- 1Division of Neurosurgery, Department of Clinical Neurosciences, University of Cambridge, Cambridge, England, United Kingdom
- 2Cancer Research UK Cambridge Institute, University of Cambridge, Cambridge, England, United Kingdom
- 3School of Clinical Medicine, University of Cambridge, Cambridge, England, United Kingdom
Introduction: Glioblastoma is the most common and malignant primary brain tumour with median survival of 14.6 months. Personalised medicine aims to improve survival by targeting individualised patient characteristics. However, a major limitation has been application of targeted therapies in a non-personalised manner without biomarker enrichment. This has risked therapies being discounted without fair and rigorous evaluation. The objective was therefore to synthesise the current evidence on survival efficacy of personalised therapies in glioblastoma.
Methods: Studies reporting a survival outcome in human adults with supratentorial glioblastoma were eligible. PRISMA guidelines were followed. MEDLINE, Embase, Scopus, Web of Science and the Cochrane Library were searched to 5th May 2022. Clinicaltrials.gov was searched to 25th May 2022. Reference lists were hand-searched. Duplicate title/abstract screening, data extraction and risk of bias assessments were conducted. A quantitative synthesis is presented.
Results: A total of 102 trials were included: 16 were randomised and 41 studied newly diagnosed patients. Of 5,527 included patients, 59.4% were male and mean age was 53.7 years. More than 20 types of personalised therapy were included: targeted molecular therapies were the most studied (33.3%, 34/102), followed by autologous dendritic cell vaccines (32.4%, 33/102) and autologous tumour vaccines (10.8%, 11/102). There was no consistent evidence for survival efficacy of any personalised therapy.
Conclusion: Personalised glioblastoma therapies remain of unproven survival benefit. Evidence is inconsistent with high risk of bias. Nonetheless, encouraging results in some trials provide reason for optimism. Future focus should address target-enriched trials, combination therapies, longitudinal biomarker monitoring and standardised reporting.
Introduction
Glioblastoma is the most common and malignant primary brain tumour (1, 2). It accounts for 48% of all primary central nervous system (CNS) cancers, with estimated annual incidence and prevalence of 3.2 and 9.2 per 100,000 population in North America (3). Incidence is 1.6 times higher in males and greater than 2 times higher in Caucasians than in Black and Asian populations (2, 4).
The nomenclature of CNS tumours is rapidly evolving. The 2021 WHO classification characterised all glioblastoma as IDH wild type, with IDH mutant glioblastoma eliminated as a term and classified within IDH mutant astrocytoma (5). Nonetheless, aetiology remains unknown, with ionizing radiation the only proven environmental risk factor (6, 7). With the exception of hereditary tumour syndromes (8), glioblastoma appears sporadic with no proven genetic predisposition (2, 9).
Standard therapy is maximal safe surgical resection (10, 11), adjuvant radiotherapy and temozolomide (TMZ) chemotherapy, maintenance TMZ and, in some countries, electromagnetic tumour treating fields (12–14). Despite the economic burden of current therapy often amassing hundreds of thousands of US dollars (15), glioblastoma cannot be cured; the disease is rapidly and uniformly fatal with a median on-treatment overall survival of 14.6 months and a 5-year survival rate of 5% (16, 17). With a prognosis that has not improved in the past 3 decades and an average number of life years lost, that at 20.1 years, is the highest of any cancer, therapeutic advances are gravely needed (2, 18).
A surgical cure appears unattainable due to the highly infiltrative nature of disease, spreading far beyond intraoperative and radiological margins(Figure 1A) (19, 20). Nonetheless, a promising approach lies in personalised medicine (21). This encompasses a paradigm shift from disease-centric therapeutics that overlook inter-patient variation (22), towards individualised and precise targeting of unique patient characteristics (Figure 1B).
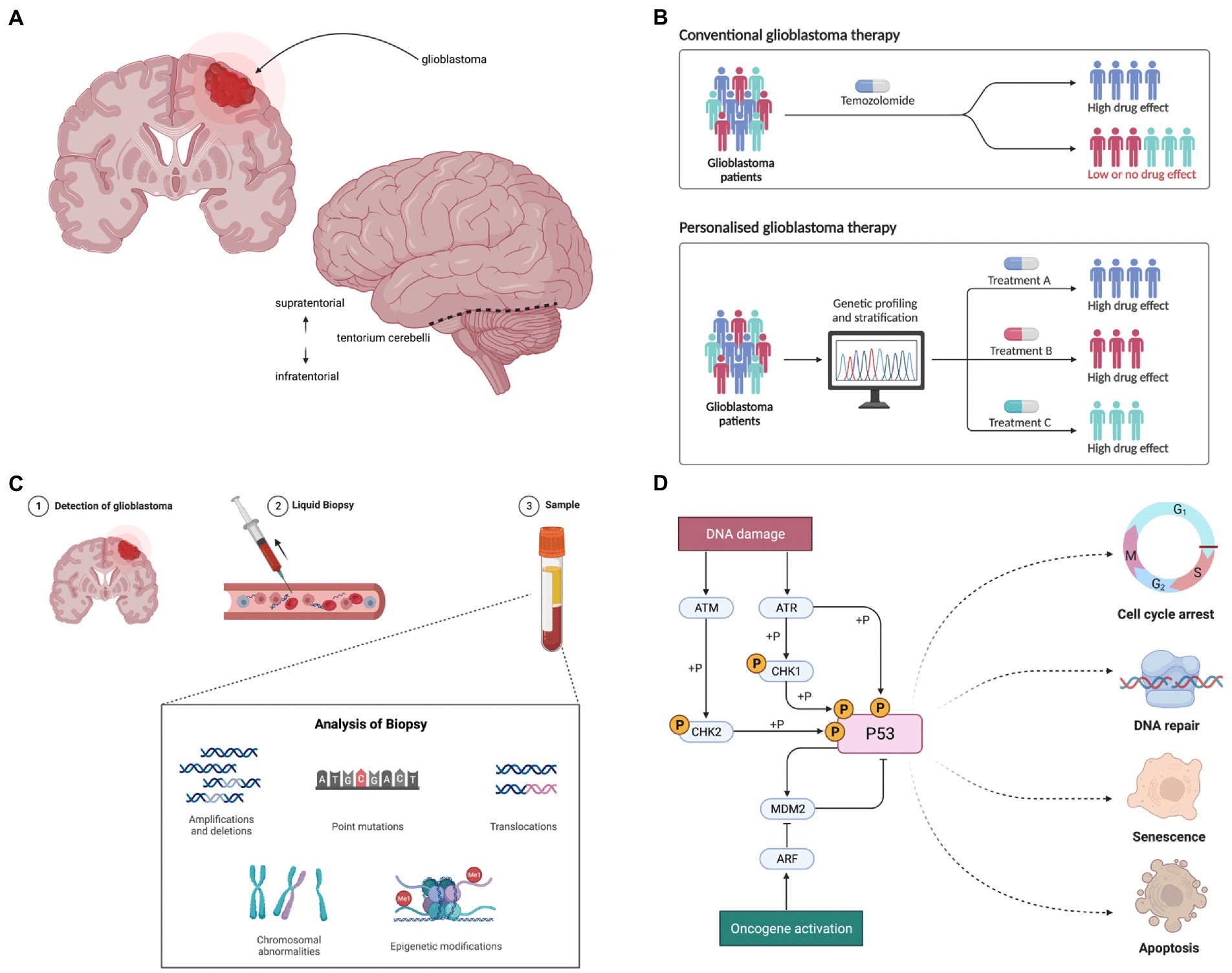
Figure 1. (A) Glioblastoma presents as a space occupying tumour within the central nervous system, most commonly occurring within the supratentorial region. (B) Personalised medicine aims to tailor therapeutics to individual patients to maximise efficacy: numerous genomic variants have been identified as potential targets in glioblastoma. (C) Molecular targets in malignant glioblastoma cells evolve over time in response to selection pressures, such as exposure to therapies. Non-invasive liquid biopsy holds significant potential as a facilitator of personalised therapy; longitudinal monitoring of tumour biomarkers may permit dynamic optimisation of targeted therapies. (D) The p53 pathway is one of the key cellular signalling pathways in which variants have been identified in malignant glioblastoma cells, including in MDM2 and TP53. The redundancy seen within and between cellular pathways likely contributes to therapeutic failure of single agents. Figure created using BioRender.com.
Glioblastoma appears particularly suited to biomarker-driven personalised genomic medicine. It was the first cancer to undergo comprehensive genomic analysis in the Cancer Genome Atlas project (23) and characterisation of variants in genes and pathways has rapidly accrued (24–26). Furthermore, glioblastoma demonstrates high inter- and intra-tumour spatial and temporal genomic heterogeneity (27–29), predicting targeted combination therapies may have higher efficacy than indiscriminate single agents. For example, mutually exclusive EGFR and PDGFRA oncogene amplification in intermingled subclones of glioblastoma cells (30) requires simultaneous inhibition of both for pathway inhibition in vitro (31). Moreover, the dynamic nature of genomic heterogeneity appears to fuel recurrence and resistance; therapy drives clonal evolution secondary to novel mutational events and selection of resistant subclones (32). Longitudinal biomarker monitoring may facilitate dynamic adjustment of personalised therapy (Figure 1C), with current focus on non-invasive methods (33, 34).
An extensive number of targets for personalised glioblastoma medicine have been identified. For example, variants disrupting receptor tyrosine kinase (RTK) pathways have been characterised, including amplification and/or mutation in EGFR, KIT, PDGFRA, FGFR1, FGFR3, and MET (28, 35). Variants have also been described in the PI3K/Akt/mTOR and MAPK signalling pathways, including in PTEN, PIK3CA, NF1, and BRAF (36). In addition, variants in cycle control genes, such as MDM2 and TP53 in the p53 pathway (Figure 1D) and CDK4, CDK6 and RB1 in the Rb pathway have been identified (28, 37). Despite this extensive genomic characterisation, the functional, biological and clinical relevance of the majority of variants is currently poorly characterised (20), making target prioritisation a major challenge (38, 39).
Furthermore, several vaccine-based personalised approaches have been explored (40). Vaccines stimulate tumour-specific immune responses to injected antigens, aiming to overcome some of the unique challenges of glioblastoma, including an immunosuppressive tumour microenvironment, low mutational burden and a relatively immunologically isolated location within the CNS (41, 42). In addition, clinical trials of non-vaccine immunotherapies are also ongoing, including immune checkpoint inhibitors and chimeric-antigen receptor (CAR) T cell therapies (41).
Despite conceptual rationale and pre-clinical data, clinical trials have so far been unable to demonstrate survival efficacy for most targeted glioblastoma therapies (20). However, most trials of targeted therapies have not been personalised due to lacking biomarker enrichment. For example, whilst 20–30% of glioblastoma is estimated to express EGFRvIII (20, 35), any efficacy signal from patients expressing the variant may be lost in a trial in which most patients would not be expected to express this target. Furthermore, nearly all trials have studied single agents, overlooking the redundancy within molecular pathways. Given the substantial need for interventions that improve glioblastoma survival, personalised targeted therapies should be rigorously and fairly evaluated before being discounted.
The aim of this quantitative systematic review of effectiveness was therefore to synthesise the current evidence on survival efficacy of personalised therapeutic approaches in glioblastoma. The objective was to evaluate past approaches and identify possible future directions.
Methods
Study design
A systematic review was conducted with reference to the Preferred Reporting Items for Systematic Reviews and Meta-Analyses (PRISMA) 2020 checklists (Appendix F) (43, 44).
Eligibility criteria
Inclusion criteria
• Adult ≥ 18 years;
• Human study;
• English language;
• Supratentorial glioblastoma population, including extraction of glioblastoma-specific data from a broader cohort;
• Any personalised therapy including genomic, transcriptomic, proteomic and vaccines;
• Clinical trial or observational study;
• Survival outcome.
Exclusion criteria
• Review or meta-analysis;
• Case report;
• Letter;
• Editorial;
• Opinion article;
• Correction.
Information sources
MEDLINE, Embase, Web of Science, Scopus and the Cochrane Library were searched from inception to 5th May 2022. MEDLINE and Embase searches were performed using the Ovid platform (Ovid Technologies, New York, NY, United States). ClinicalTrials.gov was searched on 25th May 2022; references lists of included studies and related review articles were hand searched for additional studies.
Search strategy
Scoping searches were performed to refine the review question. Final search strategies (Appendix A) were developed and piloted using an iterative process. To maximise sensitivity, no automated search limits were applied. Scottish Intercollegiate Guidelines Network (SIGN) randomised controlled trial (RCT) and observational study search filters were included in the MEDLINE and Embase searches (45). Cochrane RCT search filters were utilised in the Web of Science and Scopus searches (46); the key words from the SIGN MEDLINE observational study filter were used to capture observational studies in Web of Science and Scopus because observational study filters do not exist for these databases. Search sensitivity was evaluated using a list of 8 papers known to meet inclusion criteria: all papers were successfully captured.
Selection process
De-duplication of search results was completed in EndNote (Version 20.3.0.17787, Clarivate, London, United Kingdom) (47). Title and abstract screening were completed using Rayyan (Rayyan Systems Inc., Cambridge, MA, United States). All records were screened in duplicate by 2 blinded reviewers (ODM and JB/MEK/CPS/HB/FB); a pilot of 100 records were screened by all reviewers to ensure concordance. On screening completion, 146 conflicts from 6,738 screening decisions were resolved by discussion. Full-text screening was completed in duplicate (ODM and JB/CPS/MEK).
Data collection
Manual data extraction was completed in duplicate (ODM and JB/CPS/MEK) in Microsoft Excel (Version 16.63, Microsoft 365) using a piloted extraction form.
Data items
Data were sought for any survival outcome. Survival is typically measured as progression-free survival (PFS) and overall survival (OS). PFS is defined as the length of time from randomisation or initiation of treatment to disease progression or death; OS is the length of time from diagnosis or randomisation or initiation of treatment until death (48, 49). Participant and study characteristics for each included study were also sought (Appendix C).
Risk of bias assessment
Risk of bias of included studies was assessed in duplicate (ODM and MEK/JB/CP) using the Joanna Briggs Institute critical appraisal tools checklists (50).
Synthesis methods
Due to the heterogeneity in inclusion criteria, baseline characteristics and interventions of included studies, meta-analysis was not possible. A qualitative synthesis without meta-analysis (SWiM) (51) was therefore conducted. To facilitate synthesis, survival in individual studies was scored on a 4-point scale: associated with survival benefit, appears beneficial, appears unbeneficial and associated with no survival benefit. Only studies reporting an appropriate statistical test comparing survival between an intervention group and a control group were scored as associated with benefit or no benefit. Where no appropriate statistical test was performed, a score of appears beneficial or appears unbeneficial was assigned with reference to established survival data for standard of care therapy (12).
Certainty assessment
Confidence in the body of evidence was assessed using the Grading of Recommendations, Assessment, Development and Evaluations (GRADE) framework (52).
Results
Study selection
A total of 11,218 records were identified from database searching; 102 studies were included in the review (Figure 2).
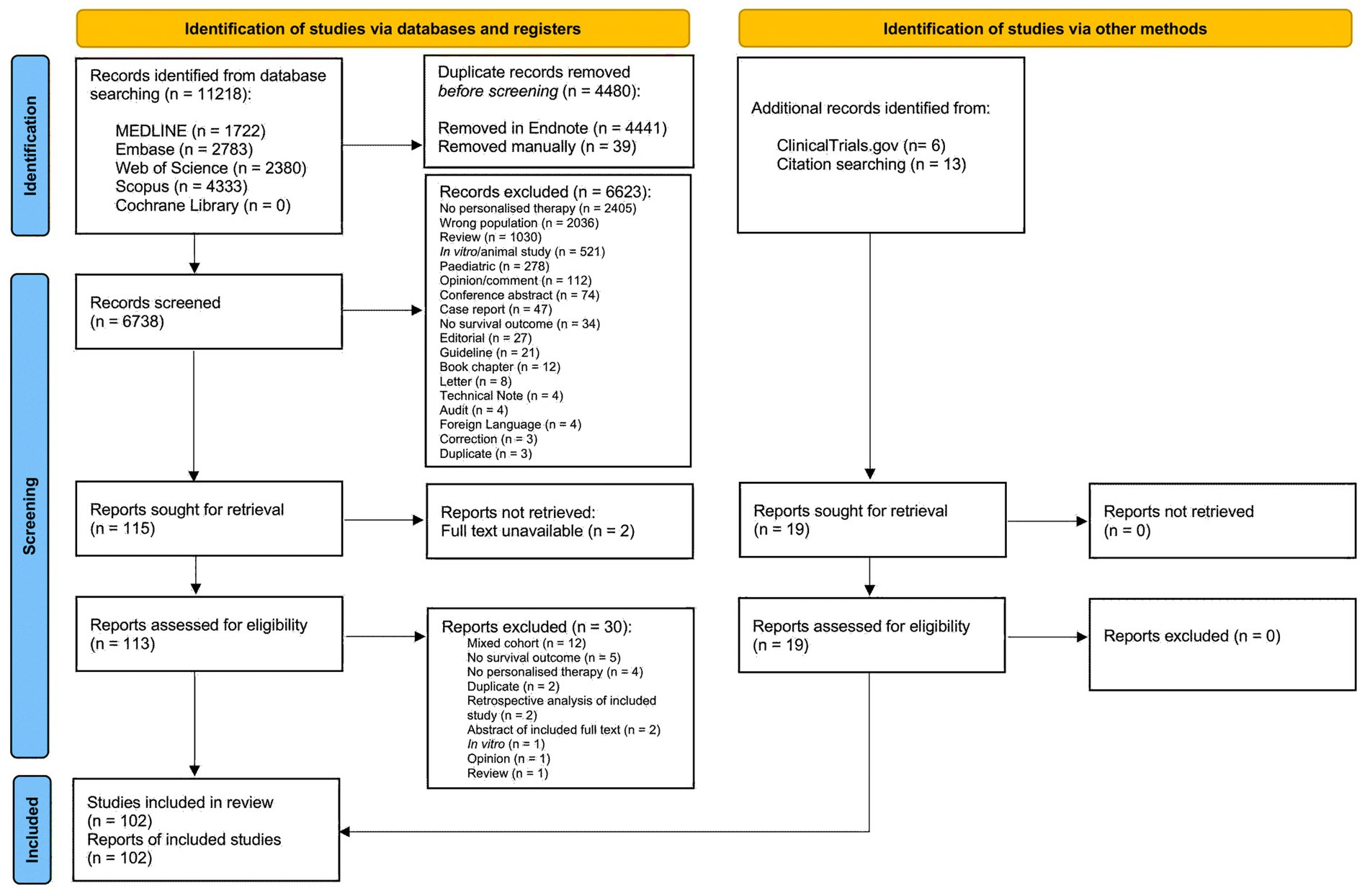
Figure 2. Preferred Reporting Items for Systematic Reviews and Meta-Analyses (PRISMA) flow diagram of study selection. Following the removal of duplicates, titles and abstracts of 6,738 records were screened and 115 studies were sought for retrieval. An additional 19 studies were identified from ClinicalTrials.gov and by hand searching reference lists of included studies and relevant review articles. Reports for 2 studies were not retrievable and 30 studies that initially appeared to meet inclusion criteria were excluded during full text screening (Appendix B). A total of 93 full text articles and 9 conference abstracts were included in the review.
Study characteristics
All included studies were interventional, of which 15.7% (16/102) were randomised trials, 84.3% (86/102) non-randomised trials and 84.3% (86/102) phase I, phase I/II or phase II trials (Figure 3A). Trials were conducted in 20 countries, with 7 international trials (Figure 4). Newly diagnosed patients and patients with recurrent disease were each studied by 40.2% (41/102) of trials (Figure 3B). Seventy-nine trials exclusively studied glioblastoma; 23 trials included glioblastoma within broader cohorts (Supplementary Table 1).
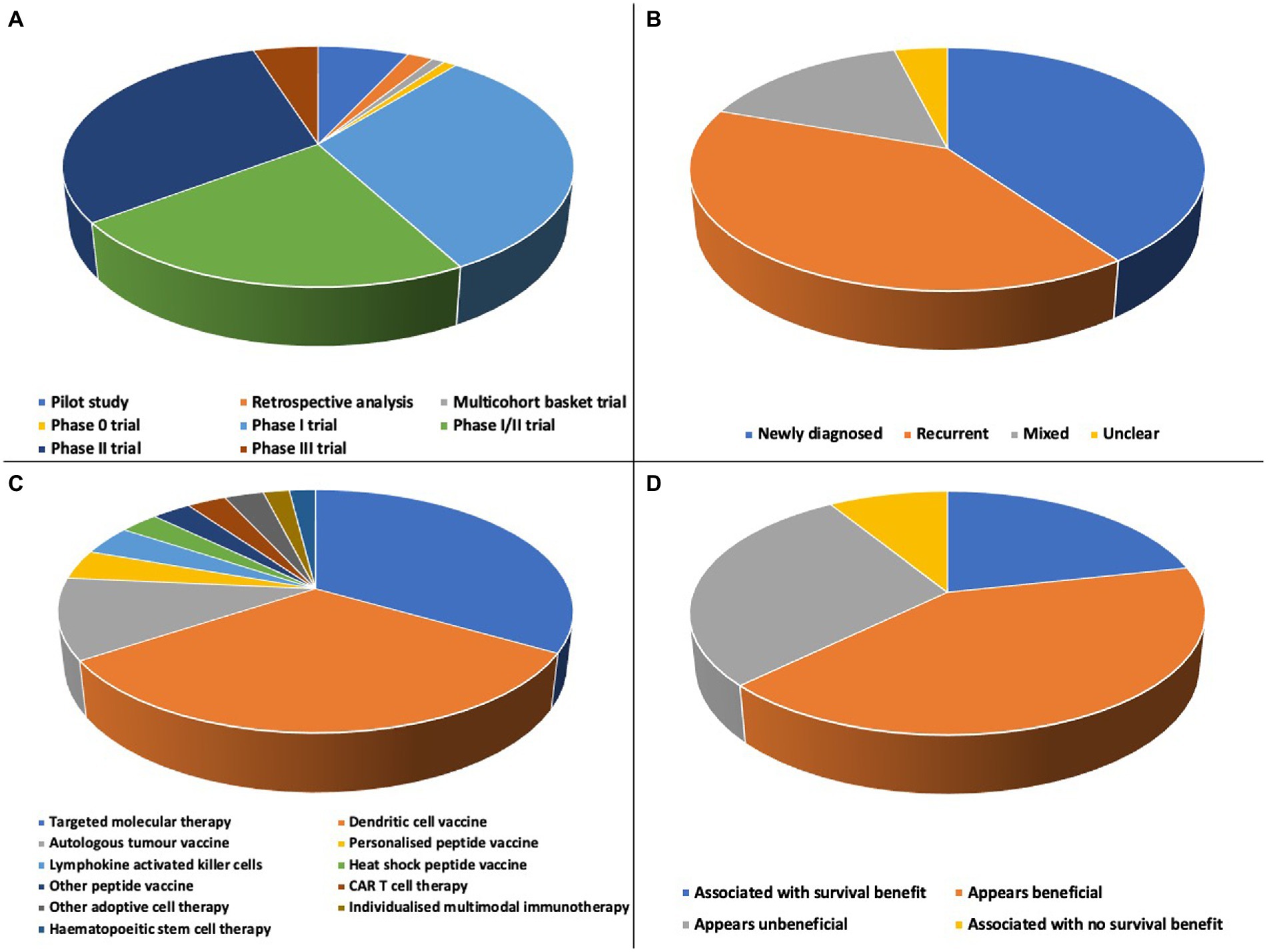
Figure 3. (A) There were a range of designs of included studies: 7 pilot studies, 2 retrospective analyses, 1 multicohort basket trial, 1 phase 0 trial, 32 phase I trials, 23 phase I/II trials, 31 phase II trials and 5 phase III trials. (B) Newly diagnosed and recurrent disease cohorts were each studied by 40.2% of included trials, 15.7% (16/102) included mixed cohorts and the status of patients was unclear for 3.9% (4/102) of trials. (C) More than 20 distinct types of personalised therapy were studied, including a range of targeted molecular therapies and dendritic cell vaccine approaches. (D) Overall survival efficacy of all personalised therapies; in 62.7% (64/102) of studies there was a survival benefit or personalised therapy appeared beneficial.
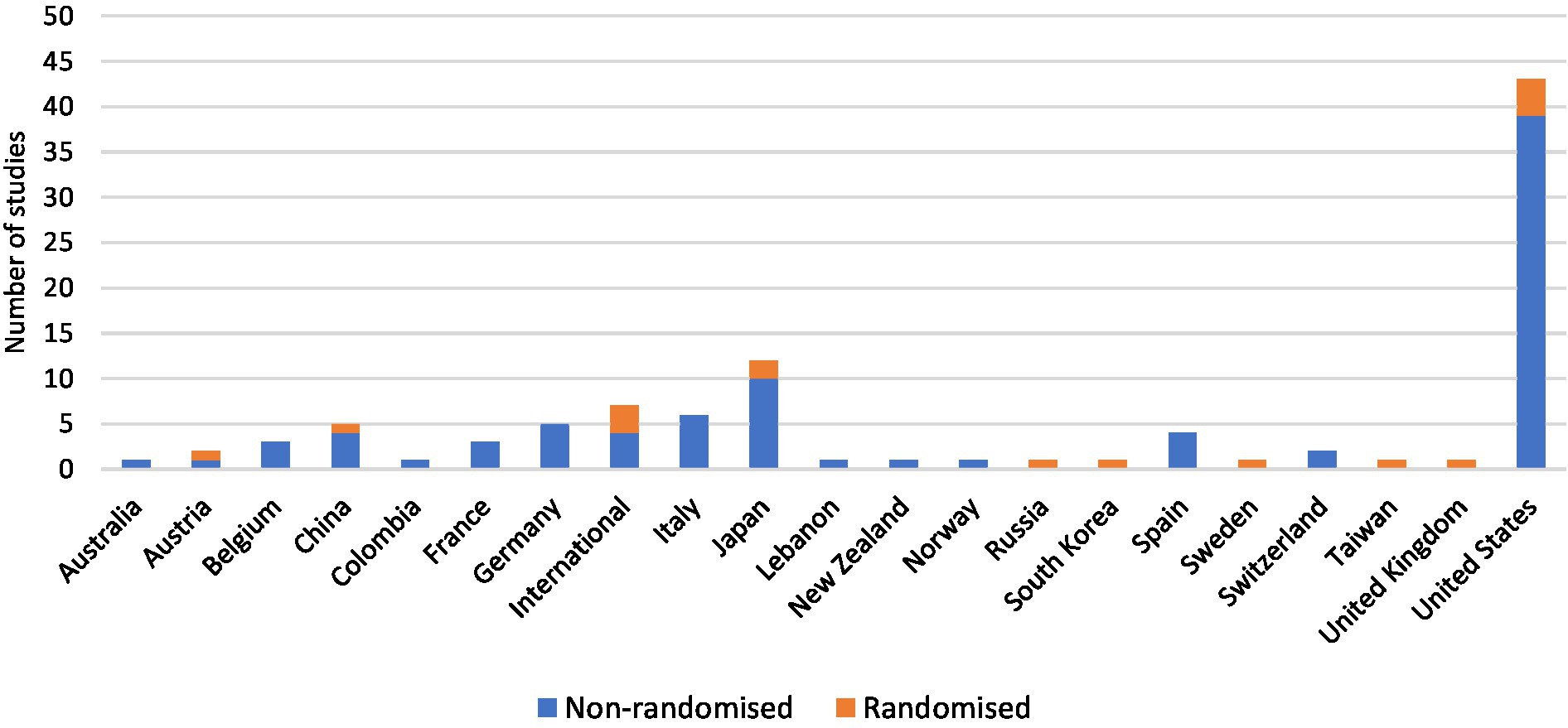
Figure 4. Personalised therapy trials were mostly non-randomised and were conducted in more than 20 countries worldwide. The United States conducted the most trials with a total of 42.2% (43/102), followed by Japan with 11.8% (12/102).
Risk of bias
Randomisation, allocation concealment and intention to treat analysis were poorly reported for randomised trials (Appendix D). For non-randomised trials, the similarity of comparison groups was not always clear, and it was common for studies to lack a control group.
Patient characteristics
A total of 5,527 patients were included (Supplementary Table 2). Ten trials included greater than 100 patients; the mean number of patients per trial was 54. A minority of trials (37.3%, 38/102) included a control group. The mean age of patients included in all trials was 53.7 years and mean Karnofsky Performance Status was 82%. A mean of 59.4% of patients were male. Ethnicity was reported by 9.8% of trials (10/102); a mean of 88.1% of patients were of white ethnicity. A mean of 62.2% of patients had undergone complete surgical resection before personalised therapy; 34.9% had MGMT promoter methylated tumours. Time from diagnosis to personalised therapy ranged from 10 days to greater than 1 year. The mean reported objective response rate was 18.9%.
Survival following personalised therapy
More than 20 types of personalised therapy were studied (Figure 3C; Supplementary Table 3). Targeted molecular therapies were the most studied (33.3%, 34/102) followed by autologous dendritic cell vaccines (32.4%, 33/102) and autologous tumour vaccines (10.8%, 11/102). Reporting of prior and concurrent therapies was inconsistent; all patients had completed at least surgical resection and radiotherapy, with most also receiving concurrent temozolomide chemotherapy.
Targeted molecular therapies
Targets included the epidermal growth factor receptor (EGFR), particularly the EGFRvIII variant, vascular endothelial growth factor receptor (VEGF), MET-kinase, cyclin-dependent kinases 4 and 6 (CDK4/6), phosphatidylinositol 3-kinase (PI3K), and programmed cell death protein 1 (PD1).
The EGFRvIII peptide vaccine rindopepimut was the most studied targeted molecular therapy, including 2 randomised and 4 non-randomised trials. Weller et al. (53) conducted a double-blind, placebo-controlled trial of 745 newly diagnosed patients and found no difference in PFS or OS between treated patients and controls in either ITT analysis or analysis of only patients with minimal residual disease after chemotherapy and radiotherapy. A subsequent double-blind, placebo-controlled, randomised phase II trial of rindopepimut in combination with the anti-VEGF monoclonal antibody bevacizumab in 73 patients with recurrent disease demonstrated a survival advantage in the treated group (54). In addition, rindopepimut was associated with a survival benefit (55, 56) or appeared beneficial (57, 58) in 4 non-randomised trials studying a total of 134 patients.
There were 2 randomised and 3 non-randomised trials of the EGFR monoclonal antibody-drug conjugate Depatuxizumab mafodotin. A randomised double-blind placebo-controlled phase III trial of 639 newly diagnosed patients reported no survival difference between treated and control patients (59); a randomised open-label phase II trial of 260 patients with recurrent disease found prolonged survival in the treated group in long-term follow up analysis but not in primary efficacy analysis at 15 months (60). The 3 non-randomised trials had mixed survival results (61–63).
A total of 9 of the non-rindopepimut/depatuxizumab molecular therapies were also targeted at EGFR (64–72) and a further 3 trials studied other receptor tyrosine kinase inhibitors (73–75). These trials were all non-randomised and had mixed survival results.
Personalised chemotherapy regimens informed by in vitro drug sensitivity testing on autologous resected tumour cells appeared beneficial in 2 non-randomised trials studying a total of 105 patients (76, 77). However, 3 non-randomised trials using genomic-profiling to guide personalised molecular therapy had mixed survival results (78–80).
Dendritic cell vaccines
The most studied vaccine therapies (17%, 17/102) consisted of autologous dendritic cells pulsed with autologous tumour lysates (ATL/DC). There were four reports of ATL/DC randomised trials, the largest of which reported interim data on 331 patients in a double-blind, placebo-controlled cross-over trial in patients with newly diagnosed glioblastoma (81). This trial reported mOS of 23.1 months and described a subgroup consisting of 30% of the ITT population that showed an extended mOS of 40.5 months that could not be explained by known prognostic factors. Furthermore, Cho et al. (82) conducted a randomised phase II trial of 34 patients, reporting significantly prolonged OS in a group of patients receiving ten subcutaneous ATL/DC vaccinations in addition to standard of care therapy compared to a control group receiving standard of care therapy alone. However, two other randomised-controlled phase II studies on 76 and 58 patients found no survival benefit associated with ATL/DC (83, 84). A total of 13 non-randomised trials had mixed results with 11 in favour (85–95) and two unsupportive (96, 97) of a survival benefit of ATL/DC.
In addition, three non-randomised trials studying vaccination with autologous tumour peptide pulsed DCs in a total of 198 patients reported significantly improved survival in treated patients (98, 99) or survival that appeared to be beneficial (100). Two trials, including a randomised trial by Mitchell et al. (101, 102), studied vaccination with cytomegalovirus phosphoprotein 65 RNA pulsed DCs, both reporting a significant association between therapy and prolonged survival. Two non-randomised trials studied vaccines consisting of DCs pulsed with mRNA from tumour stem cells in a total of 105 patients; median PFS was 2.9 times longer in vaccinated newly diagnosed patients compared to controls (103), however there was no survival benefit demonstrated in patients with recurrent disease (104).
Furthermore, a randomised double-blind placebo-controlled phase II trial of DCs pulsed with synthetic peptide epitopes targeting glioblastoma tumour and stem-cell associated antigens (ICT-107) included 124 patients and found prolonged PFS but not OS in treated patients compared to controls (105). An earlier non-randomised trial of ICT-107 in 16 patients reported an association between expression of target antigens and significantly prolonged PFS and OS in newly diagnosed patients (106).
Other DC vaccine approaches included pulsing autologous DCs with antigens including glioma stem cell like antigens (107), personalised mRNA tumour associated antigens (108) and allogeneic cells from glioma cell lines (109). Efficacy ranged from being associated with a survival benefit (107), to appearing beneficial (108, 110, 111), to appearing unbeneficial (109, 112, 113).
Other vaccines
The overall effect on survival was unclear across 2 randomised and 9 non-randomised trials of autologous tumour vaccines. Neither randomised trial was able to demonstrate a difference between treated patient and controls, including a double-blind placebo-controlled phase IIb/III trial of 60 newly diagnosed patients (114). However, a non-randomised phase I/II trial of 110 newly diagnosed patients in which tumour cells were infected with Newcastle Disease Virus before vaccination reported significantly longer PFS and OS in vaccinated patients compared to controls (115).
In single non-randomised vaccine trials, Wilms tumour 1 peptide (116), survivin peptide (117) and 9 HLA-A2 restricted peptides eluted from the surface of glioblastoma samples combined with 2 HLA-class II-binding peptides (118) all appeared beneficial for survival. However, four trials of personalised peptide vaccines (119–122), 1 of which was randomised, and 3 non-randomised trials of autologous heat shock protein peptide complex-96 (123–125) had overall mixed survival results.
Other immunotherapies
Overall, there were conflicting survival results for adoptive cell therapies. A randomised open-label phase III trial of intravenous autologous cytokine-induced killer cells in 180 patients reported prolonged PFS but not OS in treated patients (126); a randomised phase II trial of autologous lymphoid effector cells specific against tumour (ALECSAT) in 62 patients found no significant survival difference between treated and control patients (127). A further non-randomised phase I study conducted by Smith et al. (128) reported favourable survival in 25 patients treated with autologous cytokine-specific T cells; patients treated before recurrence had significantly improved OS than patients who had progressed. In addition, 3 non-randomised trials of chimeric antigen receptor (CAR) T cell therapy, two of which were directed towards EGFRvIII, appeared unbeneficial for survival (129–131).
Two non-randomised trials of haematopoietic stem cell therapies studying a total of 10 patients reported results that appeared beneficial (132, 133), whilst 4 non-randomised trials of lymphokine activated killer cells, 3 of which studied patients with recurrent disease, had mixed survival results (134–137). Finally, individualised multimodal immunotherapy (IMI) appeared beneficial when studied in 164 patients across 2 non-randomised trials conducted by van Gool et al. One trial studied newly diagnosed patients and the other a mixed population (138, 139). The trial in newly diagnosed patients reported significantly prolonged survival in patients treated with IMI and concurrent temozolomide compared to IMI alone (139).
Reporting bias
A total of 93.1% (95/102) of studies reported OS, 71.6% (73/102) reported PFS and 58.8% (60/102) reported at least 1 additional survival outcome. Additional survival outcomes were reported at time points that differed between studies. Moreover, it was not uncommon for a survival outcome specified amongst a priori endpoints not to be reported.
Certainty of evidence
Certainty of the body of evidence was low for all therapies (Table 1). There was a high risk of bias due to most trials being small and non-randomised. Associations between therapies and survival were frequently conflicting between trials, including between the highest quality randomised trials.
Discussion
Summary of main findings
The objective of this systematic review was to synthesise evidence on the survival efficacy of personalised therapies in glioblastoma. Most studies reported either a survival benefit or survival that appeared beneficial following a personalised therapy (Figure 3D), however there was no consistent high-quality evidence of efficacy for any individual personalised therapy.
Context of findings
Central nervous system tumours have historically been characterised using histological features such hypercellularity, nuclear atypia and necrosis (140). Nomenclature has evolved with each iteration of the WHO classification, driven by increasingly precise molecular characterisation (5). This is revealing increasing heterogeneity of tumour types in historical trials. Molecularly distinct tumours may require distinct therapeutic approaches. Therefore, the utility of past trials may diminish with time, with heterogeneity likely contributing to lack of efficacy in non-molecularly defined populations, specifically that associated with IDH mutation and the inclusion of patients classified, currently, as astrocytoma grade 4. This is to some extent circumvented in this review, which studied only molecularly selected patient cohorts and excluded therapies applied in a non-personalised manner.
Personalised therapy should target unique features of each patient’s disease. Included trials mostly considered single agents, which is problematic given a single molecular difference is highly unlikely to define tumour identity. Furthermore, variants do not occur in isolation but affect cellular pathways and networks of molecules, in which there is redundancy and adaptation (20). Therefore, it is unsurprising that single agent efficacy is underwhelming. As profiling of tumours becomes increasingly precise, more detailed characterisation of differences between tumours will follow, including how variants synergise and should be prioritised therapeutically. Only a small number of currently known variants have been targeted, with EGFR a major focus. Combination approaches may offer superior efficacy, with promising pre-clinical and early clinical examples (53, 54, 141, 142). However, more therapeutic options are needed to address already identified targets. Due to its low mutational burden, glioblastoma is an ideal cancer candidate in which to pursue this study.
In addition, a number of trials reported associations of therapy with prolonged PFS but not OS (103, 105, 126). PFS and OS are strongly correlated outcomes, with PFS offering earlier assessment and higher statistical power at time of analysis (143). However, progression is technically challenging to decipher from pseudoprogression, a not infrequent response following chemoradiotherapy or immunotherapy in which a tumour initially increases in size or new lesions appear (144). PFS is therefore less reproducible, reflected in many trials mandating centralised assessment of progression (145).
Limitations of included studies
Heterogenous measurement and reporting of survival
Overall, survival measurements were poorly described, limiting confidence in comparisons. A total of 49% of trials did not specify how survival was measured and those that did reported various start points including diagnosis, recurrence and commencement of therapy. The majority reported median survival, whilst others reported mean values; rates of OS and PFS were quoted at unstandardised time intervals. Furthermore, whilst PFS and TTP are strictly distinct measures (146), this terminology appeared to be used interchangeably by some included trials (64, 77).
Quality of evidence
The overall quality of evidence is low. Many trials were small, non-randomised and single arm. Whilst early phase trials and proof of principle studies have value in assessing safety and feasibility, they are not powered for survival analysis, selection criteria often limit generalisability and patients are exposed to unstandardised schedules and doses of therapy. Survival reported by these trials should therefore be interpreted cautiously. Furthermore, trials included many uncontrolled variables including surgical factors, past chemotherapy drugs and doses and radiotherapy regime before and after personalised therapy. In addition, many trials used historical control groups, which provide a convenient comparison but with higher risk of confounding.
Methodological challenges
The focus of trials was on short-term survival rates, in the context of a disease that has very low rates of long-term survival. However, it is becoming clear that survival efficacy of several immunotherapies may lie in a small subgroup of long-term survivors (81). Greater focus on this subgroup may yield insight into factors driving survival efficacy to improve future therapy personalisation.
Furthermore, it was not uncommon for patients to be excluded from trials before commencing therapy due to progression (112, 147, 148). This was particularly true for vaccine therapies, which typically took up to 8 weeks to manufacture.
Standardisation needed to aid synthesis and promote reproducibility
Personalised glioblastoma trials would benefit from definition of core data elements, measurement and outcome sets, as significant heterogeneity exists which limits comparability and synthesis (149). For example, performance status was inconsistently reported using KPS, WHO and ECOG scales and for many datapoints there was inconsistency in whether mean, median or modal values were reported. The terms newly diagnosed and recurrent were utilised in this review to address inconsistent use of the word “primary,” referring to either newly diagnosed or arising de novo without evolution from a lower grade glioma (128, 137, 150, 151). In addition, there was little consensus on the definition of complete resection, varying between 90, 95 and 99% (55, 77, 113, 152).
Furthermore, there was inconsistency in inclusion criteria, with some trials stating a focus on glioblastoma but also including anaplastic astrocytoma patients (153) and other trials studying broader cohorts of glioma (64, 123, 154). In addition, most DC vaccine trials had highly precise administration schedules, for which the rationale was often unclear. Others had limited descriptions of the schedule, hindering replicability and reproducibility. Time from diagnosis to commencement of personalised therapy was often not specified or highly variable within and between studies. Furthermore, there was inconsistency in response rate calculations; standardisation in objective response rate definition outlined by the Revised Assessment in Neuro-Oncology (RANO) criteria is therefore welcomed (155).
Generalisability
The generalisability of many included trials is limited due to included patients being highly and heterogeneously pre-treated with other therapies. Failure of a personalised therapy in a trial of last line therapy in highly treated patients with recurrent disease should not be extrapolated beyond this context.
Implications and future directions
Targeted and personalised therapy are not synonymous: most trials of targeted therapies have not used target-enrichment criteria and were consequently excluded. Comprehensive molecular characterisation is needed to identify personalised spectra of targetable molecular alterations, with next generational sequencing panels of utility (156, 157). Future efficacy trials should only be studied in a population proven to express the target of interest and employ combination approaches to overcome redundancy. Furthermore, glioblastoma is a moving target; 60% of tumours expressing EGFRvIII at primary resection lose expression by recurrence (54). Genomic instability and immune evasion appear to be driven by complex interactions encompassing epigenetic changes, metabolic reprogramming and oxidative stress responses within the tumour microenvironment (158). Therefore, methods to monitor genomic heterogeneity will be essential to tailor therapy, with non-invasive strategies most favourable (Figure 1C) (34). In addition, methods to improve delivery across the blood brain barrier, such as convection enhanced delivery (159) and nanoparticle drug delivery systems (160), may catalyse the success of personalised therapies.
Finally, differences in allele frequencies between populations are well-described, as is differing glioblastoma prevalence. Unfortunately, only 10% of included studies considered ethnicity; future work should do so as differing genomic profiles between populations may prove another important consideration in personalisation of therapy.
Conclusion
Personalised therapy remains of unproven benefit to survival for patients with glioblastoma, with no therapy currently ready for routine clinical application. Nonetheless, encouraging results in some trials provide reason for optimism. Future advances in this field may depend on target-enriched trials, dynamic combination therapies with longitudinal monitoring of genomic biomarkers and standardised reporting in clinical trials.
Data availability statement
The original contributions presented in the study are included in the article/Supplementary material, further inquiries can be directed to the corresponding author.
Author contributions
OM and RM: conceptualisation and design, search strategy, and data interpretation. OM, JB, MEK, CS, HB, and FB: screening and data extraction. OM: manuscript drafting. OM, JB, MEK, CS, HB, FB, and RM: manuscript revision. RM: supervision. All authors contributed to the article and approved the submitted version.
Funding
Research in RM’s laboratory is jointly funded by Cancer Research UK and the University of Cambridge. OM is supported by an NIHR Academic Clinical Fellowship at the University of Cambridge.
Acknowledgments
The authors are grateful to Isla Kuhn (IK) and Veronica Philips (VP), medical librarians at the University of Cambridge, for reviewing and providing critique on the database search strategies.
Conflict of interest
The authors declare that the research was conducted in the absence of any commercial or financial relationships that could be construed as a potential conflict of interest.
Publisher’s note
All claims expressed in this article are solely those of the authors and do not necessarily represent those of their affiliated organizations, or those of the publisher, the editors and the reviewers. Any product that may be evaluated in this article, or claim that may be made by its manufacturer, is not guaranteed or endorsed by the publisher.
Supplementary material
The Supplementary material for this article can be found online at: https://www.frontiersin.org/articles/10.3389/fmed.2023.1166104/full#supplementary-material
References
1.Rønning, PA, Helseth, E, Meling, TR, and Johannesen, TB. A population-based study on the effect of temozolomide in the treatment of glioblastoma multiforme. Neuro-Oncology. (2012) 14:1178–84. doi: 10.1093/neuonc/nos153
2.Tamimi, AF, and Juweid, M. Epidemiology and outcome of glioblastoma. In: S VleeschouwerDe, (ed.) Glioblastoma [Internet]. Brisbane (AU): Codon Publications; (2017).
3.Ostrom, QT, Gittleman, H, Truitt, G, Boscia, A, Kruchko, C, and Barnholtz-Sloan, JS. CBTRUS statistical report: primary brain and other central nervous system Tumors diagnosed in the United States in 2011-2015. Neuro-Oncology. (2018) 20:iv1–iv86. doi: 10.1093/neuonc/noy131
4.Tan, AC, Ashley, DM, López, GY, Malinzak, M, Friedman, HS, and Khasraw, M. Management of glioblastoma: state of the art and future directions. CA Cancer J Clin. (2020) 70:299–312. doi: 10.3322/caac.21613
5.Louis, DN, Perry, A, Wesseling, P, Brat, DJ, Cree, IA, Figarella-Branger, D, et al. The 2021 WHO classification of Tumors of the central nervous system: a summary. Neuro-Oncology. (2021) 23:1231–51. doi: 10.1093/neuonc/noab106
6.Kanu, OO, Hughes, B, Di, C, Lin, N, Fu, J, Bigner, DD, et al. Glioblastoma multiforme Oncogenomics and Signaling pathways. Clin Med Oncol. (2009) 3:39–52. doi: 10.4137/cmo.s1008
7.Fisher, JL, Schwartzbaum, JA, Wrensch, M, and Wiemels, JL. Epidemiology of brain tumors. Neurol Clin. (2007) 25:867–90, vii. doi: 10.1016/j.ncl.2007.07.002
8.Hamilton, SR, Liu, B, Parsons, RE, Papadopoulos, N, Jen, J, Powell, SM, et al. The molecular basis of Turcot’s syndrome. N Engl J Med. (1995) 332:839–47. doi: 10.1056/NEJM199503303321302
9.Krex, D, Klink, B, Hartmann, C, von Deimling, A, Pietsch, T, Simon, M, et al. Long-term survival with glioblastoma multiforme. Brain. (2007) 130:2596–606. doi: 10.1093/brain/awm204
10.Li, YM, Suki, D, Hess, K, and Sawaya, R. The influence of maximum safe resection of glioblastoma on survival in 1229 patients: can we do better than gross-total resection? J Neurosurg. (2016) 124:977–88. doi: 10.3171/2015.5.JNS142087
11.Allahdini, F, Amirjamshidi, A, Reza-Zarei, M, and Abdollahi, M. Evaluating the prognostic factors effective on the outcome of patients with glioblastoma multiformis: does maximal resection of the tumor lengthen the median survival? World Neurosurg. (2010) 73:128–34. doi: 10.1016/j.wneu.2009.06.001
12.Stupp, R, Mason, WP, van den Bent, MJ, Weller, M, Fisher, B, Taphoorn, MJB, et al. Radiotherapy plus concomitant and adjuvant temozolomide for glioblastoma. N Engl J Med. (2005) 352:987–96. doi: 10.1056/NEJMoa043330
13.Weller, M, Le Rhun, E, Preusser, M, Tonn, JC, and Roth, P. How we treat glioblastoma. ESMO Open. (2019) 4:e000520. doi: 10.1136/esmoopen-2019-000520
14.Stupp, R, Taillibert, S, Kanner, A, Read, W, Steinberg, D, Lhermitte, B, et al. Effect of tumor-treating fields plus maintenance temozolomide vs maintenance temozolomide alone on survival in patients with glioblastoma: a randomized clinical trial. JAMA. (2017) 318:2306–16. doi: 10.1001/jama.2017.18718
15.Goel, NJ, Bird, CE, Hicks, WH, and Abdullah, KG. Economic implications of the modern treatment paradigm of glioblastoma: an analysis of global cost estimates and their utility for cost assessment. J Med Econ. (2021) 24:1018–24. doi: 10.1080/13696998.2021.1964775
16.Ostrom, QT, Gittleman, H, Xu, J, Kromer, C, Wolinsky, Y, Kruchko, C, et al. CBTRUS statistical report: primary brain and other central nervous system Tumors diagnosed in the United States in 2009-2013. Neuro-Oncology. (2016) 18:v1–v75. doi: 10.1093/neuonc/now207
17.Koshy, M, Villano, JL, Dolecek, TA, Howard, A, Mahmood, U, Chmura, SJ, et al. Improved survival time trends for glioblastoma using the SEER 17 population-based registries. J Neuro-Oncol. (2012) 107:207–12. doi: 10.1007/s11060-011-0738-7
18.Burnet, NG, Jefferies, SJ, Benson, RJ, Hunt, DP, and Treasure, FP. Years of life lost (YLL) from cancer is an important measure of population burden — and should be considered when allocating research funds. Br J Cancer. (2005) 92:241–5. doi: 10.1038/sj.bjc.6602321
19.Kelly, PJ, Daumas-Duport, C, Scheithauer, BW, Kall, BA, and Kispert, DB. Stereotactic histologic correlations of computed tomography- and magnetic resonance imaging-defined abnormalities in patients with glial neoplasms. Mayo Clin Proc. (1987) 62:450–9. doi: 10.1016/S0025-6196(12)65470-6
20.Prados, MD, Byron, SA, Tran, NL, Phillips, JJ, Molinaro, AM, Ligon, KL, et al. Toward precision medicine in glioblastoma: the promise and the challenges. Neuro-Oncology. (2015) 17:1051–63. doi: 10.1093/neuonc/nov031
21.Li, J, Di, C, Mattox, AK, Wu, L, and Adamson, DC. The future role of personalized medicine in the treatment of glioblastoma multiforme. Pharmgenomics Pers Med. (2010) 3:111–27. doi: 10.2147/PGPM.S6852
22.Goetz, LH, and Schork, NJ. Personalized medicine: motivation, challenges and Progress. Fertil Steril. (2018) 109:952–63. doi: 10.1016/j.fertnstert.2018.05.006
23.McLendon, R, Friedman, A, Bigner, D, Van Meir, EG, Brat, DJ, Mastrogianakis, GM, et al. Comprehensive genomic characterization defines human glioblastoma genes and core pathways. Nature. (2008) 455:1061–8. doi: 10.1038/nature07385
24.Parsons, DW, Jones, S, Zhang, X, Lin, JCH, Leary, RJ, Angenendt, P, et al. An integrated genomic analysis of human glioblastoma multiforme. Science. (2008) 321:1807–12. doi: 10.1126/science.1164382
25.Frattini, V, Trifonov, V, Chan, JM, Castano, A, Lia, M, Abate, F, et al. The integrated landscape of driver genomic alterations in glioblastoma. Nat Genet. (2013) 45:1141–9. doi: 10.1038/ng.2734
26.Liu, A, Hou, C, Chen, H, Zong, X, and Zong, P. Genetics and epigenetics of glioblastoma: applications and overall incidence of IDH1 mutation. Front Oncol [Internet]. (2016) 6:16. doi: 10.3389/fonc.2016.00016
27.Parker, NR, Khong, P, Parkinson, JF, Howell, VM, and Wheeler, HR. Molecular heterogeneity in glioblastoma: potential clinical implications. Front Oncol [Internet]. (2015) 5:55. doi: 10.3389/fonc.2015.00055
28.Brennan, CW, Verhaak, RGW, McKenna, A, Campos, B, Noushmehr, H, Salama, SR, et al. The somatic genomic landscape of glioblastoma. Cells. (2013) 155:462–77. doi: 10.1016/j.cell.2013.09.034
29.Patel, AP, Tirosh, I, Trombetta, JJ, Shalek, AK, Gillespie, SM, Wakimoto, H, et al. Single-cell RNA-seq highlights intratumoral heterogeneity in primary glioblastoma. Science. (2014) 344:1396–401. doi: 10.1126/science.1254257
30.Snuderl, M, Fazlollahi, L, Le, LP, Nitta, M, Zhelyazkova, BH, Davidson, CJ, et al. Mosaic amplification of multiple receptor tyrosine kinase genes in glioblastoma. Cancer Cell. (2011) 20:810–7. doi: 10.1016/j.ccr.2011.11.005
31.Szerlip, NJ, Pedraza, A, Chakravarty, D, Azim, M, McGuire, J, Fang, Y, et al. Intratumoral heterogeneity of receptor tyrosine kinases EGFR and PDGFRA amplification in glioblastoma defines subpopulations with distinct growth factor response. Proc Natl Acad Sci U S A. (2012) 109:3041–6. doi: 10.1073/pnas.1114033109
32.Johnson, BE, Mazor, T, Hong, C, Barnes, M, Aihara, K, McLean, CY, et al. Mutational analysis reveals the origin and therapy-driven evolution of recurrent glioma. Science. (2014) 343:189–93. doi: 10.1126/science.1239947
33.Mouliere, F, Smith, CG, Heider, K, Su, J, van der Pol, Y, Thompson, M, et al. Fragmentation patterns and personalized sequencing of cell-free DNA in urine and plasma of glioma patients. EMBO Mol Med. (2021) 13:e12881. doi: 10.15252/emmm.202012881
34.Mair, R, and Mouliere, F. Cell-free DNA technologies for the analysis of brain cancer. Br J Cancer. (2022) 126:371–8. doi: 10.1038/s41416-021-01594-5
35.Touat, M, Idbaih, A, Sanson, M, and Ligon, KL. Glioblastoma targeted therapy: updated approaches from recent biological insights. Ann Oncol. (2017) 28:1457–72. doi: 10.1093/annonc/mdx106
36.Sakthikumar, S, Roy, A, Haseeb, L, Pettersson, ME, Sundström, E, Marinescu, VD, et al. Whole-genome sequencing of glioblastoma reveals enrichment of non-coding constraint mutations in known and novel genes. Genome Biol. (2020) 21:127. doi: 10.1186/s13059-020-02035-x
37.Gousias, K, Theocharous, T, and Simon, M. Mechanisms of cell cycle arrest and apoptosis in glioblastoma. Biomedicine. (2022) 10:564. doi: 10.3390/biomedicines10030564
38.Andre, F, Mardis, E, Salm, M, Soria, JC, Siu, LL, and Swanton, C. Prioritizing targets for precision cancer medicine. Ann Oncol. (2014) 25:2295–303. doi: 10.1093/annonc/mdu478
39.Dienstmann, R, Dong, F, Borger, D, Dias-Santagata, D, Ellisen, LW, Le, LP, et al. Standardized decision support in next generation sequencing reports of somatic cancer variants. Mol Oncol. (2014) 8:859–73. doi: 10.1016/j.molonc.2014.03.021
40.Zhao, T, Li, C, Ge, H, Lin, Y, and Kang, D. Glioblastoma vaccine tumor therapy research progress. Chin Neurosurg J. (2022) 8:2. doi: 10.1186/s41016-021-00269-7
41.Kreatsoulas, D, Bolyard, C, Wu, BX, Cam, H, Giglio, P, and Li, Z. Translational landscape of glioblastoma immunotherapy for physicians: guiding clinical practice with basic scientific evidence. J Hematol Oncol. (2022) 15:80. doi: 10.1186/s13045-022-01298-0
42.Frederico, SC, Hancock, JC, Brettschneider, EES, Ratnam, NM, Gilbert, MR, and Terabe, M. Making a cold tumor hot: the role of vaccines in the treatment of glioblastoma. Front Oncol [Internet]. (2021) 11:672508. doi: 10.3389/fonc.2021.672508
43.Page, MJ, McKenzie, JE, Bossuyt, PM, Boutron, I, Hoffmann, TC, Mulrow, CD, et al. The PRISMA 2020 statement: an updated guideline for reporting systematic reviews. BMJ. (2021) 372:n71. doi: 10.1136/bmj.n71
44.Rethlefsen, ML, Kirtley, S, Waffenschmidt, S, Ayala, AP, Moher, D, Page, MJ, et al. PRISMA-S: an extension to the PRISMA statement for reporting literature searches in systematic reviews. Syst Rev. (2021) 10:39. doi: 10.1186/s13643-020-01542-z
45.Scottish Intercollegiate Guidelines Network. Search filters [internet]. SIGN. (2022). Available at: https://www.sign.ac.uk/what-we-do/methodology/search-filters/
46.Higgins, J, Thomas, J, Chandler, J, Cumpston, M, Li, T, Page, M, et al. Cochrane handbook for systematic reviews of interventions version 6.3 (updated February 2022). [internet]. (2022). Available at: https://training.cochrane.org/handbook/current
47.Bramer, WM, Giustini, D, de Jonge, GB, Holland, L, and Bekhuis, T. De-duplication of database search results for systematic reviews in EndNote. J Med Libr Assoc. (2016) 104:240–3. doi: 10.3163/1536-5050.104.3.014
48.Anonymous. Definition of overall survival - NCI dictionary of cancer terms - NCI [internet]. (2011). Available at: https://www.cancer.gov/publications/dictionaries/cancer-terms/def/overall-survival
49.Gyawali, B, Eisenhauer, E, Tregear, M, and Booth, CM. Progression-free survival: it is time for a new name. Lancet Oncol. (2022) 23:328–30. doi: 10.1016/S1470-2045(22)00015-8
50.Tufanaru, C, Munn, Z, Aromataris, E, Campbell, J, and Hopp, L. Chapter 3: systematic reviews of effectiveness In: E Aromataris and Z Munn, editors. JBI Manual for Evidence Synthesis. Adelaide, South Australia, Australia: JBI (2020).
51.Campbell, M, McKenzie, JE, Sowden, A, Katikireddi, SV, Brennan, SE, Ellis, S, et al. Synthesis without meta-analysis (SWiM) in systematic reviews: reporting guideline. BMJ. (2020) 368:l6890. doi: 10.1136/bmj.m549
52.Guyatt, GH, Oxman, AD, Vist, GE, Kunz, R, Falck-Ytter, Y, Alonso-Coello, P, et al. GRADE: an emerging consensus on rating quality of evidence and strength of recommendations. BMJ. (2008) 336:924–6. doi: 10.1136/bmj.39489.470347.AD
53.Weller, M, Butowski, N, Tran, DD, Recht, LD, Lim, M, Hirte, H, et al. Rindopepimut with temozolomide for patients with newly diagnosed, EGFRvIII-expressing glioblastoma (ACT IV): a randomised, double-blind, international phase 3 trial. Lancet Oncol. (2017) 18:1373–85. doi: 10.1016/S1470-2045(17)30517-X
54.Reardon, DA, Desjardins, A, Vredenburgh, JJ, O’Rourke, DM, Tran, DD, Fink, KL, et al. Rindopepimut with bevacizumab for patients with relapsed EGFRvIII-expressing glioblastoma (ReACT): results of a double-blind randomized phase II trial. Clin Cancer Res. (2020) 26:1586–94. doi: 10.1158/1078-0432.CCR-18-1140
55.Sampson, JH, Heimberger, AB, Archer, GE, Aldape, KD, Friedman, AH, Friedman, HS, et al. Immunologic escape after prolonged progression-free survival with epidermal growth factor receptor variant III peptide vaccination in patients with newly diagnosed glioblastoma. J Clin Oncol. (2010) 28:4722–9. doi: 10.1200/JCO.2010.28.6963
56.Sampson, JH, Aldape, KD, Archer, GE, Coan, A, Desjardins, A, Friedman, AH, et al. Greater chemotherapy-induced lymphopenia enhances tumor-specific immune responses that eliminate EGFRvIII-expressing tumor cells in patients with glioblastoma. Neuro-Oncology. (2011) 13:324–33. doi: 10.1093/neuonc/noq157
57.Sampson, JH, Archer, GE, Mitchell, DA, Heimberger, AB, Herndon, JE, Lally-Goss, D, et al. An epidermal growth factor receptor variant III-targeted vaccine is safe and immunogenic in patients with glioblastoma multiforme. Mol Cancer Ther. (2009) 8:2773–9. doi: 10.1158/1535-7163.MCT-09-0124
58.Schuster, J, Lai, RK, Recht, LD, Reardon, DA, Paleologos, NA, Groves, MD, et al. A phase II, multicenter trial of rindopepimut (CDX-110) in newly diagnosed glioblastoma: the ACT III study. Neuro-Oncology. (2015) 17:854–61. doi: 10.1093/neuonc/nou348
59.Lassman, A, Pugh, S, Wang, T, Aldape, K, Gan, H, Preusser, M, et al. ACTR-21. A RAndomized, double-blind, placebo-controlled phase 3 trial of depatuxizumab mafodotin (ABT-414) in epidermal growth factor receptor (EGFR) amplified (AMP) newly diagnosed glioblastoma (nGBM). Neuro-Oncology. (2019) 21:vi17. doi: 10.1093/neuonc/noz175.064
60.Van Den Bent, M, Eoli, M, Sepulveda, JM, Smits, M, Walenkamp, A, Frenel, JS, et al. INTELLANCE 2/EORTC 1410 randomized phase II study of Depatux-M alone and with temozolomide vs temozolomide or lomustine in recurrent EGFR amplified glioblastoma. Neuro-Oncology. (2020) 22:684–93. doi: 10.1093/neuonc/noz222
61.van den Bent, M, Gan, HK, Lassman, AB, Kumthekar, P, Merrell, R, Butowski, N, et al. Efficacy of depatuxizumab mafodotin (ABT-414) monotherapy in patients with EGFR-amplified, recurrent glioblastoma: results from a multi-center, international study. Cancer Chemother Pharmacol. (2017) 80:1209–17. doi: 10.1007/s00280-017-3451-1
62.Lassman, AB, van den Bent, MJ, Gan, HK, Reardon, DA, Kumthekar, P, Butowski, N, et al. Safety and efficacy of depatuxizumab mafodotin + temozolomide in patients with EGFR-amplified, recurrent glioblastoma: results from an international phase I multicenter trial. Neuro-Oncology. (2019) 21:106–14. doi: 10.1093/neuonc/noy091
63.Padovan, M, Eoli, M, Pellerino, A, Rizzato, S, Caserta, C, Simonelli, M, et al. Depatuxizumab Mafodotin (Depatux-M) plus temozolomide in recurrent glioblastoma patients: real-world experience from a Multicenter study of Italian Association of Neuro-Oncology (AINO). Cancers (Basel). (2021) 13:2773. doi: 10.3390/cancers13112773
64.Neyns, B, Sadones, J, Joosens, E, Bouttens, F, Verbeke, L, Baurain, JF, et al. Stratified phase II trial of cetuximab in patients with recurrent high-grade glioma. Ann Oncol. (2009) 20:1596–603. doi: 10.1093/annonc/mdp032
65.Reardon, DA, Groves, MD, Wen, PY, Nabors, L, Mikkelsen, T, Rosenfeld, S, et al. A phase I/II trial of pazopanib in combination with lapatinib in adult patients with relapsed malignant glioma. Clin Cancer Res. (2013) 19:900–8. doi: 10.1158/1078-0432.CCR-12-1707
66.D’Alessandris, QG, Montano, N, Cenci, T, Martini, M, Lauretti, L, Bianchi, F, et al. Targeted therapy with bevacizumab and erlotinib tailored to the molecular profile of patients with recurrent glioblastoma. Preliminary experience. Acta Neurochir (Wien). (2013) 155:33–40. doi: 10.1007/s00701-012-1536-5
67.Gallego, O, Cuatrecasas, M, Benavides, M, Segura, PP, Berrocal, A, Erill, N, et al. Efficacy of erlotinib in patients with relapsed gliobastoma multiforme who expressed EGFRVIII and PTEN determined by immunohistochemistry. J Neuro-Oncol. (2014) 116:413–9. doi: 10.1007/s11060-013-1316-y
68.Sepúlveda-Sánchez, JM, Vaz, MÁ, Balañá, C, Gil-Gil, M, Reynés, G, Gallego, Ó, et al. Phase II trial of dacomitinib, a pan-human EGFR tyrosine kinase inhibitor, in recurrent glioblastoma patients with EGFR amplification. Neuro-Oncology. (2017) 19:1522–31. doi: 10.1093/neuonc/nox105
69.Chi, AS, Cahill, DP, Reardon, DA, Wen, PY, Mikkelsen, T, Peereboom, DM, et al. Exploring predictors of response to dacomitinib in EGFR-amplified recurrent glioblastoma. J Clin Oncol Precision Oncol. (2020) 4:PO.19.00295. doi: 10.1200/PO.19.00295
70.Du, XJ, Li, XM, Cai, LB, Sun, JC, Wang, SY, Wang, XC, et al. Efficacy and safety of nimotuzumab in addition to radiotherapy and temozolomide for cerebral glioblastoma: a phase II multicenter clinical trial. J Cancer. (2019) 10:3214–23. doi: 10.7150/jca.30123
71.Cardona, AF, Jaramillo-Velásquez, D, Ruiz-Patiño, A, Polo, C, Jiménez, E, Hakim, F, et al. Efficacy of osimertinib plus bevacizumab in glioblastoma patients with simultaneous EGFR amplification and EGFRvIII mutation. J Neuro-Oncol. (2021) 154:353–64. doi: 10.1007/s11060-021-03834-3
72.Kasenda, B, König, D, Manni, M, Ritschard, R, Duthaler, U, Bartoszek, E, et al. Targeting immunoliposomes to EGFR-positive glioblastoma. ESMO Open. (2022) 7:100365. doi: 10.1016/j.esmoop.2021.100365
73.Hassler, MR, Vedadinejad, M, Flechl, B, Haberler, C, Preusser, M, Hainfellner, JA, et al. Response to imatinib as a function of target kinase expression in recurrent glioblastoma. Springerplus. (2014) 3:111. doi: 10.1186/2193-1801-3-111
74.Lassman, AB, Pugh, SL, Gilbert, MR, Aldape, KD, Geinoz, S, Beumer, JH, et al. Phase 2 trial of dasatinib in target-selected patients with recurrent glioblastoma (RTOG 0627). Neuro-Oncology. (2015) 17:992–8. doi: 10.1093/neuonc/nov011
75.Hu, H, Mu, Q, Bao, Z, Chen, Y, Liu, Y, Chen, J, et al. Mutational landscape of secondary glioblastoma guides MET-targeted trial in brain tumor. Cells. (2018) 175:1665–1678.e18. doi: 10.1016/j.cell.2018.09.038
76.Iwadate, Y, Fujimoto, S, Namba, H, and Yamaura, A. Promising survival for patients with glioblastoma multiforme treated with individualised chemotherapy based on in vitro drug sensitivity testing. Br J Cancer. (2003) 89:1896–900. doi: 10.1038/sj.bjc.6601376
77.Iwadate, Y, Matsutani, T, Hasegawa, Y, Shinozaki, N, Oide, T, Tanizawa, T, et al. Selection of chemotherapy for glioblastoma expressing O(6)-methylguanine-DNA methyltransferase. Exp Ther Med. (2010) 1:53–7. doi: 10.3892/etm_00000009
78.D’Alessandris, GQ, Montano, N, Martini, M, Cenci, T, Di Bonaventura, R, Giordano, M, et al. Prospective selection of recurrent glioblastoma patients for tailored therapies. Results of an institutional experience. Neuro-Oncology. (2019) 21:vi138. doi: 10.1093/neuonc/noz175.580
79.Kessler, T, Berberich, A, Casalini, B, Drüschler, K, Ostermann, H, Dormann, A, et al. Molecular profiling-based decision for targeted therapies in IDH wild-type glioblastoma. Neurooncol Adv. (2020) 2:vdz060. doi: 10.1093/noajnl/vdz060
80.Bonneville-Levard, A, Frappaz, D, Tredan, O, Lavergne, E, Corset, V, Agrapart, V, et al. Molecular profile to guide personalized medicine in adult patients with primary brain tumors: results from the ProfiLER trial. Med Oncol. (2021) 39:4. doi: 10.1007/s12032-021-01536-4
81.Liau, LM, Ashkan, K, Tran, DD, Campian, JL, Trusheim, JE, Cobbs, CS, et al. First results on survival from a large phase 3 clinical trial of an autologous dendritic cell vaccine in newly diagnosed glioblastoma. J Transl Med. (2018) 16:142. doi: 10.1186/s12967-018-1507-6
82.Cho, DY, Yang, WK, Lee, HC, Hsu, DM, Lin, HL, Lin, SZ, et al. Adjuvant immunotherapy with whole-cell lysate dendritic cells vaccine for glioblastoma multiforme: a phase II clinical trial. World Neurosurg. (2012) 77:736–44. doi: 10.1016/j.wneu.2011.08.020
83.Buchroithner, J, Erhart, F, Pichler, J, Widhalm, G, Preusser, M, Stockhammer, G, et al. Audencel immunotherapy based on dendritic cells has no effect on overall and progression-free survival in newly diagnosed glioblastoma: a phase II randomized trial. Cancers (Basel) (2018);10:???, doi: 10.3390/cancers10100372
84.Mishinov, SV, Budnik, AY, Stupak, VV, Leplina, OY, Tyrinova, TV, Ostanin, AA, et al. Autologous and pooled tumor lysates in combined immunotherapy of patients with glioblastoma. Sovrem Tekhnologii Med. (2020) 12:34–41. doi: 10.17691/stm2020.12.2.04
85.Ardon, H, Van Gool, SW, Verschuere, T, Maes, W, Fieuws, S, Sciot, R, et al. Integration of autologous dendritic cell-based immunotherapy in the standard of care treatment for patients with newly diagnosed glioblastoma: results of the HGG-2006 phase I/II trial. Cancer Immunol Immunother. (2012) 61:2033–44. doi: 10.1007/s00262-012-1261-1
86.Wheeler, CJ, Black, KL, Liu, G, Mazer, M, Xue, ZX, Pepkowitz, S, et al. Vaccination elicits correlated immune and clinical responses in glioblastoma multiforme patients. Cancer Res. (2008) 68:5955–64. doi: 10.1158/0008-5472.CAN-07-5973
87.Inoges, S, Tejada, S, de Cerio, A, Perez-Larraya, J, Espinos, J, Idoate, M, et al. A phase II trial of autologous dendritic cell vaccination and radiochemotherapy following fluorescence-guided surgery in newly diagnosed glioblastoma patients. J Transl Med. (2017) 15:104. doi: 10.1186/s12967-017-1202-z
88.Pellegatta, S, Eoli, M, Cuccarini, V, Anghileri, E, Pollo, B, Pessina, S, et al. Survival gain in glioblastoma patients treated with dendritic cell immunotherapy is associated with increased NK but not CD8+ T cell activation in the presence of adjuvant temozolomide. Onco Targets Ther. (2018) 7:e1412901. doi: 10.1080/2162402X.2017.1412901
89.Prins, RM, Soto, H, Konkankit, V, Odesa, SK, Eskin, A, Yong, WH, et al. Gene expression profile correlates with T-cell infiltration and relative survival in glioblastoma patients vaccinated with dendritic cell immunotherapy. Clin Cancer Res. (2011) 17:1603–15. doi: 10.1158/1078-0432.CCR-10-2563
90.Ardon, H, Van Gool, S, Lopes, IS, Maes, W, Sciot, R, Wilms, G, et al. Integration of autologous dendritic cell-based immunotherapy in the primary treatment for patients with newly diagnosed glioblastoma multiforme: a pilot study. J Neuro-Oncol. (2010) 99:261–72. doi: 10.1007/s11060-010-0131-y
91.Yamanaka, R, Abe, T, Yajima, N, Tsuchiya, N, Homma, J, Kobayashi, T, et al. Vaccination of recurrent glioma patients with tumour lysate-pulsed dendritic cells elicits immune responses: results of a clinical phase I/II trial. Br J Cancer. (2003) 89:1172–9. doi: 10.1038/sj.bjc.6601268
92.Yamanaka, R, Homma, J, Yajima, N, Tsuchiya, N, Sano, M, Kobayashi, T, et al. Clinical evaluation of dendritic cell vaccination for patients with recurrent glioma: results of a clinical phase I/II trial. Clin Cancer Res. (2005) 11:4160–7. doi: 10.1158/1078-0432.CCR-05-0120
93.Yu, JS, Liu, G, Ying, H, Yong, WH, Black, KL, and Wheeler, CJ. Vaccination with tumor lysate-pulsed dendritic cells elicits antigen-specific, cytotoxic T-cells in patients with malignant glioma. Cancer Res. (2004) 64:4973–9. doi: 10.1158/0008-5472.CAN-03-3505
94.Fadul, CE, Fisher, JL, Hampton, TH, Lallana, EC, Li, Z, Gui, J, et al. Immune response in patients with newly diagnosed glioblastoma multiforme treated with intranodal autologous tumor lysate-dendritic cell vaccination after radiation chemotherapy. J Immunother. (2011) 34:382–9. doi: 10.1097/CJI.0b013e318215e300
95.Valle, RD, de Cerio, ALD, Inoges, S, Tejada, S, Pastor, F, Villanueva, H, et al. Dendritic cell vaccination in glioblastoma after fluorescence-guided resection. World J Clin Oncol. (2012) 3:142–9. doi: 10.5306/wjco.v3.i11.142
96.Pellegatta, S, Eoli, M, Frigerio, S, Antozzi, C, Bruzzone, MG, Cantini, G, et al. The natural killer cell response and tumor debulking are associated with prolonged survival in recurrent glioblastoma patients receiving dendritic cells loaded with autologous tumor lysates. Onco Targets Ther. (2013) 2:e23401. doi: 10.4161/onci.23401
97.Rudnick, JD, Sarmiento, JM, Uy, B, Nuno, M, Wheeler, CJ, Mazer, MJ, et al. A phase I trial of surgical resection with Gliadel wafer placement followed by vaccination with dendritic cells pulsed with tumor lysate for patients with malignant glioma. J Clin Neurosci. (2020) 74:187–93. doi: 10.1016/j.jocn.2020.03.006
98.Liau, LM, Prins, RM, Kiertscher, SM, Odesa, SK, Kremen, TJ, Giovannone, AJ, et al. Dendritic cell vaccination in glioblastoma patients induces systemic and intracranial T-cell responses modulated by the local central nervous system tumor microenvironment. Clin Cancer Res. (2005) 11:5515–25. doi: 10.1158/1078-0432.CCR-05-0464
99.Wheeler, CJ, Das, A, Liu, G, Yu, JS, and Black, KL. Clinical responsiveness of glioblastoma multiforme to chemotherapy after vaccination. Clin Cancer Res. (2004) 10:5316–26. doi: 10.1158/1078-0432.CCR-04-0497
100.Yu, JS, Wheeler, CJ, Zeltzer, PM, Ying, H, Finger, DN, Lee, PK, et al. Vaccination of malignant glioma patients with peptide-pulsed dendritic cells elicits systemic cytotoxicity and intracranial T-cell infiltration. Cancer Res. (2001) 61:842–7.
101.Mitchell, DA, Batich, KA, Gunn, MD, Huang, MN, Sanchez-Perez, L, Nair, SK, et al. Tetanus toxoid and CCL3 improve dendritic cell vaccines in mice and glioblastoma patients. Nature. (2015) 519:366–9. doi: 10.1038/nature14320
102.Batich, KA, Reap, EA, Archer, GE, Sanchez-Perez, L, Nair, SK, Schmittling, RJ, et al. Long-term survival in glioblastoma with cytomegalovirus pp65-targeted vaccination. Clin Cancer Res. (2017) 23:1898–909. doi: 10.1158/1078-0432.CCR-16-2057
103.Vik-Mo, EO, Nyakas, M, Mikkelsen, BV, Moe, MC, Due-Tønnesen, P, Suso, EMI, et al. Therapeutic vaccination against autologous cancer stem cells with mRNA-transfected dendritic cells in patients with glioblastoma. Cancer Immunol Immunother. (2013) 62:1499–509. doi: 10.1007/s00262-013-1453-3
104.Dunn-Pirio, A, Peters, K, DesJardins, A, Randazzo, D, Friedman, H, Healy, P, et al. Tumor stem cell RNA-loaded dendritic cell vaccine for recurrent glioblastoma: a phase 1 trial (S41.004). Neurology. (2017) 88:S41–S004.
105.Wen, PY, Reardon, DA, Armstrong, TS, Phuphanich, S, Aiken, RD, Landolfi, JC, et al. A randomized double-blind placebo-controlled phase II trial of dendritic cell vaccine ICT-107 in newly diagnosed patients with glioblastoma. Clin Cancer Res. (2019) 25:5799–807. doi: 10.1158/1078-0432.CCR-19-0261
106.Phuphanich, S, Wheeler, CJ, Rudnick, JD, Mazer, M, Wang, H, Nuño, MA, et al. Phase I trial of a multi-epitope-pulsed dendritic cell vaccine for patients with newly diagnosed glioblastoma. Cancer Immunol Immunother. (2013) 62:125–35. doi: 10.1007/s00262-012-1319-0
107.Yao, Y, Luo, F, Tang, C, Chen, D, Qin, Z, Hua, W, et al. Molecular subgroups and B7-H4 expression levels predict responses to dendritic cell vaccines in glioblastoma: an exploratory randomized phase II clinical trial. Cancer Immunol Immunother. (2018) 67:1777–88. doi: 10.1007/s00262-018-2232-y
108.Wang, QT, Nie, Y, Sun, SN, Lin, T, Han, RJ, Jiang, J, et al. Tumor-associated antigen-based personalized dendritic cell vaccine in solid tumor patients. Cancer Immunol Immunother. (2020) 69:1375–87. doi: 10.1007/s00262-020-02496-w
109.Olin, MR, Low, W, McKenna, DH, Haines, SJ, Dahlheimer, T, Nascene, D, et al. Vaccination with dendritic cells loaded with allogeneic brain tumor cells for recurrent malignant brain tumors induces a CD4(+)IL17(+) response. J Immunother Cancer. (2014) 2:4. doi: 10.1186/2051-1426-2-4
110.Akasaki, Y, Kikuchi, T, Homma, S, Koido, S, Ohkusa, T, Tasaki, T, et al. Phase I/II trial of combination of temozolomide chemotherapy and immunotherapy with fusions of dendritic and glioma cells in patients with glioblastoma. Cancer Immunol Immunother. (2016) 65:1499–509. doi: 10.1007/s00262-016-1905-7
111.Hu, JL, Omofoye, OA, Rudnick, JD, Kim, S, Tighiouart, M, Phuphanich, S, et al. A phase I study of autologous dendritic cell vaccine pulsed with allogeneic stem-like cell line lysate in patients with newly diagnosed or recurrent glioblastoma. Clin Cancer Res. (2022) 28:689–96. doi: 10.1158/1078-0432.CCR-21-2867
112.Okada, H, Lieberman, FS, Walter, KA, Lunsford, LD, Kondziolka, DS, Bejjani, GK, et al. Autologous glioma cell vaccine admixed with interleukin-4 gene transfected fibroblasts in the treatment of patients with malignant gliomas. J Transl Med. (2007) 5:67. doi: 10.1186/1479-5876-5-67
113.Hunn, MK, Bauer, E, Wood, CE, Gasser, O, Dzhelali, M, Ancelet, LR, et al. Dendritic cell vaccination combined with temozolomide retreatment: results of a phase I trial in patients with recurrent glioblastoma multiforme. J Neuro-Oncol. (2015) 121:319–29. doi: 10.1007/s11060-014-1635-7
114.Muragaki, Y, Maruyama, T, Ishikawa, E, Nitta, M, Ikuta, S, Yamamoto, T, et al. OS09.8 randomized placebo-controlled trial of autologous formalin-fixed tumor vaccine for newly diagnosed glioblastoma. Neuro-Oncology. (2017) 19:iii20. doi: 10.1093/neuonc/nox036.067
115.Steiner, HH, Bonsanto, MM, Beckhove, P, Brysch, M, Geletneky, K, Ahmadi, R, et al. Antitumor vaccination of patients with glioblastoma multiforme: a pilot study to assess feasibility, safety, and clinical benefit. J Clin Oncol. (2004) 22:4272–81. doi: 10.1200/JCO.2004.09.038
116.Izumoto, S, Tsuboi, A, Oka, Y, Suzuki, T, Hashiba, T, Kagawa, N, et al. Phase II clinical trial of Wilms tumor 1 peptide vaccination for patients with recurrent glioblastoma multiforme. J Neurosurg. (2008) 108:963–71. doi: 10.3171/JNS/2008/108/5/0963
117.Ciesielski, MJ, Ahluwalia, MS, Reardon, DA, Abad, AP, Curry, WT, Wong, ET, et al. Final data from the phase 2a single-arm trial of SurVaxM for newly diagnosed glioblastoma. JCO. (2022) 40:2037. doi: 10.1200/JCO.2022.40.16_suppl.2037
118.Migliorini, D, Dutoit, V, Allard, M, Grandjean Hallez, N, Marinari, E, Widmer, V, et al. Phase I/II trial testing safety and immunogenicity of the multipeptide IMA950/poly-ICLC vaccine in newly diagnosed adult malignant astrocytoma patients. Neuro-Oncology. (2019) 21:923–33. doi: 10.1093/neuonc/noz040
119.Yajima, N, Yamanaka, R, Mine, T, Tsuchiya, N, Homma, J, Sano, M, et al. Immunologic evaluation of personalized peptide vaccination for patients with advanced malignant glioma. Clin Cancer Res. (2005) 11:5900–11. doi: 10.1158/1078-0432.CCR-05-0559
120.Narita, Y, Arakawa, Y, Yamasaki, F, Nishikawa, R, Aoki, T, Kanamori, M, et al. A randomized, double-blind, phase III trial of personalized peptide vaccination for recurrent glioblastoma. Neuro-Oncology. (2019) 21:348–59. doi: 10.1093/neuonc/noy200
121.Hilf, N, Kuttruff-Coqui, S, Frenzel, K, Bukur, V, Stevanović, S, Gouttefangeas, C, et al. Actively personalized vaccination trial for newly diagnosed glioblastoma. Nature. (2019) 565:240–5. doi: 10.1038/s41586-018-0810-y
122.Keskin, DB, Anandappa, AJ, Sun, J, Tirosh, I, Mathewson, ND, Li, S, et al. Neoantigen vaccine generates intratumoral T cell responses in phase Ib glioblastoma trial. Nature. (2019) 565:234–9. doi: 10.1038/s41586-018-0792-9
123.Crane, CA, Han, SJ, Ahn, B, Oehlke, J, Kivett, V, Fedoroff, A, et al. Individual patient-specific immunity against high-grade glioma after vaccination with autologous tumor derived peptides bound to the 96 KD chaperone protein. Clin Cancer Res. (2013) 19:205–14. doi: 10.1158/1078-0432.CCR-11-3358
124.Bloch, O, Crane, CA, Fuks, Y, Kaur, R, Aghi, MK, Berger, MS, et al. Heat-shock protein peptide complex-96 vaccination for recurrent glioblastoma: a phase II, single-arm trial. Neuro-Oncology. (2014) 16:274–9. doi: 10.1093/neuonc/not203
125.Ji, N, Zhang, Y, Liu, Y, Xie, J, Wang, Y, Hao, S, et al. Heat shock protein peptide complex-96 vaccination for newly diagnosed glioblastoma: a phase I, single-arm trial. JCI Insight. (2018) 3:99145. doi: 10.1172/jci.insight.99145
126.Kong, DS, Nam, DH, Kang, SH, Lee, JW, Chang, JH, Kim, JH, et al. Phase III randomized trial of autologous cytokine-induced killer cell immunotherapy for newly diagnosed glioblastoma in Korea. Oncotarget. (2017) 8:7003–13. doi: 10.18632/oncotarget.12273
127.Werlenius, K, Stragliotto, G, Strandeus, M, Blomstrand, M, Carén, H, Jakola, AS, et al. A randomized phase II trial of efficacy and safety of the immunotherapy ALECSAT as an adjunct to radiotherapy and temozolomide for newly diagnosed glioblastoma. Neurooncol Adv. (2021) 3:vdab156. doi: 10.1093/noajnl/vdab156
128.Smith, C, Lineburg, KE, Martins, JP, Ambalathingal, GR, Neller, MA, Morrison, B, et al. Autologous CMV-specific T cells are a safe adjuvant immunotherapy for primary glioblastoma multiforme. J Clin Invest. (2020) 130:6041–53. doi: 10.1172/JCI138649
129.O’Rourke, DM, Nasrallah, MP, Desai, A, Melenhorst, JJ, Mansfield, K, Morrissette, JJD, et al. A single dose of peripherally infused EGFRvIII-directed CAR T cells mediates antigen loss and induces adaptive resistance in patients with recurrent glioblastoma. Sci Transl Med. (2017) 9:eaaa0984. doi: 10.1126/scitranslmed.aaa0984
130.Goff, SL, Morgan, RA, Yang, JC, Sherry, RM, Robbins, PF, Restifo, NP, et al. Pilot trial of adoptive transfer of chimeric antigen receptor-transduced T cells targeting EGFRvIII in patients with glioblastoma. J Immunother. (2019) 42:126–35. doi: 10.1097/CJI.0000000000000260
131.Brown, CE, Badie, B, Barish, ME, Weng, L, Ostberg, JR, Chang, WC, et al. Bioactivity and safety of IL13Rα2-redirected chimeric antigen receptor CD8+ T cells in patients with recurrent glioblastoma. Clin Cancer Res. (2015) 21:4062–72. doi: 10.1158/1078-0432.CCR-15-0428
132.Adair, JE, Beard, BC, Trobridge, GD, Neff, T, Rockhill, JK, Silbergeld, DL, et al. Extended survival of glioblastoma patients after chemoprotective HSC gene therapy. Sci Transl Med. (2012) 4:133ra57. doi: 10.1126/scitranslmed.3003425
133.Adair, JE, Johnston, SK, Mrugala, MM, Beard, BC, Guyman, LA, Baldock, AL, et al. Gene therapy enhances chemotherapy tolerance and efficacy in glioblastoma patients. J Clin Invest. (2014) 124:4082–92. doi: 10.1172/JCI76739
134.Merchant, RE, Grant, AJ, Merchant, LH, and Young, HF. Adoptive immunotherapy for recurrent glioblastoma multiforme using lymphokine activated killer cells and recombinant interleukin-2. Cancer. (1988) 62:665–71. doi: 10.1002/1097-0142(19880815)62:4<665::AID-CNCR2820620403>3.0.CO;2-O
135.Lillehei, KO, Mitchell, DH, Johnson, SD, McCleary, EL, and Kruse, CA. Long-term follow-up of patients with recurrent malignant gliomas treated with adjuvant adoptive immunotherapy. Neurosurgery. (1991) 28:16–23. doi: 10.1227/00006123-199101000-00003
136.Hayes, RL, Koslow, M, Hiesiger, EM, Hymes, KB, Hochster, HS, Moore, EJ, et al. Improved long term survival after intracavitary interleukin-2 and lymphokine-activated killer cells for adults with recurrent malignant glioma. Cancer. (1995) 76:840–52. doi: 10.1002/1097-0142(19950901)76:5<840::AID-CNCR2820760519>3.0.CO;2-R
137.Dillman, RO, Duma, CM, Ellis, RA, Cornforth, AN, Schiltz, PM, Sharp, SL, et al. Intralesional lymphokine-activated killer cells as adjuvant therapy for primary glioblastoma. J Immunother. (2009) 32:914–9. doi: 10.1097/CJI.0b013e3181b2910f
138.Van Gool, S, Makalowski, J, and Stuecker, W. ATIM-13.Multimodal immunotherapy with IO-VAC® for patients with GBM: a single institution experience. Neuro-Oncology. (2019) 21:vi4. doi: 10.1093/neuonc/noz175.013
139.Van Gool, SW, Makalowski, J, Bitar, M, Van de Vliet, P, Schirrmacher, V, and Stuecker, W. Synergy between TMZ and individualized multimodal immunotherapy to improve overall survival of IDH1 wild-type MGMT promoter-unmethylated GBM patients. Genes Immun. (2022) 23:255–9. doi: 10.1038/s41435-022-00162-y
140.D’Alessio, A, Proietti, G, Sica, G, and Scicchitano, BM. Pathological and molecular features of glioblastoma and its peritumoral tissue. Cancers (Basel). (2019) 11:469. doi: 10.3390/cancers11040469
141.Verreault, M, Weppler, SA, Stegeman, A, Warburton, C, Strutt, D, Masin, D, et al. Combined RNAi-mediated suppression of Rictor and EGFR resulted in complete tumor regression in an orthotopic glioblastoma tumor model. PLoS One. (2013) 8:e59597. doi: 10.1371/journal.pone.0059597
142.Zhu, Y, and Shah, K. Multiple lesions in receptor tyrosine kinase pathway determine glioblastoma response to pan-ERBB inhibitor PF-00299804 and PI3K/mTOR dual inhibitor PF-05212384. Cancer Biol Ther. (2014) 15:815–22. doi: 10.4161/cbt.28585
143.Han, K, Ren, M, Wick, W, Abrey, L, Das, A, Jin, J, et al. Progression-free survival as a surrogate endpoint for overall survival in glioblastoma: a literature-based meta-analysis from 91 trials. Neuro-Oncology. (2014) 16:696–706. doi: 10.1093/neuonc/not236
144.Jia, W, Gao, Q, Han, A, Zhu, H, and Yu, J. The potential mechanism, recognition and clinical significance of tumor pseudoprogression after immunotherapy. Cancer Biol Med. (2019) 16:655–70. doi: 10.20892/j.issn.2095-3941.2019.0144
145.Okada, H, Weller, M, Huang, R, Finocchiaro, G, Gilbert, MR, Wick, W, et al. Immunotherapy response assessment in neuro-oncology (iRANO): a report of the RANO working group. Lancet Oncol. (2015) 16:e534–42. doi: 10.1016/S1470-2045(15)00088-1
146.Saad, ED, and Katz, A. Progression-free survival and time to progression as primary end points in advanced breast cancer: often used, sometimes loosely defined. Ann Oncol. (2009) 20:460–4. doi: 10.1093/annonc/mdn670
147.Clavreul, A, Piard, N, Tanguy, JY, Gamelin, E, Rousselet, MC, Leynia, P, et al. Autologous tumor cell vaccination plus infusion of GM-CSF by a programmable pump in the treatment of recurrent malignant gliomas. J Clin Neurosci. (2010) 17:842–8. doi: 10.1016/j.jocn.2009.11.017
148.Muragaki, Y, Maruyama, T, Iseki, H, Tanaka, M, Shinohara, C, Takakura, K, et al. Phase I/IIa trial of autologous formalin-fixed tumor vaccine concomitant with fractionated radiotherapy for newly diagnosed glioblastoma. Clin Article J Neurosurg. (2011) 115:248–55. doi: 10.3171/2011.4.JNS10377
149.Prinsen, CAC, Vohra, S, Rose, MR, King-Jones, S, Ishaque, S, Bhaloo, Z, et al. Core outcome measures in effectiveness trials (COMET) initiative: protocol for an international Delphi study to achieve consensus on how to select outcome measurement instruments for outcomes included in a ‘core outcome set’. Trials. (2014) 15:247. doi: 10.1186/1745-6215-15-247
150.Zhang, L, Liu, Z, Li, J, Huang, T, Wang, Y, Chang, L, et al. Genomic analysis of primary and recurrent gliomas reveals clinical outcome related molecular features. Sci Rep. (2019) 9:16058. doi: 10.1038/s41598-019-52515-9
151.Birzu, C, French, P, Caccese, M, Cerretti, G, Idbaih, A, Zagonel, V, et al. Recurrent glioblastoma: from molecular landscape to new treatment perspectives. Cancers (Basel). (2021) 13:47. doi: 10.3390/cancers13010047
152.Bucci, MK, Maity, A, Janss, AJ, Belasco, JB, Fisher, MJ, Tochner, ZA, et al. Near complete surgical resection predicts a favorable outcome in pediatric patients with nonbrainstem, malignant gliomas. Cancer. (2004) 101:817–24. doi: 10.1002/cncr.20422
153.Bloom, HJ, Peckham, MJ, Richardson, AE, Alexander, PA, and Payne, PM. Glioblastoma multiforme: a controlled trial to assess the value of specific active immunotherapy in patients treated by radical surgery and radiotherapy. Br J Cancer. (1973) 27:253–67. doi: 10.1038/bjc.1973.30
154.Plautz, GE, Barnett, GH, Miller, DW, Cohen, BH, Prayson, RA, Krauss, JC, et al. Systemic T cell adoptive immunotherapy of malignant gliomas. J Neurosurg. (1998) 89:42–51. doi: 10.3171/jns.1998.89.1.0042
155.Wen, PY, Chang, SM, Van den Bent, MJ, Vogelbaum, MA, Macdonald, DR, and Lee, EQ. Response assessment in neuro-oncology clinical trials. JCO. (2017) 35:2439–49. doi: 10.1200/JCO.2017.72.7511
156.Tirrò, E, Massimino, M, Broggi, G, Romano, C, Minasi, S, Gianno, F, et al. A custom DNA-based NGS panel for the molecular characterization of patients with diffuse gliomas: diagnostic and therapeutic applications. Front Oncol. (2022) 12:861078. doi: 10.3389/fonc.2022.861078
157.Broggi, G, Piombino, E, Altieri, R, Romano, C, Certo, F, Barbagallo, GMV, et al. Glioblastoma, IDH-wild type with FGFR3-TACC3 fusion: when morphology may reliably predict the molecular profile of a tumor. A case report and literature review. Front Neurol. (2022) 13:823015. doi: 10.3389/fneur.2022.823015
158.Torrisi, F, D’Aprile, S, Denaro, S, Pavone, AM, Alberghina, C, Zappalà, A, et al. Epigenetics and metabolism reprogramming interplay into glioblastoma: novel insights on immunosuppressive mechanisms. Antioxidants (Basel). (2023) 12:220. doi: 10.3390/antiox12020220
159.Jahangiri, A, Chin, AT, Flanigan, PM, Chen, R, Bankiewicz, K, and Aghi, MK. Convection-enhanced delivery in glioblastoma: a review of preclinical and clinical studies. J Neurosurg. (2017) 126:191–200. doi: 10.3171/2016.1.JNS151591
Keywords: Glioblastoma, glioma, genomics, personalised therapy, survival
Citation: Mowforth OD, Brannigan J, El Khoury M, Sarathi CIP, Bestwick H, Bhatti F and Mair R (2023) Personalised therapeutic approaches to glioblastoma: A systematic review. Front. Med. 10:1166104. doi: 10.3389/fmed.2023.1166104
Edited by:
Surasak Saokaew, University of Phayao, ThailandReviewed by:
Miguel A. Idoate, University of Seville, SpainGiuseppe Broggi, University of Catania, Italy
Copyright © 2023 Mowforth, Brannigan, El Khoury, Sarathi, Bestwick, Bhatti and Mair. This is an open-access article distributed under the terms of the Creative Commons Attribution License (CC BY). The use, distribution or reproduction in other forums is permitted, provided the original author(s) and the copyright owner(s) are credited and that the original publication in this journal is cited, in accordance with accepted academic practice. No use, distribution or reproduction is permitted which does not comply with these terms.
*Correspondence: Richard Mair, cmljaGFyZC5tYWlyQGNydWsuY2FtLmFjLnVr