- 1Departamento de Microbiología, Facultad de Ciencias Biológicas, Universidad de Concepción, Concepción, Chile
- 2Programa de Microbiología y Micología, Instituto de Ciencias Biomédicas, Facultad de Medicina, Universidad de Chile, Santiago, Chile
- 3Instituto Milenio de Inmunología e Inmunoterapia, Facultad de Medicina, Universidad de Chile, Santiago, Chile
- 4Unidad de Paciente Crítico, Clínica Hospital del Profesor, Santiago, Chile
- 5Programa de Formación de Especialista en Medicina de Urgencia, Universidad Andrés Bello, Santiago, Chile
- 6Departamento de Pediatría y Cirugía Infantil, Hospital Dr. Roberto del Rio, Facultad de Medicina, Universidad de Chile, Santiago, Chile
- 7Programa de Inmunología, Instituto de Ciencias Biomédicas, Facultad de Medicina, Universidad de Chile, Santiago, Chile
Vibrio cholerae is the causative agent of cholera, a highly contagious diarrheal disease affecting millions worldwide each year. Cholera is a major public health problem, primarily in countries with poor sanitary conditions and regions affected by natural disasters, where access to safe drinking water is limited. In this narrative review, we aim to summarize the current understanding of the evolution of virulence and pathogenesis of V. cholerae as well as provide an overview of the immune response against this pathogen. We highlight that V. cholerae has a remarkable ability to adapt and evolve, which is a global concern because it increases the risk of cholera outbreaks and the spread of the disease to new regions, making its control even more challenging. Furthermore, we show that this pathogen expresses several virulence factors enabling it to efficiently colonize the human intestine and cause cholera. A cumulative body of work also shows that V. cholerae infection triggers an inflammatory response that influences the development of immune memory against cholera. Lastly, we reviewed the status of licensed cholera vaccines, those undergoing clinical evaluation, and recent progress in developing next-generation vaccines. This review offers a comprehensive view of V. cholerae and identifies knowledge gaps that must be addressed to develop more effective cholera vaccines.
1. Introduction
Cholera is an acute, watery diarrheal disease caused by Vibrio cholerae, a curved, rod-shaped, motile, Gram-negative bacterium that lives in aquatic environments. Without prompt treatment, cholera can cause severe dehydration and death. Treatment involves administering saline oral rehydration solutions, intravenous fluids, or antibiotics, depending on the severity (1–3). V. cholerae is spread from person to person via the fecal-oral route or indirectly through contaminated food and water (3). Cholera is endemic in many regions of Africa and Asia, where seasonal or sporadic outbreaks occur (4–7), predominantly in countries with poor sanitary conditions, such as open defecation, unhygienic food handling, and limited access to safe drinking water (8).
Vibrio cholerae is of major public health concern due to its potential to cause pandemics. Since 1817, there have been seven cholera pandemics, with the seventh beginning in 1961 and continuing until today. In 2015, the estimated annual incidence of cholera was 1.3–4 million cases, resulting in 21,000–143,000 deaths (9). However, the notification of cholera cases to the WHO is not mandatory; therefore, it is an underreported disease in many countries (9). For several reasons, the true burden of cholera is underestimated. For instance, it is often difficult to differentiate cholera from other acute diarrheal diseases based on clinical observation. Additionally, diagnostic and epidemiological surveillance laboratories may be deficient or even absent in cholera-endemic areas, thereby limiting accurate etiological diagnosis. It is likely that many cholera-associated cases and deaths do not present to health facilities and are therefore not included in the reports. Added to this, in some countries, there might be disincentives to report cases due to the possible negative impact on tourism and the export industry (10). Recently, the SARS-CoV-2 pandemic has affected cholera surveillance in many regions (11, 12), and there were 65% fewer cases reported to the WHO in 2020 than in 2019 (13). At the same time, preventive measures implemented during the pandemic, such as handwashing, hygiene promotion, social distancing, and banning of large gatherings, likely reduced cholera transmission. The extent to which the SARS-CoV-2 pandemic affected cholera surveillance and epidemiology is currently unknown (14, 15). Thus, cholera remains a leading cause of morbidity and mortality in several developing and resource-poor countries (14).
Cholera is a preventable and treatable disease, and several strategies can be used to control it (Box 1). In 2017, the Global Task Force for Cholera Control proposed an ambitious plan to eliminate endemic cholera in 20 countries and reduce cholera deaths by 90% by 2030 (22). The plan, called “Ending Cholera: A Global Roadmap to 2030,” focuses on strengthening public health systems, improving surveillance for early detection of cholera outbreaks, improving drinking water, sanitation, and hygiene conditions, making oral rehydration treatments more accessible, and increasing vaccination coverage.
BOX 1 Cholera prevention and control strategies.
• Improved sanitation and access to drinking water: This disease is primarily spread through the consumption of contaminated water or food. Therefore, improving access to drinking water and sanitation facilities can contribute to reducing the risk of cholera transmission (16).
• Early detection and prompt treatment: Rapid detection of cholera cases and adequate treatment can reduce the spread of the disease and decrease the number of deaths. Rapid diagnostic tests are useful in this regard (17).
• Vaccination: Oral cholera vaccines (OCVs) have been shown to be effective in preventing cholera and should be used as part of a comprehensive cholera control strategy, especially in endemic areas or during outbreaks (18).
• Health education: Education campaigns can help to raise awareness about cholera and how to prevent it. These campaigns should include information on proper food storage and preparation, hand washing, and recognizing the signs and symptoms of cholera (19).
• Strengthening health systems: A strong health system is crucial for effective prevention, detection, and response to cholera. This requires trained health workers, laboratory capacity, and adequate supplies of vaccines, antibiotics, and oral rehydration solutions (20).
• Antimicrobial resistance (AMR) surveillance: Severe cholera is treated with antibiotics, but the emergence of antibiotic-resistant strains can make treatment more difficult. AMR surveillance is essential to ensure appropriate antibiotic use and prevent the spread of resistant strains (21).
• International cooperation: Cholera is a global health problem and requires a coordinated global effort. The WHO, along with non-governmental organizations (NGOs) and other international organizations, plays a key role in coordinating efforts to control cholera.
While these strategies can help to control the burden of cholera and prevent large outbreaks, it is important to note that V. cholerae will likely never be completely eradicated, as this bacterium is ubiquitous in aquatic environments.
Antibiotic prophylaxis can theoretically prevent both human-to-human and environment-to-human cholera transmissions. Also, some field trials have suggested that chemoprophylaxis has a protective effect among household contacts of people with cholera (23, 24). However, due to the risk of resistance selection, antibiotic prophylaxis for close contacts, as well as for travelers arriving in or departing from cholera-affected areas, is not usually recommended (25).
Efforts and research directed toward the development of cholera vaccines date back more than a century. The first cholera vaccine, a live whole-cell injectable formulation, was developed in 1885 (26). A few years later, killed and attenuated cholera vaccines were reported in 1888 and 1892, respectively (27). Other injectables cholera vaccines were developed throughout the first half of the 20th century. However, all these vaccines had low levels of protective efficacy (PE) and a concerning history of adverse effects (28).
The start of the seventh cholera pandemic in the 1960s and the spread of this disease throughout Asia and Africa led to increased international interest and funding for cholera research, resulting in the development of the first oral cholera vaccine (OCV). It should be noted that current OCVs exhibit variable PE in human populations for several reasons, including the presence of different V. cholerae strains in endemic areas, immunization coverage, malnutrition, co-infections, and variations in the gut microbiome (29, 30). Thus, a cholera vaccine that provides broad and long-lasting protection remains elusive.
In this review, we will discuss recent advances in understanding the V. cholerae pathogenesis and immunity against cholera, as well as the current status of approved cholera vaccines. Lastly, we discuss how all this knowledge gained could lead to the development of next-generation cholera vaccines.
2. Vibrio cholerae classification
Vibrio cholerae is divided into more than 200 serogroups determined by the structure of the O-antigen of lipopolysaccharide (LPS) (Figure 1A). Among them, a subset of strains belonging to serogroups O1 and O139 can cause cholera and epidemics due to their ability to produce cholera toxin (CTX). Serogroups that are not O1 and O139, collectively referred to as non-O1/non-O139, typically lack the CTX and cause small gastroenteritis outbreaks, sporadic cases of bacteremia, and wound infections, but they do not cause cholera (31–33). Unlike O1, more than 85% of non-O1 serogroups (including O139) have a capsule that is critical for virulence in extraintestinal infections (34).
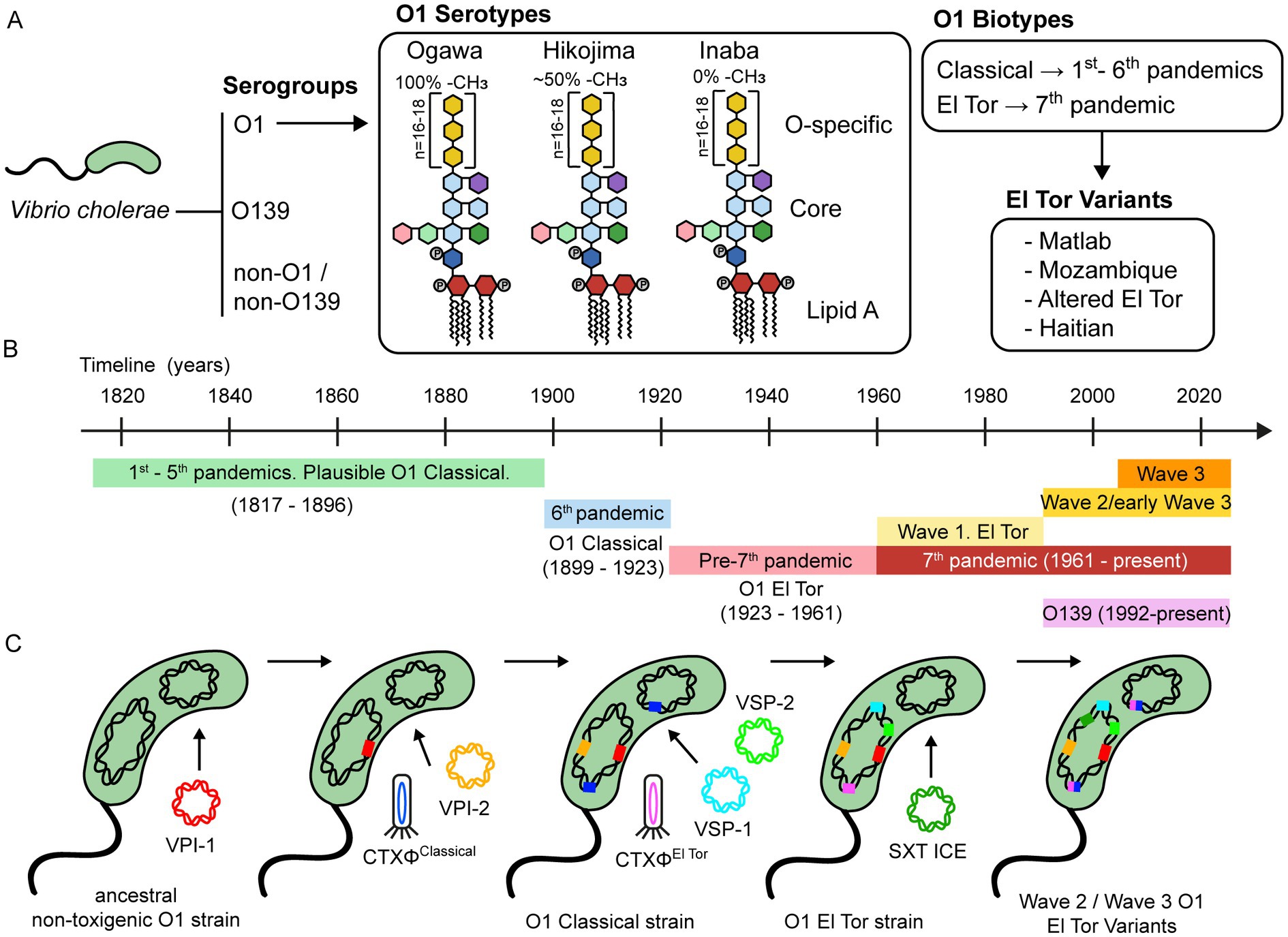
Figure 1. Classification and evolution of V. cholerae. (A) V. cholerae is classified into serogroups based on the composition of the O antigen of LPS. Strains belonging to the O1 serogroup are further divided into three serotypes, namely Ogawa, Hikojima, and Inaba. The LPS of these three serotypes is schematically represented, showing the approximate percentage of methylation of the terminal perosamine. Serogroup O1 is also classified into the Classical and El Tor biotypes, based on phenotypic and genetic markers. Over the past two decades, there has been a growing number of reports on V. cholerae strains that possess genetic features from both the Classical and El Tor biotypes, leading to the emergence of hybrid or variant strains. These strains have been linked to several cholera outbreaks worldwide and have contributed significantly to the global burden of this disease. (B) Timeline of the history of cholera pandemics. (C) A schematic representation of the evolutionary process underlying the development of virulence in serogroup O1. This process is mainly driven by the acquisition of mobile genetic elements, including bacteriophages, genomic islands, integrative and conjugative elements, among others.
Furthermore, O1 strains are divided into three serotypes, designated Ogawa, Inaba, and Hikojima, which are grouped according to the methylation status of the terminal perosamine of the LPS. Ogawa strains are methylated, Inaba strains are unmethylated, and Hikojima strains express both methylated and unmethylated O-antigens. While the Ogawa and Inaba serotypes can co-circulate during epidemics and are capable of interconverting (35), the Hikojima serotype is rare, and evidence indicates that it is an unstable transitional form that results when a strain undergoes serotype switching from Ogawa to Inaba (36).
Biotype is another key classifier of V. cholerae O1 strains. Classical and El Tor biotypes can be distinguished according to a set of phenotypic and genetic markers (37, 38). Interestingly, there are some differences in the infection patterns between both biotypes. El Tor strains are more efficient at host-to-host transmission, survive better in the environment and the human gut, and have a higher occurrence of asymptomatic than symptomatic carriers, compared to the Classical strains (39).
3. Cholera epidemics and pandemics
It seems that the first five cholera pandemics were caused by Classical biotype strains (1817–1896) (Figure 1B) (40). After this, the sixth cholera pandemic (1899–1923) was caused by the Classical biotype. The Classical biotype was prevalent until the 1960s, but during the pre-seventh-pandemic period (1923–1961), some sporadic outbreaks associated with the El Tor biotype were reported. The ongoing seventh pandemic (1961 to date) is caused by the El Tor biotype (41). Notably, after the emergence of the El Tor biotype, the Classical biotype declined and disappeared by the 1980s, and it is now considered extinct (42).
Eight distinct phylogenetic lineages have been identified based on whole-genome sequencing and genomic analyses of different pandemic strains. The L1 and L3-L6 lineages include Classical strains from the first six pandemics. The L2 lineage includes the El Tor strains of the seventh pandemic (7PET) and is subdivided into three clades (waves 1–3) that represent independent waves of transmission (43). Subsequent analysis reported subclades within individual waves and several transmission events, namely, T1-T12 from African countries, LAT-1 to LAT-3 from Latin America, and T13 from East Africa and Yemen (43–45).
Wave 1 strains were prevalent between 1961 and the early 1990s. During the 1990s, serogroup O139 emerged and caused cholera epidemics in Southeast Asia, but its incidence declined a few years later, and it is now rarely isolated. At the same time, Wave 2 and early Wave 3 strains emerged and replaced Wave 1 strains. Interestingly, many Wave 2 and Wave 3 strains display a mix of phenotypic and genotypic traits of Classical and El Tor biotypes, suggesting that they are genetic hybrids (37). These hybrid strains include the Matlab variants from Bangladesh, the Mozambique variants, the Haitian variants, and the altered El Tor biotype from various parts of the world (46). While Wave 2 strains have waned since the 2000s, Wave 3 strains are now the dominant cause of cholera globally (47).
4. Genome and evolution of virulence of Vibrio cholerae
The genus Vibrio commonly harbors two nonhomologous circular chromosomes, Chr1 and Chr2 (48). The first complete genome sequence of a V. cholerae strain was announced for the clinical isolate O1 El Tor Inaba N16961 (49). Genomic analysis of this strain revealed that Chr1 has 2.96 Mb with a 47.7% G + C content, while Chr2 has 1.07 Mb with a 46.9% G + C content. Chr1 contains a large number of genes for essential cellular functions, such as DNA replication, transcription, translation, and cell-wall biosynthesis, as well as virulence genes encoding toxins, adhesins, and surface antigens. By contrast, the Chr2 has fewer such genes and contains a very large integron comprising genes with diverse functions. Comprehensive analysis of both chromosomes revealed the presence of a suite of mobile genetic elements (MGEs), including prophages, genomic islands (GIs), and integrative and conjugative elements (ICEs) (49). Table 1 describes a select list of MGEs that are important in pandemic V. cholerae strains.
The genomic plasticity of V. cholerae and its ability to exchange genes through natural transformation, conjugation, and transduction are hallmarks of this bacterium. Its evolution is continuous due to the acquisition or loss of genomic segments (58, 59). The acquisition of MGEs is known to be the major driver for the evolution of V. cholerae virulence and a determinant of genetic divergence between environmental and pandemic strains (60, 61). In this respect, understanding the evolutionary events that lead to the emergence of pandemic clones of V. cholerae might provide new approaches for controlling this pathogen.
Chun et al. (62) proposed a hypothetical evolutionary pathway for the emergence of the seventh pandemic V. cholerae strains (Figure 1C). According to this model, the diversification of a common ancestral strain occurred through the sequential acquisition of MGEs, likely driven by environmental factors. After acquiring the O1 antigen, an O1 progenitor strain probably acquired the Vibrio pathogenicity island-1 (VPI-1) and Vibrio pathogenicity island-2 (VPI-2), which are ubiquitous among strains from the sixth (Classical biotype) and seventh (El Tor biotype) pandemics (63). VPI-1 encodes the toxin-coregulated pilus (TCP), which is the receptor for bacteriophage CTXΦ. Thus, transduction by the CTXΦ must have been preceded by the acquisition of VPI-1. The divergence between the Classical and El Tor biotypes was due to the acquisition of distinct bacteriophages CTXΦ and the Vibrio seventh pandemic islands (VSP-1 and VSP-2). Several lines of evidence support this. For example, comparative nucleotide sequence analyses have revealed that the CTXΦ from Classical and El Tor biotypes comprise two distinct lineages, indicating that they were acquired in independent events (64–66). In addition, VSP-1 and VSP-2 are consistently found in the O1 El Tor and O139 strains but are predominantly absent in the O1 Classical strains isolated between 1817 and 1923 (40, 59).
Horizontal gene transfer events have also occurred among strains from the seventh pandemic. Unlike Wave 1 strains, Wave 2 and Wave 3 strains contain a self-transmissible integrative conjugative element that carries multiple antibiotic-resistance genes (SXT ICE). The acquisition of SXT ICE likely influenced the population shift from the Wave 1 to Wave 2/3 strains (43). Interestingly, O139 strains that emerged in the 1990s also harbor the SXT ICE (56). In addition, Wave 2 and Wave 3 strains have undergone multiple CTXΦ substitutions and replacements, leading to the emergence of El Tor variant strains (47, 67).
5. Pathogenesis of Vibrio cholerae
In this section, we will review the current understanding of the pathogenesis of toxigenic V. cholerae strains, particularly the O1 serogroup. Much of this information has been obtained from in vitro assays and challenge experiments in animal models, although some findings have been subsequently confirmed in human infections. Table 2 provides a summary of the main virulence factors of V. cholerae that are expressed during infection, and Figure 2A depicts some of these virulence factors.
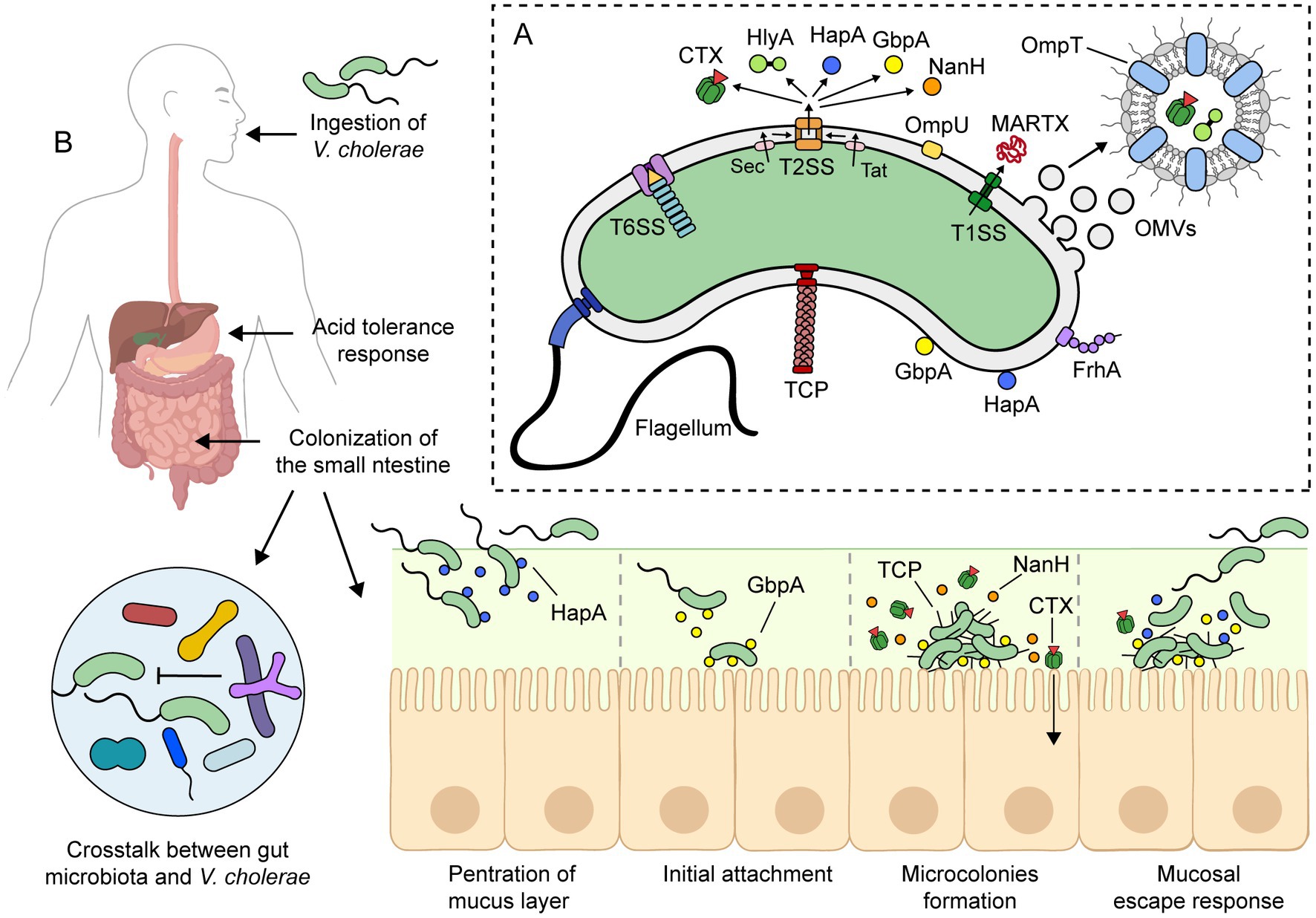
Figure 2. Pathogenesis of toxigenic V. cholerae. (A) Toxigenic V. cholerae produces several virulence factors that contribute to its pathogenesis. The precise pathogenic mechanism is not yet fully understood, but it is widely accepted that it involves the combination of these virulence factors and the ability to colonize and persist in the small intestine. (B) Upon ingestion, V. cholerae survives the low pH of the stomach via an acid tolerance response. In the small intestine, V. cholerae uses its flagellum to propel through the mucus layer and reach the epithelial surface. Meanwhile, V. cholerae must overcome host immunity and the colonization resistance mechanisms of the gut microbiota. To colonize the small intestine, it expresses virulence factors such as toxin-coregulated pilus (TCP) and cholera toxin (CTX). During infection, other factors such as HapA, GbpA, and NanH are also expressed. For more details on the roles of these virulence factors, please refer to the text. This figure was created using BioRender.com.
The incubation period of cholera can range from 12 h to 5 days (106, 107). Once ingested, V. cholerae must rapidly adapt to the human digestive system (Figure 2B). To accomplish this, the bacterium uses a complex signal transduction network that regulates gene expression in response to different environments and stimuli throughout the gastrointestinal tract.
Vibrio cholerae is highly sensitive to low pH, and during passage through the stomach, the vibrios undergo an acid tolerance response (ATR) to both inorganic and organic acid. ATR involves several proteins, including the porin OmpU and the transcriptional regulators CadC and HepA, among others (108–110). Despite the ATR, the number of vibrios reaching the small intestine is reduced. In fact, a high infectious dose (108 bacteria) is required to cause severe cholera in healthy volunteers, while a lower dose (105 bacteria) is sufficient when given with antacids to neutralize stomach acid (28, 111).
Upon reaching the small intestine, the main site of colonization, adaptation to antimicrobial agents, such as bile salts and antimicrobial peptides, is crucial. To achieve this, the bacterium modulates its outer membrane protein (OMP) profile through the activation of a tightly regulated signaling pathway known as the ToxR Regulon (112). In the presence of bile salts, ToxR upregulates the expression of OmpU and downregulates the expression of OmpT, two of the most abundant OMPs of V. cholerae (113). The change of the OMP composition also involves removal of OmpT by outer membrane vesicle (OMV) production (114). OmpU and OmpT have distinct channel properties: OmpU is more cation-selective than OmpT, and the bile salt deoxycholic acid blocks OmpT porin activity but not that of OmpU (115, 116). Therefore, OmpU confers resistance to bile salts and antimicrobial peptides, playing a crucial role in the colonization and survival of V. cholerae in the small intestine (87, 88). Other intestinal environmental signals, such as bicarbonate, mucin, and osmolarity, also modulate the expression of virulence factors in V. cholerae (117–120).
To successfully colonize the small intestine, V. cholerae must penetrate a highly viscous mucus layer that is approximately 100–400 μm thick (121), or roughly 30–130 times the size of the bacterium. For this, the vibrios use their flagellum to propel through the mucus layer and reach the epithelial surface (122). It is worth noting that nonmotile vibrios are significantly less efficient at colonization or even avirulent (84). Additionally, the penetration of the mucus layer is facilitated by the hydrolysis of mucins by a group of enzymes, such as HapA, TagA, among others (91, 93–95, 123). Vibrios that fail to penetrate the mucus layer do not colonize the intestinal mucosa and are shed in the feces due to the continuous production and replenishment of mucus (124).
Meanwhile, V. cholerae needs to overcome host immunity (see next section) and the colonization resistance mechanisms of the gut microbiota (125). In this respect, mucin activates the V. cholerae type VI secretion system (T6SS), which operates as a molecular syringe that kills bacterial competitors through the contact-dependent translocation of toxic effectors (104, 126). In mice, V. cholerae T6SS has been shown to attack members of the host commensal microbiota, facilitating intestinal colonization (105). Moreover, T6SS has been suggested as a key mechanism conferring enhanced fitness to pandemic V. cholerae strains (127). However, secondary bile acids generated by gut microbiota can inhibit the assembly of the T6SS apparatus (126). Recently, differences in the gut microbiota among individuals have been suggested as a possible explanation for the susceptibility or resistance to cholera (125, 128).
The initial attachment of V. cholerae to intestinal epithelial cells (IECs) is likely mediated by the GbpA protein. GbpA is regulated by quorum sensing and is expressed at low cell density (129). Additionally, GbpA stimulates mucin secretion by IECs, which in turn enhances GbpA expression (130). GbpA has been shown to bind mucin, and deletion of its encoding gene decreases intestinal colonization in the infant mouse model (100, 101, 130). Other adhesive factors that could play a role in attachment to the intestinal epithelium are the OmpU and FrhA proteins (90, 102, 131).
After attachment to the intestinal epithelium, V. cholerae decreases its motility, proliferates, and forms microcolonies, mostly originating from single vibrio cells (95). Colonizing vibrios express CTX and toxin-coregulated pilus (TCP), which are their main virulence factors. CTX is responsible for the secretory diarrhea characteristic of cholera, while TCP mediates adherence and microcolony formation. Both acidic bile and bicarbonate have been shown to induce CTX and TCP expression via the ToxR regulon (112, 119, 132). Importantly, TCP-deficient mutant strains are unable to colonize animal models and the human intestine (133–135).
CTX is secreted into the extracellular milieu through the type II secretion system (T2SS) (69). Then, the cellular uptake of CTX occurs via endocytosis, mediated by the binding of CTX-B pentamer to GM1 ganglioside receptors located on the surface of IECs (Figure 3). Of note, NanH cleaves sialic acid from high order gangliosides to release sialic acid and expose the GM1 ganglioside (96, 97). Therefore, NanH promotes the internalization of CTX and its toxigenic effects (139). Although GM1 is considered the primary receptor of CTX, recent studies suggest that CTX-B also binds histo-blood group antigens (HBGAs) at a secondary binding site (140). Additionally, CTX can be released as cargo inside OMVs, which protects the toxin from degradation by intestinal proteases, potentially preserving its toxic effects for longer periods of time (70–72). In particular, CTX-containing OMVs have been shown to be internalized by caveolin-mediated endocytosis in a GM1-independent mechanism that appears to require the presence of OmpU on the vesicle surface (71). After CTX is internalized, cAMP signaling in the IECs is impaired, resulting in a massive release of electrolytes and water into the intestinal lumen, leading to diarrhea (137). The mechanism of action of CTX is described in detail in Figure 3C. Furthermore, other accessory toxins produced by this pathogen can contribute to impaired epithelial barrier function and the development of diarrhea (141). Although 90–95% of infected individuals remain asymptomatic or experience mild symptoms, the remaining 10% develop severe cholera, characterized by profuse watery diarrhea (25). This diarrhea is often described as “rice-water stool” due to its pale, milky appearance (28).
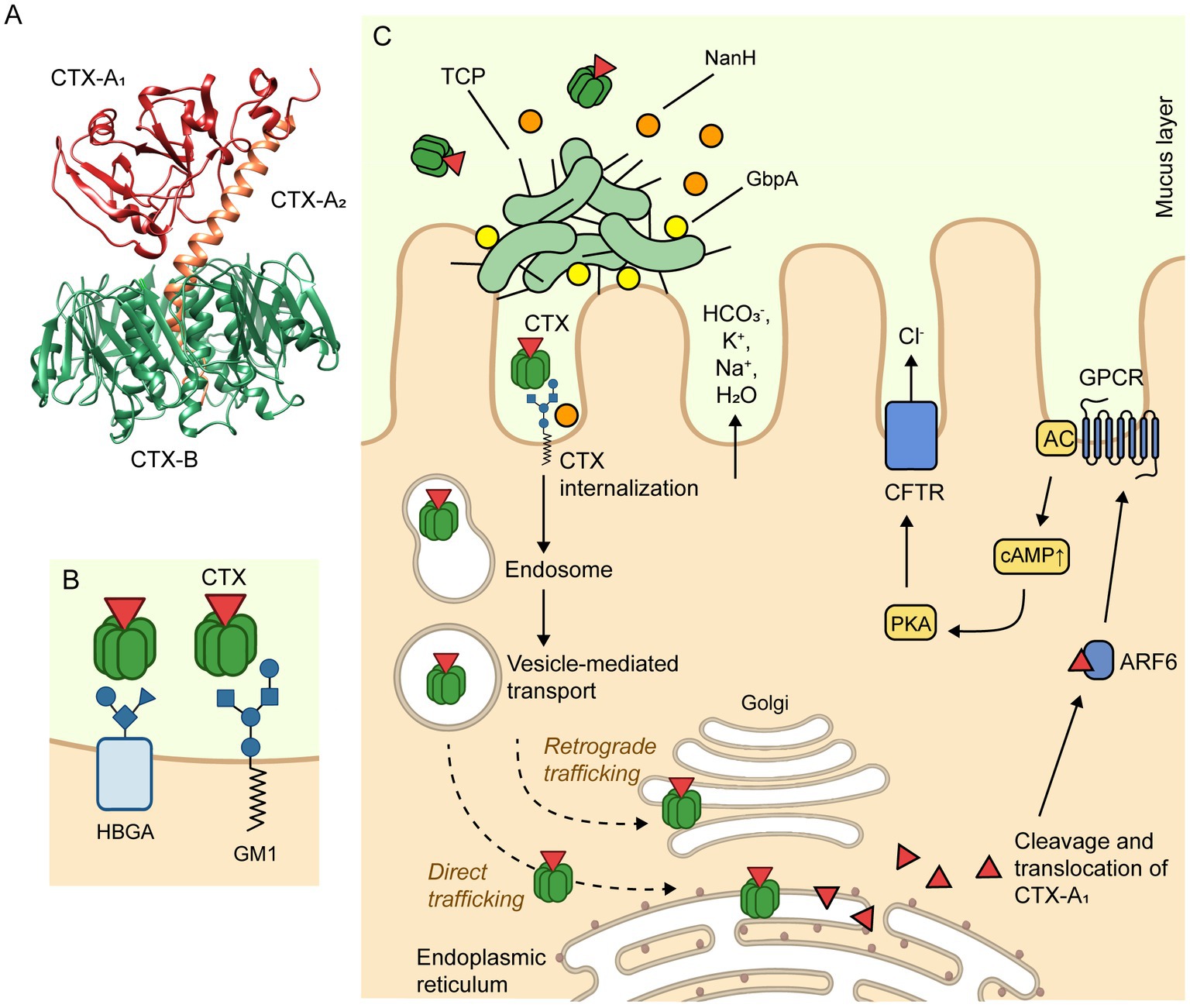
Figure 3. Mechanism of action of cholera toxin. (A) The crystal structure of CTX (PDB accession number 1XTC) was determined by Zhang et al. (136). CTX is composed of a heterodimeric CTX-A subunit, which consists of two polypeptide chains, CTX-A1 (22 kDa) and CTX-A2 (5 kDa), linked by a single disulfide bond. The CTX-A2 helical peptide links the CTX-A1 chain to the pentameric CTX-B subunit, which is composed of five identical polypeptide chains (11.6 kDa). (B) The CTX-B pentamer specifically binds to GM1 gangliosides (primary receptor) or histo-blood group antigens (HBGAs; secondary binding site) present on the apical side of intestinal epithelial cells, promoting the endocytosis of the toxin. (C) The internalization of CTX may occur through clathrin-dependent as well as caveolae- and clathrin-independent endocytosis. Regardless of the mechanism of endocytosis, the CTX is internalized to the early endosomal compartment, trafficked to the Golgi, and then onto the endoplasmic reticulum (ER), where it dissociates into a CTX-A1 and a CTX-A2/CTX-B complex. Next, the CTX-A1 is exported out of the ER to the cytosol, where it is activated by ADP ribosylation factor 6 (ARF6). The ARF6-bound, activated CT-A1 subunit, in turn, activates adenylyl cyclase (AC) by catalyzing ADP ribosylation of a G protein-coupled receptor (GPCR). The AC then catalyzes the conversion of ATP to cyclic adenosine monophosphate (cAMP), increasing the intracellular cAMP concentration. This leads to the activation of protein kinase A (PKA), which phosphorylates the cystic fibrosis transmembrane conductance regulator (CFTR) chloride channel proteins, ultimately resulting in the release of electrolytes (Cl−, HCO3−, Na+, K+) and water into the intestinal lumen, causing the secretory diarrhea characteristic of cholera (137, 138). The figure was created with BioRender.com.
In the late phase of infection, microcolonies of vibrios reach a high cell density, and the nutrients in the intestine decrease. Consequently, vibrios switch from rapid replication to bacteriostasis and downregulate the expression of major virulence factors. Some of them become motile and detach from the epithelial surface moving into the luminal fluid. This process, known as the “mucosal escape response,” is dependent on the general stress response regulator RpoS and the quorum sensing regulator HapR (142–145). Moreover, detachment of vibrios from the intestinal cells is facilitated by the HapA protease, which degrades the GbpA adhesin (129).
Lastly, individuals without effective antibiotic treatment may shed vibrios in their feces for up to 10 days after infection, releasing the bacteria into the environment and increasing the risk of further infections (25). Interestingly, vibrios shed in rice water stool are in a hyperinfectious state (146). These hyperinfectious vibrios are flagellated and highly motile, but most known virulence genes, including those for CTX and TCP, as well as those associated with chemotaxis, are downregulated (147). The exact mechanism for the regulation of the hyperinfectious state remains unknown. In any case, hyperinfectivity is a transient state and is maintained only for a few hours after shedding from the patients (148). Thus, the hyperinfectious state could play a role in the spread of cholera when transmission to another person occurs in a relatively short period of time (149). It is also worth noting that asymptomatic individuals (healthy carriers) are mostly short-term carriers and short-term shedders of vibrios but play an important role in the persistence and transmission of the disease (150).
6. Immune response to cholera
Numerous experimental and epidemiological studies have documented that V. cholerae infection induces protection against reinfection for at least 3 years in most patients who recover. In this respect, cholera confers greater protection than a subclinical infection (151). However, several factors can affect the immune response against V. cholerae and the consequent establishment of immunological memory, including age, nutritional status, blood group, endemicity, co-infections, microbiota, and others (152).
Although the exact mechanism behind protective immunity against cholera remains largely unknown, our current understanding of V. cholerae pathogenesis offers some insight into how this bacterium interacts with the intestinal mucosa and triggers multiple arms of the immune system (Figure 4).
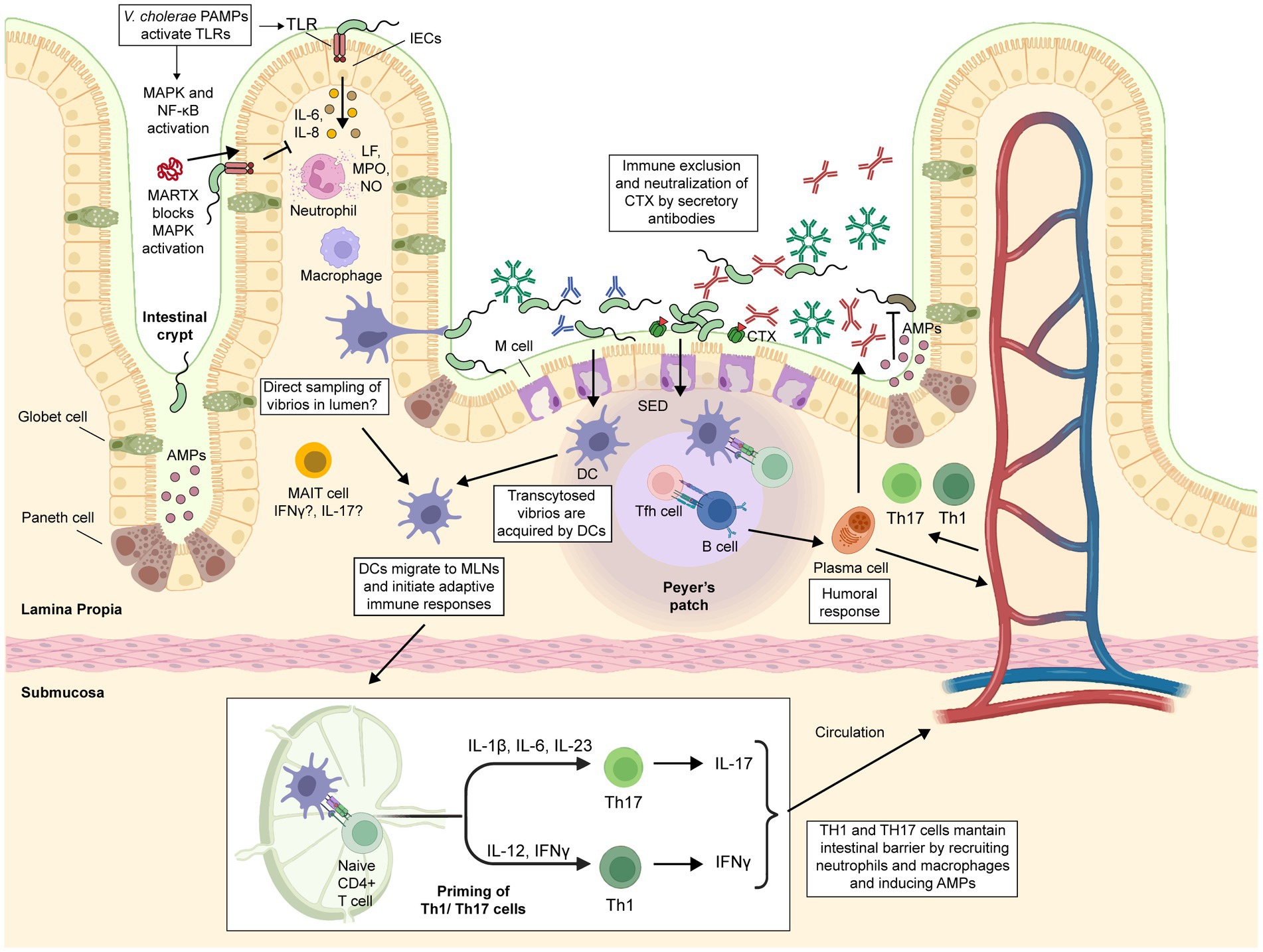
Figure 4. Immune response against cholerae infection. Intestinal epithelial cells (IECs) serve as a physical barrier that limits bacteria to the intestinal lumen. They detect PAMPs such as LPS, flagellin, CTX and OmpU, triggering the secretion of proinflammatory cytokines that recruit innate immune cells such as macrophages, dendritic cells (DCs), and neutrophils. Activated neutrophils increase the inflammation of the intestinal lumen through metabolites such as lactoferrin (LF), myeloperoxidase (MPO), and nitric oxide (NO). M cells take up and transport vibrios from the intestinal lumen to the subepithelial dome (SED) region in Peyer’s patches, where DCs engulf them. Activated DCs migrate to mesenteric lymph nodes, where they produce Th17 or Th1-driving cytokines. Macrophages can also contribute to Th17 or Th1 differentiation through the secretion of IL-23 and IL-6 or IFNγ, respectively. Th1, Th17, and Tfh cells induce B-cell differentiation and expansion. Mucosal-associated invariant T (MAIT) cells are present and highly activated in the lamina propria of the duodenum of cholera patients, but their exact role in the protection against cholera remains to be determined. Secretory antibodies (sIgA and sIgM) prevent vibrios from attaching to the epithelium, blocking their access to the epithelial surface and facilitating their removal through peristaltic activities. Some IgG antibodies could enter the intestinal lumen via passive leakage through a damaged and leaky epithelium. The figure was created with BioRender.com.
6.1. Innate immune response
Cholera has traditionally been considered a noninflammatory diarrheal disease; however, this concept has been re-evaluated, and now it is known that some inflammation occurs during the acute phase of the infection, which is followed by a non-inflammatory convalescent phase (153, 154). In fact, patients with cholera in the acute phase exhibit ultrastructural changes in the duodenal mucosa, such as the widening of the intracellular spaces and alterations of the apical junctional complexes. Moreover, these changes correlate with clinical severity and are characterized by the infiltration of innate immune cells, strongly suggesting an inflammatory response at the site of infection (155).
In the small intestine, IECs play a fundamental role in the defense against enteric pathogens. First, IECs constitute a physical barrier that restricts bacteria to the intestinal lumen. Second, they act as sensors to detect pathogen-associated molecular patterns (PAMPs) and release cytokines that recruit immune cells to the site of infection (156). Mechanistically, during the onset of V. cholerae infection, several immunogenic components of this pathogen, such as LPS, flagellins, CTX, and OmpU, can act as PAMPs and be recognized by extracellular and intracellular pattern recognition receptors (PRRs). This triggers the activation of central innate immune pathways, including the myeloid differentiation primary response gene 88 (MyD88), mitogen-activated protein kinase (MAPK), and nuclear factor kappa light-chain enhancer of activated B cells (NF-κB), which in turn activate the secretion of several proinflammatory cytokines (154, 157–162).
For example, V. cholerae flagellins induce the expression of IL-1β and IL-8 in IECs by interacting with Toll-like receptor 5 (TLR5) and activating NF-kB and MAPK pathways (159, 161). Likewise, OmpU induces the expression of IL-6, IL-8, and MCP-1 (CCL2) in IECs (163, 164). Moreover, CTX increases intracellular cAMP, leading to IL-6 secretion in IECs (165, 166). These studies were further supported by a transcriptomic analysis of IECs from patients with cholera in the acute phase, where an upregulation of several genes associated with innate immunity was observed (160). Remarkably, activation of the MAPK and NF-κB pathways persisted even 30 days after infection. Furthermore, multiple TLRs, including cell surface TLRs 1, 2, 4, 5, and 6, as well as the endosomal TLRs, including TLR3, TLR7, and TLR8 were upregulated (160).
Among the cytokines mentioned, IL-8 is a potent chemoattractant that recruits polymorphonuclear leukocytes and T cells to the infection site, and stimulates neutrophil degranulation and phagocytosis (167, 168). In addition, the CCL2 chemokine induces the migration of monocytes, dendritic cells, and memory T-cells (169, 170), while IL-6 secretion by IECs activates neutrophil degranulation in the intestinal lumen (166). As a result, innate immune cells, particularly neutrophils, are recruited to the site of infection during the acute phase of cholera (153, 155, 171). Furthermore, a recent study showed that mucosal-associated invariant T (MAIT) cells, an innate-like lymphocyte known to provide immediate effector functions in response to infections, are present and highly activated in the lamina propria of the duodenum of cholera patients (172). Recent evidence suggests that MAIT cells can also provide B cell help and support antibody production at the mucosa level (173); however, further investigation is needed to determine the exact role of MAIT cells in the protection against cholera.
Once neutrophils arrive at the infection site, they express metabolites such as lactoferrin (LF), myeloperoxidase (MPO), and nitric oxide (NO) (153, 174, 175). This may explain the detection of elevated levels of LF and MPO in stools and NO metabolites (NO2−/NO3−) in serum of patients with cholera during the acute phase (174, 175). Of note, V. cholerae is highly susceptible to the bactericidal action of LF (176, 177). By contrast, in the convalescence phase (~ up to 30 days post infection), an increase of mast cells and eosinophils and their respective effector molecules chymase and IL-3 have been reported (153). Consequently, the activation of proinflammatory signaling pathways, the recruitment of innate immune cells, and their effector functions are fundamental in the initial defense against V. cholerae.
Despite the above, V. cholerae has some strategies to evade the innate immune response of the host (178). A recent study demonstrated that the accessory MARTX (multifunctional-autoprocessing repeats-in-toxin) toxins secreted by some V. cholerae strains can block the MAPK signaling pathway in T84 cells grown in vitro. Suppression of MAPK signaling in IECs prevented the recruitment of innate immune cells, and thus this mechanism could protect colonizing vibrios from neutrophil-mediated clearance (74). Importantly, the immunomodulatory effect of these toxins may contribute to the differences in inflammation observed between various V. cholerae strains (158). In fact, the current predominant circulating altered El Tor strains lack the MARTX toxins due to a stop codon (179), which could explain in part why these hybrid strains cause a more severe diarrhea and increased intestinal inflammation (158). This raises the question: are innate immune responses in cholera beneficial or detrimental to the host? It is likely that adequate tuning of the innate immune system and a subsequent moderate inflammatory response can be protective against severe cholera.
The gut-associated lymphoid tissues (GALT) play a vital role in developing immunity following natural exposure to enteric pathogens (180). GALT is divided into inductive sites, such as the Peyer’s patches (PP) and mesenteric lymph nodes (MLNs), and effector sites, such as the lamina propria and the intraepithelial lymphocyte compartment (181). Consequently, upon exposure to V. cholerae, protective immunity against this pathogen is expected to largely depend on cellular processes that occur in GALT. In fact, in the rabbit ileal loop model, M cells take up and transport vibrios from the intestinal lumen to the subepithelial dome (SED) region in the PP (182). Thus, it is likely that resident DCs and macrophages in the SED become activated and then phagocytose these vibrios during infection in humans.
CTX induces an increase in intracellular cAMP in innate immune cells, leading to the production of IL-1β, TNF-α, and IL-6 (183). In particular, it has been shown that CTX has an immunomodulatory effect on DCs by stimulating their maturation, as well as the expression of MHC class II and costimulatory molecules (184, 185). CTX activates DCs to produce Th17-driving cytokines, including IL-6, which promotes the differentiation of Th17 cells (186, 187). Significantly, CTX also induces the migration of DCs from the SED region to B and T cell zones, where antigen presentation occurs (188). Therefore, the DCs activated by CTX can induce strong humoral and cellular immunity.
Some insights into the role of macrophages against cholera infection have been obtained using the THP-1 human monocyte-like cell line. THP-1 cells exposed to live toxigenic V. cholerae upregulate IL-23 expression (189). In another study, THP-1 cells stimulated with V. cholerae LPS exhibited increased expression of TNF-α, IL-1β, and MIP-3α through interaction with TLR4 and subsequent activation of the MyD88 pathway (190). It is important to note that both IL-23 and IL-1β are essential for the differentiation of Th17 cells (191, 192).
6.2. Adaptive immune response
The subsequent adaptive immune response is complex and involves both humoral and cellular mechanisms. In the acute stage of cholera, studies have shown that lamina propria lymphocytes (LPLs) in the duodenum express significant amounts of IL-6, IL-8, IL-12β, and IL-17 cytokines (162, 193). Later, at day 7 post-infection, cholera induces cellular immune responses in blood compatible with Th1 (IFN-γ) and Th17 (IL-17) profiles (193). Additionally, patients who recover from cholera display an increased percentage of gut-homing CD4+ T cells and gut-homing B cells that peak in the circulation 7 days after the infection. Th17 lineage and other IL-17-producing cells play a key role in host defense against bacteria at mucosal surfaces (191), making the Th17 response to V. cholerae highly significant. By contrast, the level of gut-homing CD8+ T cells reaches its peak in circulation on day 21 (194, 195).
Cholera also triggers follicular helper T (Tfh) cells, which are essential for germinal center formation, affinity maturation, and the development of most high-affinity antibodies and memory B cells (196). A recent study demonstrated that cholera infection in the acute phase induces a significant increase in circulating Tfh cells, which impacts the development of antigen-specific B cells and consequent immunoglobulin production (197).
Considering the pathogenesis of V. cholerae, a humoral response capable of neutralizing the CTX, blocking bacterial adherence to the mucosa, and opsonizing the bacteria to mediate their clearance is expected. Consistently, patients who recover from cholera develop systemic IgM, IgG, and IgA antibodies, as well as secretory IgA (sIgA) antibodies, which target several antigens, including CTX-A and CTX-B subunits, LPS, O-specific polysaccharide (OSP), LPS, NanH, flagellins (FlaB, FlaC, and FlaD), TcpA, and HlyA (139, 198–204). Nevertheless, while CTX-specific antibodies confer short-term immunity, the antibacterial (vibriocidal) antibodies are associated with protection against colonization and long-term protective immunity (205).
The best-characterized correlate of protection against cholera is the vibriocidal antibody titer (VAT), which measures the minimum concentration of serum required for antibody-dependent complement-mediated bacterial killing (206). However, VAT is not a comprehensive predictor of long-term immunity. For instance, a specific VAT threshold for absolute protection has not been identified; infection still occurs in a few individuals with very high titers (207). Moreover, there is a lack of mechanistic connection between levels of circulating VAT and prevention of V. cholerae colonization at the intestinal mucosal surface level. At the same time, anti-body-dependent complement-mediated bacterial killing is relevant for immunity against systemic infections, it appears to be less important at the intestinal mucosa due to low complement levels at this site (206, 208).
The mechanism by which IgG enters the intestinal lumen is unclear, but it may occur via passive leakage through a damaged and leaky epithelium or through FcRn-mediated epithelial transport (209, 210). Notably, recent studies have demonstrated that IgG anti-OSP contributes to protection against cholera by inhibiting the motility of V. cholerae, potentially limiting its access to the apical surface of the intestine (211). It is also possible that anti-OSP sIgA may contribute to protection by inhibiting motility and trapping the bacteria at the mucosal surface (1, 212). Even in the absence of circulating anti-OSP antibodies, OSP-specific memory B cells may maintain protective immunity by rapidly expanding and differentiating into plasmablasts upon antigen exposure (213). Furthermore, a recent study showed that patients with cholera develop IgG, IgA, and IgM antibodies against NanH, and that plasma responses targeting this antigen correlate with protection (214). The protective role of other antibacterial antibodies against cholera is currently unknown.
Thus, this body of studies demonstrates that cholera infection stimulates innate cells at the site of infection, primarily neutrophils and their corresponding effector molecules. The subsequent adaptive response is characterized by Th1, Th17, and Tfh CD4+ cells, which shape the antibody production targeting the CTX and various surface-exposed antigens. However, there are currently many knowledge gaps in understanding how these immunological processes occur. In this regard, to develop a highly effective cholera vaccine, it may be necessary to mimic these immune responses. As such, progress should be made in understanding the differences between the immune response triggered by V. cholerae infection and that induced by vaccination. This could pave the way for the development of the next generation of cholera vaccines.
7. Current vaccines licensed worldwide or with restricted license
The knowledge gained on immunity against V. cholerae has not only facilitated the development of current cholera vaccines, but also highlighted the possibility of developing novel vaccines that provide broader and longer-lasting protection. In this section, we will briefly review licensed cholera vaccines, while subsequent sections will focus on candidate vaccines currently undergoing clinical and preclinical evaluation.
In the 1960s, several large field studies conducted in Asian countries showed that injectable killed whole-cell cholera vaccines had modest efficacy and a high rate of adverse reactions, such as fever, local pain and swelling (215). Subsequently, interest shifted to exploring the potential of oral vaccination, which led to the development of the OCVs. Oral vaccines mainly interact with the immune system through the Waldeyer’s tonsillar ring in the oral cavity or via the PP in the small intestine. Compared to vaccines administered by parenteral routes, oral vaccines have been found to induce stronger immune responses at the intestinal mucosa level, partly via antigen-specific sIgA (216). However, oral vaccines face some challenges, including the potential degradation of acid-labile antigens in the stomach, the lack of licensed oral adjuvants for human use, and the difficulty of their release at mucosal immune inductive sites (217).
At present, four OCVs based on killed whole cell vibrios are prequalified by the WHO (meaning that they can be purchased by United Nations agencies): Dukoral®, Shanchol™, Euvichol, and Euvichol-Plus (Table 3 and Box 2) (217).
BOX 2 Advantages and limitations of killed OCVs.Killed OCVs possess several characteristics that make them effective in combating cholera:
• Safety: These vaccines have been proven safe, with only minor side effects reported.
• Easy administration: They can be easily administered in mass vaccination campaigns and in settings where injection-based vaccines may be logistically difficult to implement.
• Cost-effectiveness: Killed OCVs are relatively inexpensive, making them accessible to populations in resource-limited areas where cholera is prevalent.
• Herd immunity: OCVs not only protect the individuals who receive them but also create herd immunity, which can help to decrease transmission of the disease in the community (218, 219).
While killed OCVs offer several benefits as a tool for controlling cholera, they also have some limitations:
• Limited effectiveness: Their effectiveness can vary depending on the vaccinated population and the level of cholera transmission in the area. The protection provided by OCVs is short-term and decreases over time.
• Limited immune response: These vaccines do not contain live bacteria; thus, the immune response elicited may differ from that triggered by a natural cholera infection. This difference may result in a different pattern of immune response and antibody production, which can affect the duration and quality of the immunity provided.
• Cold chain requirements: They must be stored at a specific temperature range (typically between 2 and 8°C) to preserve their immunogenic properties, which can be challenging in areas with limited infrastructure.
• Requirement for multiple doses: They require at least two doses to provide adequate protection, which can be a barrier to achieving high coverage in some settings.
• Limited role in outbreaks: They do not provide immediate protection against cholera and are not intended to replace other control measures.
Dukoral® was licensed in 1991 and since then has been distributed in over 60 countries. It is a monovalent vaccine composed of a mixture of three heat- or formalin-inactivated V. cholerae O1 strains (Classical Inaba strain Cairo 48, Classical Ogawa strain Cairo 50, and El Tor Inaba strain Phil 6973) along with the recombinant CTX-B (rCTX-B) subunit. The vaccine is free of the CTX-A subunit due to its toxicity. A sodium bicarbonate buffer is also added to the formulation to prevent the degradation of rCTX-B by gastric acid. This vaccine can be administered to individuals over 2 years of age and requires at least two doses for optimal efficacy. The protective efficacy (PE) of this vaccine has been demonstrated in several field trials in different countries, achieving over 80% protection in the first 6 months and gradually decreasing thereafter, ultimately providing negligible protection after 2 years. No significant severe adverse effects were attributed to this vaccine (220–222). Further analyses of volunteers vaccinated with Dukoral® revealed that this formulation induces high levels of specific sIgA antibodies and IFN-γ production in the duodenal mucosa (223). Notably, Dukoral® also provides significant protection for 3–9 months (PE: 67%) against diarrhea caused by enterotoxigenic E. coli (ETEC) producing heat-labile toxin (LT). This cross-protection is due to the cross-reactivity between the CTX-B subunit and LT (224).
In the late 1980s, the technology for manufacturing Dukoral® was transferred from Sweden to Vietnam for local production. This vaccine contained the same Dukoral strain composition, but the rCTX-B subunit was removed to simplify manufacturing, reduce costs, and improve stability. In 1992, the O139 epidemic in India and Bangladesh led to the addition of a killed O139 strain. This formulation was initially licensed in Vietnam as ORC-Vax™ and later after its modification as mORC-Vax™. It should be noted that the incorporation of the O139 component did not affect the responses to the original Dukoral components; instead, anti-O1 and anti-O139 antibodies were induced in serum and the intestinal mucosa (225, 226). However, the National Regulatory Agency (NRA) of Vietnam at that time did not have WHO approval, which limited the international use and WHO prequalification of this Vietnamese OCV. To make the vaccine available for international use, the manufacturing technology was transferred from Vietnam to Shantha Biotechnics in India, which had a WHO-approved NRA (195, 227). The PE of this vaccine was evaluated in a trial conducted in Kolkata, demonstrating that a two-dose immunization schedule provides an overall 65% protection over a five-year observation period. In 2009, this vaccine was licensed in India as Shanchol™, and WHO prequalified it in 2011 (228, 229).
The manufacturing technology of Shanchol™ was later transferred to Eubiologics in Seoul, Republic of Korea, resulting in the production of Euvichol®, which has an identical composition to Shanchol™. Studies in different countries have shown that Euvichol® and Shanchol™ elicit similar vibriocidal antibody responses and have comparable safety profiles. Euvichol® received licensure and WHO prequalification in 2015. Euvichol-Plus® is an improved vaccine that utilizes plastic tubes instead of conventional glass vials, providing better conditions for storage, transportation, and administration. This change has facilitated the delivery of this vaccine in emergency situations or humanitarian campaigns. Euvichol-Plus® received WHO prequalification in 2017 (230).
Two killed OCVs are licensed in some countries but are not WHO-prequalified. OraVacs™ is a dry formulation enteric-coated capsule vaccine containing a composition similar to Dukoral®. It is licensed in China and the Philippines (231). Cholvax™ is licensed in Bangladesh for use in the national cholera control program and has demonstrated safety and immunogenicity comparable to Shanchol™ (232).
The OCVs have achieved an important milestone in public health by providing herd immunity in vaccinated communities, thereby reducing person-to-person transmission (218, 219). In addition, the accumulation of evidence regarding the safety and efficacy of these vaccines has led the WHO to recommend their mass use as a preventive strategy in cholera-endemic areas, as well as a response measure during cholera outbreaks. Consequently, the WHO established the global OCV stockpile in 2011, which received support from the Global Alliance for Vaccines and Immunizations (Gavi Alliance) in 2014 (233). The main objectives of the OCV stockpile are to store and provide cholera vaccines during outbreaks and humanitarian campaigns, among other measures to control this disease. Presently, the OCV stockpile primarily uses Euvichol-Plus as its main formulation.
Despite their importance and usefulness, killed OCVs have several limitations. First, the PE of these vaccines is low (~42%) in children under the age of five, who are most vulnerable to the long-term effects and higher mortality associated with cholera (228). Second, they require multiple doses to achieve a high level of protection, which increases economic costs and the time required to elicit immunity. In fact, with a single dose, PE is only 8% for those under the age of five and 57.5% for those over the age of five (234, 235). Third, PE is short-term since it begins to decrease after 6 months and practically disappears after 3 or 5 years. In some sense, these limitations may be intrinsically related to the nature of killed vaccines. For instance, the in vitro cultures used to grow the vibrios included in these formulations do not reproduce host conditions and some important antigens may not be expressed. This is the case of the TcpA antigen, which is absent in the killed OCVs (236). Moreover, the formalin and heat treatment used to kill the bacteria may destroy or alter epitopes (237). Ultimately, killed vaccines are unable to mimic natural infection, so immune stimulation may be different from what is needed to achieve long-lasting protection.
Live attenuated OCVs have the potential to overcome many of the intrinsic limitations of killed OCVs. For instance, live attenuated vibrios closely mimic natural infection, and thus, they may trigger immune responses in the GALT, with the potential to target antigens which are only expressed in vivo during infection (199). Moreover, live attenuated OCVs may require a single dose, reducing the time required to achieve significant PE; this is particularly advantageous for individuals requiring travel at short notice to areas where an outbreak is occurring or where cholera is endemic (238).
Currently, only one live attenuated OCV is available, named Vaxchora™, which is approved in the United States and Europe for travelers visiting regions where cholera is endemic (238). The approval of Vaxchora™ in other markets is pending. This vaccine is based on the V. cholerae strain CVD 103-HgR, serogroup O1, serotype Inaba, classical biotype, which is derived from the strain 569B. The CVD 103-HgR strain is genetically modified and contains a deletion of the ctxA gene and an insertion of the Hg++ resistance gene to enable differentiation of the vaccine strain from the wild type (239). Although the CVD 103-HgR strain cannot produce active CTX, it can synthesize the CTX-B subunit and the TcpA antigen, and colonize the small intestine transiently (199). The initial CVD 103-HgR formulation was introduced in 1993, and since then, it has been manufactured by various companies and known by other trade names such as Orochol®, Mutacol®, and Orochol-E®.
The effectiveness of the CVD 103-HgR vaccine was initially evaluated in four experimental challenge studies between 1987 and 1999, where the PE against severe diarrhea was 92.7, 95.4, 79.0, and 67.6% (239). Moreover, this vaccine can elicit a significant VAT 10 days after immunization, but the duration of protection has not been fully determined (240, 241). However, conflicting results were obtained in two field studies conducted in North Jakarta, Indonesia, between 1993 and 1997 (242), and on the island of Pohnpei, Micronesia, during an outbreak in 2001 (243). The PE obtained in the Indonesian study was only 14%, whereas in the Micronesian study, it was 79.2%. The poor performance of this vaccine in the Indonesian study was attributed to a lower-than-expected cholera incidence (242). Thus, the effectiveness of Vaxchora™ in cholera-endemic areas remains unclear.
Additionally, several factors have limited the use of the CVD 103-HgR vaccine beyond the traveler’s market, including possible toxigenic reversal, high cost, and the requirement of a cold chain (−25 to −15°C) (244). For further details beyond what is provided here on the CVD 103-HgR vaccine and the history of its development, the reader is referred to recent comprehensive reviews (238, 239, 244).
8. Vaccines candidates in clinical development
Much work has been done in recent years to improve the manufacturing process of killed OVCs, to enhance their stability, and to further reduce costs. An example of this is Hillchol® (245), which was developed by Bharat Biotech International in India. Hillchol® is based on the formalin-killed V. cholerae O1 Hikojima strain MS1568 (Table 4). The MS1568 strain is a derivative of Phil 6973 strain, which is a component of Shanchol™. It has a partially inactivating mutation in the wbeT gene that is responsible for LPS methylation, which differentiates the Ogawa and Inaba serotypes; thus, this strain expresses ~50% of both LPS. As a result, Hillchol® requires a single-strain manufacturing process that is less expensive than other killed OCVs but still maintains a mixed O1 antigen composition (36). Hillchol® completed a phase I/II study evaluating its safety, tolerability and immunogenicity. The study demonstrated that it is not inferior to Shanchol™ in individuals of different age groups residing in a cholera-endemic region (246). In August 2022, Hillchol® began a phase III study (Clinical Trial NCT 05507229).
Over the past three decades, several live attenuated OCV candidates have been developed. However, only four of them have progressed to clinical trials. The oldest among them, CholeraGarde® (Peru-15), was reported in 1995. It is based on a V. cholerae O1 El Tor Inaba strain derived from the C6709 strain, which was isolated in Peru in 1991. The Peru-15 strain is attenuated due to a deletion of the CTXΦ prophage and a spontaneous mutation that affects motility. It also has an insertion of the ctxB gene in the recA gene for the constitutive expression of the CTX-B subunit. Since the recA gene is required for homologous recombination, the Peru-15 strain has a reduced capacity for horizontal gene transfer (247, 248). CholeraGarde® was shown to be safe and immunogenic in phase I/II studies conducted in the United States, Bangladesh and Thailand (249–252). In challenge studies, a single dose of this formulation demonstrated a PE of 100% against moderate and severe diarrhea. Additionally, only a small percentage of individuals (7%) developed mild diarrhea after challenge (253). The last clinical trial of this vaccine candidate was reported in 2015, and it is unclear whether it will be evaluated in a phase III study.
Another live attenuated OCV candidate, Vax-COLER® (Cuban 638), was reported in 1999. It is based on the V. cholerae El Tor Ogawa strain 638, which is derived from the C7258 strain isolated in Peru in 1991. The 638 strain is attenuated due to the deletion of the CTXΦ prophage and an insertion of the Clostridium thermocellum endoglucanase A (celA) gene into the hemagglutinin/protease (hapA) gene (254). Vax-COLER® has been shown to be safe and immunogenic in phase I/II studies conducted in Cuba and in a cholera endemic area in Maputo, Mozambique (254–256). It has also been found to provide protection against a challenge with the V. cholerae O1 El Tor strain 3,008 (257). However, there is currently no available information on whether this vaccine candidate will be evaluated in a phase III study.
A third live attenuated OCV candidate is VA1.4, which was initially reported in 1999 as VA1.3 (258). The VA1.3 is a non-toxigenic V. cholerae O1 El Tor Inaba strain with an insertion of the ctxB gene (under the control of the ctx promoter) into the hlyA gene. This strain naturally lacked the CTXΦ prophage and has proven to be non-reactogenic in a rabbit ileal loop assay. In 2009, a phase I/II study conducted in a cholera-endemic area in Kolkata, India, showed that the VA1.3 strain is safe and immunogenic (259). A later version of this vaccine candidate is the VA1.4 strain, which is identical to VA1.3, except for the absence of an ampicillin resistance gene. In 2014, a phase I/II study conducted in Kolkata, India, showed that VA1.4 is also safe and immunogenic (260). This vaccine candidate was evaluated with a two-dose schedule of 1.9 × 109 CFU, but no additional benefit was observed after the second dose. Currently, there is no information available regarding a phase III study for this formulation.
The fourth and most recent live attenuated OCV candidate is PanChol (HaitiV), which was developed in 2018 in the USA (261). HaitiV is derived from a variant O1 El Tor Ogawa strain isolated during the 2010 Haiti outbreak. The HaitiV strain has several genetic modifications that make it avirulent and resistant to reversion, but it maintains the ability to colonize the intestine and induce immune responses. These genetic modifications include deletions of: (i) the entire CTXΦ and its boundaries encoding the MARTX toxin (rtxABCDE) genes; (ii) the hupB gene required for episomal maintenance of CTXΦ; (iii) five flagellin subunits (flaA-E) genes; (iv) a region of DNA containing resistance genes for the antibiotics trimethoprim (dfrA), sulfamethoxazole (sul2), streptomycin (strAB), and chloramphenicol (floR); and (v) the recA gene involved in gene acquisition by homologous recombination. In addition, HaitiV has an insertion of the ctxB gene (under the control of the htpG promoter) in the neutral locus N900_11550. To prevent toxigenic reversion, the HaitiV strain also encodes a CRISPR/Cas9 system targeting the ctxA gene.
It should be noted that oral administration of HaitiV in animal models has demonstrated a protective effect within 24 h post-vaccination against a lethal dose of the parent V. cholerae strain HaitiWT (261, 262). This rapid protection was achieved before the induction of any adaptive immune response, suggesting that HaitiV exhibits a probiotic-like activity. However, it is unclear whether this “probiotic” effect is specific to HaitiV or also present in other live attenuated OVCs. Moreover, immunization of mice with this vaccine candidate was well-tolerated and immunogenic, triggering humoral responses consisting of anti-OSP and anti-CTX-B IgM, IgG, and IgA antibodies. In December 2022, PanChol began a phase I study for safety, tolerability, and immunogenicity in healthy volunteers (Clinical Trial NCT05657782).
As previously mentioned, protection against cholera is mainly serogroup-specific. Furthermore, the generation of anti-OSP antibodies is a common immune response elicited by various cholera vaccines, and these antibodies have been associated with protection in both animal models and in humans. This has been the rationale for the use of LPS and the O-antigen as a target for the development of cholera vaccines. In this regard, vaccine candidates based on the O-antigen conjugated with protein carriers are an interesting alternative to OCVs.
One of the first cholera conjugate vaccine candidates was prepared by binding the detoxified (deacylated) LPS (DeA-LPS) with the CTX (263). Subsequent evaluation of the DeA-LPS-CTX conjugate in a phase I study in adult volunteers showed that it was immunogenic by eliciting vibriocidal (anti-LPS) antibodies and IgG anti-CTX antibodies (264). However, this vaccine candidate was not further evaluated.
More recently, cholera conjugate vaccine candidates were developed by binding the Inaba or Ogawa OSP with the recombinant tetanus toxoid heavy chain fragment (rTThc). Preclinical evaluation of the OSP: rTTHc conjugates has shown that they are immunogenic and protective in mice (265, 266). Interestingly, a combined vaccination approach which includes an oral priming with Vaxchora™ followed by a parenteral boost with the OSP: rTTHc conjugate resulted in increased immune responses in mice (267). In 2021, the OSP: rTTHc conjugate candidate was produced in a scalable manner, and the addition of aluminum phosphate adjuvant increased the OSP-specific immune responses in mice (268). In September 2022, the OSP: rTTHc conjugate vaccine began a phase I study primarily to determine the safety of the dose range with or without aluminum phosphate adjuvant, and secondarily to assess humoral immune responses in the nonendemic population, which will guide the selection of future doses (Clinical Trial NCT 05559983).
Plant-based vaccines represent a step toward new vaccinology technologies and oral vaccination. These innovative vaccines have some advantages over classical vaccines, including the long-term preservation of antigenic proteins without the need for a cold chain, resistance to digestion in the stomach, lower cost, increased safety, and scalability (269). Potato, tomato, and rice are attractive antigen-expressing plants that have been used as a platform for the development of candidate vaccines against some infectious diseases in animals and humans (269–271). Notably, the expression of CTX-B subunit oligomers has been reported in transgenic potato (272), tomato (273, 274), and rice plants (275, 276). Additionally, the TcpA antigen has also been expressed in transgenic tomato plants (277). However, transgenic rice expressing the CTX-B subunit has been by far the most studied.
In 2007, MucoRice-CTB, a transgenic rice-based vaccine expressing the CTX-B subunit, was developed. This platform produced an average of 30 μg of recombinant CTX-B per transgenic rice seed, which was stored in protein bodies (PBs), a type of storage organelle in rice. The in vitro assays with pepsin showed that the CTX-B was not degraded, suggesting that the PBs may act as a natural capsule for oral administration of the vaccine. Preclinical studies in mice and pigs orally immunized with the seed powder showed that MucoRice-CTB induced CTX-B-specific serum IgG and intestinal sIgA antibodies (275, 278–281). In the intestinal loop assay, the sIgA antibodies that were generated were found to confer protection against V. cholerae and LT-ETEC challenges (278). However, this formulation was not evaluated in an animal challenge assay to test whether it conferred protection against colonization by V. cholerae. This is probably because CTX-B-specific antibodies do not have vibriocidal activity. As a step toward the use of MucoRice-CTB in humans, this vaccine candidate was evaluated in non-human primates (Macaca fascicularis), inducing CTX-B-specific antibodies without adverse effects (279). Recently, a phase I study conducted in Japan showed that MucoRice-CTB increased CTB-specific serum IgG and IgA antibody levels without inducing serious adverse events. A similar phase 1 study is planned with individuals of other ethnicities (282).
9. Vaccine candidates in preclinical development
Some live OCVs were developed and evaluated in animal models several years ago, including IEM108 (283, 284), TLP01 (285), and VCUSM2 (286). However, no further related studies have been published since then. Although mentioned for historical reasons, interested readers are recommended to refer to earlier reviews where these vaccine candidates have already been discussed (287, 288).
Recent technological advances in vaccine design and manufacture have led to promising cholerae vaccine candidates, such as DuoChol™. This killed OCV is a lyophilized mixture of formalin-killed isogenic El Tor Ogawa and Inaba strains and rCTB in an enterocoated capsule. This formulation improves thermostability and could facilitate its integration into standard immunization programs in cholera-endemic areas. DuoChol™ is currently in preclinical development at the University of Gothenburg, Sweden (215, 227).
OMVs have emerged as a promising strategy for developing vaccines against Gram-negative bacterial pathogens, including V. cholerae. V. cholerae OMVs contain important virulence factors such as CTX, TcpA, OmpU, NanH, LPS, and others (70–72, 289, 290). Several preparations of OMVs derived from WT or mutant V. cholerae strains have been administered to mice through different routes, resulting in strong humoral responses against a variety of OMV-associated antigens. Immunization with OMVs protects against V. cholerae colonization regardless of the route of administration (291). In particular, intranasal immunization with OMVs induces O-specific antibodies, particularly IgG, which inhibit V. cholerae motility (292, 293). In another study, Leitner et al. (290) developed a combined formulation of OMVs derived from V. cholerae and ETEC. Interestingly, this OMV mixture conferred protection in mice against both pathogens, suggesting the potential for developing a broadly protective OMV-based vaccine against several Gram-negative pathogens by combining OMVs.
Virus-like particles (VLPs) are multi-protein structures that mimic the organization and conformation of native viruses, but lack the viral genome, making them a safe template for vaccine development (294). Over the past three decades, VLPs have served as a successful platform for developing vaccines against various viral diseases (295). However, their potential use against non-viral pathogens has scarcely been explored. A recent study reported the coupling of VLPs from the bacteriophage Qβ to the V. cholerae OSP antigen, which was immunogenic in mice, eliciting IgG antibodies with vibriocidal activity (296).
The development of chimeric proteins is a growing trend in the design of next-generation vaccines. The biotechnological revolution, particularly the improvements in gene synthesis, has opened new doors for the rational design of protein-based vaccines (297). Chimeric proteins carrying selected epitopes from several strains or different pathogens can enhance the immunogenicity of the recombinant antigen, eliciting a broader immune response (298). Chimeric protein-based vaccines against cholera have focused on known antigenic proteins, including the CTX-A and CTX-B subunits, flagellins, OmpW, OmpU, TcpA, TcpF, and NanH. Two vaccine candidates, TcpF-CTA2-CT-B and TcpA-CTA2-CT-B, are chimeric proteins (299, 300). Both chimeras were immunogenic in mice and triggered specific antibodies that conferred protection in passively immunized infant mice. However, no further studies have been published regarding these vaccine candidates. Similar results were obtained by the OTC chimera (OmpW, TcpA, and CTX-B), which elicited specific IgG antibodies that were protective in the ileal loop assay and in passively immunized infant mice (301).
An interesting recent study describes the polyvalent cholera MEFA protein, which contains antigenic domains of TcpA, CTX, NanH, HlyA, flagellins, and peptides mimicking the OSP on a flagellin B backbone (302). Mice and rabbits immunized intramuscularly with the MEFA protein developed antibodies to all the virulence factors targeted by the immunogen, except LPS. The antibodies generated neutralized CTX, bacterial motility, and in vitro adherence of V. cholerae O1, O139, and non-O1/non-O139 strains. Moreover, this vaccine provided cross-protective against V. cholerae O1, O139, and non-O1/non-O139 strains in adult and infant rabbit colonization models.
Despite promising results, protein-based vaccines have several limitations. For instance, they are often poorly immunogenic and require multiple doses and adjuvants to achieve protective immunity. In addition, they are generally administered parenterally to avoid enzymatic degradation in the stomach, inducing strong humoral responses at the systemic level but not at the intestinal mucosa level. Although this type of vaccine represents a potential alternative to OCVs, none of them have been tested in human trials. More importantly, they must compete in a market that demands cholera vaccines that are cost-effective and administered in a single-dose regimen.
10. Concluding remarks and prospects
Over the last few decades, much knowledge has been gained about the pathogenesis and immune response of V. cholerae infection, which has resulted in the development of treatments and vaccines. However, progress toward a highly effective cholera vaccine has been hindered by several limitations. These include the lack of a well-defined correlate of long-term protective immunity as well as an animal model that fully recapitulates the disease (303). In addition, it is largely unknown how the microbiota confers resistance or susceptibility to cholera and how it affects the immune response generated by vaccines against this disease (125). In this respect, human microbiota-associated mice could be a valuable animal model to consider (30).
Further studies are needed to investigate how immune responses are produced during V. cholerae infection. In particular, it is important to understand the innate immune pathways that are modulated during the natural course of infection, and whether these responses are beneficial or detrimental to the host. Additionally, it is crucial to clarify how long-term immune memory is generated in patients recovering from cholera. This information is essential because a highly effective cholera vaccine must recapitulate or mimic these immune responses.
OCVs have been shown to be safe, and although they confer short-term protection, their usefulness in cholera control has been reliably demonstrated. It is likely that new oral adjuvants, such as nanocarriers (304), lipid-based adjuvants (305), among other (306–308), could increase the efficacy of these vaccines.
The OMV-based vaccines, plant-based vaccines, and chimeric antigens are emerging and promising approaches in vaccine development. Moreover, mRNA vaccines against SARS-CoV-2 have been rapidly developed and have proven to be highly efficacious and adaptable as required. Recent studies have demonstrated the potential of mRNA vaccines against bacterial pathogens (309–311). Therefore, new cholera vaccine candidates based on these platforms are expected to appear in the coming years.
Another strategy to improve cholera vaccines could be the development of multivalent vaccines that protect against various enteric pathogens. Finally, in the human-pathogen arms race, the development of new vaccine technologies is likely the key factor in winning the battle and, ideally, in finding a highly long-lasting protective cholera vaccine.
Author contributions
DM and RV wrote the draft of the manuscript. MO’R edited the manuscript. All authors contributed to data acquisition and analysis and approved the publication of the content.
Funding
This work was supported by Postdoctoral FONDECYT grant 3190524, awarded to DM. Regular FONDECYT grant 1211647, awarded to RV.
Acknowledgments
In memory of Carmenza Forero (1955 – 2020), beloved mother and best teacher. We thank Helen Lowry for the careful revision and edition of the manuscript.
Conflict of interest
The authors declare that the research was conducted in the absence of any commercial or financial relationships that could be construed as a potential conflict of interest.
Publisher’s note
All claims expressed in this article are solely those of the authors and do not necessarily represent those of their affiliated organizations, or those of the publisher, the editors and the reviewers. Any product that may be evaluated in this article, or claim that may be made by its manufacturer, is not guaranteed or endorsed by the publisher.
References
1. Sit, B , Fakoya, B , and Waldor, MK . Emerging concepts in cholera vaccine design. Annu Rev Microbiol. (2022) 76:681–702. doi: 10.1146/annurev-micro-041320-033201
2. Williams, PCM , and Berkley, JA . Guidelines for the management of paediatric cholera infection: a systematic review of the evidence. Paediatr Int Child Health. (2018) 38:S16–31. doi: 10.1080/20469047.2017.1409452
3. Ganesan, D , Sen, GS , and Legros, D . Cholera surveillance and estimation of burden of cholera. Vaccine. (2020) 38:A13–7. doi: 10.1016/j.vaccine.2019.07.036
4. Domman, D , Chowdhury, F , Khan, AI , Dorman, MJ , Mutreja, A , Uddin, MI, et al. Defining endemic cholera at three levels of spatiotemporal resolution within Bangladesh. Nat Genet. (2018) 50:951–5. doi: 10.1038/s41588-018-0150-8
5. Ingelbeen, B , Hendrickx, D , Miwanda, B , van der Sande, MAB , Mossoko, M , Vochten, H, et al. Recurrent cholera outbreaks, democratic republic of the Congo, 2008–2017. Emerg Infect Dis. (2019) 25:856–64. doi: 10.3201/eid2505.181141
6. Zheng, Q , Luquero, FJ , Ciglenecki, I , Wamala, JF , Abubakar, A , Welo, P, et al. Cholera outbreaks in sub-Saharan Africa during 2010-2019: a descriptive analysis. Int J Infect Dis. (2022) 122:215–21. doi: 10.1016/j.ijid.2022.05.039
7. Muzembo, BA , Kitahara, K , Debnath, A , Ohno, A , Okamoto, K , and Miyoshi, SI . Cholera outbreaks in India, 2011–2020: a systematic review. Int J Environ Res Public Health. (2022) 19:2011–20. doi: 10.3390/ijerph19095738
8. Charnley, GEC , Kelman, I , and Murray, KA . Drought-related cholera outbreaks in Africa and the implications for climate change: a narrative review. Pathog Glob Health. (2022) 116:3–12. doi: 10.1080/20477724.2021.1981716
9. Ali, M , Nelson, AR , Lopez, AL , and Sack, DA . Updated global burden of cholera in endemic countries. PLoS Negl Trop Dis. (2015) 9:e0003832. doi: 10.1371/journal.pntd.0003832
10. Legros, D . Global cholera epidemiology: opportunities to reduce the burden of cholera by 2030. J Infect Dis. (2018) 218:S137–40. doi: 10.1093/infdis/jiy486
11. Thakur, S , and Chauhan, V . State of the globe: revisiting cholera – the larger ongoing pandemic. J Glob Infect Dis. (2021) 13:113. doi: 10.4103/jgid.jgid_234_21
12. Musa, SS , Gyeltshen, D , Manirambona, E , Wada, YH , Sani, AF , Ullah, I, et al. Dual tension as Nigeria battles cholera during the COVID-19 pandemic. Clin Epidemiol Glob Heal. (2021) 12:100913. doi: 10.1016/j.cegh.2021.100913
13. World Health Organization . Cholera, 2020. Wkly Epidemiol Rec. (2020) 96:445–54. Available at: https://apps.who.int/iris/bitstream/handle/10665/345271/WER9637-445-454-eng-fre.pdf?sequence=1&isAllowed=y
14. World Health Organization . Wkly Epidemiol Rec. (2022) 97:Cholera, 2021:453–64. Available at: https://apps.who.int/iris/handle/10665/362858
15. Amani, A , Fouda, AAB , Nangmo, AJ , Bama, SN , Tatang, CA , Mbang, MA, et al. Reactive mass vaccination campaign against cholera in the covid-19 context in Cameroon: challenges, best practices and lessons learned. Pan Afr Med J. (2021) 38:392. doi: 10.11604/pamj.2021.38.392.27754
16. Taylor, DL , Kahawita, TM , Cairncross, S , and Ensink, JHJ . The impact of water, sanitation and hygiene interventions to control cholera: a systematic review. PLoS One. (2015) 10:e0135676. doi: 10.1371/journal.pone.0135676
17. Ramamurthy, T , Das, B , Chakraborty, S , Mukhopadhyay, AK , and Sack, DA . Diagnostic techniques for rapid detection of Vibrio cholerae O1/O139. Vaccine. (2020) 38:A73–82. doi: 10.1016/j.vaccine.2019.07.099
18. Abramo, JM , Reynolds, A , Crisp, GT , Weurlander, M , Söderberg, M , Scheja, M, et al. Individuality in music performance. Assess Eval High Educ. (2012) 37:435. doi: 10.1007/82
19. Farmer, P , Almazor, CP , Bahnsen, ET , Barry, D , Bazile, J , Bloom, BR, et al. Meeting Cholera’s challenge to Haiti and the world: a joint statement on cholera prevention and care. PLoS Negl Trop Dis. (2011) 5:e1145. doi: 10.1371/journal.pntd.0001145
20. Das, M , Singh, H , Girish Kumar, CP , John, D , Panda, S , and Mehendale, SM . Non-vaccine strategies for cholera prevention and control: India’s preparedness for the global roadmap. Vaccine. (2020) 38:A167–74. doi: 10.1016/j.vaccine.2019.08.010
21. Chatterjee, P , Kanungo, S , Bhattacharya, SK , and Dutta, S . Mapping cholera outbreaks and antibiotic resistant Vibrio cholerae in India: an assessment of existing data and a scoping review of the literature. Vaccine. (2020) 38:A93–A104. doi: 10.1016/j.vaccine.2019.12.003
22. World Health Organization . Ending cholera: a global roadmap to 2030. (2017). Available at: https://www.gtfcc.org/wp-content/uploads/2019/10/gtfcc-ending-cholera-a-global-roadmap-to-2030.pdf (accessed July 12, 2022)
23. Michel, E , Gaudart, J , Beaulieu, S , Bulit, G , Piarroux, M , Boncy, J, et al. Estimating effectiveness of case-area targeted response interventions against cholera in Haiti. Elife. (2019) 8:1–34. doi: 10.7554/eLife.50243
24. Reveiz, L , Chapman, E , Ramon-Pardo, P , Koehlmoos, TP , Cuervo, LG , Aldighieri, S, et al. Chemoprophylaxis in contacts of patients with cholera: systematic review and meta-analysis. PLoS One. (2011) 6:6–11. doi: 10.1371/journal.pone.0027060
25. Gabutti, G , Rossanese, A , Tomasi, A , Giuffrida, S , Nicosia, V , Barriga, J, et al. Cholera the current status of cholera vaccines and recommendations for travellers. Vaccine. (2020) 8:1–17. doi: 10.3390/vaccines8040606
26. Bornside, GH . Jaime Ferran and preventive inoculation against cholera. Bull Hist Med. (1981) 55:516–32.
27. Bornside, GH . Waldemar Haffkine’s cholera vaccines and the Ferran-Haffkine priority dispute. J Hist Med Allied Sci. (1982) 37:399–422. doi: 10.1093/jhmas/XXXVII.4.399
28. Sack, DA , Sack, RB , Nair, GB , and Siddique, A . Cholera (the lancet). Lancet. (2004) 363:223–33. doi: 10.1016/S0140-6736(03)15328-7
29. Levine, MM . Developing countries: lessons from a live cholera vaccine. BMC Biol. (2010) 8:129. doi: 10.1186/1741-7007-8-129
30. Macbeth, JC , Liu, R , Alavi, S , and Hsiao, A . A dysbiotic gut microbiome suppresses antibody mediated-protection against Vibrio cholerae. iScience. (2021) 24:103443. doi: 10.1016/j.isci.2021.103443
31. Dutta, D , Chowdhury, G , Pazhani, GP , Guin, S , Dutta, S , Ghosh, S, et al. Vibrio cholerae non-O1, non-O139 serogroups and cholera-like diarrhea, Kolkata. India Emerg Infect Dis. (2013) 19:464–7. doi: 10.3201/eid1903.121156
32. Arteaga, M , Velasco, J , Rodriguez, S , Vidal, M , Arellano, C , Silva, F, et al. Genomic characterization of the non-O1/non-O139 Vibrio cholerae strain that caused a gastroenteritis outbreak in Santiago, Chile, 2018. Microb Genomics. (2020) 6:e000340. doi: 10.1099/mgen.0.000340
33. Octavia, S , Salim, A , Kurniawan, J , Lam, C , Leung, Q , Ahsan, S, et al. Population structure and evolution of non-O1/non-O139 Vibrio cholerae by multilocus sequence typing. PLoS One. (2013) 8:e65342. doi: 10.1371/journal.pone.0065342
34. Chen, Y , Bystricky, P , Adeyeye, J , Panigrahi, P , Ali, A , Johnson, JA, et al. The capsule polysaccharide structure and biogenesis for non-O1 Vibrio cholerae NRT36S: genes are embedded in the LPS region. BMC Microbiol. (2007) 7:1–15. doi: 10.1186/1471-2180-7-20
35. Stroeher, UH , Karageorgos, LE , Morona, R , and Manning, PA . Serotype conversion in Vibrio cholerae O1. Proc Natl Acad Sci U S A. (1992) 89:2566–70. doi: 10.1073/pnas.89.7.2566
36. Karlsson, SL , Ax, E , Nygren, E , Källgård, S , Blomquist, M , Ekman, A, et al. Development of stable Vibrio cholerae O1 Hikojima type vaccine strains co-expressing the Inaba and Ogawa lipopolysaccharide antigens. PLoS One. (2014) 9:e108521. doi: 10.1371/journal.pone.0108521
37. Mohammadi Barzelighi, H , Bakhshi, B , and Boustanshenas, M . Genetic determinants differences between Vibrio cholerae biotypes. Infect Epidemiol Med. (2016) 2:26–30. doi: 10.18869/modares.iem.2.2.26
38. Clemens, JD , Nair, GB , Ahmed, T , Qadri, F , and Holmgren, J . Cholera. Lancet. (2017) 390:1539–49. doi: 10.1016/S0140-6736(17)30559-7
39. Nair, GB , Qadri, F , Holmgren, J , Svennerholm, AM , Safa, A , Bhuiyan, NA, et al. Cholera due to altered El Tor strains of Vibrio cholerae O1 in Bangladesh. J Clin Microbiol. (2006) 44:4211–3. doi: 10.1128/JCM.01304-06
40. Devault, AM , Golding, GB , Waglechner, N , Enk, JM , Kuch, M , Tien, JH, et al. Second-pandemic strain of Vibrio cholerae from the Philadelphia cholera outbreak of 1849. N Engl J Med. (2014) 370:334–40. doi: 10.1056/NEJMoa1308663
41. Chowdhury, F , Ross, AG , Islam, MT , McMillan, NAJ , and Qadri, F . Diagnosis, management, and future control of cholera. Clin Microbiol Rev. (2022) 35:e0021121. doi: 10.1128/cmr.00211-21
42. Samadi, AR , Shahid, N , Eusof, A , Yunus, M , Huq, MI , Khan, MU, et al. Classical Vibrio cholerae biotype displaces EL tor in Bangladesh. Lancet. (1983) 321:805–7. doi: 10.1016/S0140-6736(83)91860-3
43. Mutreja, A , Kim, DW , Thomson, NR , Connor, TR , Lee, JH , Kariuki, S, et al. Evidence for several waves of global transmission in the seventh cholera pandemic. Nature. (2011) 477:462–5. doi: 10.1038/nature10392
44. Weill, F , Domman, D , Njamkepo, E , Tarr, C , Rauzier, J , Fawal, N, et al. Genomic history of the seventh pandemic of cholera in Africa. Science. (2017) 358:785–9. doi: 10.1126/science.aad5901
45. Bhandari, M , Jennison, AV , Rathnayake, IU , and Huygens, F . Evolution, distribution and genetics of atypical Vibrio cholerae – a review. Infect Genet Evol. (2021) 89:104726. doi: 10.1016/j.meegid.2021.104726
46. Mukhopadhyay, AK , Takeda, Y , and Balakrish, NG . Cholera outbreaks in the El Tor biotype era and the impact of the new El Tor variants. Assess Eval High Educ. (2014) 379:17–47. doi: 10.1007/82_2014_363
47. Kim, EJ , Lee, CH , Nair, GB , and Kim, DW . Whole-genome sequence comparisons reveal the evolution of Vibrio cholerae O1. Trends Microbiol. (2015) 23:479–89. doi: 10.1016/j.tim.2015.03.010
48. Okada, K , Iida, T , Kita-Tsukamoto, K , and Honda, T . Vibrios commonly possess two chromosomes. J Bacteriol. (2005) 187:752–7. doi: 10.1128/JB.187.2.752-757.2005
49. Heidelberg, JF , Elsen, JA , Nelson, WC , Clayton, RA , Gwinn, ML , Dodson, RJ, et al. DNA sequence of both chromosomes of the cholera pathogen Vibrio cholerae. Nature. (2000) 406:477–83. doi: 10.1038/35020000
50. Kimsey, HH , and Waldor, MK . CTXφ immunity: application in the development of cholera vaccines. Proc Natl Acad Sci U S A. (1998) 95:7035–9. doi: 10.1073/pnas.95.12.7035
51. Hassan, F , Kamruzzaman, M , Mekalanos, JJ , and Faruque, SM . Satellite phage TLC φ enables toxigenic conversion by CTX phage through dif site alteration. Nature. (2010) 467:982–5. doi: 10.1038/nature09469
52. Rajanna, C , Wang, J , Zhang, D , Xu, Z , Ali, A , Hou, YM, et al. The vibrio pathogenicity Island of epidemic Vibrio cholerae forms precise extrachromosomal circular excision products. J Bacteriol. (2003) 185:6893–901. doi: 10.1128/JB.185.23.6893-6901.2003
53. Jermyn, WS , and Boyd, EF . Characterization of a novel vibrio pathogenicity island (VPI-2) encoding neuraminidase (nanH) among toxigenic Vibrio cholerae isolates. Microbiology. (2002) 148:3681–93. doi: 10.1099/00221287-148-11-3681
54. Davies, BW , Bogard, RW , Young, TS , and Mekalanos, JJ . Coordinated regulation of accessory genetic elements produces cyclic di-nucleotides for V. cholerae virulence. Cells. (2012) 149:358–70. doi: 10.1016/j.cell.2012.01.053
55. O’Shea, YA , Finnan, S , Reen, FJ , Morrissey, JP , O’Gara, F , and Boyd, EF . The Vibrio seventh pandemic island-II is a 26·9 kb genomic island present in Vibrio cholerae El Tor and O139 serogroup isolates that shows homology to a 43·4 kb genomic island in V. vulnificus. Microbiology. (2004) 150:4053–63. doi: 10.1099/mic.0.27172-0
56. Waldor, MK , Tschäpe, H , and Mekalanos, JJ . A new type of conjugative transposon encodes resistance to sulfamethoxazole, trimethoprim, and streptomycin in Vibrio cholerae O139. J Bacteriol. (1996) 178:4157–65. doi: 10.1128/jb.178.14.4157-4165.1996
57. Mazel, D , Dychinco, B , Webb, VA , and Davies, J . A distinctive class of integron in the Vibrio cholerae genome. Science. (1998) 280:605–8. doi: 10.1126/science.280.5363.605
58. Murphy, RA , and Fidelma, BE . Three Pathogenicity Islands of Vibrio cholerae can excise from the chromosome and form circular intermediates †. J Bacteriol. (2008) 190:636–47. doi: 10.1128/JB.00562-07
59. Pant, A , Bag, S , Saha, B , Verma, J , Kumar, P , Banerjee, S, et al. Molecular insights into the genome dynamics and interactions between core and acquired genomes of Vibrio cholerae. Proc Natl Acad Sci U S A. (2020) 117:23762–73. doi: 10.1073/pnas.2006283117
60. Faruque, SM , Chowdhury, N , Kamruzzaman, M , Dziejman, M , Rahman, MH , Sack, DA, et al. Genetic diversity and virulence potential of environmental Vibrio cholerae population in a cholera-endemic area. Proc Natl Acad Sci U S A. (2004) 101:2123–8. doi: 10.1073/pnas.0308485100
61. Faruque, SM , and Mekalanos, JJ . Pathogenicity islands and phages in Vibrio cholerae evolution. Trends Microbiol. (2003) 11:505–10. doi: 10.1016/j.tim.2003.09.003
62. Chun, J , Grim, CJ , Hasan, NA , Je, HL , Seon, YC , Haley, BJ, et al. Comparative genomics reveals mechanism for short-term and long-term clonal transitions in pandemic Vibrio cholerae. Proc Natl Acad Sci U S A. (2009) 106:15442–7. doi: 10.1073/pnas.0907787106
63. Karaolis, DKR , Lan, R , Kaper, JB , and Reeves, PR . Comparison of vibrio cholerae pathogenicity islands in sixth and seventh pandemic strains. Infect Immun. (2001) 69:1947–52. doi: 10.1128/IAI.69.3.1947
64. Boyd, EF , Heilpern, AJ , and Waldor, MK . Molecular analyses of a putative CTXφ precursor and evidence for independent acquisition of distinct CTXφs by toxigenic Vibrio cholerae. J Bacteriol. (2000) 182:5530–8. doi: 10.1128/jb.182.19.5530-5538.2000
65. Boyd, EF , Moyer, KE , Shi, LEI , Waldor, MK , and Al, BET . Infectious CTXPhi and the vibrio pathogenicity island prophage in Vibrio mimicus: evidence for recent horizontal transfer between V. mimicus and V. cholerae. Infect Immun. (2000) 68:1507–13. doi: 10.1128/IAI.68.3.1507-1513.2000
66. Banerjee, R , Das, B , Balakrish Nair, G , and Basak, S . Dynamics in genome evolution of Vibrio cholerae. Infect Genet Evol. (2014) 23:32–41. doi: 10.1016/j.meegid.2014.01.006
67. Kim, EJ , Lee, D , Moon, SH , Lee, CH , Kim, SJ , Lee, JH, et al. Molecular insights into the evolutionary pathway of Vibrio cholerae O1 atypical el tor variants. PLoS Pathog. (2014) 10:e1004384. doi: 10.1371/journal.ppat.1004384
68. Waldor, MK , and Mekalanos, JJ . Lysogenic conversion by a filamentous phage encoding. Science. (1996) 272:1910–4. doi: 10.1126/science.272.5270.1910
69. Korotkov, KV , Sandkvist, M , and Hol, WGJ . The type II secretion system: biogenesis, molecular architecture and mechanism. Nat Rev Microbiol. (2012) 10:336–51. doi: 10.1038/nrmicro2762
70. Rasti, ES , Schappert, ML , and Brown, AC . Association of Vibrio cholerae 569B outer membrane vesicles with host cells occurs in a GM1-independent manner. Cell Microbiol. (2018) 20:1–11. doi: 10.1111/cmi.12828
71. Zingl, FG , Thapa, HB , Scharf, M , Kohl, P , Müller, AM , and Schild, S . Outer membrane vesicles of Vibrio cholerae protect and deliver active cholera toxin to host cells via porin-dependent uptake. mBio. (2021) 12:e0053421. doi: 10.1128/mBio.00534-21
72. Rasti, ES , and Brown, AC . Cholera toxin encapsulated within several Vibrio cholerae O1 serotype inaba outer membrane vesicles lacks a functional B-subunit. Toxins. (2019) 11:1–15. doi: 10.3390/toxins11040207
73. Mathieu-Denoncourt, A , Giacomucci, S , and Duperthuy, M . “The Secretome of Vibrio cholerae,” in Infections and Sepsis Development. eds. V. Neri, L. Huang, and J. Li, IntechOpen.
74. Woida, PJ , and Satchell, KJF . The Vibrio cholerae MARTX toxin silences the inflammatory response to cytoskeletal damage before inducing actin cytoskeleton collapse. Sci Signal. (2020) 13:1–16. doi: 10.1126/scisignal.aaw9447
75. Satchell, KJF . Multifunctional-autoprocessing repeats-in-toxin (MARTX) toxins of vibrios. Microbiol Spectr. (2015) 3:VE-0002-2014. doi: 10.1128/microbiolspec.ve-0002-2014
76. Coelho, A , Andrade, JRC , Vicente, ACP , and Dirita, VJ . Cytotoxic cell vacuolating activity from Vibrio cholerae hemolysin. Infect Immun. (2000) 68:1700–5. doi: 10.1128/IAI.68.3.1700-1705.2000
77. Mitra, R , Figueroa, P , Mukhopadhyay, AK , Shimada, T , Takeda, Y , Berg, DE, et al. Cell vacuolation, a manifestation of the El Tor hemolysin of Vibrio cholerae. Infect Immun. (2000) 68:1928–33. doi: 10.1128/IAI.68.4.1928-1933.2000
78. Sikora, AE , Zielke, RA , Lawrence, DA , Andrews, PC , and Sandkvist, M . Proteomic analysis of the Vibrio cholerae type II secretome reveals new proteins, including three related serine proteases. J Biol Chem. (2011) 286:16555–66. doi: 10.1074/jbc.M110.211078
79. Elluri, S , Enow, C , Vdovikova, S , Rompikuntal, PK , Dongre, M , Carlsson, S, et al. Outer membrane vesicles mediate transport of biologically active Vibrio cholerae cytolysin (VCC) from V. cholerae strains. PLoS One. (2014) 9:e106731. doi: 10.1371/journal.pone.0106731
80. Alm, RA , Mayrhofer, G , Kotlarski, I , and Manning, PA . Amino-terminal domain of the El Tor haemolysin of Vibrio cholerae O1 is expressed in classical strains and is cytotoxic. Vaccine. (1991) 9:588–94. doi: 10.1016/0264-410X(91)90247-4
81. Levine, MM , Kaper, JB , Herrington, D , Losonsky, G , Morris, JG , Clements, ML, et al. Volunteer studies of deletion mutants of Vibrio cholerae O1 prepared by recombinant techniques. Infect Immun. (1988) 56:161–7. doi: 10.1128/iai.56.1.161-167.1988
82. Goldblum, SE , Rai, U , Tripathi, A , Thakar, M , De Leo, L , Di Toro, N, et al. The active zot domain (aa 288–293) increases ZO-1 and myosin 1C serine/threonine phosphorylation, alters interaction between ZO-1 and its binding partners, and induces tight junction disassembly through proteinase activated receptor 2 activation. FASEB J. (2011) 25:144–58. doi: 10.1096/fj.10-158972
83. Chatterjee, T , Mukherjee, D , Dey, S , Pal, A , Hoque, KM , and Chakrabarti, P . Accessory cholera enterotoxin, ace, from Vibrio cholerae: structure, unfolding, and virstatin binding. Biochemistry. (2011) 50:2962–72. doi: 10.1021/bi101673x
84. Attridge, SR , and Rowley, D . The role of the flagellum in the adherence of Vibrio cholerae. J Infect Dis. (1983) 147:864–72. doi: 10.1093/infdis/147.5.864
85. Richardson, K . Roles of motility and flagellar structure in pathogenicity of Vibrio cholerae: analysis of motility mutants in three animal models. Infect Immun. (1991) 59:2727–36. doi: 10.1128/iai.59.8.2727-2736.1991
86. Butler, SM , and Camilli, A . Both chemotaxis and net motility greatly influence the infectivity of Vibrio cholerae. Proc Natl Acad Sci U S A. (2004) 101:5018–23. doi: 10.1073/pnas.0308052101
87. Pennetzdorfer, N , Höfler, T , Wölflingseder, M , Tutz, S , Schild, S , and Reidl, J . σE controlled regulation of porin OmpU in Vibrio cholerae. Mol Microbiol. (2021) 115:1244–61. doi: 10.1111/mmi.14669
88. Sakharwade, SC , Sharma, PK , and Mukhopadhaya, A . Vibrio cholerae Porin OmpU induces pro-inflammatory responses, but down-regulates LPS-mediated effects in RAW 264.7, THP-1 and human PBMCs. PLoS One. (2013) 8:e76583. doi: 10.1371/journal.pone.0076583
89. Mathur, J , and Waldor, MK . The Vibrio cholerae ToxR-regulated porin OmpU confers resistance to antimicrobial peptides. Infect Immun. (2004) 72:3577–83. doi: 10.1128/IAI.72.6.3577-3583.2004
90. Ganie, HA , Choudhary, A , and Baranwal, S . Structure, regulation, and host interaction of outer membrane protein U (OmpU) of Vibrio species. Microb Pathog. (2022) 162:105267. doi: 10.1016/j.micpath.2021.105267
91. Finkelstein, RA , Boesman Finkelstein, M , and Holt, P . Vibrio cholerae hemagglutinin/lectin/protease hydrolyzes fibronectin and ovomucin: F.M. Burnet revisited. Proc Natl Acad Sci U S A. (1983) 80:1092–5. doi: 10.1073/pnas.80.4.1092
92. Wu, Z , Milton, D , Nybom, P , Sjö, A , and Magnusson, KE . Vibrio cholerae hemagglutinin/protease (HA/protease) causes morphological changes in cultured epithelial cells and perturbs their paracellular barrier function. Microb Pathog. (1996) 21:111–23. doi: 10.1006/mpat.1996.0047
93. Silva, AJ , Pham, K , and Benitez, JA . Haemagglutinin/protease expression and mucin gel penetration in El Tor biotype Vibrio cholerae. Microbiology. (2003) 149:1883–91. doi: 10.1099/mic.0.26086-0
94. Szabady, RL , Yanta, JH , Halladin, DK , Schofield, MJ , and Welch, RA . TagA is a secreted protease of Vibrio cholerae that specifically cleaves mucin glycoproteins. Microbiology. (2011) 157:516–25. doi: 10.1099/mic.0.044529-0
95. Millet, YA , Alvarez, D , Ringgaard, S , von Andrian, UH , Davis, BM , and Waldor, MK . Insights into Vibrio cholerae intestinal colonization from monitoring fluorescently labeled bacteria. PLoS Pathog. (2014) 10:e1004405. doi: 10.1371/journal.ppat.1004405
96. Galen, JE , Ketley, JM , Fasano, A , Richardson, SH , Wasserman, SS , and Kaper, JB . Role of Vibrio cholerae neuraminidase in the function of cholera toxin. Infect Immun. (1991) 60:406–15. doi: 10.1128/iai.60.2.406-415.1992
97. Moustafa, I , Connaris, H , Taylor, M , Zaitsev, V , Wilson, JC , Kiefel, MJ, et al. Sialic acid recognition by Vibrio cholerae neuraminidase. J Biol Chem. (2004) 279:40819–26. doi: 10.1074/jbc.M404965200
98. Almagro-Moreno, S , and Boyd, EF . Sialic acid catabolism confers a competitive advantage to pathogenic Vibrio cholerae in the mouse intestine. Infect Immun. (2009) 77:3807–16. doi: 10.1128/IAI.00279-09
99. Sikora, AE . Proteins secreted via the type II secretion system: smart strategies of Vibrio cholerae to maintain fitness in different ecological niches. PLoS Pathog. (2013) 9:e1003126. doi: 10.1371/journal.ppat.1003126
100. Kirn, TJ , Jude, BA , and Taylor, RK . A colonization factor links Vibrio cholerae environmental survival and human infection. Nature. (2005) 438:863–6. doi: 10.1038/nature04249
101. Wong, E , Vaaje-Kolstad, G , Ghosh, A , Hurtado-Guerrero, R , Konarev, PV , Ibrahim, AFM, et al. The Vibrio cholerae colonization factor GbpA possesses a modular structure that governs binding to different host surfaces. PLoS Pathog. (2012) 8:1–12. doi: 10.1371/journal.ppat.1002373
102. Syed, KA , Beyhan, S , Correa, N , Queen, J , Liu, J , Peng, F, et al. The Vibrio cholerae flagellar regulatory hierarchy controls expression of virulence factors. J Bacteriol. (2009) 191:6555–70. doi: 10.1128/JB.00949-09
103. Johnson, TL , Fong, JC , Rule, C , Rogers, A , Yildiz, FH , and Sandkvist, M . The type II secretion system delivers matrix proteins for biofilm formation by Vibrio cholerae. J Bacteriol. (2014) 196:4245–52. doi: 10.1128/JB.01944-14
104. MacIntyre, DL , Miyata, ST , Kitaoka, M , and Pukatzki, S . The Vibrio cholerae type VI secretion system displays antimicrobial properties. Proc Natl Acad Sci U S A. (2010) 107:19520–4. doi: 10.1073/pnas.1012931107
105. Zhao, W , Caro, F , Robins, W , and Mekalanos, JJ . Antagonism toward the intestinal microbiota and its effect on Vibrio cholerae virulence. Science. (2018) 359:210–3. doi: 10.1126/science.aap8775
106. Azman, AS , Rudolph, KE , Cummings, DAT , Lessler, J , and Tor, E . The incubation period of cholera: a systematic review supplement. J Infect. (2014) 66:432–8. doi: 10.1016/j.jinf.2012.11.013.The
107. Azman, AS , Rudolph, KE , Cummings, DAT , and Lessler, J . The incubation period of cholera: a systematic review. J Infect. (2013) 66:432–8. doi: 10.1016/j.jinf.2012.11.013
108. Scott Merrell, D , Bailey, C , Kaper, JB , and Camilli, A . The ToxR-mediated organic acid tolerance response of Vibrio cholerae requires OmpU. J Bacteriol. (2001) 183:2746–54. doi: 10.1128/JB.183.9.2746-2754.2001
109. Merrell, DS , Hava, DL , and Camilli, A . Identification of novel factors involved in colonization and acid tolerance of Vibrio cholerae. Mol Microbiol. (2002) 43:1471–91. doi: 10.1046/j.1365-2958.2002.02857.x
110. Merrell, DS , and Camilli, A . The cadA gene of Vibrio cholerae is induced during infection and plays a role in acid tolerance. Mol Microbiol. (1999) 34:836–49. doi: 10.1046/j.1365-2958.1999.01650.x
111. Sack, DA , Tacket, CO , Cohen, MB , Sack, RB , Losonsky, GA , Shimko, J, et al. Validation of a volunteer model of cholera with frozen bacteria as the challenge. Infect Immun. (1998) 66:1968–72. doi: 10.1128/iai.66.5.1968-1972.1998
112. Ramamurthy, T , Nandy, RK , Mukhopadhyay, AK , Dutta, S , Mutreja, A , Okamoto, K, et al. Virulence regulation and innate host response in the pathogenicity of Vibrio cholerae. Front Cell Infect Microbiol. (2020) 10:1–22. doi: 10.3389/fcimb.2020.572096
113. Childers, BM , and Klose, KE . Regulation of virulence in Vibrio cholerae: the ToxR regulon. Future Microbiol. (2007) 2:335–44. doi: 10.2217/17460913.2.3.335
114. Zingl, FG , Kohl, P , Cakar, F , Leitner, DR , Mitterer, F , Bonnington, KE, et al. Outer membrane vesiculation facilitates surface exchange and in vivo adaptation of Vibrio cholerae. Cell Host Microbe. (2020) 27:225–237.e8. doi: 10.1016/j.chom.2019.12.002
115. Simonet, VC , Baslé, A , Klose, KE , and Delcour, AH . The Vibrio cholerae porins OmpU and OmPT have distinct channel properties. J Biol Chem. (2003) 278:17539–45. doi: 10.1074/jbc.M301202200
116. Duret, G , and Delcour, AH . Deoxycholic acid blocks Vibrio cholerae OmpT but not OmpU porin. J Biol Chem. (2006) 281:19899–905. doi: 10.1074/jbc.M602426200
117. Almagro-Moreno, S , Pruss, K , and Taylor, RK . Intestinal colonization dynamics of Vibrio cholerae. PLoS Pathog. (2015) 11:1–11. doi: 10.1371/journal.ppat.1004787
118. Hung, DT , and Mekalanos, JJ . Bile acids induce cholera toxin expression in Vibrio cholerae in a ToxT-independent manner. Proc Natl Acad Sci U S A. (2005) 102:3028–33. doi: 10.1073/pnas.0409559102
119. Abuaita, BH , and Withey, JH . Bicarbonate induces Vibrio cholerae virulence gene expression by enhancing ToxT activity. Infect Immun. (2009) 77:4111–20. doi: 10.1128/IAI.00409-09
120. Pennetzdorfer, N , Lembke, M , Pressler, K , Matson, JS , Reidl, J , and Schild, S . Regulated proteolysis in Vibrio cholerae allowing rapid adaptation to stress conditions. Front Cell Infect Microbiol. (2019) 9:1–9. doi: 10.3389/fcimb.2019.00214
121. Atuma, C , Strugala, V , Allen, A , and Holm, L . The adherent gastrointestinal mucus gel layer: thickness and physical state in vivo. Am J Physiol Gastrointest Liver Physiol. (2001) 280:922–9. doi: 10.1152/ajpgi.2001.280.5.g922
122. Butler, SM , and Camilli, A . Going against the grain: chemotaxis and infection in Vibrio cholerae. Nat Rev Microbiol. (2005) 3:611–20. doi: 10.1038/nrmicro1207
123. Stewart-Tull, DES , Ollar, RA , and Scobie, TS . Studies on the Vibrio cholerae mucinase complex. I. Enzymic activities associated with the complex. J Med Microbiol. (1986) 22:325–33. doi: 10.1099/00222615-22-4-325
124. Silva, AJ , and Benitez, JA . Vibrio cholerae biofilms and cholera pathogenesis. PLoS Negl Trop Dis. (2016) 10:1–25. doi: 10.1371/journal.pntd.0004330
125. Cho, JY , Liu, R , Macbeth, JC , and Hsiao, A . The Interface of Vibrio cholerae and the gut microbiome. Gut Microbes. (2021) 13:1937015. doi: 10.1080/19490976.2021.1937015
126. Bachmann, V , Kostiuk, B , Unterweger, D , Diaz-Satizabal, L , Ogg, S , and Pukatzki, S . Bile salts modulate the mucin-activated type VI secretion system of pandemic Vibrio cholerae. PLoS Negl Trop Dis. (2015) 9:e0004031. doi: 10.1371/journal.pntd.0004031
127. Santoriello, FJ , Michel, L , Unterweger, D , and Pukatzki, S . Pandemic Vibrio cholerae shuts down site-specific recombination to retain an interbacterial defence mechanism. Nat Commun. (2020) 11:6246. doi: 10.1038/s41467-020-20012-7
128. Alavi, S , Mitchell, JD , Cho, JY , Liu, R , Macbeth, JC , and Hsiao, A . Interpersonal gut microbiome variation drives susceptibility and resistance to cholera infection. Cells. (2020) 181:1533–1546.e13. doi: 10.1016/j.cell.2020.05.036
129. Jude, BA , Martinez, RM , Skorupski, K , and Taylor, RK . Levels of the secreted Vibrio cholerae attachment factor GbpA are modulated by quorum-sensing-induced proteolysis. J Bacteriol. (2009) 191:6911–7. doi: 10.1128/JB.00747-09
130. Bhowmick, R , Ghosal, A , Das, B , Koley, H , Saha, DR , Ganguly, S, et al. Intestinal adherence of Vibrio cholerae involves a coordinated interaction between colonization factor GbpA and mucin. Infect Immun. (2008) 76:4968–77. doi: 10.1128/IAI.01615-07
131. Sperandio, V , Giron, JA , Silveira, WD , and Kaper, JB . The OmpU outer membrane protein, a potential adherence factor of Vibrio cholerae. Infect Immun. (1995) 63:4433–8. doi: 10.1128/iai.63.11.4433-4438.1995
132. Yang, M , Liu, Z , Hughes, C , Stern, AM , Wang, H , Zhong, Z, et al. Bile salt-induced intermolecular disulfide bond formation activates Vibrio cholerae virulence. Proc Natl Acad Sci U S A. (2013) 110:2348–53. doi: 10.1073/pnas.1218039110
133. Herrington, DA , Hall, RH , Losonsky, G , Mekalanos, JJ , Taylor, RK , and Levine, MM . Toxin, toxin-coregulated pili, and the toxR regulon are essential for Vibrio cholerae pathogenesis in humans. J Exp Med. (1988) 168:1487–92. doi: 10.1084/jem.168.4.1487
134. Kirn, TJ , Lafferty, MJ , Sandoe, CMP , and Taylor, RK . Delineation of pilin domains required for bacterial association into microcolonies and intestinal colonization by Vibrio cholerae. Mol Microbiol. (2000) 35:896–910. doi: 10.1046/j.1365-2958.2000.01764.x
135. Miniewcz, A , Jakubas, R , and Eoolivei, C . Ferroelasticity of [NH2(CH3)2]3Sb2Br9 (dmaba). Ferroelectrics. (1990) 106:249–54. doi: 10.1080/00150199008214591
136. Zhang, RG , Westbrook, ML , Nance, S , Spangler, BD , Westbrook, EM , Scott, DL, et al. The three-dimensional crystal structure of cholera toxin. J Mol Biol. (1995) 251:563–73. doi: 10.1006/jmbi.1995.0456
137. Nours, L , Rossjohn, J , Beddoe, T , Paton, AW , and Paton, JC . Structure, biological functions and applications of the AB 5 toxins. Trends Biochem Sci. (2010) 35:411–8. doi: 10.1016/j.tibs.2010.02.003
138. Vanden, DV , Horvath, C , and De Wolf, MJ . Vibrio cholerae: cholera toxin. Int J Biochem Cell Biol. (2020) 39:1771–5. doi: 10.1016/j.biocel.2007.07.005
139. Kauffman, RC , Bhuiyan, TR , Nakajima, R , Mayo-Smith, LM , Rashu, R , Hoq, MR, et al. Single-cell analysis of the plasmablast response to Vibrio cholerae demonstrates expansion of cross-reactive memory B cells. mBio. (2016) 7:e02021-16. doi: 10.1128/mBio.02021-16
140. Heim, JB , Hodnik, V , Heggelund, JE , and Anderluh, G . Crystal structures of cholera toxin in complex with fucosylated receptors point to importance of secondary binding site. Sci Rep. (2019) 9:12243. doi: 10.1038/s41598-019-48579-2
141. Pérez-Reytor, D , Jaña, V , Pavez, L , Navarrete, P , and García, K . Accessory toxins of Vibrio pathogens and their role in epithelial disruption during infection. Front Microbiol. (2018) 9:1–11. doi: 10.3389/fmicb.2018.02248
142. Nielsen, AT , Dolganov, NA , Otto, G , Miller, MC , Cheng, YW , and Schoolnik, GK . RpoS controls the Vibrio cholerae mucosal escape response. PLoS Pathog. (2006) 2:0933–48. doi: 10.1371/journal.ppat.0020109
143. Zhu, J , Miller, MB , Vance, RE , Dziejman, M , Bassler, BL , and Mekalanos, JJ . Quorum-sensing regulators control virulence gene expression in Vibrio cholerae. Proc Natl Acad Sci U S A. (2002) 99:3129–34. doi: 10.1073/pnas.052694299
144. Wang, H , Ayala, JC , Benitez, JA , and Silva, AJ . Interaction of the histone-like nucleoid structuring protein and the general stress response regulator rpos at Vibrio cholerae promoters that regulate motility and hemagglutinin/protease expression. J Bacteriol. (2012) 194:1205–15. doi: 10.1128/JB.05900-11
145. Zhu, J , and Mekalanos, JJ . Quorum sensing-dependent biofilms enhance colonization in Vibrio cholerae, the phenomenon by which bacteria monitor their cell population density through the extracellular accumulation of signaling molecules called autoinduc. Dev Cell. (2003) 5:647–656. doi: 10.1016/S1534-5807(03)00295-8
146. Merrell, DS , Butler, SM , Qadri, F , Dolganov, NA , Alam, A , Cohen, MB, et al. Host-induced epidemic spread of the cholera bacterium. Nature. (2002) 417:642–5. doi: 10.1038/nature00778
147. Butler, SM , Nelson, EJ , Chowdhury, N , Faruque, SM , Calderwood, SB , and Camilli, A . Cholera stool bacteria repress chemotaxis to increase infectivity. Mol Microbiol. (2006) 60:417–26. doi: 10.1111/j.1365-2958.2006.05096.x
148. Nelson, EJ , Chowdhury, A , Flynn, J , Schild, S , Bourassa, L , Shao, Y, et al. Transmission of Vibrio cholerae is antagonized by lytic phage and entry into the aquatic environment. PLoS Pathog. (2008) 4:e1000187. doi: 10.1371/journal.ppat.1000187
149. Nelson, EJ , Harris, JB , Morris, JG , Calderwood, SB , and Camilli, A . Cholera transmission: the host, pathogen and bacteriophage dynamic. Nat Rev Microbiol. (2009) 7:693–702. doi: 10.1038/nrmicro2204
150. Benčić, Z , and Sinha, R . Cholera carriers and circulation of cholera vibrios in the community. Int J Epidemiol. (1972) 1:13–4. doi: 10.1093/ije/1.1.13
151. Leung, T , and Matrajt, L . Protection afforded by previous Vibrio cholerae infection against subsequent disease and infection: a review. PLoS Negl Trop Dis. (2021) 15:1–17. doi: 10.1371/journal.pntd.0009383
152. Naidu, A , and Lulu, SS . Mucosal and systemic immune responses to Vibrio cholerae infection and oral cholera vaccines (OCVs) in humans: a systematic review. Expert Rev Clin Immunol. (2022) 18:1307–18. doi: 10.1080/1744666X.2022.2136650
153. Qadri, F , Bhuiyan, TR , Dutta, KK , Raqib, R , Alam, MS , and Alam, NH . Acute dehydrating disease caused by Vibrio cholerae serogroups O1 and O139 induce increases in innate cells and inflammatory mediators at the mucosal surface of the gut. Gut. (2004) 53:62–9. doi: 10.1136/gut.53.1.62
154. Flach, CF , Qadri, F , Bhuiyan, TR , Alam, NH , Jennische, E , Lönnroth, I, et al. Broad up-regulation of innate defense factors during acute cholera. Infect Immun. (2007) 75:2343–50. doi: 10.1128/IAI.01900-06
155. Mathan, MM , Chandy, G , and Mathan, VI . Ultrastructural changes in the upper small intestinal mucosa in patients with cholera. Gastroenterology. (1995) 109:422–30. doi: 10.1016/0016-5085(95)90329-1
156. Peterson, LW , and Artis, D . Intestinal epithelial cells: regulators of barrier function and immune homeostasis. Nat Rev Immunol. (2014) 14:141–53. doi: 10.1038/nri3608
157. Bandyopadhaya, A , Sarkar, M , and Chaudhuri, K . Transcriptional upregulation of inflammatory cytokines in human intestinal epithelial cells following Vibrio cholerae infection. FEBS J. (2007) 274:4631–42. doi: 10.1111/j.1742-4658.2007.05991.x
158. Rodríguez, BL , Rojas, A , Campos, J , Ledon, T , Valle, E , Toledo, W, et al. Differential interleukin-8 response of intestinal epithelial cell line to reactogenic and nonreactogenic candidate vaccine strains of Vibrio cholerae. Infect Immun. (2001) 69:613–6. doi: 10.1128/IAI.69.1.613-616.2001
159. Harrison, LM , Rallabhandi, P , Michalski, J , Zhou, X , Steyert, SR , Vogel, SN, et al. Vibrio cholerae flagellins induce toll-like receptor 5-mediated interleukin-8 production through mitogen-activated protein kinase and NF-κB activation. Infect Immun. (2008) 76:5524–34. doi: 10.1128/IAI.00843-08
160. Bourque, DL , Bhuiyan, TR , Genereux, DP , Rashu, R , Ellis, CN , Chowdhury, F, et al. Analysis of the human mucosal response to cholera reveals sustained activation of innate immune signaling pathways. Infect Immun. (2018) 86:e00594-17. doi: 10.1128/IAI.00594-17
161. Sarkar, M , and Chaudhuri, K . Association of adherence and motility in interleukin 8 induction in human intestinal epithelial cells by Vibrio cholerae. Microbes Infect. (2004) 6:676–85. doi: 10.1016/j.micinf.2004.02.018
162. Ellis, CN , LaRocque, RC , Uddin, T , Krastins, B , Mayo-Smith, LM , Sarracino, D, et al. Comparative proteomic analysis reveals activation of mucosal innate immune signaling pathways during cholera. Infect Immun. (2015) 83:1089–103. doi: 10.1128/IAI.02765-14
163. Bandyopadhaya, A , Sarkar, M , and Chaudhuri, K . Human intestinal epithelial cell cytokine mRNA responses mediated by NF-κB are modulated by the motility and adhesion process of Vibrio cholerae. Int J Biochem Cell Biol. (2007) 39:1863–76. doi: 10.1016/j.biocel.2007.05.005
164. Yang, JS , Jeon, JH , Jang, MS , Kang, SS , Ahn, KB , Song, M, et al. Vibrio cholerae OmpU induces IL-8 expression in human intestinal epithelial cells. Mol Immunol. (2018) 93:47–54. doi: 10.1016/j.molimm.2017.11.005
165. McGee, DW , Elson, CO , and McGhee, JR . Enhancing effect of cholera toxin on interleukin-6 secretion by IEC-6 intestinal epithelial cells: mode of action and augmenting effect of inflammatory cytokines. Infect Immun. (1993) 61:4637–44. doi: 10.1128/iai.61.11.4637-4644.1993
166. Sitaraman, SV , Merlin, D , Wang, L , Wong, M , Gewirtz, AT , Si-Tahar, M, et al. Neutrophil-epithelial crosstalk at the intestinal lumenal surface mediated by reciprocal secretion of adenosine and IL-6. J Clin Invest. (2001) 107:861–9. doi: 10.1172/JCI11783
167. Taub, DD , Anver, M , Oppenheim, JJ , Longo, DL , and Murphy, WJ . T lymphocyte recruitment by interleukin-8 (IL-8): IL-8-induced degranulation of neutrophils releases potent chemoattractants for human T lymphocytes both in vitro and in vivo. J Clin Invest. (1996) 97:1931–41. doi: 10.1172/JCI118625
168. Remick, DG . Interleukin-8. Crit Care Med. (2005) 33:466–7. doi: 10.1097/01.CCM.0000186783.34908.18
169. Carr, MW , Roth, SJ , Luther, E , Rose, SS , and Springer, TA . Monocyte chemoattractant protein 1 acts as a T-lymphocyte chemoattractant. Proc Natl Acad Sci U S A. (1994) 91:3652–6. doi: 10.1073/pnas.91.9.3652
170. Xu, LL , Warren, MK , Rose, WL , Gong, W , and Wang, JM . Human recombinant monocyte chemotactic protein and other c-c chemokines bind and induce directional migration of dendritic cells in vitro. J Leukoc Biol. (1996) 60:365–71. doi: 10.1002/jlb.60.3.365
171. Pulimood, AB , Ramakrishna, BS , Rita, AB , Srinivasan, P , Mohan, V , Gupta, S, et al. Early activation of mucosal dendritic cells and macrophages in acute Campylobacter colitis and cholera: an in vivo study. J Gastroenterol Hepatol. (2008) 23:752–8. doi: 10.1111/j.1440-1746.2008.05325.x
172. Bhuiyan, TR , Rahman, MA , Trivedi, S , Afroz, T , Al Banna, H , Hoq, MR, et al. Mucosal-associated invariant T (MAIT) cells are highly activated in duodenal tissue of humans with Vibrio cholerae O1 infection: a preliminary report. PLoS Negl Trop Dis. (2022) 16:e0010411. doi: 10.1371/journal.pntd.0010411
173. Jensen, O , Trivedi, S , Meier, JD , Fairfax, KC , Hale, JS , and Leung, DT . A subset of follicular helper-like MAIT cells can provide B cell help and support antibody production in the mucosa. Sci Immunol. (2022) 7:1–14. doi: 10.1126/sciimmunol.abe8931
174. Qadri, F , Raqib, R , Ahmed, F , Rahman, T , Wenneras, C , Das, SK, et al. Increased levels of inflammatory mediators in children and adults infected with Vibrio cholerae O1 and O139. Clin Diagn Lab Immunol. (2002) 9:221–9. doi: 10.1128/CDLI.9.2.221-229.2002
175. Janoff, EN , Hayakawa, H , Taylor, DN , Fasching, CE , Kenner, JR , Jaimes, E, et al. Nitric oxide production during Vibrio cholerae infection. Am J Physiol Gastrointest Liver Physiol. (1997) 273:1160–7. doi: 10.1152/ajpgi.1997.273.5.g1160
176. Arnold, RR , Cole, MF , and McGhee, JR . A bactericidal effect for human lactoferrin. Science. (1977) 197:263–5. doi: 10.1126/science.327545
177. Arnold, RR , Brewer, M , and Gauthier, JJ . Bactericidal activity of human lactoferrin: sensitivity of a variety of microorganisms. Infect Immun. (1980) 28:893–8. doi: 10.1128/iai.28.3.893-898.1980
178. Queen, J , and Fullner Satchell, KJ . Neutrophils are essential for containment of Vibrio cholerae to the intestine during the proinflammatory phase of infection. Infect Immun. (2012) 80:2905–13. doi: 10.1128/IAI.00356-12
179. Dolores, J , and Satchell, KJF . Analysis of Vibrio cholerae genome sequences reveals unique rtxA variants in environmental strains and an rtxA-null mutation in recent altered EL tor isolates. mBio. (2013) 4:e00624-12. doi: 10.1128/mBio.00624-12
180. Mörbe, UM , Jørgensen, PB , Fenton, TM , von Burg, N , Riis, LB , Spencer, J, et al. Human gut-associated lymphoid tissues (GALT); diversity, structure, and function. Mucosal Immunol. (2021) 14:793–802. doi: 10.1038/s41385-021-00389-4
181. Sheridan, BS , and Lefrançois, L . Regional and mucosal memory T cells. Nat Immunol. (2011) 12:485–91. doi: 10.1038/ni.2029
182. Owen, RL , Pierce, NF , Apple, RT , and Cray, WC . M cell transport of Vibrio cholerae from the intestinal lumen into Peyer’s patches: a mechanism for antigen sampling and for microbial transepithelial migration. J Infect Dis. (1986) 153:1108–18. doi: 10.1093/infdis/153.6.1108
183. Kim, MS , Yi, EJ , Kim, YI , Kim, SH , Jung, YS , Kim, SR, et al. ERDj5 in innate immune cells is a crucial factor for the mucosal adjuvanticity of cholera toxin. Front Immunol. (2019) 10:1249. doi: 10.3389/fimmu.2019.01249
184. Gagliardi, MC , Sallusto, F , Marinaro, M , Langenkamp, A , Lanzavecchia, A , and De Magistris, MT . Cholera toxin induces maturation of human dendritic cells and licences them for Th2 priming. Eur J Immunol. (2000) 30:2394–403. doi: 10.1002/1521-4141(2000)30:8<2394::AID-IMMU2394>3.0.CO;2-Y
185. Lavelle, EC , McNeela, E , Armstrong, ME , Leavy, O , Higgins, SC , and Mills, KHG . Cholera toxin promotes the induction of regulatory T cells specific for bystander antigens by modulating dendritic cell activation. J Immunol. (2003) 171:2384–92. doi: 10.4049/jimmunol.171.5.2384
186. Datta, SK , Sabet, M , Nguyen, KPL , Valdez, PA , Gonzalez-Navajas, JM , Islam, S, et al. Mucosal adjuvant activity of cholera toxin requires Th17 cells and protects against inhalation anthrax. Proc Natl Acad Sci U S A. (2010) 107:10638–43. doi: 10.1073/pnas.1002348107
187. Kimura, A , and Kishimoto, T . IL-6: regulator of Treg/Th17 balance. Eur J Immunol. (2010) 40:1830–5. doi: 10.1002/eji.201040391
188. Shreedhar, VK , Kelsall, BL , and Neutra, MR . Cholera toxin induces migration of dendritic cells from the subepithelial dome region to T- and B-cell areas of Peyer’s patches. Infect Immun. (2003) 71:504–9. doi: 10.1128/IAI.71.1.504-509.2003
189. Weil, AA , Ellis, CN , Debela, MD , Bhuiyan, TR , Rashu, R , Bourque, DL, et al. Posttranslational regulation of IL-23 production distinguishes the innate immune responses to live toxigenic versus heat-inactivated Vibrio cholerae. mSphere. (2019) 4:e00206-19. doi: 10.1128/msphere.00206-19
190. Zughaier, SM , Zimmer, SM , Datta, A , Carlson, RW , and Stephens, DS . Differential induction of the toll-like receptor 4-MyD88-dependent and -independent signaling pathways by endotoxins. Infect Immun. (2005) 73:2940–50. doi: 10.1128/IAI.73.5.2940-2950.2005
191. Khader, SA , Gaffen, SL , and Kolls, JK . Th17 cells at the crossroads of innate and adaptive immunity against infectious diseases at the mucosa. Mucosal Immunol. (2009) 2:403–11. doi: 10.1038/mi.2009.100
192. Volpe, E , Servant, N , Zollinger, R , Bogiatzi, SI , Hupé, P , Barillot, E, et al. A critical function for transforming growth factor-β, interleukin 23 and proinflammatory cytokines in driving and modulating human TH-17 responses. Nat Immunol. (2008) 9:650–7. doi: 10.1038/ni.1613
193. Kuchta, A , Rahman, T , Sennott, EL , Bhuyian, TR , Uddin, T , Rashu, R, et al. Vibrio cholerae O1 infection induces proinflammatory CD4 + T-cell responses in blood and intestinal mucosa of infected humans. Clin Vaccine Immunol. (2011) 18:1371–7. doi: 10.1128/CVI.05088-11
194. Bhuiyan, TR , Lundin, SB , Khan, AI , Lundgren, A , Harris, JB , Calderwood, SB, et al. Cholera caused by Vibrio cholerae O1 induces T-cell responses in the circulation. Infect Immun. (2009) 77:1888–93. doi: 10.1128/IAI.01101-08
195. Qadri, F , Clemens, JD , and Holmgren, J . Cholera immunity and development and use of oral cholera vaccines for disease control In: H Kiyono and DW Pascual, editors. Mucosal vaccines. 2nd ed. Amsterdam: Elsevier Inc (2019). 537–61.
196. Crotty, S . T follicular helper cell differentiation, function, and roles in disease. Immunity. (2014) 41:529–42. doi: 10.1016/j.immuni.2014.10.004
197. Rashu, R , Bhuiyan, TR , Hoq, MR , Hossain, L , Paul, A , Khan, AI, et al. Cognate T and B cell interaction and association of follicular helper T cells with B cell responses in Vibrio cholerae O1 infected Bangladeshi adults. Microbes Infect. (2019) 21:176–83. doi: 10.1016/j.micinf.2018.12.002
198. Charles, RC , Nakajima, R , Liang, L , Jasinskas, A , Berger, A , Leung, DT, et al. Plasma and mucosal immunoglobulin M, immunoglobulin a, and immunoglobulin G responses to the Vibrio cholerae O1 protein immunome in adults with cholera in Bangladesh. J Infect Dis. (2017) 216:125–34. doi: 10.1093/infdis/jix253
199. Mayo-Smith, LM , Simon, JK , Chen, WH , Haney, D , Lock, M , Lyon, CE, et al. The live attenuated cholera vaccine CVD 103-HgR primes responses to the toxin-coregulated pilus antigen TcpA in subjects challenged with wild-type Vibrio cholerae. Clin Vaccine Immunol. (2017) 24:e00470-16. doi: 10.1128/CVI.00470-16
200. Harris, AM , Bhuiyan, MS , Chowdhury, F , Khan, AI , Hossain, A , Kendall, EA, et al. Antigen-specific memory B-cell responses to Vibrio cholerae O1 infection in Bangladesh. Infect Immun. (2009) 77:3850–6. doi: 10.1128/IAI.00369-09
201. Arifuzzaman, M , Rashu, R , Leung, DT , Hosen, MI , Bhuiyan, TR , Bhuiyan, MS, et al. Antigen-specific memory T cell responses after vaccination with an oral killed cholera vaccine in Bangladeshi children and comparison to responses in patients with naturally acquired cholera. Clin Vaccine Immunol. (2012) 19:1304–11. doi: 10.1128/CVI.00196-12
202. Weil, AA , Becker, RL , and Harris, JB . Vibrio cholerae at the intersection of immunity and the microbiome. mSphere. (2019) :e00597-19. doi: 10.1128/msphere.00597-19
203. Weil, AA , Arifuzzaman, M , Bhuiyan, TR , LaRocque, RC , Harris, AM , Kendall, EA, et al. Memory T-cell responses to Vibrio cholerae O1 infection. Infect Immun. (2009) 77:5090–6. doi: 10.1128/IAI.00793-09
204. Chowdhury, F , Khan, AI , Harris, JB , Larocque, RC , Chowdhury, MI , Ryan, ET, et al. A comparison of clinical and immunologic features in children and older patients hospitalized with severe cholera in Bangladesh. Pediatr Infect Dis J. (2008) 27:986–92. doi: 10.1097/INF.0b013e3181783adf
205. Aktar, A , Rahman, MA , Afrin, S , Akter, A , Uddin, T , Yasmin, T, et al. Plasma and memory B cell responses targeting O-specific polysaccharide (OSP) are associated with protection against Vibrio cholerae O1 infection among household contacts of cholera patients in Bangladesh. PLoS Negl Trop Dis. (2018) 12:1–15. doi: 10.1371/journal.pntd.0006399
206. Harris, JB . Cholera: immunity and prospects in vaccine development. J Infect Dis. (2018) 218:S141–6. doi: 10.1093/infdis/jiy414
207. Saha, D , LaRocque, RC , Khan, AI , Harris, JB , Begum, YA , Akramuzzaman, SM, et al. Incomplete correlation of serum vibriocidal antibody titer with protection from Vibrio cholerae infection in urban Bangladesh. J Infect Dis. (2004) 189:2318–22. doi: 10.1086/421275
208. Haney, DJ , Lock, MD , Simon, JK , Harris, J , and Gurwith, M . Antibody-based correlates of protection against cholera: analysis of a challenge study of a cholera-naive population. Clin Vaccine Immunol. (2017) 24:1–11. doi: 10.1128/CVI.00098-17
209. Chen, K , Magri, G , Grasset, EK , and Cerutti, A . Rethinking mucosal antibody responses: IgM, IgG and IgD join IgA. Nat Rev Immunol. (2020) 20:427–41. doi: 10.1038/s41577-019-0261-1
210. Kamada, N , Sakamoto, K , Seo, S-U , Zeng, MY , Kim, Y-G , Cascalho, M, et al. Humoral immunity in the gut selectively targets phenotypically virulent attaching-and-effacing Bacteria for intraluminal elimination. Cell Host Microbe. (2015) 17:617–27. doi: 10.1016/j.chom.2015.04.001
211. Charles, RC , Kelly, M , Tam, JM , Akter, A , Hossain, M , Islam, K, et al. Humans surviving cholera develop antibodies against Vibrio cholerae O-specific polysaccharide that inhibit pathogen motility. MBio. (2020) 11:1–13. doi: 10.1128/mBio.02847-20
212. Apter, FM , Michetti, P , Winner, LS , Mack, JA , Mekalanos, JJ , and Neutra, MR . Analysis of the roles of antilipopolysaccharide and anti-cholera toxin immunologlubulin A (IgA) antibodies in protection against Vibrio cholerae and cholera toxin by use of monoclonal IgA antibodies in vivo. Infect Immun. (1993) 61:5279–85. doi: 10.1128/iai.61.12.5279-5285.1993
213. Patel, SM , Rahman, MA , Mohasin, M , Riyadh, MA , Leung, DT , Alam, MM, et al. Memory B cell responses to Vibrio cholerae O1 lipopolysaccharide are associated with protection against infection from household contacts of patients with cholera in Bangladesh. Clin Vaccine Immunol. (2012) 19:842–8. doi: 10.1128/CVI.00037-12
214. Kaisar, MH , Bhuiyan, MS , Akter, A , Saleem, D , Iyer, AS , Dash, P, et al. Vibrio cholerae sialidase-specific immune responses are associated with protection against cholera. mSphere. (2021) 6:e01232-20. doi: 10.1128/msphere.01232-20
215. Holmgren, J . Modern history of cholera vaccines and the pivotal role of icddr,b. J Infect Dis. (2021) 224:S742–8. doi: 10.1093/infdis/jiab423
216. Czerkinsky, C , and Holmgren, J . Mucosal delivery routes for optimal immunization: targeting immunity to the right tissues In: P Kozlowski , editor. Mucosal vaccines. Current topics in microbiology and immunology. Berlin, Heidelberg: Springer (2010)
217. Davitt, CJH , and Lavelle, EC . Delivery strategies to enhance oral vaccination against enteric infections. Adv Drug Deliv Rev. (2015) 91:52–69. doi: 10.1016/j.addr.2015.03.007
218. Ali, M , Emch, M , Von Seidlein, L , Yunus, M , Sack, DA , Rao, M, et al. Herd immunity conferred by killed oral cholera vaccines in Bangladesh: a reanalysis. Lancet. (2005) 366:44–9. doi: 10.1016/S0140-6736(05)66550-6
219. Ali, M , Kim, P , Zaman, K , and Clemens, J . Herd protection of unvaccinated adults by oral cholera vaccines in rural Bangladesh. Int Health. (2019) 11:229–34. doi: 10.1093/inthealth/ihy085
220. Clemens, JD , Sack, DA , Harris, JR , van Loon, F , Chakraborty, J , Ahmed, F, et al. Field trial of oral cholera vaccines in Bangladesh: results from three-year follow-up. Lancet. (1990) 335:270–3. doi: 10.1016/0140-6736(90)90080-O
221. Sanchez, JL , Vasquez, B , Begue, RE , Meza, R , Castellares, G , Cabezas, C, et al. Protective efficacy of oral whole-cell/recombinant-B-subunit cholera vaccine in Peruvian military recruits. Lancet. (1994) 344:1273–6. doi: 10.1016/S0140-6736(94)90755-2
222. Lucas, MES , Deen, JL , von Seidlein, L , Wang, X-Y , Ampuero, J , Puri, M, et al. Effectiveness of mass oral cholera vaccination in Beira, Mozambique. N Engl J Med. (2005) 352:757–67. doi: 10.1056/NEJMoa043323
223. Quiding, M , Nordström, I , Kilander, A , Andersson, G , Hanson, LÅ , Holmgren, J, et al. Intestinal immune responses in humans: Oral cholera vaccination induces strong intestinal antibody responses and interferon-γ production and evokes local immunological memory. J Clin Invest. (1991) 88:143–8. doi: 10.1172/JCI115270
224. Clemens, JD , Sack, DA , Harris, JR , Chakraborty, J , Neogy, PK , Stanton, B, et al. Cross-protection by B subunit-whole cell cholera vaccine against diarrhea associated with heat-labile toxin-producing enterotoxigenic Escherichia coli: results of a large-scale field trial. J Infect Dis. (1988) 158:372–7. doi: 10.1093/infdis/158.2.372
225. Jertborn, M , Svennerholm, AM , and Holmgren, J . Intestinal and systemic imune responses in humans after oral immunization with a bivalent B subunit-O1/O139 whole cell cholera vaccine. Vaccine. (1996) 14:1459–65. doi: 10.1016/S0264-410X(96)00071-0
226. Shamsuzzaman, S , Ahmed, T , Mannoor, K , Begum, YA , Bardhan, PK , Sack, RB, et al. Robust gut associated vaccine-specific antibody-secreting cell responses are detected at the mucosal surface of Bangladeshi subjects after immunization with an oral killed bivalent V. cholerae O1/O139 whole cell cholera vaccine: comparison with other muco. Vaccine. (2009) 27:1386–92. doi: 10.1016/j.vaccine.2008.12.041
227. Holmgren, J . An update on cholera immunity and current and future cholera vaccines. Trop Med Infect Dis. (2021) 6:64. doi: 10.3390/tropicalmed6020064
228. Bhattacharya, SK , Sur, D , Ali, M , Kanungo, S , You, YA , Manna, B, et al. 5 year efficacy of a bivalent killed whole-cell oral cholera vaccine in Kolkata, India: a cluster-randomised, double-blind, placebo-controlled trial. Lancet Infect Dis. (2013) 13:1050–6. doi: 10.1016/S1473-3099(13)70273-1
229. Sur, D , Lopez, AL , Kanungo, S , Paisley, A , Manna, B , Ali, M, et al. Efficacy and safety of a modified killed-whole-cell oral cholera vaccine in India: an interim analysis of a cluster-randomised, double-blind, placebo-controlled trial. Lancet. (2009) 374:1694–702. doi: 10.1016/S0140-6736(09)61297-6
230. Odevall, L , Hong, D , Digilio, L , Sahastrabuddhe, S , Mogasale, V , Baik, Y, et al. The Euvichol story – development and licensure of a safe, effective and affordable oral cholera vaccine through global public private partnerships. Vaccine. (2018) 36:6606–14. doi: 10.1016/j.vaccine.2018.09.026
231. Chen, Q , Yu, S , and Wang, Y . Community trial for safety and immunogenicity of oral-administered lyophilized rBS-WC cholera vaccine. Zhonghua Yu Fang Yi Xue Za Zhi. (1996) 30:330–3.
232. Chowdhury, F , Akter, A , Bhuiyan, TR , Tauheed, I , Teshome, S , Sil, A, et al. A non-inferiority trial comparing two killed, whole cell, oral cholera vaccines (Cholvax vs. Shanchol) in Dhaka, Bangladesh. Vaccine. (2022) 40:640–9. doi: 10.1016/j.vaccine.2021.12.015
233. Deen, J , and Clemens, JD . Licensed and recommended inactivated Oral CholeraVaccines: from development to innovative deployment. Trop Med Infect Dis. (2021) 6:32. doi: 10.3390/tropicalmed6010032
234. Qadri, F , Wierzba, TF , Ali, M , Chowdhury, F , Khan, AI , Saha, A, et al. Efficacy of a single-dose, inactivated oral cholera vaccine in Bangladesh. N Engl J Med. (2016) 374:1723–32. doi: 10.1056/nejmoa1510330
235. Qadri, F , Ali, M , Lynch, J , Chowdhury, F , Khan, AI , Wierzba, TF, et al. Efficacy of a single-dose regimen of inactivated whole-cell oral cholera vaccine: results from 2 years of follow-up of a randomised trial. Lancet Infect Dis. (2018) 18:666–74. doi: 10.1016/S1473-3099(18)30108-7
236. Hauke, CA , and Taylor, RK . Production of putative enhanced oral cholera vaccine strains that express toxin-coregulated pilus. PLoS One. (2017) 12:e0175170. doi: 10.1371/journal.pone.0175170
237. Di Tommaso, A , De Magistris, MT , Bugnoli, M , Marsili, I , Rappuoli, R , and Abrignani, S . Formaldehyde treatment of proteins can constrain presentation to T cells by limiting antigen processing. Infect Immun. (1994) 62:1830–4. doi: 10.1128/iai.62.5.1830-1834.1994
238. McCarty, J , Bedell, L , De Lame, P-A , Cassie, D , Lock, M , Bennett, S, et al. Update on CVD 103-HgR single-dose, live oral cholera vaccine. Expert Rev Vaccines. (2022) 21:9–23. doi: 10.1080/14760584.2022.2003709
239. Levine, MM , Chen, WH , Kaper, JB , Lock, M , Danzig, L , and Gurwith, M . PaxVax CVD 103-HgR single-dose live oral cholera vaccine. Expert Rev Vaccines. (2017) 16:197–213. doi: 10.1080/14760584.2017.1291348
240. Tacket, CO , Cohen, MB , Wasserman, SS , Losonsky, G , Livio, S , Kotloff, K, et al. Randomized, double-blind, placebo-controlled, multicentered trial of the efficacy of a single dose of live oral cholera vaccine CVD 103-HgR in preventing cholera following challenge with Vibrio cholerae O1 El Tor Inaba three months after vaccination. Infect Immun. (1999) 67:6341–5. doi: 10.1128/iai.67.12.6341-6345.1999
241. McCarty, JM , Gierman, EC , Bedell, L , Lock, MD , and Bennett, S . Safety and immunogenicity of live oral cholera vaccine CVD 103-HgR in children and adolescents aged 6-17 years. Am J Trop Med Hyg. (2020) 102:48–57. doi: 10.4269/ajtmh.19-0241
242. Richie, E , Punjabi, NH , Sidharta, Y , Peetosutan, K , Sukandar, M , Wasserman, SS, et al. Efficacy trial of single-dose live oral cholera vaccine CVD 103-HgR in North Jakarta, Indonesia, a cholera-endemic area. Vaccine. (2000) 18:2399–410. doi: 10.1016/S0264-410X(00)00006-2
243. Calain, P , Chaine, JP , Johnson, E , Lou, HM , O’Leary, MJ , Oshitani, H, et al. Can oral cholera vaccination play a role in controlling a cholera outbreak? Vaccine. (2004) 22:2444–51. doi: 10.1016/j.vaccine.2003.11.070
244. Song, KR , Lim, JK , Park, SE , Saluja, T , Il, CS , Wartel, TA, et al. Oral cholera vaccine efficacy and effectiveness. Vaccine. (2021) 9:1482. doi: 10.3390/vaccines9121482
245. Sharma, T , Joshi, N , Kumar Mandyal, A , Nordqvist, SL , Lebens, M , Kanchan, V, et al. Development of Hillchol®, a low-cost inactivated single strain Hikojima oral cholera vaccine. Vaccine. (2020) 38:7998–8009. doi: 10.1016/j.vaccine.2020.10.043
246. Chowdhury, F , Ali Syed, K , Akter, A , Rahman Bhuiyan, T , Tauheed, I , Khaton, F, et al. A phase I/II study to evaluate safety, tolerability and immunogenicity of Hillchol®, an inactivated single Hikojima strain based oral cholera vaccine, in a sequentially age descending population in Bangladesh. Vaccine. (2021) 39:4450–7. doi: 10.1016/j.vaccine.2021.06.069
247. Taylor, DN , Killeen, KP , Hack, DC , Kenner, JR , Coster, TS , Beattie, DT, et al. Development of a live, oral, attenuated vaccine against el tor cholera. J Infect Dis. (1994) 170:1518–23. doi: 10.1093/infdis/170.6.1518
248. Kenner, JR , Coster, TS , Taylor, DN , Trofa, AF , Barrera-Oro, M , Hyman, T, et al. Peru-15, an improved live attenuated oral vaccine candidate for Vibrio cholerae O1. J Infect Dis. (1995) 172:1126–9. doi: 10.1093/infdis/172.4.1126
249. Sack, DA , Sack, RB , Shimko, J , Gomes, G , O’Sullivan, D , Metcalfe, K, et al. Evaluation of Peru-15, a new live oral vaccine for cholera, in volunteers. J Infect Dis. (1997) 176:201–5. doi: 10.1086/514025
250. Qadri, F , Chowdhury, MI , Faruque, SM , Salam, MA , Ahmed, T , Begum, YA, et al. Randomized, controlled study of the safety and immunogenicity of Peru-15, a live attenuated oral vaccine candidate for cholera, in adult volunteers in Bangladesh. J Infect Dis. (2005) 192:573–9. doi: 10.1086/432074
251. Qadri, F , Chowdhury, MI , Faruque, SM , Salam, MA , Ahmed, T , Begum, YA, et al. Peru-15, a live attenuated oral cholera vaccine, is safe and immunogenic in Bangladeshi toddlers and infants. Vaccine. (2007) 25:231–8. doi: 10.1016/j.vaccine.2006.08.031
252. Ratanasuwan, W , Kim, YH , Sah, BK , Suwanagool, S , Kim, DR , Anekthananon, A, et al. Peru-15 (Choleragarde®), a live attenuated oral cholera vaccine, is safe and immunogenic in human immunodeficiency virus (HIV)-seropositive adults in Thailand. Vaccine. (2015) 33:4820–6. doi: 10.1016/j.vaccine.2015.07.073
253. Cohen, MB , Giannella, RA , Bean, J , Taylor, DN , Parker, S , Hoeper, A, et al. Randomized, controlled human challenge study of the safety, immunogenicity, and protective efficacy of a single dose of Peru-15, a live attenuated oral cholera vaccine. Infect Immun. (2002) 70:1965–70. doi: 10.1128/IAI.70.4.1965-1970.2002
254. Benítez, JA , García, L , Silva, A , García, H , Fando, R , Cedré, B, et al. Preliminary assessment of the safety and immunogenicity of a new CTXΦ- negative, hemagglutinin/protease-defective El Tor strain as a cholera vaccine candidate. Infect Immun. (1999) 67:539–45. doi: 10.1128/iai.67.2.539-545.1999
255. Valera, R , García, HM , Díaz Jidy, M , Mirabal, M , Armesto, MI , Fando, R, et al. Randomized, double-blind, placebo-controlled trial to evaluate the safety and immunogenicity of live oral cholera vaccine 638 in Cuban adults. Vaccine. (2009) 27:6564–9. doi: 10.1016/j.vaccine.2009.08.042
256. García, HM , Thompson, R , Valera, R , Fando, R , Fumane, J , Jani, I, et al. A single dose of live-attenuated 638 Vibrio cholerae oral vaccine is safe and immunogenic in adult volunteers in Mozambique. VacciMonitor. (2011) 20:1–8.
257. García, L , Jidy, MD , García, H , Rodríguez, BL , Fernández, R , Año, G, et al. The vaccine candidate Vibrio cholerae 638 is protective against cholera in healthy volunteers. Infect Immun. (2005) 73:3018–24. doi: 10.1128/IAI.73.5.3018-3024.2005
258. Thungapathra, M , Sharma, C , Gupta, N , Ghosh, RK , Mukhopadhyay, A , Koley, H, et al. Construction of a recombinant live oral vaccine from a non-toxigenic strain of Vibrio cholerae O1 serotype Inaba biotype El Tor and assessment of its reactogenicity and immunogenicity in the rabbit model. Immunol Lett. (1999) 68:219–27. doi: 10.1016/S0165-2478(99)00076-0
259. Mahalanabis, D , Ramamurthy, T , Nair, GB , Ghosh, A , Shaikh, S , Sen, B, et al. Randomized placebo controlled human volunteer trial of a live oral cholera vaccine VA1.3 for safety and immune response. Vaccine. (2009) 27:4850–6. doi: 10.1016/j.vaccine.2009.05.065
260. Kanungo, S , Sen, B , Ramamurthy, T , Sur, D , Manna, B , Pazhani, GP, et al. Safety and immunogenicity of a live oral recombinant cholera vaccine VA1.4: a randomized, placebo controlled trial in healthy adults in a cholera endemic area in Kolkata, India. PLoS One. (2014) 9:e99381. doi: 10.1371/journal.pone.0099381
261. Hubbard, TP , Billings, G , Dörr, T , Sit, B , Warr, AR , Kuehl, CJ, et al. A live vaccine rapidly protects against cholera in an infant rabbit model. Sci Transl Med. (2018) 10:1–11. doi: 10.1126/scitranslmed.aap8423
262. Sit, B , Zhang, T , Fakoya, B , Akter, A , Biswas, R , Ryan, ET, et al. Oral immunization with a probiotic cholera vaccine induces broad protective immunity against Vibrio cholerae colonization and disease in mice. PLoS Negl Trop Dis. (2019) 13:e0007417. doi: 10.1371/journal.pntd.0007417
263. Gupta, RK , Szu, SC , Finkelstein, RA , and Robbins, JB . Synthesis, characterization, and some immunological properties of conjugates composed of the detoxified lipopolysaccharide of Vibrio cholerae O1 serotype inaba bound to cholera toxin. Infect Immun. (1992) 60:3201–8. doi: 10.1128/iai.60.8.3201-3208.1992
264. Gupta, RK , Taylor, DN , Bryla, DA , Robbins, JB , and Szu, SC . Phase 1 evaluation of Vibrio cholerae O1, serotype Inaba, polysaccharide-cholera toxin conjugates in adult volunteers. Infect Immun. (1998) 66:3095–9. doi: 10.1128/iai.66.7.3095-3099.1998
265. Alam, MM , Bufano, MK , Xu, P , Kalsy, A , Yu, Y , Freeman, YW, et al. Evaluation in mice of a conjugate vaccine for cholera made from Vibrio cholerae O1 (Ogawa) O-specific polysaccharide. PLoS Negl Trop Dis. (2014) 8:e2683. doi: 10.1371/journal.pntd.0002683
266. Sayeed, MA , Bufano, MK , Xu, P , Eckhoff, G , Charles, RC , Alam, MM, et al. A cholera conjugate vaccine containing ospecific polysaccharide (OSP) of V. cholerae O1 inaba and recombinant fragment of tetanus toxin heavy chain (OSP:rTTHC) induces serum, memory and lamina proprial responses against OSP and is protective in mice. PLoS Negl Trop Dis. (2015) 9:e0003881. doi: 10.1371/journal.pntd.0003881
267. Akter, A , Kelly, M , Charles, RC , Harris, JB , Calderwood, SB , Bhuiyan, TR, et al. Parenteral vaccination with a cholera conjugate vaccine boosts vibriocidal and anti-osp responses in mice previously immunized with an oral cholera vaccine. Am J Trop Med Hyg. (2021) 104:2024–30. doi: 10.4269/ajtmh.20-1511
268. Jeon, S , Kelly, M , Yun, J , Lee, B , Park, M , Whang, Y, et al. Scalable production and immunogenicity of a cholera conjugate vaccine. Vaccine. (2021) 39:6936–46. doi: 10.1016/j.vaccine.2021.10.005
269. Azegami, T , Itoh, H , Kiyono, H , and Yuki, Y . Novel transgenic rice-based vaccines. Arch Immunol Ther Exp. (2015) 63:87–99. doi: 10.1007/s00005-014-0303-0
270. Gupta, P , Andankar, I , Gunasekaran, B , Easwaran, N , and Kodiveri, MG . Genetically modified potato and rice based edible vaccines – an overview. Biocatal Agric Biotechnol. (2022) 43:102405. doi: 10.1016/j.bcab.2022.102405
271. Salyaev, RK , Rigano, MM , and Rekoslavskaya, NI . Development of plant-based mucosal vaccines against widespread infectious diseases. Expert Rev Vaccines. (2010) 9:937–46. doi: 10.1586/erv.10.81
272. Arakawa, T , Chong, DKX , Lawrence Merritt, J , and Langridge, WHR . Expression of cholera toxin B subunit oligomers in transgenic potato plants. Transgenic Res. (1997) 6:403–13. doi: 10.1023/A:1018487401810
273. Jani, D , Meena, LS , Rizwan-ul-Haq, QM , Singh, Y , Sharma, AK , and Tyagi, AK . Expression of cholera toxin B subunit in transgenic tomato plants. Transgenic Res. (2002) 11:447–54. doi: 10.1023/A:1020336332392
274. Loc, NH , Thinh, LT , Yang, MS , and Kim, TG . Highly expressed cholera toxin B subunit in the fruit of a transgenic tomato (Lycopersicon esculentum L.). Biotechnol Bioprocess Eng. (2011) 16:576–80. doi: 10.1007/s12257-010-0195-8
275. Nochi, T , Takagi, H , Yuki, Y , Yang, L , Masumura, T , Mejima, M, et al. Rice-based mucosal vaccine as a global strategy for cold-chain- and needle-free vaccination. Proc Natl Acad Sci U S A. (2007) 104:10986–91. doi: 10.1073/pnas.0703766104
276. Oszvald, M , Kang, TJ , Tomoskozi, S , Jenes, B , Kim, TG , Cha, YS, et al. Expression of cholera toxin B subunit in transgenic rice endosperm. Mol Biotechnol. (2008) 40:261–8. doi: 10.1007/s12033-008-9083-2
277. Sharma, MK , Singh, NK , Jani, D , Sisodia, R , Thungapathra, M , Gautam, JK, et al. Expression of toxin co-regulated pilus subunit a (TCPA) of Vibrio cholerae and its immunogenic epitopes fused to cholera toxin B subunit in transgenic tomato (Solanum lycopersicum). Plant Cell Rep. (2008) 27:307–18. doi: 10.1007/s00299-007-0464-y
278. Tokuhara, D , Yuki, Y , Nochi, T , Kodama, T , Mejima, M , Kurokawa, S, et al. Secretory IgA-mediated protection against V. cholerae and heat-labile enterotoxin-producing enterotoxigenic Escherichia coli by rice-based vaccine. Proc Natl Acad Sci U S A. (2010) 107:8794–9. doi: 10.1073/pnas.0914121107
279. Nochi, T , Yuki, Y , Katakai, Y , Shibata, H , Tokuhara, D , Mejima, M, et al. A Rice-based Oral cholera vaccine induces macaque-specific systemic neutralizing antibodies but does Not influence pre-existing intestinal immunity. J Immunol. (2009) 183:6538–44. doi: 10.4049/jimmunol.0901480
280. Takeyama, N , Yuki, Y , Tokuhara, D , Oroku, K , Mejima, M , Kurokawa, S, et al. Oral rice-based vaccine induces passive and active immunity against enterotoxigenic E. coli-mediated diarrhea in pigs. Vaccine. (2015) 33:5204–11. doi: 10.1016/j.vaccine.2015.07.074
281. Tokuhara, D . Challenges in developing mucosal vaccines and antibodies against infectious diarrhea in children. Pediatr Int. (2018) 60:214–23. doi: 10.1111/ped.13497
282. Yuki, Y , Nojima, M , Hosono, O , Tanaka, H , Kimura, Y , Satoh, T, et al. Oral MucoRice-CTB vaccine for safety and microbiota-dependent immunogenicity in humans: a phase 1 randomised trial. Lancet Microbe. (2021) 2:e429–40. doi: 10.1016/S2666-5247(20)30196-8
283. Liang, W , Wang, S , Yu, F , Zhang, L , Qi, G , Liu, Y, et al. Construction and evaluation of a safe, live, oral Vibrio cholerae vaccine candidate, IEM108. Infect Immun. (2003) 71:5498–504. doi: 10.1128/IAI.71.10.5498-5504.2003
284. Liu, G , Yan, M , Liang, W , Qi, G , Liu, Y , Gao, S, et al. Resistance of the cholera vaccine candidate IEM108 against CTXΦ infection. Vaccine. (2006) 24:1749–55. doi: 10.1016/j.vaccine.2005.09.059
285. Ledón, T , Ferrán, B , Pérez, C , Suzarte, E , Vichi, J , Marrero, K, et al. TLP01, an mshA mutant of Vibrio cholerae O139 as vaccine candidate against cholera. Microbes Infect. (2012) 14:968–78. doi: 10.1016/j.micinf.2012.04.004
286. Ravichandran, M , Ali, SA , Rashid, NHA , Kurunathan, S , Yean, CY , Ting, LC, et al. Construction and evaluation of a O139 Vibrio cholerae vaccine candidate based on a hemA gene mutation. Vaccine. (2006) 24:3750–61. doi: 10.1016/j.vaccine.2005.07.016
287. O’Ryan, M , Vidal, R , del Canto, F , Salazar, JC , and Montero, D . Vaccines for viral and bacterial pathogens causing acute gastroenteritis: Part I: overview, vaccines for enteric viruses and Vibrio cholerae. Hum Vaccin Immunother. (2015) 11:584–600. doi: 10.1080/21645515.2015.1011019
288. Pastor, M , Pedraz, JL , and Esquisabel, A . The state-of-the-art of approved and under-development cholera vaccines. Vaccine. (2013) 31:4069–78. doi: 10.1016/j.vaccine.2013.06.096
289. Altindis, E , Fu, Y , and Mekalanos, JJ . Proteomic analysis of Vibrio cholerae outer membrane vesicles. Proc Natl Acad Sci U S A. (2014) 111:E1548–56. doi: 10.1073/pnas.1403683111
290. Leitner, DR , Lichtenegger, S , Temel, P , Zingl, FG , Ratzberger, D , Roier, S, et al. A combined vaccine approach against Vibrio cholerae and ETEC based on outer membrane vesicles. Front Microbiol. (2015) 6:823. doi: 10.3389/fmicb.2015.00823
291. Schild, S , Nelson, EJ , and Camilli, A . Immunization with Vibrio cholerae outer membrane vesicles induces protective immunity in mice. Infect Immun. (2008) 76:4554–63. doi: 10.1128/IAI.00532-08
292. Bishop, AL , Schild, S , Patimalla, B , Klein, B , and Camilli, A . Mucosal immunization with Vibrio cholerae outer membrane vesicles provides maternal protection mediated by antilipopolysaccharide antibodies that inhibit bacterial motility. Infect Immun. (2010) 78:4402–20. doi: 10.1128/IAI.00398-10
293. Wang, Z , Lazinski, DW , and Camilli, A . Immunity provided by an outer membrane vesicle cholera vaccine is due to O-antigenspecific antibodies inhibiting bacterial motility. Infect Immun. (2017) 85:e00626-16. doi: 10.1128/IAI.00626-16
294. Buonaguro, FM , and Buonaguro, L . Virus-like particles in vaccine development. Virus Like Part Vaccine Dev. (2014) 9:1–136. doi: 10.2217/9781780844176
295. Mohsen, MO , Zha, L , Cabral-Miranda, G , and Bachmann, MF . Major findings and recent advances in virus–like particle (VLP)-based vaccines. Semin Immunol. (2017) 34:123–32. doi: 10.1016/j.smim.2017.08.014
296. Rashidijahanabad, Z , Kelly, M , Kamruzzaman, M , Qadri, F , Bhuiyan, R , Mcfall-boegeman, H, et al. Virus-like particle display of Vibrio choleraeO-specific polysaccharide as a potential vaccine against cholera. ACS Infect Dis. (2022) 8:574–83. doi: 10.1021/acsinfecdis.1c00585
297. Soria-Guerra, RE , Moreno-Fierros, L , and Rosales-Mendoza, S . Two decades of plant-based candidate vaccines: a review of the chimeric protein approaches. Plant Cell Rep. (2011) 30:1367–82. doi: 10.1007/s00299-011-1065-3
298. Montero, DA , Del Canto, F , Salazar, JC , Céspedes, S , Cádiz, L , Arenas-Salinas, M, et al. Immunization of mice with chimeric antigens displaying selected epitopes confers protection against intestinal colonization and renal damage caused by Shiga toxin-producing Escherichia coli. NPJ Vaccines. (2020) 5:20. doi: 10.1038/s41541-020-0168-7
299. Price, GA , and Holmes, RK . Evaluation of TcpF-A2-CTB chimera and evidence of additive protective efficacy of immunizing with TcpF and CTB in the suckling mouse model of cholera. PLoS One. (2012) 7:e42434. doi: 10.1371/journal.pone.0042434
300. Price, GA , and Holmes, RK . Immunizing adult female mice with a TcpA-A2-CTB chimera provides a high level of protection for their pups in the infant mouse model of cholera. PLoS Negl Trop Dis. (2014) 8:e3356. doi: 10.1371/journal.pntd.0003356
301. Zareitaher, T , Sadat Ahmadi, T , and Latif Mousavi Gargari, S . Immunogenic efficacy of DNA and protein-based vaccine from a chimeric gene consisting OmpW, TcpA and CtxB, of Vibrio cholerae. Immunobiology. (2022) 227:152190. doi: 10.1016/j.imbio.2022.152190
302. Upadhyay, I , Li, S , Ptacek, G , Seo, H , Sack, DA , and Zhang, W . A polyvalent multiepitope protein cross-protects against Vibrio cholerae infection in rabbit colonization and passive protection models. Proc Natl Acad Sci. (2022) 119:2017. doi: 10.1073/pnas.2202938119
303. Sit, B , Fakoya, B , and Waldor, MK . Animal models for dissecting Vibrio cholerae intestinal pathogenesis and immunity. Curr Opin Microbiol. (2022) 65:1–7. doi: 10.1016/j.mib.2021.09.007
304. Raahati, Z , Bakhshi, B , and Najar-Peerayeh, S . Selenium nanoparticles induce potent protective immune responses against Vibrio cholerae WC vaccine in a mouse model. J Immunol Res. (2020) 2020:8874288. doi: 10.1155/2020/8874288
305. Albutti, A , Longet, S , McEntee, CP , Quinn, S , Liddicoat, A , Rîmniceanu, C, et al. Type ii nkt cell agonist, sulfatide, is an effective adjuvant for oral heat-killed cholera vaccines. Vaccine. (2021) 9:1–15. doi: 10.3390/vaccines9060619
306. Wang, ZB , and Xu, J . Better adjuvants for better vaccines: progress in adjuvant delivery systems, modifications, and adjuvant–antigen codelivery. Vaccine. (2020) 8:128. doi: 10.3390/vaccines8010128
307. Subiza, J , El-Qutob, D , and Fernandez-Caldas, E . New developments in oral vaccines and mucosal adjuvants. Recent Patents Inflamm Allergy Drug Discov. (2015) 9:4–15. doi: 10.2174/1872213x09666150211122313
308. Jazayeri, SD , Lim, HX , Shameli, K , Yeap, SK , and Poh, CL . Nano and microparticles as potential oral vaccine carriers and adjuvants against infectious diseases. Front Pharmacol. (2021) 12:682286. doi: 10.3389/fphar.2021.682286
309. Chen, W . Will the mRNA vaccine platform be the panacea for the development of vaccines against antimicrobial resistant (AMR) pathogens? Expert Rev Vaccines. (2022) 21:155–7. doi: 10.1080/14760584.2022.2011226
310. Mayer, RL , Verbeke, R , Asselman, C , Aernout, I , Gul, A , Eggermont, D, et al. Immunopeptidomics-based design of mRNA vaccine formulations against Listeria monocytogenes. Nat Commun. (2022) 13:6075. doi: 10.1038/s41467-022-33721-y
Keywords: Vibrio cholerae , cholera toxin, cholera, diarrhea, oral vaccine, next-generation vaccines
Citation: Montero DA, Vidal RM, Velasco J, George S, Lucero Y, Gómez LA, Carreño LJ, García-Betancourt R and O’Ryan M (2023) Vibrio cholerae, classification, pathogenesis, immune response, and trends in vaccine development. Front. Med. 10:1155751. doi: 10.3389/fmed.2023.1155751
Edited by:
Milad Badri, Qazvin University of Medical Sciences, IranReviewed by:
Farhad Nikkhahi, Qazvin University of Medical Sciences, IranAida Vafae Eslahi, Tarbiat Modares University, Iran
Copyright © 2023 Montero, Vidal, Velasco, George, Lucero, Gómez, Carreño, García-Betancourt and O’Ryan. This is an open-access article distributed under the terms of the Creative Commons Attribution License (CC BY). The use, distribution or reproduction in other forums is permitted, provided the original author(s) and the copyright owner(s) are credited and that the original publication in this journal is cited, in accordance with accepted academic practice. No use, distribution or reproduction is permitted which does not comply with these terms.
*Correspondence: Miguel O’Ryan, bW9yeWFuQHVjaGlsZS5jbA==
†These authors have contributed equally to this work and share first authorship