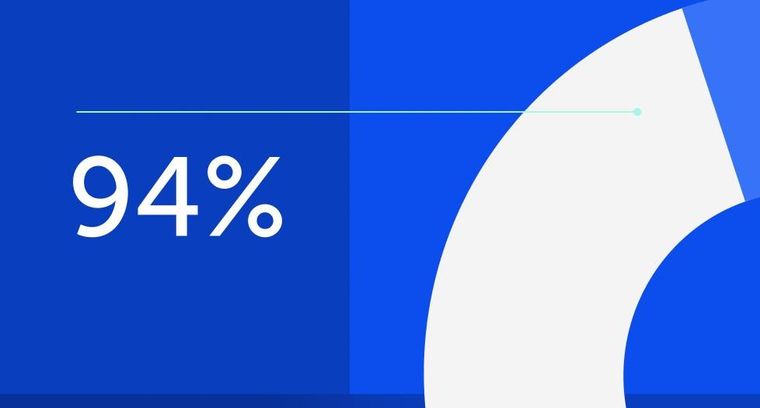
94% of researchers rate our articles as excellent or good
Learn more about the work of our research integrity team to safeguard the quality of each article we publish.
Find out more
ORIGINAL RESEARCH article
Front. Med., 19 June 2024
Sec. Translational Medicine
Volume 10 - 2023 | https://doi.org/10.3389/fmed.2023.1159586
This article is part of the Research TopicGenetics of Sudden Unexplained Death in Children and Young Adults: State of the Art, Testing and Implications for Translational Research, Public Health and Forensic PathologyView all 5 articles
Background: Brugada Syndrome (BrS) is an inherited arrhythmia syndrome in which mutations in the cardiac sodium channel SCN5A (NaV1.5) account for approximately 20% of cases. Mutations in sodium channel-modifying genes may account for additional BrS cases, though BrS may be polygenic given common SNPs associated with BrS have been identified. Recent analysis, however, has suggested that SCN5A should be regarded as the sole monogenic cause of BrS.
Objective: We sought to re-assess the genetic underpinnings of BrS in a large mutligenerational family with a putative mutation in GPD1L that affects surface membrane expression of NaV1.5 in vitro.
Methods: Fine linkage mapping was performed in the family using the Illumina Global Screening Array. Whole exome sequencing of the proband was performed to identify rare variants and mutations, and Sanger sequencing was used to assay previously-reported risk single nucleotide polymorphsims (SNPs) for BrS.
Results: Linkage analysis decreased the size of the previously-reported microsatellite linkage region to approximately 3 Mb. GPD1L-A280V was the only coding non-synonymous variation present at less than 1% allele frequency in the proband within the linkage region. No rare non-synonymous variants were present outside the linkage area in affected individuals in genes associated with BrS. Risk SNPs known to predispose to BrS were overrepresented in affected members of the family.
Conclusion: Together, our data suggest GPD1L-A280V remains the most likely cause of BrS in this large multigenerational family. While care should be taken in interpreting variant pathogenicity given the genetic uncertainty of BrS, our data support inclusion of other putative BrS genes in clinical genetic panels.
Brugada Syndrome (BrS) is an inherited arrhythmia syndrome characterized by ST-segment elevation in the right precordial leads of the electrocardiogram (EKG; V1 through V3) and sudden cardiac death (1). The molecular mechanism underpinning BrS was initially described as autosomal-dominant loss-of-function mutations in the main cardiac sodium channel, NaV1.5, encoded on chromosome 3 by SCN5A (2). These loss-of-function mutations decrease inward depolarizing sodium current, which can result in premature repolarization of the epicardium in the right ventricle, slowed conduction, ventricular tachyarrhythmias, and sudden cardiac death (3). However, only approximately 20% of patients with Brugada Syndrome have mutations in SCN5A. The advent of massively parallel sequencing has allowed many groups to investigate the genetic underpinnings of BrS over the past two decades. These genetic data, in combination with molecular studies, have expanded the number of putative disease-causing genes in BrS from one gene, SCN5A, to over 20 genes today. Genes reported to have mutations which cause BrS can be broadly classified as those which encode (1) sodium channels, (2) sodium channel interacting proteins, (3) other ion channels, and (4) metabolic proteins (4). Recent reports have also suggested BrS may be polygenic in nature, and that single nucleotide polymorphisms (SNPs) near SCN5A-SCN10A and loci responsible for SCN5A transcription and trafficking are associated with BrS (5, 6).
Our laboratory used mapping with microsatellites in a large, multigenerational family with BrS to identify a linkage region on chromosome 3 with a LOD score >4 that did not contain SCN5A or SCN10A (7, 8). Positional cloning and subsequent molecular analysis identified a variant in glycerol 3-phosphate dehydrogenase 1-like (GPD1L) as the putative cause of this family's disease. Peak sodium current in HEK293 cells transfected with NaV1.5 decreased if cells were co-transfected with GPD1L-A280V compared to those co-transfected with GPD1L-WT. Additional studies suggested GPD1L-A280V decreases NaV1.5 membrane expression, and that GPD1L mutations were associated with sudden infant death syndrome (SIDS) on molecular autopsies (9). The identification of GPD1L by linkage and the subsequent demonstration that it decreases sodium channel membrane localization and current together suggests GPD1L-A280V is pathogenic for BrS.
The explosion of genetic data, especially variants of uncertain significance, from clinical genetic testing has led to a plethora of putative mutations but a dearth of scientific evidence supporting the pathogenicity of these variants. Concerns about variants of uncertain significance being classified as pathogenic without data to support the claim has been reviewed in many publications (10, 11). This concern sparked a review of the pathogenicity of reported BrS genes using the Clinical Genome Resources (ClinGen) framework. This review determined that SCN5A was the only gene with sufficient scientific evidence to definitively be regarded as a causative gene for BrS (12). GPD1L was excluded due to a large linkage region in which other genes had not been sequenced and the relatively high allele frequency of GPD1L-A280V (gnomAD V2.1.1 allele frequency = 1.29E-4) (13, 14).
In the present study, we provide high-depth whole exome sequencing data in the proband of our originally-reported large multigenerational family, SNP-based linkage analysis of affected individuals, and sequencing data of previously defined BrS risk SNPs to support our initial reports that the A280V mutation in GPD1L, a sodium channel interacting protein, is pathogenic for BrS.
Patients were enrolled under protocols approved through the University of Pittsburgh and University of Iowa Institutional Review Boards (IRBs). All individuals in the study provided informed consent for participation in the study. Type 1, Type 2, or Type 3 Brugada Syndrome, or clinically unaffected status, was determined through clinical history, electrocardiogram analysis, and clinical provocative testing using procainamide as previously described (8).
Genomic DNA was isolated from peripheral blood using commercially-available kits or automated machine as previously described (8). Isolated DNA was treated with RNAse A and re-isolated by ethanol precipitation prior to sequencing. DNA integrity was assessed by agarose gel electrophoresis and quantified using Qubit High Sensitivty DNA assays (ThermoFisher Scientific).
Ten affected and five unaffected individuals from the family had genomic DNA prepared as described above. After passing quality control and subsequent library preparation, SNPs were called using the Infinium Global Screening Array (Illumina) at CD Genomics (Shirley, NY, USA) which sequences approximately 654,027 SNPs. Call rates were greater than 98% for all individuals (Supplementary Table 1). Chromosomes were phased using ShapeIt2 with DuoHMM enabled and the reference HapMap Phase II data (15, 16). The resultant phased haplotypes were investigated manually for recombination using our previously-reported microsatellite data as the starting point. Logarithm of odds scores were calculated using Superlink-Online SNP (17).
Whole exome sequencing was performed at the Iowa Institute of Human Genetics (IIHG) on genomic DNA prepared as described above. Library preparation was performed using standard protocols for the Agilent SureSelect V6 + UTR library preparation kit (Agilent Technologies). The resulting 150 base pair paired-end library was sequenced on an Illumina HiSeq 4000. Resultant .fastq files underwent quality control using FastQC (18). Alignment, variant calling, and variant annotation were performed using BWA-MEM (alignment), GATK4 (variant calling), and SnpEff (variant annotation) using an implementation of BCBIO and the GRCh37 reference genome (19–24). A second variant calling tool, Freebayes, was used in a near-identical pipeline to ensure consensus variant calls were achieved (25). Greater than 80% of targeted bases had greater than 50x coverage (Supplementary Figure 1). A brief summary of sequencing results is shown in Supplementary Table 2.
Two approaches were used to identify candidate variants (1) a linkage-region focused analyses and (2) a linkage-naive approach. The linkage-region focused analysis was limited to the newly-defined linkage region on chromosome 3, while the linkage-naive approach entertained variants on any autosome. For both approaches, the resulting annotated variant call file was a used to generate a database compatible with Gemini (26). Variants present at less than 1% minor allele frequency, a lenient threshold for autosomal dominant disease, were included. Variants were then segregated according to impact (nonsense, splicing, missense, synonymous) with synonymous variants removed from consideration. Variant location information, either exonic, UTR, intronic, or intergenic, was subsequently used in prioritization of variants.
Sanger sequencing of BrS risk SNPs in SCN5A, SCN10A, and HEY2 was performed at the Iowa Institute of Human Genetics using custom primers designed in-lab and synthesized by Integrated DNA Technologies (Supplementary Table 3). The resulting chromatograms were analyzed using NCBI Blast, Finch, and SnapGene from which wild-type, heterozygous, or homozygous SNP calls were made and manually validated.
A single unpublished protein model of GPD1L is present on the RCSB Protein Databank (26, 27). AlphaFold computational models for GPD1L are also publicly available from EMBL. The AlphaFold GPD1L model AF-Q8N335-F1-model_v1 was downloaded and imported into PyMol (28). The mutagenesis function of PyMol was used to visualize the impact of the three predicted GPD1L-A280V rotamers. Strain scores were calculated for all rotamers within PyMol (Supplementary Figure 2). Variant data for GPD1L was downloaded from ClinVar (29).
To more precisely define the linked genomic regions within this family we performed linkage analysis using the Illumina Global Screening Array with ten affected and five unaffected family members. The previously-identified 15 cm linkage region in this family which we reported was used as a starting point for our investigation (8). Analysis of phased haplotypes identified a narrowed linkage region defined by the SNPs rs13059657 and rs7651953 (GRCh37, chr3:29,899,567-32,970,737) (Figure 1). Individuals III-2 and III-8 defined the 5′ and 3′ breakpoints, respectively. Calculation of affected only LOD scores using individuals having a Type 1 EKG pattern or positive procainamide test using Superlink-Online SNP results in a LOD score of 2.02 for this region (17). The addition of individuals with Type II EKG patterns results in a LOD score of 3.18. The region of interest contains 14 genes, including GPD1L, but not SCN5A or SCN10A which are approximately 6 Mb downstream (Figure 1).
Figure 1. Representative pedigree of the family, linkage analysis, and assessment of Brugada Syndrome (BrS) risk SNPs. (A) Linkage analysis demonstrates a small region on chromosome 3 (inset map and green bracketed region) which has undergone recombination in the third generation of the pedigree. Previously reported risk SNPs (SCN5A and SCN10A, red) for the BrS phenotype are associated with the GPD1L-A280V. (B) The 3 Mb region on chromosome 3 identified by linkage analysis contains 14 genes, including GPD1L. SCN5A and SCN10A lie upstream of GPD1L (ideogram, red text) and outside of the refined linkage region (ideogram, red box). ICD, implantable cardioverter defibrillator; +Proc, positive procainamide challenge; RBBB, right bundle branch block; VT, ventricular tachycardia.
High-depth (100x) whole exome sequencing of the proband was performed using the Agilent SureSelect V6+UTR capture kit. Limiting the analysis to the newly defined linkage region identified GPD1L-A280V as the only rare, exonic coding variation. No other exonic or splice variants occurred at an allele frequency of less than 1% in or near this region.
Outside of the linkage region, the proband carried no variants that were annotated as pathogenic for cardiovascular diseases in the ClinVar database. In addition, he carried no rare missense or nonsense variants in genes previously assocated with BrS or that have known interactions with NaV1.5. He was heterozygous for a synonymous SNP in CACNA1C (p.G1738G;c.5358C>T, f = 1.21E-5), and for non-synonymous missense variants of uncertain significance in genes associated with cardiomyopathy and arrhythmia, including a variant in ANK2 (p.R2416G;c.7246C>G, unreported), a variant in FHL2 (p.V187M;c.559G>A, f = 6.78E-5), and two variants in TTN (p.A31503T;c94507G>A, f = 5.04E-5 and p.F14410C;c.43229T>G, unreported) (Table 1). Linkage analysis and Sagner sequencing confirmed that none of these variants cosegregated with BrS in this family.
Table 1. Variants in reported Brugada Syndrome Genes or in the Inherited Cardiac Condition (ICC) Gene List.
Three published risk SNPs for BrS [rs10428132 (SCN10A; total minor allele frequency, dbSNP (MAF) = 0.40), rs11708996 (SCN5A; MAF = 0.14), and rs9388451 (HEY2; MAF = 0.42)] identified by Bezzina et al. were assessed in family members with sufficient DNA, showing 8/15 affected subjects (carrying the GPD1L putative mutation) in generations II and III are homozygous for the SCN10A risk allele and 12/15 subjects are heterozygous for the SCN5A risk allele (Figure 1). SCN5A and SCN10A are near GPD1L on chromosome 3; in most affected individuals the GPD1L-A280V putative mutation co-segregated with one SCN5A and one SCN10A risk allele. The HEY2 risk SNP on chromosome 6 segregated in Mendelian fashion. Overall, individuals affected with BrS (n = 15, including one individual not shown in Figure 1) carried 3.3 ± 0.2 risk SNPs, while unaffected individuals (n = 12) carried 2.1 ± 0.4 risk SNPs (p = 0.012) (Figure 2). The SCN5A and SCN10A risk SNPs carried along with GPD1L-A280V in this family may potentiate the pathogenicity of the GPD1L-A280V variant.
Figure 2. Distribution of Brugada Syndrome Risk Polymoprhisms in the large mutli-generational family. Individuals with Brguada Syndrome in our family carry more risk polymorphisms on average (3.3) than unaffected individuals (2.1) in our large mutli-generational family. The distribution of risk polymorphisms is similar to that which was reported by Bezzina et al. (5).
In silico mutagenesis of the A280 residue to V280 in a computational model of GPD1L demonstrates increased strain within the alpha helix in which residue 280 resides (Figure 3 and Supplementary Figure 2). Thus, in silico mutagenesis results in increased computationally-predicted strain stores indicating GPD1L-A280V may introduce steric hindrance in the GPD1L tertiary structure.
Figure 3. Computational modeling of GPD1L suggests A280V introduces steric clashing. (Left) The wild-type GPD1L structure as calculated by AlphaFold (AF-Q8N335-F1-model_v1) with residue A280 highlighted in blue. A280 resides in an alpha helix. (Right) One of three putative GPD1L-A280V rotamers as calculated by PyMol. All three predicted rotamers introduce strain in the alpha helix.
The report by Hosseini et al. (12) using the Clinical Genome Resources (ClinGen) framework downgraded GPD1L as a potential cause of BrS, due to (1) the initial large reported linkage region (>14 MB); (2) the failure to fully screen all of the genes in the region for mutation, which was limited by the technology available in 2007; and (3) the relatively high allele frequency of GPD1L-A280V. Our analysis of this large, multigenerational family narrows the linkage region to approximately 3.1 Mb which includes GPD1L but excludes SCN5A and SCN10A. Whole exome sequencing confirmed GPD1L-A280V as the only rare exonic variant at an allele frequency < 1% within this region. The affected-only LOD score of 3.18 for individuals displaying Type I and Type II BrS patterns (Type 1 BrS alone = 2.02) is consistent with the previous reported LOD score of >3 which included unaffected individuals. In addition, while the A280V variant is relatively common in white people and south Asian people (minor allele frequency 1/6,000), this does not preclude pathogenicity in a founder population or fully account for incomplete penetrance (30).
Our findings show that BrS associated with GPD1L mutations behaves similarly to BrS caused by SCN5A and other unidentified mutations. The Type III BrS EKG pattern observed in patient II-7, an obligate carrier whose male children display Type I and Type II EKG patterns, exemplifies the incomplete penetrance more common in women and reported in other BrS patients (13, 31) (Figure 1).
GPD1L-A280V is not the lone reported GPD1L putative mutation (Figure 4). Three putative mutations, p.E83K, p.I124V, and p.R273C), were identified in infants by molecular autopsy of 83 cases of sudden unexplained death and 221 cases of sudden infant death syndrome (9). As was found for A280V, each of these GPD1L putative mutations decreased peak sodium current in heterologous expression system or neonatal cardiac myocytes. A loss-of-function GPD1L mutation (p.R189X; c.565C>T) was reported to cosegregate in a small family with ventricular tachycardia and sudden death (32). The EKG of the proband showed a Type 2 BrS pattern [Huang et al. (32), Figure 1B]. In addition, ClinVar reports 3 other variants of uncertain significance in GPD1L (p.E174K, p.R231C, Q345H) (33).
Figure 4. Reported GPD1L variants in ClinVar show GPD1L-A280V is not the sole reported putative mutation. Missense variants with conflicting interpretation (yellow circles, with GPD1L-A280V highlighted as red) and variants of uncertain significance (black bars, 83 total) were downloaded from ClinVar (accessed November 12, 2021) and plotted along the full length of GPD1L. All five variants with conflicting interpretation, including GPD1L-A280V, were submitted to ClinVar by their respective submitters with Brugada Syndrome as the clinical condition.
Mutations in SCN5A that disrupt NaV1.5 expression, trafficking, or function and decrease peak depolarizing sodium current are the predominant genetically-identifiable cause of BrS (1). The decrease in inward current is postulated to either cause premature repolarization or result in marked conduction slowing and block in the right ventricular outflow tract and septum, where the repolarizing transient outward potassium current is greater (3, 34). Animal studies suggest a wide safety margin is present for the cardiac sodium current, with a loss of ≥ 50% in association with other factors such as age and sex being necessary to generate a marked phenotype (35). As such, SCN5A may be the only single gene for which mutations are able to decrease inward sodium current sufficiently to cause BrS with high penetrance.
Many of the other genes reported to potentially cause BrS modulate NaV1.5 expression, trafficking, or function (4). In addition, GWAS studies have identified common variants in both sodium channel interacting proteins and transcription factors associated with NaV1.5 expression that are associated with BrS (5, 6). Thus, the majority of cases of BrS may be polygenic, with decreased inward sodium current related to both rare and common variants in a number of genes, in association with metabolic and environmental factors.
In the multigenerational family with the putative GPD1L mutation studied here, we identified two common variants associated with BrS that were overrepresented compared to the general population. These variants, one in SCN5A and one in SCN10A, are linked to the mutant GPD1L allele in 12 of the 14 affected individuals. The presence of these common variants in association with the mutant GPD1L allele could exacerbate the loss of sodium current, and explain in part why the relatively common A280V-GPD1L variant is pathogenic in the family presented here.
Genetic testing is now standard in evaluation of patients with suspected sudden cardiac death syndromes. Physicians and genetic counselors can test for mutations in a single gene, in gene panels, in the exome, or in the genome. While useful, present-day testing results only offer an approximately 25% identification rate in BrS (36). Current recommendations for clinical genetic testing panels in BrS suggest exclusion of all genes except SCN5A. While the intent to avoid undue harm from provider misinterpretation of variants in unproven genes is well-intentioned, this could reduce identification of novel rare variation contributing to BrS in sodium channel modifying genes, among others, and prevent acquiring the data that would prove causality, such as linkage in a large family. We believe these concerns highlight the necessity of appropriate interpretation of genetic testing panels through cardiologists trained in genetics and genetic counselors. We also note the need for widespread reporting of identified variants and research laboratories that can identify and assess putative mutations (37, 38).
Our study has a number of limitations. First, we used linkage and exome sequencing data from a single family. Linkage data provide an incomplete picture of the genetic architecture for a disease, though we believe the addition of exome sequencing partly addresses this limitation. Notably, exome sequencing libraries are not targeted to capture intronic sequences, and deep intronic variants within GPD1L or nearby genes may contribute to disease in this family. Second, we assessed only a fraction of the common SNPs that are associated with BrS (6). Because only SCN5A and SCN10A are near the linkage area on chromosome 3p, we would not expect that the other variants would be heavily overexpressed or underexpressed in our affected individuals. Third, the mechanism by which GPD1L variants alter NaV1.5 expression and/or function is not addressed. GPD1L is involved in the metabolic regulation of cellular activity, and this is an area of active investigation using heterologous expression systems and gene-targeted mice.
We identified GPD1L-A280V as the only rare non-synonymous coding variation in the refined linkage region in a large, multigenerational family. Associated BrS risk SNPs may be permissive for the BrS phenotype in affected family members. In addition, the A280V mutation may alter the GPD1L tertiary structure. While mutation in SCN5A is the most common cause genetically-identified cause of BrS, our study suggests that GPD1L should still be considered when evaluating the genetic underpinnings of BrS and sudden death. Ultimately, continued study of BrS through genome-wide analyses, targeted familial sequencing studies, mechanistic studies, and investigation of putative mutations will further our knowledge of this disease.
The original contributions presented in the study are publicly available. This data can be found here: https://www.ncbi.nlm.nih.gov/projects/gap/cgi-bin/study.cgi?study_id=phs003468.v1.p1.
The studies involving human participants were reviewed and approved by Institutional review boards of the University of Pittsburgh and the University of Iowa. Written informed consent to participate in this study was provided by the participants' legal guardian/next of kin. Written informed consent was obtained from the individual(s), and minor(s)' legal guardian/next of kin, for the publication of any potentially identifiable images or data included in this article.
AG and BL were responsible for the conception and design of the work. RG and BL enrolled the family, acquired clinical records, and obtained DNA from the family. AG, CC, and HM were responsible for the acquisition and analysis of the work. AG, HM, CC, RG, and BL interpreted data contained in this work. All authors contributed to the process of manuscript drafting and revision.
This work was supported by R01HL062300, R01HL077398, and R01HL115955. AG was supported by an NIH Ruth L. Kirschstein F30 fellowship (F30HL143908) and by a T32 training grant held by the University of Iowa Medical Scientist Training Program (T32GM007337).
The authors declare that the research was conducted in the absence of any commercial or financial relationships that could be construed as a potential conflict of interest.
All claims expressed in this article are solely those of the authors and do not necessarily represent those of their affiliated organizations, or those of the publisher, the editors and the reviewers. Any product that may be evaluated in this article, or claim that may be made by its manufacturer, is not guaranteed or endorsed by the publisher.
The Supplementary Material for this article can be found online at: https://www.frontiersin.org/articles/10.3389/fmed.2023.1159586/full#supplementary-material
BrS, Brugada Syndrome; SNP, single nucleotide polymorphism; CM, centimorgan; LOD, logarithm of the odds; Mb, megabase; ICD, implantable cardioverter defibrillator; RBBB, right bundle branch block; VT, ventricular tachycardia.
1. Brugada J, Brugada P, Brugada R. The syndrome of right bundle branch block ST segment elevation in V1 to V3 and sudden death–the Brugada syndrome. Europace. (1999) 1:156–66. doi: 10.1053/eupc.1999.0033
2. Chen Q, Kirsch GE, Zhang D, Brugada R, Brugada J, Brugada P, et al. Genetic basis and molecular mechanism for idiopathic ventricular fibrillation. Nature. (1998) 392:293–6. doi: 10.1038/32675
3. Antzelevitch C, Brugada P, Brugada J, Brugada R, Towbin JA, Nademanee K. Brugada syndrome: 1992–2002. J Am Coll Cardiol. (2003) 41:1665–71. doi: 10.1016/s0735-1097(03)00310-3
4. Brugada J, Campuzano O, Arbelo E, Sarquella-Brugada G, Brugada R. Present status of Brugada syndrome: JACC state-of-the-art review. J Am Coll Cardiol. (2018) 72:1046–59. doi: 10.1016/j.jacc.2018.06.037
5. Bezzina CR, Barc J, Mizusawa Y, Remme CA, Gourraud JB, Simonet F, et al. Common variants at SCN5A-SCN10A and HEY2 are associated with Brugada syndrome, a rare disease with high risk of sudden cardiac death. Nat Genet. (2013) 45:1044–9. doi: 10.1038/ng.2712
6. Barc J, Tadros R, Glinge C, Chiang DY, Jouni M, Simonet F, et al. Genome-wide association analyses identify new Brugada syndrome risk loci and highlight a new mechanism of sodium channel regulation in disease susceptibility. Nat Genet. (2022) 54:232–9. doi: 10.1038/s41588-021-01007-6
7. Weiss R, Barmada MM, Nguyen T, Seibel JS, Cavlovich D, Kornblit CA, et al. Clinical and molecular heterogeneity in the Brugada syndrome. Circulation. (2022) 105:707–13. doi: 10.1161/hc0602.103618
8. London B, Michalec M, Mehdi H, Zhu X, Kerchner L, Sanyal S, et al. Mutation in glycerol-3-phosphate dehydrogenase 1 like gene (GPD1-L) decreases cardiac Na+ current and causes inherited arrhythmias. Circulation. (2007) 116:2260–8. doi: 10.1161/CIRCULATIONAHA.107.703330
9. Van Norstrand DW, Valdivia CR, Tester DJ, Ueda K, London B, Makielski JC, et al. Molecular and functional characterization of novel glycerol-3-phosphate dehydrogenase 1 like gene (GPD1-L) mutations in sudden infant death syndrome. Circulation. (2017) 116:2253–9. doi: 10.1161/CIRCULATIONAHA.107.704627
10. Ackerman MJ. Genetic purgatory and the cardiac channelopathies: exposing the variants of uncertain/unknown significance issue. Heart Rhythm. (2015) 12:2325–31. doi: 10.1016/j.hrthm.2015.07.002
11. Kapplinger JD, Giudicessi JR, Ye D, Tester DJ, Callis TE, Valdivia CR, et al. Enhanced classification of Brugada syndrome-associated and long-QT syndrome-associated genetic variants in the SCN5A-encoded Nav1.5 cardiac sodium channel. Circ Cardiovasc Genet. (2015) 8:582–95. doi: 10.1161/CIRCGENETICS.114.000831
12. Hosseini SM, Kim R, Udupa S, Costain G, Jobling R, Liston E, et al. Reappraisal of reported genes for sudden arrhythmic death: evidence-based evaluation of gene validity for Brugada syndrome. Circulation. (2018) 138:1195–205. doi: 10.1161/CIRCULATIONAHA.118.035070
13. Roden DM. Growing pains in cardiovascular genetics. Circulation. (2018) 138:1206–9. doi: 10.1161/CIRCULATIONAHA.118.035933
14. Karczewski KJ, Francioli LC, Tiao G, Cummings BB, Alföldi J, Wang Q, et al. The mutational constraint spectrum quantified from variation in 141,456 humans. Nature. (2020) 581:434–43. doi: 10.1038/s41586-020-2308-7
15. Delaneau O, Zagury JF, Marchini J. Improved whole-chromosome phasing for disease and population genetic studies. Nat Methods. (2013) 10:5–6. doi: 10.1038/nmeth.2307
16. The International HapMap Consortium. A second generation human haplotype map of over 3.1 million SNPs. Nature. (2007) 449:851–61. doi: 10.1038/nature06258
17. Silberstein M, Weissbrod O, Otten L, Tzemach A, Anisenia A, Shtark O, et al. A system for exact and approximate genetic linkage analysis of SNP data in large pedigrees. Bioinformatics. (2013) 29:197–205. doi: 10.1093/bioinformatics/bts658
18. Andrews S. FastQC: A Quality Control Tool for High Throuhgput Sequence Data. Available online at: http://www.bioinformatics.babraham.ac.uk/projects/fastqc
19. Li H. Aligning sequence reads, clone sequences and assembly contigs with BWA-MEM. arXiv: Genomics. (2013). doi: 10.6084/M9.FIGSHARE.963153.V1
20. DePristo MA, Banks E, Poplin R, Garimella KV, Maguire JR, Hartl C, et al. A framework for variation discovery and genotyping using next-generation DNA sequencing data. Nat Genet. (2011) 43:491–8. doi: 10.1038/ng.806
21. Poplin R, Ruano-Rubio V, DePristo MA, Fennell TJ, Carneiro MO, der Auwera GAV, et al. Scaling accurate genetic variant discovery to tens of thousands of samples. bioRxiv. (2018) 201178. doi: 10.1101/201178
22. Cingolani P, Platts A, Wang Le L, Coon M, Nguyen T, Wang L, et al. A program for annotating and predicting the effects of single nucleotide polymorphisms, SnpEff: SNPs in the genome of Drosophila melanogaster strain w1118; iso-2; iso-3. Fly (Austin). (2012) 6:80–92. doi: 10.4161/fly.19695
23. Chapman B, Kirchner R, Pantano L, Naumenko S, Smet MD, Beltrame L, et al. bcbio/bcbio-nextgen. Zenodo. (2021). doi: 10.5281/zenodo.4686097
24. Church DM, Schneider VA, Graves T, Auger K, Cunningham F, Bouk N, et al. Modernizing reference genome assemblies. PLoS Biol. (2011) 9:e1001091. doi: 10.1371/journal.pbio.1001091
25. Garrison E, Marth M. Haplotype-Based Variant Detection From Short-Read Sequencing. (2016) 1–20. Available online at: https://www.cs.umd.edu/class/spring2016/cmsc702/public/FreeBayesDraft2015Jan12.pdf
26. Paila U, Chapman BA, Kirchner R, Quinlan AR. GEMINI: Integrative exploration of genetic variation and genome annotations. PLoS Comput Biol. (2013) 9:e1003153. doi: 10.1371/journal.pcbi.1003153
27. RCBS Protein Data Bank. RCSB PDB-2PLA: Crystal Structure of Human Glycerol-3-Phosphate Dehydrogenase 1-Like Protein. Available online at: https://www.rcsb.org/structure/2PLA (accessed November 22, 2021).
28. Jumper J, Evans R, Pritzel A, Green T, Figurnov M, Ronneberger O, et al. Highly accurate protein structure prediction with AlphaFold. Nature. (2021) 596:583–9. doi: 10.1038/s41586-021-03819-2
29. Landrum MJ, Lee JM, Benson M, Brown GR, Chao C, Chitipiralla S, et al. ClinVar: improving access to variant interpretations and supporting evidence. Nucleic Acids Res. (2018) 46:D1062–7. doi: 10.1093/nar/gkx1153
30. Roden DM, Glazer AM, Kroncke B. Arrhythmia genetics: not dark and lite, but 50 shades of gray. Heart Rhythm. (2018) 15:1231–2. doi: 10.1016/j.hrthm.2018.04.031
31. Asatryan B, Yee L, Ben-Haim Y, Dobner S, Servatius H, Roten L, et al. Sex-related differences in cardiac channelopathies: implications for clinical practice. Circulation. (2021) 143:739–52. doi: 10.1161/CIRCULATIONAHA.120.048250
32. Huang H, Chen Y-Q, Fan L-L, Guo S, Li J-J, Jin J-Y, et al. Whole-exome sequencing identifies a novel mutation of GPD1L (R189X) associated with familial conduction disease and sudden death. J Cell Mol Med. (2018) 22:1350–4. doi: 10.1111/jcmm.13409
33. Whiffin N, Minikel E, Walsh R, O'Donnell-Luria AH, Karczewski K, Ing AY, et al. Using high-resolution variant frequencies to empower clinical genome interpretation. Genet Med. (2017) 19:1151–8. doi: 10.1038/gim.2017.26
34. Zhang J, Sacher F, Hoffmayer K, O'Hara T, Strom M, Cuculich P, et al. Cardiac electrophysiological substrate underlying the ECG phenotype and electrogram abnormalities in Brugada syndrome patients. Circulation. (2015) 131:1950–9. doi: 10.1161/CIRCULATIONAHA.114.013698
35. Jeevaratnam K, Poh Tee S, Zhang Y, Rewbury R, Guzadhur L, Duehmke R, et al. Delayed conduction and its implications in murine Scn5a(+/-) hearts: independent and interacting effects of genotype, age, and sex. Pflugers Arch. (2011) 461:29–44. doi: 10.1007/s00424-010-0906-1
36. Ackerman MJ, Priori SG, Willems S, Berul C, Brugada R, Calkins H, et al. HRS/EHRA expert consensus statement on the state of genetic testing for the channelopathies and cardiomyopathies. Heart Rhythm. (2011) 8:1308–39. doi: 10.1016/j.hrthm.2011.05.020
37. Ahmad F, McNally EM, Ackerman MJ, Baty LC, Day SM, Kullo IJ, et al. Establishment of specialized clinical cardiovascular genetics programs: recognizing the need and meeting standards: a scientific statement from the American Heart Association. Circ Genom Precis Med. 12:e000054. doi: 10.1161/HCG.0000000000000054
Keywords: inherited arrhythmia syndrome, Brugada Syndrome (BrS), sudden cardiac death (SCD), exome sequencing, genetics, arrhythmia
Citation: Greiner AM, Mehdi H, Cevan C, Gutmann R and London B (2024) The role of GPD1L, a sodium channel interacting gene, in the pathogenesis of Brugada Syndrome. Front. Med. 10:1159586. doi: 10.3389/fmed.2023.1159586
Received: 06 February 2023; Accepted: 06 March 2023;
Published: 19 June 2024.
Edited by:
Simone Grassi, University of Florence, ItalyReviewed by:
Francesca Cazzato, Catholic University of the Sacred Heart, ItalyCopyright © 2024 Greiner, Mehdi, Cevan, Gutmann and London. This is an open-access article distributed under the terms of the Creative Commons Attribution License (CC BY). The use, distribution or reproduction in other forums is permitted, provided the original author(s) and the copyright owner(s) are credited and that the original publication in this journal is cited, in accordance with accepted academic practice. No use, distribution or reproduction is permitted which does not comply with these terms.
*Correspondence: Barry London, YmFycnktbG9uZG9uQHVpb3dhLmVkdQ==
Disclaimer: All claims expressed in this article are solely those of the authors and do not necessarily represent those of their affiliated organizations, or those of the publisher, the editors and the reviewers. Any product that may be evaluated in this article or claim that may be made by its manufacturer is not guaranteed or endorsed by the publisher.
Research integrity at Frontiers
Learn more about the work of our research integrity team to safeguard the quality of each article we publish.