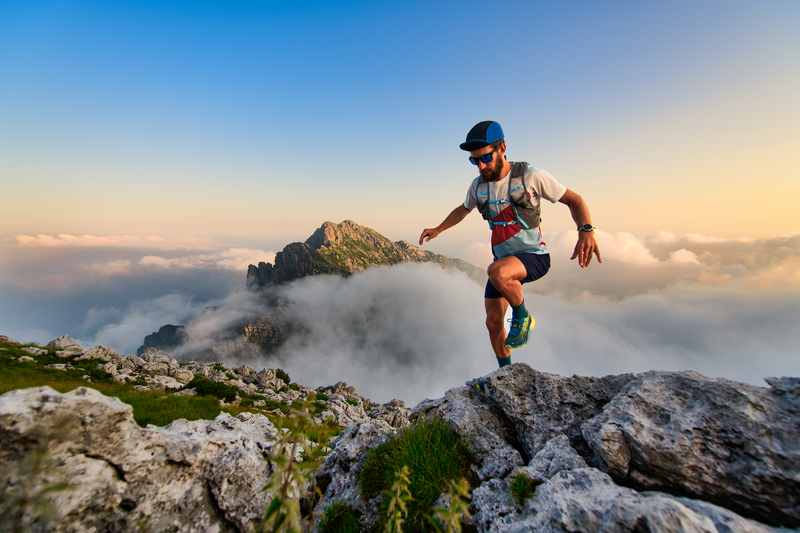
95% of researchers rate our articles as excellent or good
Learn more about the work of our research integrity team to safeguard the quality of each article we publish.
Find out more
REVIEW article
Front. Med. , 17 April 2023
Sec. Nephrology
Volume 10 - 2023 | https://doi.org/10.3389/fmed.2023.1132355
This article is part of the Research Topic Novel Insights and Prospects of Targeted Therapy in Kidney Diseases View all 8 articles
With the development of nanotechnology, nanoparticles have been used in various industries. In medicine, nanoparticles have been used in the diagnosis and treatment of diseases. The kidney is an important organ for waste excretion and maintaining the balance of the internal environment; it filters various metabolic wastes. Kidney dysfunction may result in the accumulation of excess water and various toxins in the body without being discharged, leading to complications and life-threatening conditions. Based on their physical and chemical properties, nanoparticles can enter cells and cross biological barriers to reach the kidneys and therefore, can be used in the diagnosis and treatment of chronic kidney disease (CKD). In the first search, we used the English terms “Renal Insufficiency, Chronic” [Mesh] as the subject word and terms such as “Chronic Renal Insufficiencies,” “Chronic Renal Insufficiency,” “Chronic Kidney Diseases,” “Kidney Disease, Chronic,” “Renal Disease, Chronic” as free words. In the second search, we used “Nanoparticles” [Mesh] as the subject word and “Nanocrystalline Materials,” “Materials, Nanocrystalline,” “Nanocrystals,” and others as free words. The relevant literature was searched and read. Moreover, we analyzed and summarized the application and mechanism of nanoparticles in the diagnosis of CKD, application of nanoparticles in the diagnosis and treatment of renal fibrosis and vascular calcification (VC), and their clinical application in patients undergoing dialysis. Specifically, we found that nanoparticles can detect CKD in the early stages in a variety of ways, such as via breath sensors that detect gases and biosensors that detect urine and can be used as a contrast agent to avoid kidney damage. In addition, nanoparticles can be used to treat and reverse renal fibrosis, as well as detect and treat VC in patients with early CKD. Simultaneously, nanoparticles can improve safety and convenience for patients undergoing dialysis. Finally, we summarize the current advantages and limitations of nanoparticles applied to CKD as well as their future prospects.
Many diseases originate from pathological changes at the molecular level, such as gene mutations, protein misfolding, and cell dysfunction, caused by microbial infections. These molecules and infectious agents are nanometers in size and may be located in biological systems protected by nanometer-sized barriers (1). Although this definition identifies nanoparticles with dimensions below 0.1 μm or 100 nm, particularly in the area of drug delivery, relatively large (>100 nm) nanoparticles may be required to load a sufficient amount of drug onto the particles (2). With the development of nanotechnology, nanomaterials have been designed as micro particles with different sizes, shapes, and chemical properties to help diagnose diseases and deliver drugs through biological barriers (3, 4). With a decrease in size, the surface area of nanocarriers increases with a higher particle surface energy, which could help them traverse different biological barriers (5, 6). These unique characteristics, which are not observed in macromolecules, enable the use of nanocarriers for novel applications.
Nanoparticles are mainly divided into inorganic-based nanoparticles, which can be designed in various sizes, structures, and geometries, and are ideal candidates for drug delivery and molecular imaging applications (7, 8). Based on their mechanical, electrical, thermal, and physicochemical properties and biological abilities, carbon-based nanoparticles have been widely explored for various applications, including bioimaging, biosensing, and drug delivery (9, 10). Lipid-based nanoparticles are outstanding drug carriers (11–13). Polymeric nanoparticles are divided into nanocapsules and nanospheres and are ideal drug delivery platforms with the ability to optimize therapeutic strategies for vascular aging-related disorders (14, 15). Finally, biomimetic nanoparticles are formed by integrating different biomaterials onto the surfaces of nanoparticles, which enables them to mimic the biological characteristics and roles of native cells (16). Nanoparticles with diameters ranging from 1 to 160 nm can target various parts of the kidney through various mechanisms. Among them, approximately 1-nm nanoparticles can be used to accumulate in the glycocalyx of the glomerular filtration barrier, whereas 80–90 nm nanoparticles can be used to target the mesangium. In addition, larger nanoparticles (>100 nm) can enter the kidney by secreting in peritubular capillaries into the proximal tubules (17–20). Drug delivery through nanoparticles can improve drug bioavailability and cycle half-life and achieve targeted drug delivery by binding specific ligands to the surface of nanoparticles (21). Generally, the treatment of kidney disease requires high drug concentrations, which may lead to adverse reactions and non-specific uptake in other parts of the body (20). For example, tolvaptan is approved for autosomal dominant polycystic kidney disease, which is reported to cause a variety of adverse reactions, including extreme thirst, polyuria, and drug-induced liver damage (22–24). Therefore, the development of kidney-targeting nanoparticles as a therapeutic strategy for kidney diseases has attracted great interest. After reviewing published literature on nanoparticles and patients with CKD, we discuss the application and development prospects of nanoparticles in CKD.
Irreversible loss of renal function ultimately makes patients rely on renal replacement therapy such as dialysis or kidney transplantation (25, 26). With the loss of kidney function, toxins cannot be discharged and eventually accumulate in the body (27, 28). According to some reports, hundreds of urine toxins accumulate in the human body (29). At present, renal function in patients with CKD is mainly evaluated based on blood creatinine and urea levels, but these are affected by age, sex, weight, diet, and other factors (30). The diagnosis and treatment of CKD are often delayed because the disease has no obvious symptoms (31). The plasma biomarkers of patients with CKD or some urotoxins in their metabolites are transferred to alveolar exhalation through lung exchange, and even in the late stages of the disease, the patient’s breath has a fishy smell (32). Haick et al. used a carbon nanotube-based sensor to distinguish between healthy and end-stage renal disease rats using breathing samples. This sensor, aimed at the early detection of patients with CKD, can distinguish 27 gases that appear in end-stage renal disease but not in healthy mice (33). In 2012, Marom et al. used a sensor based on organic functionalized gold nanoparticles combined with a support vector machine to analyze respiratory samples. The combination of the three gold nanoparticle sensors could effectively distinguish between early CKD and healthy states (accuracy: 79%) and the fourth and fifth CKD states (accuracy: 85%). This shows that the gold nanoparticle sensor can be used as a fast and reliable diagnostic instrument for the early detection and monitoring of CKD progression (31). Later, a device for measuring human breath ammonia was developed based on polyaniline nanoparticles and used to measure human respiratory ammonia, which could facilitate and lower the cost of monitoring patients with CKD (34). However, these sensors have not been widely used in clinical practice, which may be because their convenience and accuracy require improvement, and recent research on the application of respiratory sensors in CKD is relatively limited (Table 1).
Figure 1. Diagram of application of nanoparticles in auxiliary inspection. (A) polyaniline nanoparticles and Gold nanoparticles based sensors can detect harmful gases in the breath of CKD patients. (B) Polyaniline nanoparticles and Gold nanoparticles based biosensor can detect trace albumin in the urine of patients with CKD at an early stage. (C) Gold nanoparticles and magnetic nanoparticles can be used in CT or MRI imaging to reduce kidney damage caused by iodine or gadolinium contrast agents.
Urinary albumin level is a risk factor for CKD or CKD progression (52, 53). However, in recent years, many studies have suggested that urinary albumin excretion below the defined microalbuminuria range (i.e., in the normal-to-mildly increased albumin range) is an independent risk factor for renal disease (54, 55), and some studies have proposed the lowering of albuminuria levels that require intervention from 30 mg/24 h to 8–10 mg/24 h (56, 57). These studies have led to an increasing demand for rapid and easy-to-use screening tests to detect low albumin levels in the general population (35). Biosensors based on nanoparticles have the advantages of simple operation, high sensitivity, good stability, good specificity, fast response, and low analysis cost, among other advantages (58). Surface-enhanced Raman spectroscopy (SERS) is an emission technique using silver/copper/gold surfaces that involves the inelastic scattering of incident laser energy (59)and is highly sensitive, simple, and fast (60). Stefancu et al. developed a SERS-based screening method using iodide-modified silver nanoparticles for assessing albuminuria in the normal-to-mildly increased albuminuria range and showed an excellent correlation between predicted and reference albumin concentrations with an R2 and RMSEP of 0.98 and 2.82, respectively, which highlighted the potential of this strategy for absolute quantification of albuminuria (35). In a recent report on the development of an inexpensive and disposable immunosensor for sensitivity, the carbon working electrode was sequentially modified with electropolymerized polyaniline (PANI) and electrodeposited gold nanocrystals (AuNCs), and the resulting PANI/AuNC nanocomposite synergistically improved the immunosensor response. The PANI/AuNC nanocomposite can detect changes in HSA concentrations in the range of 3–300 μg/mL to screen for early CKD (36, 61). Shaikh et al. developed novel PS/Ag/ab-HSA nanoprobes (polystyrene nanoparticle core with silver nanoshells covalently conjugated to HSA antibodies) and a portable immunosensor for the early detection of CKD (37) (Figure 1).
In general, the use of nanotechnology for urine detection has enabled the development of a new method to detect CKD at an early stage, leading to more efficient treatment monitoring aimed at slowing or even stopping the evolution of macroalbuminuria. Currently, new nanosensors to detect the level of early urinary albumin more sensitively and conveniently, helping to diagnose CKD in the early clinical stages, are still being developed.
Magnetic nanoparticles were developed as angiography agents for molecular imaging in the 1990s (62). The magnetic particle imaging signal is only generated by magnetic nanoparticles, and diamagnetic tissue generates a zero background signal to produce better vascular contrast (63, 64). Iodine and gadolinium contrast agents for X-ray imaging and magnetic resonance imaging can further lead to renal fibrosis, and nephrogenic systemic fibrosis is recognized as a serious complication related to gadolinium contrast agents; therefore, the use of these examinations in patients is restricted (65–67). Unlike other contrast agents, iron is an essential element for the human body; therefore, a good choice for patients with CKD is to use ultra-small superparamagnetic iron oxide nanoparticles, such as ferumoxytol, as contrast agents (68). Although feumoxytol nanoparticles have an ultra-small core, their large carbohydrate-coated shell prevents renal filtration, and they are removed from the circulatory system through phagocytosis by macrophages. The remaining iron oxide particles are absorbed by the reticuloendothelial system of the liver, spleen, and bone marrow and are incorporated into iron storage in the body for red blood cell synthesis, thus prolonging the stay time in blood vessels and allowing for a more lasting contrast (64, 69–72). Therefore, it is relatively safe for patients with renal failure (73) and can be considered for patients with a glomerular filtration rate ≤ 30 mL/min/1.7 (74). Some studies have shown that ferumoxytol can also be used as a contrast agent for clear imaging in children who have undergone dialysis, and the imaging results are consistent with the real anatomy, with good diagnostic effects and no adverse events; thus, it is expected to replace other contrast agents and be widely used in patients with CKD (75, 76).
Yu et al. recently used renal-clearable near-infrared-emitting glutathione-coated gold nanoparticles (GS-AuNPs) as contrast agents in fluorescence imaging to evaluate and determine the stage of renal dysfunction, which is consistent with renal injury evaluated by pathological results (77). Previously, they applied GS-AuNPs to the fluorescence imaging of renal clearance kinetics in normal mice to prove that they are non-toxic and do not affect metabolism in vivo (78). The hydrodynamic diameter of GS-AuNPs (3.3 ± 0.4 nm) is below the renal filtration threshold (6–8 nm), so they can be effectively excreted through the kidneys (79). At present, many nanoparticles can be filtered by the kidney, including gold nanoparticles, copper nanoparticles (80), iron oxide nanoparticles (81), silicon dioxide nanoparticles (82), carbon dots (83), and palladium nanoparticles (84), making the application of nanoparticles in non-invasive renal imaging possible.
Vascular calcification (VC) is a risk factor for cardiovascular events and death in patients with CKD (85, 86). VC formation is caused by multiple factors, such as the proliferation, differentiation, and apoptosis of vascular smooth muscle cells (VSMC), oxidative stress, endothelial dysfunction, increased remodeling of the extracellular matrix, release of calcified extracellular vesicles, loss of mineralization inhibitors, and chronic inflammation (87). High P content can induce the formation of Ca/P-related nanocrystals in the cell matrix. The presence of these crystals leads to an increased expression of osteoblast bone morphogenetic protein 2 (BMP-2) and osteopontin. Among these, Ca/P crystals with diameters of 1–2 nm or less are swallowed by VSMCs, causing toxic effects. The dissolution of crystals in the lysosomes leads to the release of calcium ions into the cytoplasm, resulting in apoptosis (88). Nanocrystalline Ca/P can affect a variety of cell functions, including proliferation, activation of the mitogen-activated white kinase signaling cascade, activation of matrix metalloproteinases, induction of proinflammatory cytokines, and stimulation of endocytosis (89–91). Therefore, the presence of nanocrystalline Ca/P may lead to a pathological positive feedback loop, resulting in increased cell death, inflammation, phenotypic changes, matrix degradation, and calcification (92, 93). Schlieper et al. collected iliac artery segments from 30 patients undergoing dialysis before kidney transplantation and showed that many calcifications consisted of 2–10 nm nanocrystals with a hydroxyapatite and whitlockite crystalline structure and a mineral phase (94). This confirms that the VC may have originated from nanocrystals formed by mineral deposition.
Calcium phosphate overloading in the human body induces CKD (95, 96). Increased phosphate excretion per nephron increases phosphate concentration in renal tubular fluid and triggers CaPi crystal precipitation (97). The CaPi precipitate may combine with fetuin-A to form calcium protein granules (CPP). CPP deposits in the proximal renal tubule epithelium and induces the destruction of lysosome homeostasis, autophagic flux, and plasma membrane integrity, resulting in a vicious cycle and finally leading to progressive nephron loss (98). Pasca et al. described a nanoparticle-based assay that, in the presence of artificially elevated calcium and phosphate concentrations, detects the spontaneous transformation of spherical colloidal primary calciprotein particles to elongate crystalline secondary CPPs. Using this assay, we found that both the sera of mice deficient in fetuin-A, a serum protein that inhibits calcification, and the sera of patients on hemodialysis had reduced intrinsic properties to inhibit calcification (99). Karamched et al. developed elastin antibody-conjugated albumin nanoparticles that target only degraded elastin in the vasculature while sparing healthy tissues. Targeted nanoparticle-based ethylenediaminetetraacetic acid chelation therapy to reverse CKD-associated Medial arterial calcification (MAC) and targeted nanoparticle therapy may provide an attractive option to reverse calcification and have a high potential for clinical translation (38) (Figure 2).
Figure 2. Diagram of application of nanoparticles in various comorbidities caused by chronic kidney disease. D: Deposition of CA/ Pi-related nanocrystalline compounds in the vascular wall leads to vascular calcification. E: Elastin antibodies to albumin nanoparticles can reverse CKD—associated MAC. F: Emodin-NP regulates gut microbiome and delays the progression of CKD. G: Plasmid DNA of BMP7 or HGF/NK1 encapsulated in chitosan nanoparticles coated with hyaluronic acid reversed fibrosis progression of the cell and regenerative tubules. H: Ferumoxytol is a novel, semi-synthetic, carb-coated, superparamagnetic iron oxide nanoparticle that can be administered intravenously for iron supplementation. I: Ag nanoparticles modified dialysis membranes to reduce thrombosis. J: A biosensor based on a gold nanoparticle (AuNP) modified Pt electrode can measure relative amino acid loss in patients undergoing renal replacement therapy. K: Oxidized starch nanoparticles can adsorb and remove 95% urea in dialysate.
The VC material of CKD has been confirmed to exist in the form of nanoparticles, and experimental research has shown that albumin nanoparticles with elastin antibodies have the potential to reverse vascular calcification, which is important for clinical applications. However, at present, there are few results and insufficient evidence. Thus, the application of nanotechnology in the diagnosis and treatment of VC in patients with CKD requires more basic research to confirm its utility. Thus, this is a promising direction for future research.
Fibrosis is an adverse repair reaction of extracellular matrix deposition after tissue damage that may eventually lead to organ dysfunction (100, 101). Progressive fibrosis is a potential pathophysiological process of CKD (102), which is closely related to inflammation (103, 104), secondary hyperparathyroidism (105), FGF23 (106, 107), diabetes (108), vitamin D deficiency (109), and other factors. Determining the degree of renal fibrosis is conducive to monitoring disease progression and management. Currently, renal biopsy is the reference standard for evaluating fibrosis; however, its application is limited owing to its invasivity (110). There is a great need to develop non-invasive imaging methods for the evaluation of renal fibrosis, and magnetic resonance (MR) with or without gadolinium enhancement shows great potential (111). However, gadolinium or iodine administration for MR and computed tomography (CT) angiography is not applicable in all cases and introduces the risk of contrast agent nephropathy (112). Therefore, researchers have begun to explore the application of nanoparticles in the diagnosis of renal fibrosis. Shirai et al. developed silica nanoparticles with fluorescent-labeled anti-CD11b as an imaging tool to efficiently evaluate inflammation and fibrosis in an animal model of unilateral ureteral obstruction (39). In addition, based on the fact that collagen-I is one of the main components of pathological fibrosis, selectively directing gold nanoparticles (AuNP) to collagen-I may help identify damaged kidneys (113). Zhu et al. (40) combined an anti-collagen-I antibody to the surface of AuNPs and injected them into mouse arteries. After 4 h of CT evaluation, the retention of Co-I-AuNPs in the kidney on the side of fibrosis increased. These experiments showed that nanotechnology can be used to detect renal function and study pathologies in the future.
In addition, it is important to develop treatment methods for CKD to prevent or reverse the fibrogenic cell phenotypes. Nanoparticles can provide new methods of anti-fibrosis therapy for damaged tissues and resident cells by limiting the expression of pro-fibrotic phenotypes.
The size and surface properties of polymer nanoparticles can be adjusted to help deliver drugs to specific sites; for example, loading dexamethasone nanoparticles into selected biocompatible nanoparticles successfully repaired damaged podocytes (114). They can be used to modify drugs to achieve improved stability, durability, and therapeutic effects. For example, emodin, a traditional Chinese medicine, can regulate intestinal microbiota and delay CKD progress (115, 116). However, poor solubility, limited colonic irrigation retention time, and inadequate colon adhesion hinder the clinical application of emodin. Lu et al. (42) prepared monomethoxy-poly (ethylene glycol)-poly (lactic acid)-chitosan-2-mercaptobenzimidazole nanoparticles that incorporated emodin (emodin-NPs). Emodin-NP has higher stability and better colonic adhesion and durability than emodin alone, and can alleviate kidney dysfunction and tubulointerstitial fibrosis by modifying gut microbiota disorders. In addition, nanoparticles can be used to decorate small molecules and deliver them to specific cells to play an anti-fibrotic role. For example, plasmid DNA expressing bone morphogenetic protein 7 or hepatocyte growth factor (HGF)-NK1 (HGF/NK1) was encapsulated in nanoparticles to safely administer multifunctional nanoparticles containing plasmid DNA to the kidneys for localized and sustained expression of antifibrotic factors (41). In addition, nanoparticles can improve the water solubility and bioavailability of molecular substances. Farnesyl thiosalicylic acid (FTS) is a specific inhibitor of the proto-oncogene Ras. It has been reported that FTS can inhibit Transforming growth factor (TGF) by inhibiting the EMT process-β 1 induced activation of NRK-49F in renal fibroblasts and may have therapeutic potential in treating renal fibrosis (117–120). However, its application is hindered by its water insolubility and low bioavailability. Huang et al. encapsulated FTS in bovine serum albumin nanoparticles (AN-FTS) to improve its characteristics. Mouse experiments have demonstrated that AN-FTS preferentially accumulates in the fibrotic kidney, which can significantly reduce renal fibrosis and inflammation compared to free drugs (43).
In general, nanotechnology can not only evaluate renal fibrosis in combination with CT, but also treat renal fibrosis owing to its advantages of strong plasticity, targeting, and stability. It can improve the bioavailability of drugs and reduce their adverse effects. Therefore, the application of nanoparticles has opened a new chapter in the treatment of CKD and has enormous potential for the treatment of renal fibrosis.
Oral or intravenous iron drops are primarily used to treat renal anemia. Ferumoxytol is a new, semisynthetic, carbohydrate-coated, superparamagnetic iron oxide nanoparticle that can be administered intravenously. It produces the least dialyzable free iron and can release free iron more stably than the other iron products. Therefore, its bioavailability is better than that of oral administration, and its tolerance is better, with fewer side effects (44, 70, 121). Many randomized controlled trials and cohort studies have shown that it is more effective than oral iron in improving anemia and can increase transferrin saturation (TSAT) and ferritin levels. The probability of most adverse events is similar to that of other oral iron (122–128). A higher risk of hypotension may be associated with fewer adverse gastrointestinal events (129). However, Ferumoxytol received a black box warning from the FDA in March 2015, reminding doctors to pay attention to the possibility of hypersensitivity reactions, including allergic reactions. Therefore, it is necessary to reduce the infusion rate.
Renal failure was defined as a glomerular filtration rate of <15 mL/min/1.73 m2 (130). Dialysis is one of the main treatment methods for patients with renal failure (131), and the number of dialysis patients has gradually increased over the last 20 years (132). Although dialysis treatment has become increasingly developed, the mortality rate of patients undergoing dialysis remains very high (133). Various complications may arise as a result of dialysis, such as cardiovascular events, catheter-related infections, peritonitis, among others (134–136). In addition, dialysis may cause great inconvenience to patients, especially those undergoing hemodialysis, because a dialysis frequency of three times/week may result in patients risking unemployment to attend dialysis appointments (137). The increase in economic burden causes the disease burden on patients to rise continuously (138); thus, our goal is to make dialysis technology safer, more efficient, and more convenient.
Nanotechnology can be used as a new method for treating arteriovenous fistulas. Ferumoxytol is an iron-oxide nanoparticle that can be used as a substitute for MRI contrast agents. Ferumoxytol can identify the condition of the central artery, better identify peripheral artery diseases, and better predict the results of arteriovenous fistula surgery (45).
One of the main obstacles to blood filtration is blockage caused by thrombosis, which limits the maximum filter life during hemodialysis and continuous renal replacement therapy (46). Dialysis membrane pores must be sufficiently small to prevent protein loss from plasma, and membrane surfaces should be carefully designed to provide high membrane blood compatibility and minimal thrombosis (47). Engineering changes in membrane surface chemistry and structure by doping with nanomaterials has attracted extensive attention. Ag nanoparticles and multiwalled carbon nanotubes (MWNT) may be the most important nanomaterials with blood compatibility and low toxicity to blood cells (47). For example, a gold nanoparticle-modified artificial kidney, which expresses a negatively charged surface, reduced some acute adverse effects caused by dialysis-induced protein adsorption, platelet adhesion, and coagulation activation, thus avoiding thrombosis during hemodialysis (48). In addition, an AuNP-modified Pt electrode was used to measure the relative loss of amino acids in patients undergoing renal replacement therapy by analyzing the amino acid levels in diluted serum samples before and after entering/leaving the hemodialysis apparatus (49).
Nanomaterials have the advantage of enhanced accumulation in the target area and can be used in peritoneal dialysis treatment. Zheng et al. (50) developed vitamin D-loaded magnetic nanoparticles (MNPs), Vitamin D-loaded MNPs targeted the peritoneum better than vitamin D3 and had the same therapeutic effect as vitamin D3 in ameliorating peritoneal fibrosis and functional deterioration in a peritoneal dialysis animal model. In addition, nanomaterials as adsorbents are expected to facilitate portable dialysis. A nano-adsorbent derived from corn starch (oxy-SNPs) for urea removal and adsorption achieved equilibrium after 4 h, with 95% removal (139). Thus, oxy-SNPs are promising adsorbents for dialysate regeneration and urea removal. In addition, cationic cellulose nanocrystals are also used as phosphate binders to treat patients with hyperphosphatemia, and their phosphate binding rate is higher than that of commonly used phosphate binders, such as calcium carbonate (51).
In general, nanomaterials can be used in various fields for patients undergoing dialysis, such as optimizing dialysis effects, reducing adverse reactions to dialysis, evaluating dialysis conditions, and treating dialysis-related complications. However, most of these studies have been limited to mouse models.
However, despite our increased exposure to nanoparticles, information regarding nanoparticle safety lags behind research on the application of nanoparticles (140). The toxic effects of nanoparticles on tissues and organs should not be ignored. In nanomedicine, a large amount of drugs needs to accumulate in target cells to achieve a therapeutic effect (141), and through the blood circulation, nanoparticles can be distributed and can accumulate in different organs such as the liver, spleen, lungs, and kidneys. Some studies suggest that nanoparticles may also accumulate in the brain if they are small enough (<10 nm) and/or the blood–brain barrier is not intact (142, 143). The toxicity of nanoparticles is dependent on their biophysical properties, including size, surface area, surface charge, and aggregation state (144). These properties have been shown to affect their distribution and deposition in different organ systems (145). Solid nanoparticles such as metal-containing or metal oxide nanoparticles have reportedly caused oxidative stress, inflammation, and DNA damage, and they have been shown to induce oxidative stress in the liver, spleen, and kidneys and inhibit the effects of antioxidants (146–148). At present, most studies evaluating the toxicity of nanoparticles are short-term exposure studies, and few studies have evaluated the toxic effects of nanoparticles after chronic exposure (149). In addition, the pharmacokinetics of nanoparticles as drug delivery systems are not well studied, and most studies have focused only on the pharmacokinetics of encapsulated drugs as opposed to the drug carriers themselves (150, 151).
Although attempts have been made to modify nanoparticle surface properties using inaccessible coating materials to reduce their potential toxicity, this may alter the cellular uptake of nanoparticles and their efficacy as a drug delivery system (152, 153). Therefore, the toxic effects of nanoparticles limit their clinical application (154), and approximately 20% of the nanoparticles rejected during clinical trials are for safety reasons (149). In the future, a complete toxicological analysis of nanoparticles and the development of structure–activity relationships will help determine the key physical or chemical characteristics of nanoparticles that cause their toxicity. By optimizing their physical and chemical characteristics, the toxicity of nanoparticles can be minimized and their biological effects can be maximized (155, 156).
The emergence of nanoparticles has brought hope to medicine. Regarding kidney disease, early potential patients and disease progression can be identified using gas measurements and urine detection. Magnetic nanoparticles can reduce the renal fibrosis caused by iodine or gadolinium contrast agents in patients with CKD. Gold nanoparticles and silica nanoparticles are also used for renal imaging to evaluate renal inflammation and fibrosis, and may become a tool to replace renal biopsy puncture in the future. CKD is associated with vascular and renal fibrosis. Albumin nanoparticles can target degraded elastin to reverse vascular calcification. While polymer nanoparticles can package and deliver related drugs to specific parts of the kidney to improve their bioavailability and half-life and effectively treat renal fibrosis, superparamagnetic iron oxide nanoparticles can be administered intravenously to treat renal anemia and appear to be more effective than traditional oral iron agents. In addition, during dialysis treatment, the use of gold nanoparticles can improve the chemical structure of the dialysis membrane, thus reducing the adverse reactions of thrombosis and detecting the loss of amino acids in patients after dialysis. In summary, many types of nanoparticles have been used for the diagnosis and treatment of patients with CKD; however, most trials are still in the preclinical stage, and nanoparticles have not been routinely used in clinical practice. We believe that the application of nanoparticles to patients with CKD is clinically meaningful, as it may allow for more accurate and effective diagnosis and treatment of CKD in the future. Therefore, more clinical experiments on nanoparticles should be conducted so that nanotechnology can be more widely and effectively utilized in clinical settings.
KY drafted and revised the manuscript. YS drafted and revised the manuscript and designed the tables/figures. SP and NY helped collect data. JJ drafted and revised the manuscript. QH initiated the collaboration, directed, drafted, and revised the manuscript.
This research was supported by the Huadong Medicine Joint Funds of the Zhejiang Provincial Natural Science Foundation of China (grant no. LHDMZ22H050001), Construction of Key Projects by the Zhejiang Provincial Ministry (project no.WKJ-ZJ-2302), Zhejiang Province Chinese Medicine Modernization Program (project no. 2020ZX001), Key Project of Scientific Research Foundation of Chinese Medicine (2022ZZ002), Key Project of Zhejiang Science and Technology Department (2022C03118), and Key Project of Basic Scientific Research Operating Funds of Hangzhou Medical College (KYZD202002).
The authors would like to thank all the people who participated in this work.
The authors declare that the research was conducted in the absence of any commercial or financial relationships that could be construed as a potential conflict of interest.
All claims expressed in this article are solely those of the authors and do not necessarily represent those of their affiliated organizations, or those of the publisher, the editors and the reviewers. Any product that may be evaluated in this article, or claim that may be made by its manufacturer, is not guaranteed or endorsed by the publisher.
1. Kim, BY, Rutka, JT, and Chan, WC. Nanomedicine. N Engl J Med. (2010) 363:2434–43. doi: 10.1056/NEJMra0912273
2. De Jong, WH, and Borm, PJ. Drug delivery and nanoparticles:applications and hazards. Int J Nanomedicine. (2008) 3:133–49. doi: 10.2147/ijn.s596
3. Peer, D, Karp, JM, Hong, S, Farokhzad, OC, Margalit, R, and Langer, R. Nanocarriers as an emerging platform for cancer therapy. Nat Nanotechnol. (2007) 2:751–60. doi: 10.1038/nnano.2007.387
4. Xia, Y, Xiong, Y, Lim, B, and Skrabalak, SE. Shape-controlled synthesis of metal nanocrystals: simple chemistry meets complex physics? Angew Chem Int Ed Engl. (2009) 48:60–103. doi: 10.1002/anie.200802248
5. Sacks, D, Baxter, B, Campbell, BCV, Carpenter, JS, Cognard, C, Dippel, D, et al. Multisociety consensus quality improvement revised consensus statement for endovascular therapy of acute ischemic stroke. Int J Stroke. (2018) 13:612–32. doi: 10.1177/1747493018778713
6. Sood, S, Maddiboyina, B, Rawat, P, Garg, AK, Foudah, AI, Alam, A, et al. Enhancing the solubility of nitazoxanide with solid dispersions technique: formulation, evaluation, and cytotoxicity study. J Biomater Sci Polym Ed. (2021) 32:477–87. doi: 10.1080/09205063.2020.1844506
7. Wu, K, Su, D, Liu, J, Saha, R, and Wang, JP. Magnetic nanoparticles in nanomedicine: a review of recent advances. Nanotechnology. (2019) 30:502003. doi: 10.1088/1361-6528/ab4241
8. Vangijzegem, T, Stanicki, D, and Laurent, S. Magnetic iron oxide nanoparticles for drug delivery: applications and characteristics. Expert Opin Drug Deliv. (2019) 16:69–78. doi: 10.1080/17425247.2019.1554647
9. Gupta, TK, Budarapu, PR, Chappidi, SR, Paggi, M, and Bordas, SP. Advances in carbon based Nanomaterials for bio-medical applications. Curr Med Chem. (2019) 26:6851–77. doi: 10.2174/0929867326666181126113605
10. Saleem, J, Wang, L, and Chen, C. Carbon-based Nanomaterials for cancer therapy via targeting tumor microenvironment. Adv Healthc Mater. (2018) 7:e1800525. doi: 10.1002/adhm.201800525
11. Rao, S, Tan, A, Thomas, N, and Prestidge, CA. Perspective and potential of oral lipid-based delivery to optimize pharmacological therapies against cardiovascular diseases. J Control Release. (2014) 193:174–87. doi: 10.1016/j.jconrel.2014.05.013
12. Yonezawa, S, Koide, H, and Asai, T. Recent advances in siRNA delivery mediated by lipid-based nanoparticles. Adv Drug Deliv Rev. (2020) 154-155:64–78. doi: 10.1016/j.addr.2020.07.022
13. Tang, WL, Tang, WH, and Li, SD. Cancer theranostic applications of lipid-based nanoparticles. Drug Discov Today. (2018) 23:1159–66. doi: 10.1016/j.drudis.2018.04.007
14. Skourtis, D, Stavroulaki, D, Athanasiou, V, Fragouli, PG, and Iatrou, H. Nanostructured polymeric, liposomal and other materials to control the drug delivery for cardiovascular diseases. Pharmaceutics. (2020) 12:1160. doi: 10.3390/pharmaceutics12121160
15. Mitchell, MJ, Billingsley, MM, Haley, RM, Wechsler, ME, Peppas, NA, and Langer, R. Engineering precision nanoparticles for drug delivery. Nat Rev Drug Discov. (2021) 20:101–24. doi: 10.1038/s41573-020-0090-8
16. Beh, CY, Prajnamitra, RP, Chen, LL, and Hsieh, PC. Advances in biomimetic nanoparticles for targeted cancer therapy and diagnosis. Molecules. (2021) 26:5052. doi: 10.3390/molecules26165052
17. Choi, CH, Zuckerman, JE, Webster, P, and Davis, ME. Targeting kidney mesangium by nanoparticles of defined size. Proc Natl Acad Sci U S A. (2011) 108:6656–61. doi: 10.1073/pnas.1103573108
18. Singh, G, Ddungu, JLZ, Licciardello, N, Bergmann, R, De Cola, L, and Stephan, H. Ultrasmall silicon nanoparticles as a promising platform for multimodal imaging. Faraday Discuss. (2020) 222:362–83. doi: 10.1039/c9fd00091g
19. Yu, M, Zhou, C, Liu, L, Zhang, S, Sun, S, Hankins, JD, et al. Interactions of renal-clearable gold nanoparticles with tumor microenvironments: vasculature and acidity effects. Angew Chem Int Ed Engl. (2017) 56:4314–9. doi: 10.1002/anie.201612647
20. Huang, Y, Wang, J, Jiang, K, and Chung, EJ. Improving kidney targeting: the influence of nanoparticle physicochemical properties on kidney interactions. J Control Release. (2021) 334:127–37. doi: 10.1016/j.jconrel.2021.04.016
21. Gelperina, S, Kisich, K, Iseman, MD, and Heifets, L. The potential advantages of nanoparticle drug delivery systems in chemotherapy of tuberculosis. Am J Respir Crit Care Med. (2005) 172:1487–90. doi: 10.1164/rccm.200504-613PP
22. Wang, A, Hirose, T, Ohsaki, Y, Takahashi, C, Sato, E, Oba-Yabana, I, et al. Hydrochlorothiazide ameliorates polyuria caused by tolvaptan treatment of polycystic kidney disease in PCK rats. Clin Exp Nephrol. (2019) 23:455–64. doi: 10.1007/s10157-018-1669-9
23. Torres, VE, Chapman, AB, Devuyst, O, Gansevoort, RT, Grantham, JJ, Higashihara, E, et al. Tolvaptan in patients with autosomal dominant polycystic kidney disease. N Engl J Med. (2012) 367:2407–18. doi: 10.1056/NEJMoa1205511
24. Cárdenas, A, Ginès, P, Marotta, P, Czerwiec, F, Oyuang, J, Guevara, M, et al. Tolvaptan, an oral vasopressin antagonist, in the treatment of hyponatremia in cirrhosis. J Hepatol. (2012) 56:571–8. doi: 10.1016/j.jhep.2011.08.020
25. Levey, AS, Eckardt, KU, Tsukamoto, Y, Levin, A, Coresh, J, Rossert, J, et al. Definition and classification of chronic kidney disease: a position statement from kidney disease: improving global outcomes (KDIGO). Kidney Int. (2005) 67:2089–100. doi: 10.1111/j.1523-1755.2005.00365.x
26. Hirsch, S. Preventing end-stage renal disease: flexible strategies to overcome obstacles. Curr Opin Nephrol Hypertens. (2006) 15:473–80. doi: 10.1097/01.mnh.0000242171.29329.1d
27. Vanholder, R, Baurmeister, U, Brunet, P, Cohen, G, Glorieux, G, and Jankowski, J. A bench to bedside view of uremic toxins. J Am Soc Nephrol. (2008) 19:863–70. doi: 10.1681/asn.2007121377
28. Jourde-Chiche, N, Dou, L, Cerini, C, Dignat-George, F, Vanholder, R, and Brunet, P. Protein-bound toxins--update 2009. Semin Dial. (2009) 22:334–9. doi: 10.1111/j.1525-139X.2009.00576.x
29. Duranton, F, Cohen, G, de Smet, R, Rodriguez, M, Jankowski, J, Vanholder, R, et al. Normal and pathologic concentrations of uremic toxins. J Am Soc Nephrol. (2012) 23:1258–70. doi: 10.1681/asn.2011121175
30. Devarajan, P. Review: neutrophil gelatinase-associated lipocalin: a troponin-like biomarker for human acute kidney injury. Nephrology. (2010) 15:419–28. doi: 10.1111/j.1440-1797.2010.01317.x
31. Marom, O, Nakhoul, F, Tisch, U, Shiban, A, Abassi, Z, and Haick, H. Gold nanoparticle sensors for detecting chronic kidney disease and disease progression. Nanomedicine. (2012) 7:639–50. doi: 10.2217/nnm.11.135
32. Moorhead, KT, Hill, JV, Chase, JG, Hann, CE, Scotter, JM, Storer, MK, et al. Modelling acute renal failure using blood and breath biomarkers in rats. Comput Methods Prog Biomed. (2011) 101:173–82. doi: 10.1016/j.cmpb.2010.07.010
33. Haick, H, Hakim, M, Patrascu, M, Levenberg, C, Shehada, N, Nakhoul, F, et al. Sniffing chronic renal failure in rat model by an array of random networks of single-walled carbon nanotubes. ACS Nano. (2009) 3:1258–66. doi: 10.1021/nn9001775
34. Hibbard, T, Crowley, K, Kelly, F, Ward, F, Holian, J, Watson, A, et al. Point of care monitoring of hemodialysis patients with a breath ammonia measurement device based on printed polyaniline nanoparticle sensors. Anal Chem. (2013) 85:12158–65. doi: 10.1021/ac403472d
35. Stefancu, A, Moisoiu, V, Bocsa, C, Bálint, Z, Cosma, DT, Veresiu, IA, et al. SERS-based quantification of albuminuria in the normal-to-mildly increased range. Analyst. (2018) 143:5372–9. doi: 10.1039/c8an01072b
36. Shaikh, MO, Srikanth, B, Zhu, PY, and Chuang, CH. Impedimetric Immunosensor utilizing Polyaniline/gold Nanocomposite-modified screen-printed electrodes for early detection of chronic kidney disease. Sensors. (2019) 19. doi: 10.3390/s19183990
37. Shaikh, MO, Zhu, PY, Wang, CC, Du, YC, and Chuang, CH. Electrochemical immunosensor utilizing electrodeposited au nanocrystals and dielectrophoretically trapped PS/Ag/ab-HSA nanoprobes for detection of microalbuminuria at point of care. Biosens Bioelectron. (2019) 126:572–80. doi: 10.1016/j.bios.2018.11.035
38. Karamched, SR, Nosoudi, N, Moreland, HE, Chowdhury, A, and Vyavahare, NR. Site-specific chelation therapy with EDTA-loaded albumin nanoparticles reverses arterial calcification in a rat model of chronic kidney disease. Sci Rep. (2019) 9:2629. doi: 10.1038/s41598-019-39639-887
39. Shirai, T, Kohara, H, and Tabata, Y. Inflammation imaging by silica nanoparticles with antibodies orientedly immobilized. J Drug Target. (2012) 20:535–43. doi: 10.3109/1061186x.2012.693500
40. Zhu, XY, Zou, X, Mukherjee, R, Yu, Z, Ferguson, CM, Zhou, W, et al. Targeted imaging of renal fibrosis using antibody-conjugated gold nanoparticles in renal artery stenosis. Investig Radiol. (2018) 53:623–8. doi: 10.1097/rli.0000000000000476
41. Midgley, AC, Wei, Y, Zhu, D, Gao, F, Yan, H, Khalique, A, et al. Multifunctional natural polymer nanoparticles as Antifibrotic gene carriers for CKD therapy. J Am Soc Nephrol. (2020) 31:2292–311. doi: 10.1681/asn.2019111160
42. Lu, Z, Ji, C, Luo, X, Lan, Y, Han, L, Chen, Y, et al. Nanoparticle-mediated delivery of Emodin via colonic irrigation attenuates renal injury in 5/6 Nephrectomized rats. Front Pharmacol. (2020) 11:606227. doi: 10.3389/fphar.2020.606227
43. Huang, H, Liu, Q, Zhang, T, Zhang, J, Zhou, J, Jing, X, et al. Farnesylthiosalicylic acid-loaded albumin nanoparticle alleviates renal fibrosis by inhibiting Ras/Raf1/p38 signaling pathway. Int J Nanomedicine. (2021) 16:6441–53. doi: 10.2147/ijn.S318124
44. Singh, A, Patel, T, Hertel, J, Bernardo, M, Kausz, A, and Brenner, L. Safety of ferumoxytol in patients with anemia and CKD. Am J Kidney Dis. (2008) 52:907–15. doi: 10.1053/j.ajkd.2008.08.001
45. Stoumpos, S, Tan, A, Hall Barrientos, P, Stevenson, K, Thomson, PC, Kasthuri, R, et al. Ferumoxytol MR angiography versus duplex US for vascular mapping before Arteriovenous fistula surgery for hemodialysis. Radiology. (2020) 297:214–22. doi: 10.1148/radiol.2020200069
46. Dukhin, SS, Tabani, Y, Lai, R, Labib, OA, Zydney, AL, and Labib, ME. Outside-in hemofiltration for prolonged operation without clogging. J Membr Sci. (2014) 464:173–8. doi: 10.1016/j.memsci.2014.01.069
47. Zare-Zardini, H, Amiri, A, Shanbedi, M, Taheri-Kafrani, A, Kazi, SN, Chew, BT, et al. In vitro and in vivo study of hazardous effects of Ag nanoparticles and arginine-treated multi walled carbon nanotubes on blood cells: application in hemodialysis membranes. J Biomed Mater Res A. (2015) 103:2959–65. doi: 10.1002/jbm.a.35425
48. Chen, HC, Cheng, CY, Lin, HC, Chen, HH, Chen, CH, Yang, CP, et al. Multifunctions of excited gold nanoparticles decorated artificial kidney with efficient hemodialysis and therapeutic potential. ACS Appl Mater Interfaces. (2016) 8:19691–700. doi: 10.1021/acsami.6b05905
49. Miškinis, J, Ramonas, E, Gurevičienė, V, Razumienė, J, Dagys, M, and Ratautas, D. Capacitance-based biosensor for the measurement of Total loss of L-amino acids in human serum during hemodialysis. ACS Sensors. (2022) 7:3352–9. doi: 10.1021/acssensors.2c01342
50. Cheng, FY, Chiou, YY, Hung, SY, Lin, TM, Wang, HK, Lin, CW, et al. Novel application of magnetite nanoparticle-mediated vitamin D3 delivery for peritoneal dialysis-related peritoneal damage. Int J Nanomedicine. (2021) 16:2137–46. doi: 10.2147/ijn.S291001
51. Zhang, Q, Wang, M, Mu, G, Ren, H, He, C, Xie, Q, et al. Adsorptivity of cationic cellulose nanocrystals for phosphate and its application in hyperphosphatemia therapy. Carbohydr Polym. (2021) 255:117335. doi: 10.1016/j.carbpol.2020.117335
52. Reichel, H, Zee, J, Tu, C, Young, E, Pisoni, RL, Stengel, B, et al. Chronic kidney disease progression and mortality risk profiles in Germany: results from the chronic kidney disease outcomes and practice patterns study. Nephrol Dial Transplant. (2020) 35:803–10. doi: 10.1093/ndt/gfz260
53. Nelson, RG, Grams, ME, Ballew, SH, Sang, Y, Azizi, F, Chadban, SJ, et al. Development of risk prediction equations for incident chronic kidney disease. JAMA. (2019) 322:2104–14. doi: 10.1001/jama.2019.17379
54. Wahab, MA, Saad, MM, and Baraka, KA. Microalbuminuria is a late event in patients with hypertension: do we need a lower threshold? J Saudi Heart Assoc. (2017) 29:30–6. doi: 10.1016/j.jsha.2015.12.003
55. Sung, KC, Ryu, S, Lee, JY, Lee, SH, Cheong, E, Hyun, YY, et al. Urine albumin/Creatinine ratio below 30 mg/g is a predictor of incident hypertension and cardiovascular mortality. J Am Heart Assoc. (2016) 5. doi: 10.1161/jaha.116.003245
56. Klausen, K, Borch-Johnsen, K, Feldt-Rasmussen, B, Jensen, G, Clausen, P, Scharling, H, et al. Very low levels of microalbuminuria are associated with increased risk of coronary heart disease and death independently of renal function, hypertension, and diabetes. Circulation. (2004) 110:32–5. doi: 10.1161/01.Cir.0000133312.96477.48
57. Klausen, KP, Scharling, H, and Jensen, JS. Very low level of microalbuminuria is associated with increased risk of death in subjects with cardiovascular or cerebrovascular diseases. J Intern Med. (2006) 260:231–7. doi: 10.1111/j.1365-2796.2006.01679.x
58. Dolati, S, Soleymani, J, Kazem Shakouri, S, and Mobed, A. The trends in nanomaterial-based biosensors for detecting critical biomarkers in stroke. Int J Clin Chem. (2021) 514:107–21. doi: 10.1016/j.cca.2020.12.034
59. Mosier-Boss, PA. Review of SERS substrates for chemical sensing. Nanomaterials. (2017) 7:142. doi: 10.3390/nano7060142
60. McNay, G, Eustace, D, Smith, WE, Faulds, K, and Graham, D. Surface-enhanced Raman scattering (SERS) and surface-enhanced resonance Raman scattering (SERRS): a review of applications. Appl Spectrosc. (2011) 65:825–37. doi: 10.1366/11-06365
61. Voss, A, Baier, V, Reisch, R, von Roda, K, Elsner, P, Ahlers, H, et al. Smelling renal dysfunction via electronic nose. Ann Biomed Eng. (2005) 33:656–60. doi: 10.1007/s10439-005-1438-2
62. Senéterre, E, Weissleder, R, Jaramillo, D, Reimer, P, Lee, AS, Brady, TJ, et al. Bone marrow: ultrasmall superparamagnetic iron oxide for MR imaging. Radiology. (1991) 179:529–33. doi: 10.1148/radiology.179.2.2014305
63. Goodwill, PW, Saritas, EU, Croft, LR, Kim, TN, Krishnan, KM, Schaffer, DV, et al. X-space MPI: magnetic nanoparticles for safe medical imaging. Adv Mater. (2012) 24:3870–7. doi: 10.1002/adma.201200221
64. Weizenecker, J, Gleich, B, Rahmer, J, Dahnke, H, and Borgert, J. Three-dimensional real-time in vivo magnetic particle imaging. Phys Med Biol. (2009) 54:L1–l10. doi: 10.1088/0031-9155/54/5/l01
65. Katzberg, RW, and Haller, C. Contrast-induced nephrotoxicity: clinical landscape. Kidney Int Suppl. (2006) 100:S3–7. doi: 10.1038/sj.ki.5000366
66. Sadowski, EA, Bennett, LK, Chan, MR, Wentland, AL, Garrett, AL, Garrett, RW, et al. Nephrogenic systemic fibrosis: risk factors and incidence estimation. Radiology. (2007) 243:148–57. doi: 10.1148/radiol.2431062144
67. Neuwelt, EA, Hamilton, BE, Varallyay, CG, Rooney, WR, Edelman, RD, Jacobs, PM, et al. Ultrasmall superparamagnetic iron oxides (USPIOs): a future alternative magnetic resonance (MR) contrast agent for patients at risk for nephrogenic systemic fibrosis (NSF)? Kidney Int. (2009) 75:465–74. doi: 10.1038/ki.2008.496
68. Vasanawala, SS, Nguyen, KL, Hope, MD, Bridges, MD, Hope, TA, Reeder, SB, et al. Safety and technique of ferumoxytol administration for MRI. Magn Reson Med. (2016) 75:2107–11. doi: 10.1002/mrm.26151
69. Toth, GB, Varallyay, CG, Horvath, A, Bashir, MR, Choyke, PL, Daldrup-Link, HE, et al. Current and potential imaging applications of ferumoxytol for magnetic resonance imaging. Kidney Int. (2017) 92:47–66. doi: 10.1016/j.kint.2016.12.037
70. Balakrishnan, VS, Rao, M, Kausz, AT, Brenner, L, Pereira, BJ, Frigo, TB, et al. Physicochemical properties of ferumoxytol, a new intravenous iron preparation. Eur J Clin Investig. (2009) 39:489–96. doi: 10.1111/j.1365-2362.2009.02130.x
71. Knobloch, G, Colgan, T, Wiens, CN, Wang, X, Schubert, T, Hernando, D, et al. Relaxivity of Ferumoxytol at 1.5 T and 3.0 T. Investig Radiol. (2018) 53:257–63. doi: 10.1097/rli.0000000000000434
72. Stoumpos, S, Hennessy, M, Vesey, AT, Radjenovic, A, Kasthuri, R, Kingsmore, DB, et al. Ferumoxytol magnetic resonance angiography: a dose-finding study in patients with chronic kidney disease. Eur Radiol. (2019) 29:3543–52. doi: 10.1007/s00330-019-06137-4
73. Hope, MD, Hope, TA, Zhu, C, Faraji, F, Haraldsson, H, Ordovas, KG, et al. Vascular imaging with Ferumoxytol as a contrast agent. AJR Am J Roentgenol. (2015) 205:W366–73. doi: 10.2214/ajr.15.14534
74. Mukundan, S, Steigner, ML, Hsiao, LL, Malek, SK, Tullius, SG, Chin, MS, et al. Ferumoxytol-enhanced magnetic resonance imaging in late-stage CKD. Am J Kidney Dis. (2016) 67:984–8. doi: 10.1053/j.ajkd.2015.12.017
75. Nayak, AB, Luhar, A, Hanudel, M, Gales, B, Hall, TR, Finn, JP, et al. High-resolution, whole-body vascular imaging with ferumoxytol as an alternative to gadolinium agents in a pediatric chronic kidney disease cohort. Pediatr Nephrol. (2015) 30:515–21. doi: 10.1007/s00467-014-2953-x
76. Luhar, A, Khan, S, Finn, JP, Ghahremani, S, Griggs, R, Zaritsky, J, et al. Contrast-enhanced magnetic resonance venography in pediatric patients with chronic kidney disease: initial experience with ferumoxytol. Pediatr Radiol. (2016) 46:1332–40. doi: 10.1007/s00247-016-3605-z
77. Yu, M, Zhou, J, du, B, Ning, X, Authement, C, Gandee, L, et al. Noninvasive staging of kidney dysfunction enabled by renal-clearable luminescent gold nanoparticles. Angew Chem Int Ed Engl. (2016) 55:2787–91. doi: 10.1002/anie.201511148
78. Yu, M, Liu, J, Ning, X, and Zheng, J. High-contrast noninvasive imaging of kidney clearance kinetics enabled by renal clearable Nanofluorophores. Angew Chem Int Ed Engl. (2015) 54:15434–8. doi: 10.1002/anie.201507868
79. Yu, M, and Zheng, J. Clearance pathways and tumor targeting of imaging nanoparticles. ACS Nano. (2015) 9:6655–74. doi: 10.1021/acsnano.5b01320
80. Yang, S, Sun, S, Zhou, C, Hao, G, Liu, J, Ramezani, S, et al. Renal clearance and degradation of glutathione-coated copper nanoparticles. Bioconjug Chem. (2015) 26:511–9. doi: 10.1021/acs.bioconjchem.5b00003
81. Zhou, Z, Wang, L, Chi, X, Bao, J, Yang, L, Zhao, W, et al. Engineered iron-oxide-based nanoparticles as enhanced T1 contrast agents for efficient tumor imaging. ACS Nano. (2013) 7:3287–96. doi: 10.1021/nn305991e
82. Burns, AA, Vider, J, Ow, H, Herz, E, Penate-Medina, O, Baumgart, M, et al. Fluorescent silica nanoparticles with efficient urinary excretion for nanomedicine. Nano Lett. (2009) 9:442–8. doi: 10.1021/nl803405h
83. Huang, X, Zhang, F, Zhu, L, Choi, KY, Guo, N, Guo, J, et al. Effect of injection routes on the biodistribution, clearance, and tumor uptake of carbon dots. ACS Nano. (2013) 7:5684–93. doi: 10.1021/nn401911k
84. Tang, S, Chen, M, and Zheng, N. Sub-10-nm Pd nanosheets with renal clearance for efficient near-infrared photothermal cancer therapy. Small. (2014) 10:3139–44. doi: 10.1002/smll.201303631
85. Alani, H, Tamimi, A, and Tamimi, N. Cardiovascular co-morbidity in chronic kidney disease: current knowledge and future research needs. World J Nephrol. (2014) 3:156–68. doi: 10.5527/wjn.v3.i4.156
86. Cozzolino, M, Mangano, M, Stucchi, A, Ciceri, P, Conte, F, and Galassi, A. Cardiovascular disease in dialysis patients. Nephrol Dial Transplant. (2018) 33:iii28–34. doi: 10.1093/ndt/gfy174
87. Leopold, JA. Vascular calcification: mechanisms of vascular smooth muscle cell calcification. Trends Cardiovasc Med. (2015) 25:267–74. doi: 10.1016/j.tcm.2014.10.021
88. Sage, AP, Lu, J, Tintut, Y, and Demer, LL. Hyperphosphatemia-induced nanocrystals upregulate the expression of bone morphogenetic protein-2 and osteopontin genes in mouse smooth muscle cells in vitro. Kidney Int. (2011) 79:414–22. doi: 10.1038/ki.2010.390
89. Morgan, MP, and McCarthy, GM. Signaling mechanisms involved in crystal-induced tissue damage. Curr Opin Rheumatol. (2002) 14:292–7. doi: 10.1097/00002281-200205000-00017
90. Nadra, I, Mason, JC, Philippidis, P, Florey, O, Smythe, CD, McCarthy, GM, et al. Proinflammatory activation of macrophages by basic calcium phosphate crystals via protein kinase C and MAP kinase pathways: a vicious cycle of inflammation and arterial calcification? Circ Res. (2005) 96:1248–56. doi: 10.1161/01.RES.0000171451.88616.c2
91. Sun, Y, Zeng, XR, Wenger, L, and Cheung, HS. Basic calcium phosphate crystals stimulate the endocytotic activity of cells--inhibition by anti-calcification agents. Biochem Biophys Res Commun. (2003) 312:1053–9. doi: 10.1016/j.bbrc.2003.11.048
92. Aikawa, E, Nahrendorf, M, Figueiredo, JL, Swirski, FK, Shtatland, T, Kohler, RH, et al. Osteogenesis associates with inflammation in early-stage atherosclerosis evaluated by molecular imaging in vivo. Circulation. (2007) 116:2841–50. doi: 10.1161/circulationaha.107.732867
93. Shroff, RC, McNair, R, Skepper, JN, Figg, N, Schurgers, LJ, Deanfield, J, et al. Chronic mineral dysregulation promotes vascular smooth muscle cell adaptation and extracellular matrix calcification. J Am Soc Nephrol. (2010) 21:103–12. doi: 10.1681/asn.2009060640
94. Schlieper, G, Aretz, A, Verberckmoes, SC, Krüger, T, Behets, GJ, Ghadimi, R, et al. Ultrastructural analysis of vascular calcifications in uremia. J Am Soc Nephrol. (2010) 21:689–96. doi: 10.1681/asn.2009080829
95. Mackay, EM, and Oliver, J. Renal damage following the ingestion of a diet containing an excess of inorganic phosphate. J Exp Med. (1935) 61:319–34. doi: 10.1084/jem.61.3.319
96. Ori, Y, Herman, M, Gafter, U, Chagnac, A, Korzets, A, Tobar, A, et al. Acute phosphate nephropathy-an emerging threat. Am J Med Sci. (2008) 336:309–14. doi: 10.1097/MAJ.0b013e318167410c
97. Kuro, OM. Klotho and endocrine fibroblast growth factors: markers of chronic kidney disease progression and cardiovascular complications? Nephrol Dial Transplant. (2019) 34:15–21. doi: 10.1093/ndt/gfy126
98. Kunishige, R, Mizoguchi, M, Tsubouchi, A, Hanaoka, K, Miura, Y, Kurosu, H, et al. Calciprotein particle-induced cytotoxicity via lysosomal dysfunction and altered cholesterol distribution in renal epithelial HK-2 cells. Sci Rep. (2020) 10:20125. doi: 10.1038/s41598-020-77308-3
99. Pasch, A, Farese, S, Gräber, S, Wald, J, Richtering, W, Floege, J, et al. Nanoparticle-based test measures overall propensity for calcification in serum. J Am Soc Nephrol. (2012) 23:1744–52. doi: 10.1681/asn.2012030240
100. Distler, JHW, Györfi, AH, Ramanujam, M, Whitfield, ML, Königshoff, M, and Lafyatis, R. Shared and distinct mechanisms of fibrosis. Nat Rev Rheumatol. (2019) 15:705–30. doi: 10.1038/s41584-019-0322-7
101. Djudjaj, S, and Boor, P. Cellular and molecular mechanisms of kidney fibrosis. Mol Asp Med. (2019) 65:16–36. doi: 10.1016/j.mam.2018.06.002
103. Waasdorp, M, de Rooij, DM, Florquin, S, Duitman, J, and Spek, CA. Protease-activated receptor-1 contributes to renal injury and interstitial fibrosis during chronic obstructive nephropathy. J Cell Mol Med. (2019) 23:1268–79. doi: 10.1111/jcmm.14028
104. Chen, J, Chen, JK, and Harris, RC. EGF receptor deletion in podocytes attenuates diabetic nephropathy. J Am Soc Nephrol. (2015) 26:1115–25. doi: 10.1681/asn.2014020192
105. Wu, M, Tang, RN, Liu, H, Pan, MM, Lv, LL, Zhang, JD, et al. Cinacalcet ameliorates cardiac fibrosis in uremic hearts through suppression of endothelial-to-mesenchymal transition. Int J Cardiol. (2014) 171:e65–9. doi: 10.1016/j.ijcard.2013.11.105
106. Smith, ER, Tan, SJ, Holt, SG, and Hewitson, TD. FGF23 is synthesised locally by renal tubules and activates injury-primed fibroblasts. Sci Rep. (2017) 7:3345. doi: 10.1038/s41598-017-02709-w
107. Smith, ER, Holt, SG, and Hewitson, TD. FGF23 activates injury-primed renal fibroblasts via FGFR4-dependent signalling and enhancement of TGF-β autoinduction. Int J Biochem Cell Biol. (2017) 92:63–78. doi: 10.1016/j.biocel.2017.09.009
108. Singh, VP, Bali, A, Singh, N, and Jaggi, AS. Advanced glycation end products and diabetic complications. Korean J Physiol Pharmacol. (2014) 18:1–14. doi: 10.4196/kjpp.2014.18.1.1
109. Mizobuchi, M, Morrissey, J, Finch, JL, Martin, DR, Liapis, H, Akizawa, T, et al. Combination therapy with an angiotensin-converting enzyme inhibitor and a vitamin D analog suppresses the progression of renal insufficiency in uremic rats. J Am Soc Nephrol. (2007) 18:1796–806. doi: 10.1681/asn.2006091028
110. Khosroshahi, HT, Abedi, B, Daneshvar, S, Sarbaz, Y, and Shakeri, BA. Future of the renal biopsy: time to change the conventional modality using nanotechnology. Int J Biomed Imaging. (2017) 2017:6141734. doi: 10.1155/2017/6141734
111. Zhao, J, Wang, ZJ, Liu, M, Zhu, J, Zhang, X, Zhang, T, et al. Assessment of renal fibrosis in chronic kidney disease using diffusion-weighted MRI. Clin Radiol. (2014) 69:1117–22. doi: 10.1016/j.crad.2014.06.011
112. Martin, DR, Semelka, RC, Chapman, A, Peters, H, Finn, PJ, Kalb, B, et al. Nephrogenic systemic fibrosis versus contrast-induced nephropathy: risks and benefits of contrast-enhanced MR and CT in renally impaired patients. J Magn Reson Imaging. (2009) 30:1350–6. doi: 10.1002/jmri.21968
113. Trojanowska, M, LeRoy, EC, Eckes, B, and Krieg, T. Pathogenesis of fibrosis: type 1 collagen and the skin. J Mol Med. (1998) 76:266–74. doi: 10.1007/s001090050216
114. Colombo, C, Li, M, Watanabe, S, Messa, P, Edefonti, A, Montini, G, et al. Polymer nanoparticle engineering for Podocyte repair: from in vitro models to new Nanotherapeutics in kidney diseases. ACS Omega. (2017) 2:599–610. doi: 10.1021/acsomega.6b00423
115. Dong, X, Fu, J, Yin, X, Cao, S, Li, X, Lin, L, et al. Emodin: a review of its pharmacology, toxicity and pharmacokinetics. Phytother Res. (2016) 30:1207–18. doi: 10.1002/ptr.5631
116. Zeng, YQ, Dai, Z, Lu, F, Lu, Z, Liu, X, Chen, C, et al. Emodin via colonic irrigation modulates gut microbiota and reduces uremic toxins in rats with chronic kidney disease. Oncotarget. (2016) 7:17468–78. doi: 10.18632/oncotarget.8160
117. Rodríguez-Peña, AB, Santos, E, Arévalo, M, and López-Novoa, JM. Activation of small GTPase Ras and renal fibrosis. J Nephrol. (2005) 18:341–9.
118. Newbury, LJ, Wang, JH, Hung, G, Hendry, BM, and Sharpe, CC. Inhibition of Kirsten-Ras reduces fibrosis and protects against renal dysfunction in a mouse model of chronic folic acid nephropathy. Sci Rep. (2019) 9:14010. doi: 10.1038/s41598-019-50422-7
119. Grande, MT, Fuentes-Calvo, I, Arévalo, M, Heredia, F, Santos, E, Martínez-Salgado, C, et al. Deletion of H-Ras decreases renal fibrosis and myofibroblast activation following ureteral obstruction in mice. Kidney Int. (2010) 77:509–18. doi: 10.1038/ki.2009.498
120. Clarke, HC, Kocher, HM, Khwaja, A, Kloog, Y, Cook, HT, and Hendry, BM. Ras antagonist farnesylthiosalicylic acid (FTS) reduces glomerular cellular proliferation and macrophage number in rat thy-1 nephritis. J Am Soc Nephrol. (2003) 14:848–54. doi: 10.1097/01.asn.0000057543.55318.8b
121. Coyne, DW. Ferumoxytol for treatment of iron deficiency anemia in patients with chronic kidney disease. Expert Opin Pharmacother. (2009) 10:2563–8. doi: 10.1517/14656560903224998
122. Lu, M, Cohen, MH, Rieves, D, and Pazdur, R. FDA report: Ferumoxytol for intravenous iron therapy in adult patients with chronic kidney disease. Am J Hematol. (2010) 85:315–9. doi: 10.1002/ajh.21656
123. Schiller, B, Bhat, P, and Sharma, A. Safety and effectiveness of ferumoxytol in hemodialysis patients at 3 dialysis chains in the United States over a 12-month period. Clin Ther. (2014) 36:70–83. doi: 10.1016/j.clinthera.2013.09.028
124. Macdougall, IC, Strauss, WE, McLaughlin, J, Li, Z, Dellanna, F, and Hertel, J. A randomized comparison of ferumoxytol and iron sucrose for treating iron deficiency anemia in patients with CKD. Clin J Am Soc Nephrol. (2014) 9:705–12. doi: 10.2215/cjn.05320513
125. Macdougall, IC, Strauss, WE, Dahl, NV, Bernard, K, and Li, Z. Ferumoxytol for iron deficiency anemia in patients undergoing hemodialysis. The FACT randomized controlled trial. Clin Nephrol. (2019) 91:237–45. doi: 10.5414/cn109512
126. McCormack, PL. Ferumoxytol: in iron deficiency anaemia in adults with chronic kidney disease. Drugs. (2012) 72:2013–22. doi: 10.2165/11209880-000000000-00000
127. Wetmore, JB, Weinhandl, ED, Zhou, J, and Gilbertson, DT. Relative incidence of acute adverse events with Ferumoxytol compared to other intravenous iron compounds: a matched cohort study. PLoS One. (2017) 12:e0171098. doi: 10.1371/journal.pone.0171098
128. Provenzano, R, Schiller, B, Rao, M, Coyne, D, Brenner, L, and Pereira, BJ. Ferumoxytol as an intravenous iron replacement therapy in hemodialysis patients. Clin J Am Soc Nephrol. (2009) 4:386–93. doi: 10.2215/cjn.02840608
129. Shepshelovich, D, Rozen-Zvi, B, Avni, T, Gafter, U, and Gafter-Gvili, A. Intravenous versus Oral iron supplementation for the treatment of anemia in CKD: an updated systematic review and meta-analysis. Am J Kidney Dis. (2016) 68:677–90. doi: 10.1053/j.ajkd.2016.04.018
130. Andrassy, KM. Comments on 'KDIGO 2012 clinical practice guideline for the evaluation and Management of Chronic Kidney Disease'. Kidney Int. (2013) 84:622–3. doi: 10.1038/ki.2013.243
131. Hole, B, Hemmelgarn, B, Brown, E, Brown, M, McCulloch, MI, Zuniga, C, et al. Supportive care for end-stage kidney disease: an integral part of kidney services across a range of income settings around the world. Kidney Int Suppl. (2020) 10:e86–94. doi: 10.1016/j.kisu.2019.11.008
132. Kramer, A, Pippias, M, Noordzij, M, Stel, VS, Afentakis, N, Ambühl, PM, et al. The European renal association—European dialysis and transplant association (ERA-EDTA) registry annual report 2015: a summary. Clin Kidney J. (2018) 11:108–22. doi: 10.1093/ckj/sfx149
133. Chan, KE, Maddux, FW, Tolkoff-Rubin, N, Karumanchi, SA, Thadhani, R, and Hakim, RM. Early outcomes among those initiating chronic dialysis in the United States. Clin J Am Soc Nephrol. (2011) 6:2642–9. doi: 10.2215/cjn.03680411
134. Modi, ZJ, Lu, Y, Ji, N, Kapke, A, Selewski, DT, Dietrich, X, et al. Risk of cardiovascular disease and mortality in Young adults with end-stage renal disease: an analysis of the US renal data system. JAMA Cardiol. (2019) 4:353–62. doi: 10.1001/jamacardio.2019.0375
135. Wetmore, JB, Li, S, Molony, JT, Guo, H, Herzog, CA, Gilbertson, DT, et al. Insights from the 2016 Peer kidney care initiative report: still a ways to go to improve Care for Dialysis Patients. Am J Kidney Dis. (2018) 71:123–32. doi: 10.1053/j.ajkd.2017.08.023
136. Pruthi, R, Steenkamp, R, and Feest, T. UK renal registry 16th annual report: chapter 8 survival and cause of death of UK adult patients on renal replacement therapy in 2012: national and Centre-specific analyses. Nephron Clin Pract. (2013) 125:139–69. doi: 10.1159/000360027
137. Erickson, KF, Zhao, B, Ho, V, and Winkelmayer, WC. Employment among patients starting dialysis in the United States. Clin J Am Soc Nephrol. (2018) 13:265–73. doi: 10.2215/cjn.06470617
138. Wang, V, Vilme, H, Maciejewski, ML, and Boulware, LE. The economic burden of chronic kidney disease and end-stage renal disease. Semin Nephrol. (2016) 36:319–30. doi: 10.1016/j.semnephrol.2016.05.008
139. Abidin, MNZ, Goh, PS, Ismail, AF, Said, N, Othman, MHD, Hasbullah, H, et al. Highly adsorptive oxidized starch nanoparticles for efficient urea removal. Carbohydr Polym. (2018) 201:257–63. doi: 10.1016/j.carbpol.2018.08.069
140. Missaoui, WN, Arnold, RD, and Cummings, BS. Toxicological status of nanoparticles: what we know and what we don't know. Chem Biol Interact. (2018) 295:1–12. doi: 10.1016/j.cbi.2018.07.015
141. Sousa de Almeida, M, Susnik, E, Drasler, B, Taladriz-Blanco, P, Petri-Fink, A, and Rothen-Rutishauser, B. Understanding nanoparticle endocytosis to improve targeting strategies in nanomedicine. Chem Soc Rev. (2021) 50:5397–434. doi: 10.1039/d0cs01127d
142. Liao, C, Li, Y, and Tjong, SC. Bactericidal and cytotoxic properties of silver nanoparticles. Int J Mol Sci. (2019) 20:449. doi: 10.3390/ijms20020449
143. Tang, W, Fan, W, Lau, J, Deng, L, Shen, Z, and Chen, X. Emerging blood-brain-barrier-crossing nanotechnology for brain cancer theranostics. Chem Soc Rev. (2019) 48:2967–3014. doi: 10.1039/C8CS00805A
144. Wu, T, and Tang, M. Review of the effects of manufactured nanoparticles on mammalian target organs. J Appl Toxicol. (2018) 38:25–40. doi: 10.1002/jat.3499
145. Kreyling, WG, Semmler-Behnke, M, Seitz, J, Scymczak, W, Wenk, A, Mayer, P, et al. Size dependence of the translocation of inhaled iridium and carbon nanoparticle aggregates from the lung of rats to the blood and secondary target organs. Inhal Toxicol. (2009) 21:55–60. doi: 10.1080/08958370902942517
146. Nemmar, A, Yuvaraju, P, Beegam, S, Yasin, J, Kazzam, EE, and Ali, BH. Oxidative stress, inflammation, and DNA damage in multiple organs of mice acutely exposed to amorphous silica nanoparticles. Int J Nanomedicine. (2016) 11:919–28. doi: 10.2147/ijn.S92278
147. Couto, D, Freitas, M, Costa, VM, Chisté, RC, Almeida, A, Lopez-Quintela, MA, et al. Biodistribution of polyacrylic acid-coated iron oxide nanoparticles is associated with proinflammatory activation and liver toxicity. J Appl Toxicol. (2016) 36:1321–31. doi: 10.1002/jat.3323
148. Teodoro, JS, Silva, R, Varela, AT, Duarte, FV, Rolo, AP, Hussain, S, et al. Low-dose, subchronic exposure to silver nanoparticles causes mitochondrial alterations in Sprague-Dawley rats. Nanomedicine. (2016) 11:1359–75. doi: 10.2217/nnm-2016-0049
149. Najahi-Missaoui, W, Arnold, RD, and Cummings, BS. Safe nanoparticles: are we there yet? Int J Mol Sci. (2020) 22:385. doi: 10.3390/ijms22010385
150. Desai, N. Challenges in development of nanoparticle-based therapeutics. AAPS J. (2012) 14:282–95. doi: 10.1208/s12248-012-9339-4
151. Onoue, S, Yamada, S, and Chan, HK. Nanodrugs: pharmacokinetics and safety. Int J Nanomedicine. (2014) 9:1025–37. doi: 10.2147/ijn.S38378
152. Simpson, CA, Salleng, KJ, Cliffel, DE, and Feldheim, DL. In vivo toxicity, biodistribution, and clearance of glutathione-coated gold nanoparticles. Nanomedicine. (2013) 9:257–63. doi: 10.1016/j.nano.2012.06.002
153. Buzea, C, Pacheco, II, and Robbie, K. Nanomaterials and nanoparticles: sources and toxicity. Biointerphases. (2007) 2:Mr17–71. doi: 10.1116/1.2815690
154. Yang, G, Phua, SZF, Bindra, AK, and Zhao, Y. Degradability and clearance of inorganic nanoparticles for biomedical applications. Adv Mater. (2019) 31:e1805730. doi: 10.1002/adma.201805730
155. Janssen, EM, Dy, SM, Meara, AS, Kneuertz, PJ, Presley, CJ, and Bridges, JFP. Analysis of patient preferences in lung cancer—estimating acceptable tradeoffs between treatment benefit and side effects. Patient Prefer Adherence. (2020) 14:927–37. doi: 10.2147/ppa.S235430
Keywords: kidney, CKD, diagnosis, treatment, nanoparticles
Citation: Yang K, Shang Y, Yang N, Pan S, Jin J and He Q (2023) Application of nanoparticles in the diagnosis and treatment of chronic kidney disease. Front. Med. 10:1132355. doi: 10.3389/fmed.2023.1132355
Received: 27 December 2022; Accepted: 22 March 2023;
Published: 17 April 2023.
Edited by:
Shan Mou, Shanghai Jiao Tong University, ChinaReviewed by:
Satheesh Natarajan, Indian Institute of Technology Madras, IndiaCopyright © 2023 Yang, Shang, Yang, Pan, Jin and He. This is an open-access article distributed under the terms of the Creative Commons Attribution License (CC BY). The use, distribution or reproduction in other forums is permitted, provided the original author(s) and the copyright owner(s) are credited and that the original publication in this journal is cited, in accordance with accepted academic practice. No use, distribution or reproduction is permitted which does not comply with these terms.
*Correspondence: Juan Jin, bGFuZ18wMThAMTYzLmNvbQ==; Qiang He, cWlhbmdoZTE5NzNAMTI2LmNvbQ==
†These authors have contributed equally to this work
Disclaimer: All claims expressed in this article are solely those of the authors and do not necessarily represent those of their affiliated organizations, or those of the publisher, the editors and the reviewers. Any product that may be evaluated in this article or claim that may be made by its manufacturer is not guaranteed or endorsed by the publisher.
Research integrity at Frontiers
Learn more about the work of our research integrity team to safeguard the quality of each article we publish.