: 10.1002/pbc.27304
48. Oeffinger, KC, and Sklar, CA. Abdominal radiation and diabetes: one more piece in the puzzle. Lancet Oncol. (2012) 13:961–2. doi: 10.1016/S1470-2045(12)70340-6
49. Hausman, GJ, Basu, U, Du, M, Fernyhough-Culver, M, and Dodson, MV. Intermuscular and intramuscular adipose tissues: bad vs. good adipose tissues. Adipocytes. (2014) 3:242–55. doi: 10.4161/adip.28546
50. Poglio, S, Galvani, S, Bour, S, Andre, M, Prunet-Marcassus, B, Penicaud, L, et al. Adipose tissue sensitivity to radiation exposure. Am J Pathol. (2009) 174:44–53. doi: 10.2353/ajpath.2009.080505
51. Romano, A, Del Vescovo, E, Rivetti, S, Triarico, S, Attina, G, Mastrangelo, S, et al. Biomarkers predictive of metabolic syndrome and cardiovascular disease in childhood Cancer survivors. J Pers Med. (2022) 12:880. doi: 10.3390/jpm12060880
52. Rossi, F, Di Paola, A, Pota, E, Argenziano, M, Di Pinto, D, Marrapodi, MM, et al. Biological aspects of Inflamm-aging in childhood Cancer survivors. Cancers (Basel). (2021) 13:4933. doi: 10.3390/cancers13194933
53. Mostoufi-Moab, S, Seidel, K, Leisenring, WM, Armstrong, GT, Oeffinger, KC, Stovall, M, et al. Endocrine abnormalities in aging survivors of childhood Cancer: a report from the childhood Cancer survivor study. J Clin Oncol. (2016) 34:3240–7. doi: 10.1200/JCO.2016.66.6545
54. Chemaitilly, W, Li, Z, Huang, S, Ness, KK, Clark, KL, Green, DM, et al. Anterior hypopituitarism in adult survivors of childhood cancers treated with cranial radiotherapy: a report from the St Jude lifetime cohort study. J Clin Oncol. (2015) 33:492–500. doi: 10.1200/JCO.2014.56.7933
55. Izquierdo, AG, Crujeiras, AB, Casanueva, FF, and Carreira, MC. Leptin obesity, and leptin resistance: where are we 25 years later? Nutrients. (2019) 11:2704. doi: 10.3390/nu11112704
56. van Iersel, L, Mulder, RL, Denzer, C, Cohen, LE, Spoudeas, HA, Meacham, LR, et al. Hypothalamic-pituitary and other endocrine surveillance among childhood Cancer survivors. Endocr Rev. (2022) 43:794–823. doi: 10.1210/endrev/bnab040
57. Howard, SC, and Pui, CH. Endocrine complications in pediatric patients with acute lymphoblastic leukemia. Blood Rev. (2002) 16:225–43. doi: 10.1016/S0268-960X(02)00042-5
58. Lowas, SR, Marks, D, and Malempati, S. Prevalence of transient hyperglycemia during induction chemotherapy for pediatric acute lymphoblastic leukemia. Pediatr Blood Cancer. (2009) 52:814–8. doi: 10.1002/pbc.21980
59. Williams, HE, Howell, CR, Chemaitilly, W, Wilson, CL, Karol, SE, Nolan, VG, et al. Diabetes mellitus among adult survivors of childhood acute lymphoblastic leukemia: a report from the St. Jude lifetime cohort study. Cancer. (2020) 126:870–8. doi: 10.1002/cncr.32596
60. Chemaitilly, W, Mertens, AC, Mitby, P, Whitton, J, Stovall, M, Yasui, Y, et al. Acute ovarian failure in the childhood cancer survivor study. J Clin Endocrinol Metab. (2006) 91:1723–8. doi: 10.1210/jc.2006-0020
61. Chow, EJ, Stratton, KL, Leisenring, WM, Oeffinger, KC, Sklar, CA, Donaldson, SS, et al. Pregnancy after chemotherapy in male and female survivors of childhood cancer treated between 1970 and 1999: a report from the childhood Cancer survivor study cohort. Lancet Oncol. (2016) 17:567–76. doi: 10.1016/S1470-2045(16)00086-3
62. Mejdahl Nielsen, M, Mathiesen, S, Suominen, A, Sorensen, K, Ifversen, M, Molgaard, C, et al. Altered body composition in male long-term survivors of paediatric allogeneic haematopoietic stem cell transplantation: impact of conditioning regimen, chronic graft-versus-host disease and hypogonadism. Bone Marrow Transplant. (2021) 56:457–60. doi: 10.1038/s41409-020-01038-3
63. Yoshida, H, Imamura, T, Saito, AM, Takahashi, Y, Suenobu, S, Hasegawa, D, et al. Protracted administration of L-Asparaginase in maintenance phase is the risk factor for hyperglycemia in older patients with pediatric acute lymphoblastic leukemia. PLoS One. (2015) 10:e0136428. doi: 10.1371/journal.pone.0136428
64. Pui, CH, Burghen, GA, Bowman, WP, and Aur, RJ. Risk factors for hyperglycemia in children with leukemia receiving L-asparaginase and prednisone. J Pediatr. (1981) 99:46–50. doi: 10.1016/S0022-3476(81)80955-9
65. Stout, MB, Justice, JN, Nicklas, BJ, and Kirkland, JL. Physiological aging: links among Adipose tissue dysfunction, diabetes, and frailty. Physiology (Bethesda). (2017) 32:9–19. doi: 10.1152/physiol.00012.2016
66. Cao, X, Yang, Z, Li, X, Chen, C, Hoogendijk, EO, Zhang, J, et al. Association of frailty with the incidence risk of cardiovascular disease and type 2 diabetes mellitus in long-term cancer survivors: a prospective cohort study. BMC Med. (2023) 21:74. doi: 10.1186/s12916-023-02774-1
67. Ness, KK, Krull, KR, Jones, KE, Mulrooney, DA, Armstrong, GT, Green, DM, et al. Physiologic frailty as a sign of accelerated aging among adult survivors of childhood cancer: a report from the St Jude lifetime cohort study. J Clin Oncol. (2013) 31:4496–503. doi: 10.1200/JCO.2013.52.2268
68. Armstrong, GT, Oeffinger, KC, Chen, Y, Kawashima, T, Yasui, Y, Leisenring, W, et al. Modifiable risk factors and major cardiac events among adult survivors of childhood cancer. J Clin Oncol. (2013) 31:3673–80. doi: 10.1200/JCO.2013.49.3205
69. Johannsen, DL, Conley, KE, Bajpeyi, S, Punyanitya, M, Gallagher, D, Zhang, Z, et al. Ectopic lipid accumulation and reduced glucose tolerance in elderly adults are accompanied by altered skeletal muscle mitochondrial activity. J Clin Endocrinol Metab. (2012) 97:242–50. doi: 10.1210/jc.2011-1798
70. Conley, KE, Jubrias, SA, and Esselman, PC. Oxidative capacity and ageing in human muscle. J Physiol. (2000) 526:203–10. doi: 10.1111/j.1469-7793.2000.t01-1-00203.x
71. Johnson, ML, Irving, BA, Lanza, IR, Vendelbo, MH, Konopka, AR, Robinson, MM, et al. Differential effect of endurance training on mitochondrial protein damage, degradation, and acetylation in the context of aging. J Gerontol A Biol Sci Med Sci. (2015) 70:1386–93. doi: 10.1093/gerona/glu221
72. Amorim, NML, Kee, A, Coster, ACF, Lucas, C, Bould, S, Daniel, S, et al. Irradiation impairs mitochondrial function and skeletal muscle oxidative capacity: significance for metabolic complications in cancer survivors. Metabolism. (2020) 103:154025. doi: 10.1016/j.metabol.2019.154025
73. Gilliam, LAA, Fisher-Wellman, KH, Lin, CT, Maples, JM, Cathey, BL, and Neufer, PD. The anticancer agent doxorubicin disrupts mitochondrial energy metabolism and redox balance in skeletal muscle. Free Radic Biol Med. (2013) 65:988–96. doi: 10.1016/j.freeradbiomed.2013.08.191
74. Sangwung, P, Petersen, KF, Shulman, GI, and Knowles, JW. Mitochondrial dysfunction, insulin resistance, and potential genetic implications. Endocrinology. (2020) 161:bqaa017. doi: 10.1210/endocr/bqaa017
75. Mogensen, M, Sahlin, K, Fernstrom, M, Glintborg, D, Vind, BF, Beck-Nielsen, H, et al. Mitochondrial respiration is decreased in skeletal muscle of patients with type 2 diabetes. Diabetes. (2007) 56:1592–9. doi: 10.2337/db06-0981
76. Ritov, VB, Menshikova, EV, Azuma, K, Wood, R, Toledo, FG, Goodpaster, BH, et al. Deficiency of electron transport chain in human skeletal muscle mitochondria in type 2 diabetes mellitus and obesity. Am J Physiol Endocrinol Metab. (2010) 298:E49–58. doi: 10.1152/ajpendo.00317.2009
77. Schrauwen-Hinderling, VB, Kooi, ME, Hesselink, MK, Jeneson, JA, Backes, WH, van Echteld, CJ, et al. Impaired in vivo mitochondrial function but similar intramyocellular lipid content in patients with type 2 diabetes mellitus and BMI-matched control subjects. Diabetologia. (2007) 50:113–20. doi: 10.1007/s00125-006-0475-1
78. Richard, MA, Mostoufi-Moab, S, Rathore, N, Baedke, J, Brown, AL, Chanock, SJ, et al. Germline genetic and treatment-related risk factors for diabetes mellitus in survivors of childhood Cancer: a report from the childhood Cancer survivor study and St Jude lifetime cohorts. JCO Precis Oncol. (2022) 6:e2200239. doi: 10.1200/PO.22.00239
79. Mahajan, A, Taliun, D, Thurner, M, Robertson, NR, Torres, JM, Rayner, NW, et al. Fine-mapping type 2 diabetes loci to single-variant resolution using high-density imputation and islet-specific epigenome maps. Nat Genet. (2018) 50:1505–13. doi: 10.1038/s41588-018-0241-6
80. Dhawan, S, and Natarajan, R. Epigenetics and type 2 diabetes risk. Curr Diab Rep. (2019) 19:47. doi: 10.1007/s11892-019-1168-8
81. American Diabetes Association Professional Practice Committee. 2. Classification and diagnosis of diabetes: standards of medical Care in Diabetes-2022. Diabetes Care. (2022) 45:S17–38. doi: 10.2337/dc22-S002
82. American, DA. 13. Children and adolescents: standards of medical Care in Diabetes-2020. Diabetes Care. (2020) 43:S163–82. doi: 10.2337/dc20-S013
83. Echouffo-Tcheugui, JB, Perreault, L, Ji, L, and Dagogo-Jack, S. Diagnosis and Management of Prediabetes: a review. JAMA. (2023) 329:1206–16. doi: 10.1001/jama.2023.4063
84. Gyberg, V, De Bacquer, D, Kotseva, K, De Backer, G, Schnell, O, Sundvall, J, et al. Screening for dysglycaemia in patients with coronary artery disease as reflected by fasting glucose, oral glucose tolerance test, and HbA1c: a report from EUROASPIRE IV—a survey from the European Society of Cardiology. Eur Heart J. (2015) 36:1171–7. doi: 10.1093/eurheartj/ehv008
85. Children’s Oncology Group. Long-term follow-up guidelines for survivors of childhood, adolescent and young adult cancers, version 5.0. Monrovia, CA: Children’s Oncology Group (2018).
86. Zaharia, OP, Strassburger, K, Strom, A, Bonhof, GJ, Karusheva, Y, Antoniou, S, et al. Risk of diabetes-associated diseases in subgroups of patients with recent-onset diabetes: a 5-year follow-up study. Lancet Diabetes Endocrinol. (2019) 7:684–94. doi: 10.1016/S2213-8587(19)30187-1
Keywords: diabetes, childhood cancer survivor, insulin resistance, radiation, body composition
Citation: Bhandari R, Armenian SH, McCormack S, Natarajan R and Mostoufi-Moab S (2023) Diabetes in childhood cancer survivors: emerging concepts in pathophysiology and future directions. Front. Med. 10:1206071. doi: 10.3389/fmed.2023.1206071
Received: 14 April 2023; Accepted: 10 August 2023;
Published: 22 August 2023.
Edited by:
Noha Sharafeldin, University of Alabama at Birmingham, United StatesReviewed by:
Giacomina Brunetti, University of Bari Aldo Moro, ItalyCopyright © 2023 Bhandari, Armenian, McCormack, Natarajan and Mostoufi-Moab. This is an open-access article distributed under the terms of the Creative Commons Attribution License (CC BY). The use, distribution or reproduction in other forums is permitted, provided the original author(s) and the copyright owner(s) are credited and that the original publication in this journal is cited, in accordance with accepted academic practice. No use, distribution or reproduction is permitted which does not comply with these terms.
*Correspondence: Rusha Bhandari, cmJoYW5kYXJpQGNvaC5vcmc=
Disclaimer: All claims expressed in this article are solely those of the authors and do not necessarily represent those of their affiliated organizations, or those of the publisher, the editors and the reviewers. Any product that may be evaluated in this article or claim that may be made by its manufacturer is not guaranteed or endorsed by the publisher.
Research integrity at Frontiers
Learn more about the work of our research integrity team to safeguard the quality of each article we publish.