- Department of Biological Sciences, State University of New York College of Optometry, New York, NY, United States
The benign public perception of myopia (nearsightedness) as a visual inconvenience masks the severity of its sight-threatening consequences. Myopia is a significant risk factor for posterior pole conditions such as maculopathy, choroidal neovascularization and glaucoma, all of which have a vascular component. These associations strongly suggest that myopic eyes might experience vascular alterations prior to the development of complications. Myopic eyes are out of focus because they are larger in size, which in turn affects their overall structure and function, including those of the vascular beds. By reviewing the vascular changes that characterize myopia, this review aims to provide an understanding of the gross, cellular and molecular alterations identified at the structural and functional levels with the goal to provide an understanding of the latest evidence in the field of experimental and clinical myopia vascular research. From the evidence presented, we hypothesize that the interaction between excessive myopic eye growth and vascular alterations are tipping-points for the development of sight-threatening changes.
1. Introduction
The blinding consequences of myopia are often overlooked (1–7). Myopic eyes are larger in size, which alters not only their focusing ability, but also their anatomy and physiology (8). All myopes, regardless of degree, are at increased risk of visual impairment (3, 9, 10). This has significant implications due to the predicted global increase in myopia prevalence and the potential public health crisis it represents (8, 11). As we learn about the role that peripheral refraction (12, 13), light intensity (14, 15), time spent outdoors (16, 17), or the on/off pathways play in the development of myopia (18, 19), the controversy is no longer whether myopia is genetic or environmental, but whether we can identify the variables that interact in this multifactorial condition. Currently, there are no preventive markers for myopic degeneration, which is predicted to threaten the eyesight of five billion people by 2050. Myopic eyes have thinner choroids and scleras and, if they progress into high myopia, they can have secondary macular defects in Bruch’s membrane along with a complete loss of retinal pigment epithelium, choriocapillaris, and retinal photoreceptors, which confirms the effect of myopia on the ocular vasculature.
The eye’s vascular network comprises a complex grid of supply and drainage structures. The retina has a high metabolic rate and oxygen consumption per unit weight in the body (20). In humans, the retina is supplied by the central retinal artery (CRA) - directly in charge of the inner two thirds by diffusion to rods, cones and outer layers - and the choroid, supplying the outer third. The retina is particularly vulnerable to ischemia because of its high oxygen demand and low vascularity of the inner layers (21, 22). The choroid has a high flow rate, low oxygen exchange and a fenestrated capillary bed. While the choroidal circulation is mainly controlled by sympathetic innervation and thought not to be autoregulated (23), the retinal circulation is ruled by locally controlled autoregulatory mechanisms, including mediators released by endothelial cells (23). A web-like capillary network spreads throughout the retina to provide additional supply (24), connects arteries and veins, and allows direct transport of oxygen, water and lipids to the tissues by diffusion. Capillaries are most abundant in the macula but absent from the fovea (capillary-free zone), which obtains its nutrients from the choriocapillaris (25). The superficial optic nerve head zone is supplied by the central retinal artery, while the short posterior ciliary arteries supply the lamina cribrosa (24, 26).
Until recently, the perfusion features of the human myopic eye had only been studied in human pathological myopia (27, 28) and experimental models of myopia (29–33). This review aims to summarize the latest evidence and controversies in the field of experimental and clinical myopia vascular research by addressing the structural and functional gross, cellular and molecular vascular alterations identified in myopic eyes.
2. Vascular features of the human myopic eye
Most of the techniques used to assess ocular hemodynamics in vivo are non-invasive imaging systems that assess retinal blood-flow velocity directly or indirectly (laser doppler velocimetry, LDV) (34), oxygen saturation (oximetry) (35), capillary perfusion (optical coherence tomography angiography, OCTA) (36), microvascular health (adaptive optics scanning light ophthalmoscope fluorescein angiography, AOSLO FA) (37), blood flow (laser speckle contrast imaging LSCI) (38), choroidal pulsatile ocular blood flow (POBF) (39), retrobulbar blood velocity (color doppler imaging, CDI) and retinal blood velocity (laser doppler velocimetry, LDV) (34), amongst others. Until recently, the only method providing a measure of absolute retinal blood flow was the combination of LDV blood velocity with retinal vessel diameter measures from fundus photographs (40, 41). This technique is time-consuming and impractical in the clinic. The recent development of doppler optical coherence tomography (DOCT) provides full quantitative volumetric information of blood flow and vascular/structural anatomy (42). Due to the variety of techniques available, it is imperative to consider the unique technical, anatomical and clinical characteristics of each instrument when interpreting outcomes.
It is hypothesized that the compromised hemodynamics observed in young healthy myopes is an early feature of the decreased ocular blood flow reported in pathological myopia. Such vascular features would increase the susceptibility of the myopic eye for vascular and age-related eye diseases. For instance, impaired retinal blood flow might increase the risk to develop chorioretinal atrophy in high myopia. These changes possibly interact with the known effect of aging on the retinal and choroidal vasculature, including decreased tissue perfusion, deep capillary plexus vessel density, venous, capillary and choroidal blood flow and loss of endothelial cells amongst others (43–50). Below we review experimental and clinical evidence suggesting the existence of vascular alterations in physiological and degenerative myopia (Figure 1).
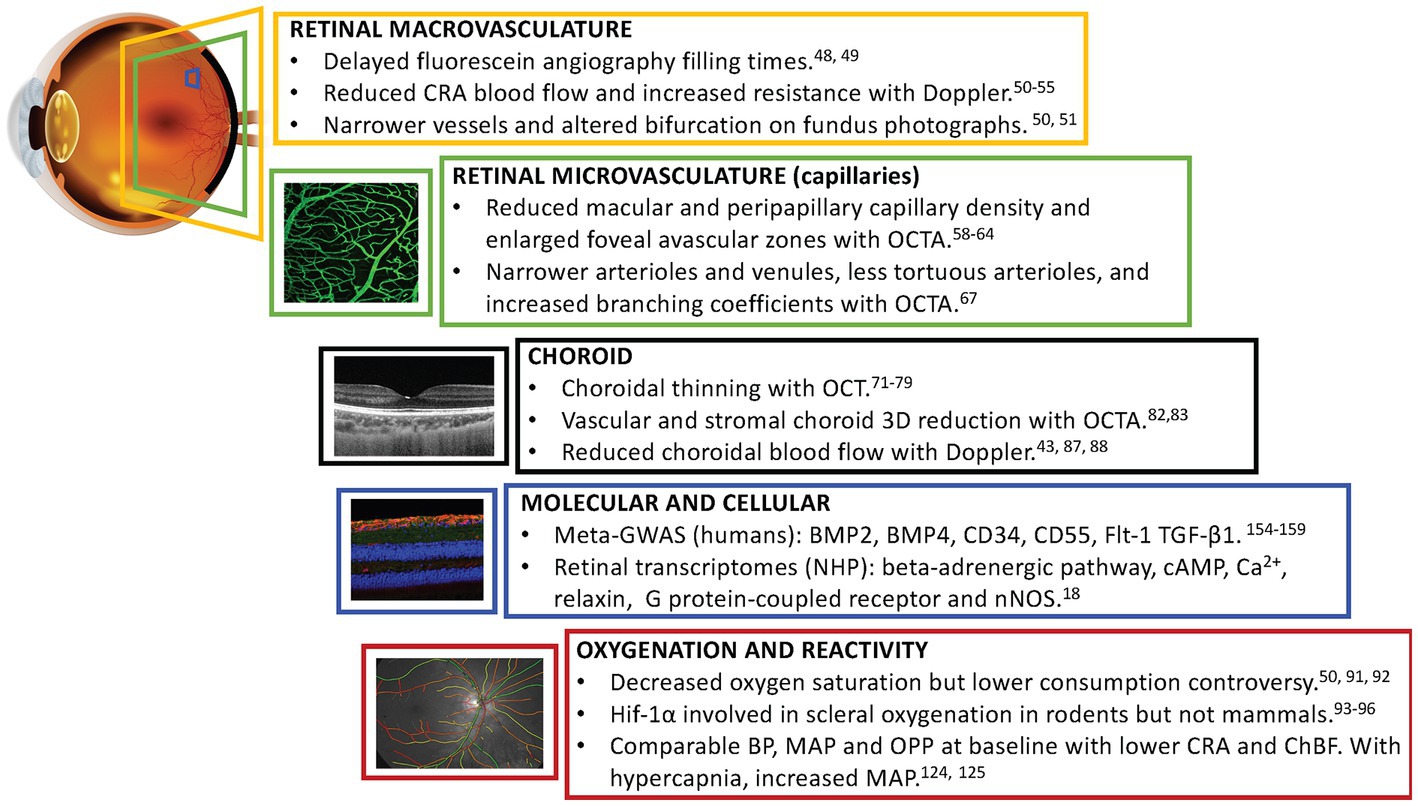
Figure 1. Schematic summary describing key vascular findings identified in myopic eyes to date. CRA, central retinal artery; OCTA, optical coherence tomography angiography; BMP2 and BMP4, bone morphogenetic proteins 2 and 4; CD34 and CD55, transmembrane proteins CD34 and CD55; Flt-1, vascular endothelial growth factor receptor-1; TGF-β1, transforming growth factor beta receptor; cAMP, cyclic adenosine monophosphate; Ca2+, calcium; nNOS, neuronal isoform of nitric oxide; Hif-1α, hypoxia-inducible factor 1-alpha; BP, blood pressure; MAP, mean arterial pressure; OPP, ocular perfusion pressure; ChBF, choroidal blood flow.
3. The effect of myopia on the retinal vasculature
The retinal vasculature provides metabolic support to neural and glial cells while minimally interfering with light-sensing mechanisms (51). One of the first pieces of evidence describing an altered retinal circulation in myopic eyes was the discovery of delayed filling times in the arterial, arterial–venous and venous phases of high myopic eyes using fluorescein angiography in the 1970s (52, 53). Subsequently, myopic eyes have been found to exhibit narrower vessel diameters (54), altered bifurcation (55) and reduced central retinal artery (CRA) diameter and blood flow (56–59). This effect appears localized to the CRA once it branches out of the ophthalmic artery (OA), and therefore affects the inner retina but not structures supplied by the OA such as eyelids, lacrimal gland, conjunctiva, posterior uveal tract or extraocular muscles. In degenerative myopia, both choroidal and retinal blood flow appear reduced, which has been hypothesized to be partly due to increased vascular resistance or adaptive changes the myopic eye experiences to cope with its enlargement (27, 28, 60).
At the retinal microvascular level, healthy myopic eyes show reduced macular superficial, deep and radial peripapillary capillary vessel density, along with increased density inside the disc and enlarged foveal avascular zones (61–67). There is controversy, however, to whether these reductions in capillary densities actually result in altered capillary blood flow (63, 64), since there is evidence that reductions in capillary density are not necessarily associated with choroidal thickness (66) or retinal nerve fiber layer function (68). In terms of anatomy, myopic eyes with greater axial lengths exhibit narrower and less tortuous arterioles and venules, and greater branching coefficients (69). The lower capillary density observed in myopic eyes has been proposed to be a protective mechanism for decreased risk of diabetic retinopathy, but the protective effect has not been confirmed in later studies (70).
The alterations observed in healthy myopic eyes may be precursors of changes seen in pathological myopia and possibly involved in the pathophysiology of myopic degeneration: decreased density of the deep radial capillary plexus and a reduction in OA blood flow that relates to the severity of the retinal degeneration (27).
4. The effect of myopia on the choroidal vasculature
Choroidal thickness is a marker of myopia development first identified in experimental myopia in avian eyes (32, 71). In fact, gross anatomical changes in choroidal appearance from myopic chick eyes led to a series of publications confirming bidirectional choroidal thickness changes in response to defocus (72). These changes have been observed in mammal, non-human and human primate eyes (72–80). In addition, during childhood the choroid thickens with normal eye development, but to a lesser extent in children developing myopia, which confirms the role the choroid may play during myopia development (81, 82). There is also evidence of a three-dimensional reduction in choroidal vascular and stromal components in myopic eyes, mainly in the nasal and subfoveal region (83), although this vascular thinning remains controversial (84). The ocular pulsatile blood flow, thought to be mainly choroidal (85), also appears reduced in myopic eyes (39). This reduction, however, might be an artefact of enlarged eye volume (56, 86), since ocular pulsatile calculations depend on intraocular volume (87). In experimental models of myopia, choroidal flow reductions relate to thickness changes, suggesting an altered choroidal supply and thickness changes that might be responsible for choroidal flow changes or vice versa (88). However, this relationship has not been confirmed in humans - choroidal blood flow might remain constant in eyes with non-pathological myopia (89). There is also evidence that moderate, but not high myopes, exhibit greater ocular perfusion pressure as the choroid thins (90), and choriocapillaris flow deficits are greater in high myopes with no pathology (91). This suggests that early vascular anatomical and functional differences between moderate and high myopes need to be evaluated longitudinally and might represent clinically applicable biomarkers of early pathologic myopia.
A major cause of visual impairment in pathological myopia is loss of photoreceptors, which are nourished by the choroid. Therefore, any choroidal dysfunction can have detrimental consequences in myopic eyes. In fact, eyes with degenerative myopia exhibit lower posterior ciliary artery blood flow, which supply the choroid (27). Choroidal structure measures such as thickness, luminal and stromal area, and choroidal vascularity index are also significantly reduced in pathological myopia. In addition, thickness and vascularity index appear associated with degeneration severity and visual acuity, highlighting the role the choroid represents for degenerative myopia (92, 93).
5. Retinal oxygen saturation in myopia
The retina is characterized by its high metabolic rate and considered one of the tissues with the largest oxygen consumption per unit weight in the body (20). Retinal oximetry performed using commercially available systems like the Oxymap has identified a lower arterio/venous oxygen saturation ratio in myopic eyes that points towards a possibly lower retinal oxygen consumption (94). However, a significantly larger cross-sectional study with 1,461 participants found that when age, gender, body mass index (BMI), intraocular pressure (IOP) and axial length (AL) were corrected for, longer and more myopic eyes did not exhibited a lower, but a greater oxygen and arterio-venous ratio saturation (95). These findings suggest that as eyes grow larger, they might be able to maintain an adequate oxygenation profile for its growing size, until they reach a degenerative state and the oxygenation profile is affected (96). There is also work suggesting that myopic choroidal thinning and reduced choroidal blood flow might affect scleral oxygenation (97). If this is correct, manipulating hypoxia signaling pathways might be a myopia control alternative in the future (98). Recent evidence suggests that, in fact, anti-hypoxia drugs reducing Hif-1α levels can slow axial elongation (99), which points towards a possible relationship between myopia, hypoxia and Hif-1α. This relationship has also been described in genetic analyses revealing a moderate involvement of the Hif-1α signaling pathway in myopia (98, 100), However, tree shrews induced with myopia do not exhibit changes in scleral Hif-1α mRNA expression (101, 102), and guinea pigs with induced myopia show reduced scleral Hif-1α mRNA, highlighting the need for additional work in this field (102).
6. Vascular reactivity in myopia
The presence of structural and hemodynamic changes in the retinal vasculature in myopia suggests that myopic eyes might be suffering from an abnormal vascular function before degenerative changes occur (1, 54, 103–108). However, assessing ocular blood flow under normal conditions is not sufficient to detect vascular dysfunction. Retinal vascular function is assessed using provocation tests, indispensable to evaluate retinal reactivity and autoregulation (109–115). Autoregulation is the inherent local mechanism that ensures sufficient and stable blood flow under changing conditions to preserve adequate function of the surrounding tissues (116). Vascular regulation or vasoreactivity can be assessed by quantifying blood flow or vessel diameter changes that occur in response to flicker light (metabolic autoregulation), variations in the concentration of breathing oxygen (metabolic), or changes in systemic and intraocular pressure (shear-dependent and myogenic) (113, 117–120). Both the ocular and cerebral circulation exhibit an autoregulatory capacity (110, 117, 121–124). Three studies have evaluated the vascular reactivity profile of myopic eyes to date, and they used hypercapnia (increased pCO2) and hyperoxia (increased p02) as provocation tests to assess the response from the retinal vasculature under stress conditions (125–127). Under room-air conditions prior to the provocation test, myopic and non-myopic eyes exhibited comparable systemic and ocular perfusion pressures –myopes exhibiting lower central retinal artery and choroidal blood flow. After inducing hypercapnia, myopic but not emmetropic eyes showed an increase in mean arterial pressure, along with a greater choroidal blood flow response, suggesting that myopes had a significantly lower resting choroidal flow that was highly responsive to CO2 (125, 126). These results insinuate an altered autoregulation ability in myopic eyes that, due to the increase mean arterial pressure observed, may lie in an autonomic dysregulation. Interestingly, there is evidence that eyes with pathological myopia eyes have comparable retinal vascular reactivity, suggesting that the retinal oxygen consumption, but not the choroidal, is altered in high-myopic eyes (128).
7. Molecular and cellular evidence of vascular changes in myopia
In order to understand the nature of the architectural and functional vascular described in myopia, it is important to comprehend the molecular and cellular changes taking place. The most extensive genetic myopia pathway analyses to date are clinical studies (meta-GWAS from 23andMe and the Consortium of Refractive Error And Myopia, CREAM) (129–134) and studies in common marmosets (Callithrix jacchus), a well-established non-human primate model of myopia (18).
Genetic meta-GWAS studies using human specimens have identified several signaling pathways involved in myopia, many of which were previously known, such as the extracellular matrix and ion channel pathways, while others were new, like those involved in angiogenesis. The following genes have been recently identified in meta-GWAS studies and found to have a role in vascular homeostasis: BMP2 and BMP4, CD34, CD55, Flt-1 and TGF-β receptor 1. BMP2 and BMP4 bone morphogenetic proteins (BMPs), named for their bone and cartilage formation ability (135, 136), are increasingly recognized as multifunctional regulators of angiogenesis. BMP2 has a pathological role in the development of vascular inflammation (137, 138), and induces retinal endothelial cell barrier dysfunction in diabetic macular edema and pathological retinal neovascularization (135). Over-expression of BMP4 inhibits experimental choroidal neovascularization by modulating VEGF and MMP-9 (139). CD34 is a transmembrane protein expressed in endothelial cells that promotes the formation of invasive vessels during neovascularization (140). The membrane-bound complement regulator CD55 is highly expressed in the retinal vascular endothelium (141), and significantly decreased in hyperoxic retinas (142). VEGF receptor–1 (also known as Flt-1) is needed for adequate blood vessel patterning on retinal astrocytes and can modulate VEGF-A activation of endothelial cells (143, 144). Flt-1 has unique and important roles in coordinating endothelial sprouting (145, 146), blood vessel anastomosis (147), and genetic loss of flt-1 leading to vascular overgrowth and reduced network complexity (148). TGF-β receptor 1 inhibits and deep vascular plexus formation (149, 150), and its endothelial loss leads to aberrant contractile pericyte differentiation and hemorrhagic vascular malformations, and is essential for maintaining the integrity of mature vessels (151).
The analysis of retinal transcriptomes in marmosets induced with myopia has identified major molecular pathways activated during myopic eye growth (18). Some of the key pathways described are involved in vascular signaling and include the beta-adrenergic pathway, cyclic adenosine monophosphate (cAMP), Ca2+, relaxin (152), G protein-coupled receptor and nNOS. Beta-adrenergic signaling, for instance, is involved in hypoxia (153); there is a 90% increase in noradrenaline levels during hypoxia (154, 155), and beta-adrenorceptor activation is followed by an upregulation of hypoxia-inducible factor-1α (HIF-1α) and vascular endothelial growth factor (VEGF), both involved in neovascularization (156, 157). The cAMP pathway regulates neuronal, vascular, and inflammatory components of diabetic retinopathy (158). Calcium signaling is involved in capillary - but not arteriole - retinal blood flow as seen by the active dilation observed following astroglial Ca2+ signaling (159, 160). Relaxin, a peptide found at high concentrations during pregnancy, is found in endothelial and smooth muscle cells in arteries and veins, supporting its vasodilating role (161–163). G protein-coupled receptors 91 and 81 (GPR91and GPR81), localized in ganglion cells and Muller cells respectively, are involved in the pathogenesis of diabetic retinopathy (DR) and hypoxic retinal diseases such as retinopathy of prematurity (ROP), inner vascular network development and restoration of the vasculature in response to injury (152, 164). The neuronal isoform of nitric oxide (nNOS) is present in the vascular endothelium and contributes to the maintenance of homeostasis in the cardiovascular system. NO contributes to both retinal and choroidal neovascularization (165).
In terms of vascular cellular anatomy, the neurovascular interplay between neuronal, vascular, and glial cells, which is crucial for retinal structural and nutritional support and ion and neurotransmitter homeostasis, appears to be affected by myopia. Marmosets with induced myopia show a lower astrocyte density, increased GFAP-immunopositive staining, lower peripheral capillary branching, and increased numbers of string vessels compared to controls. These changes suggest an activation and reorganization of the astrocyte and vascular templates during myopia development and progression (166). Whether or not these adaptations are beneficial or harmful to the developing retina remains to be investigated.
8. Myopia-associated conditions showing vascular alterations
Myopic macular degeneration, glaucoma, choroidal neovascularization, retinal detachment, posterior staphyloma and cataract amongst the most prevalent myopia complications (167). In this section we review the vascular features of these myopia-associated conditions to help understand the vascular nature of myopia.
8.1. Myopic macular degeneration
Myopic maculopathy is the most common cause of vision loss in myopic eyes (5, 168), but its etiology remains unclear. The distinctive elongation and deformation of the myopic eye, along with its characteristic neovascularization, suggest that vascular pathways likely contribute to the degenerative process. Evidence for a vascular etiology of myopic maculopathy include a significant choroid thinning, enlarged foveal avascular zone, smaller choriocapillaris flow area, vascular dropout, lower fractal dimension, and a more profound decrease in deep but not superficial retinal capillary plexus density, suggesting that microvascular alterations appear crucial for myopic maculopathy (169–171). In addition, choroidal watershed zones, which are areas situated at the edge of end-arteries usually exhibiting a delayed choroidal filling, have been proposed to play a vascular role in the etiology of myopic maculopathy (172).
8.2. Glaucoma
Glaucoma is a complex neuropathy that preferentially affects the ganglion cell complex (GCC) (173) and exhibits features of vascular etiology and dysfunction (174). The relationship between myopia and glaucoma has been confirmed by several large population-based studies (175–180). Yet, the nature of the myopia-glaucoma relationship remains unknown. Low ocular perfusion has been identified as a risk factor for glaucoma progression independent of intraocular pressure (181–183). Both glaucomatous and myopic eyes show parallel vascular changes: retinal microvasculature attenuation (184), decreased capillary density (63, 66, 184–186), and reduced retinal and ONH blood flow and vascular dysregulation (56, 57, 65, 68, 89, 91, 187–189). In fact, the longer the axial eye length and the thinner the ocular wall in glaucoma patients, the greater the retinal microcirculation reduction (190). In addition, when the vascular features of glaucomatous patients with and without myopia were compared, myopic glaucomatous eyes exhibited greater vascular changes than non-myopic glaucomatous eyes: larger reductions in choroidal blood flow and velocity (191), lower macular and peripapillary capillary density (184, 192), and impaired peripapillary vasoreactivity (127). Therefore, it has been hypothesized that the relationship between myopia and glaucoma might partly be vascular in nature, specifically microvascular, and may be present before the glaucomatous degeneration is evident. In addition, the study of vascular reactivity in glaucomatous patients with and without high myopia has confirmed that the retinal vasoreactivity of the peripapillary capillaries is compromised in glaucomatous eyes with high myopia (127).
8.3. Choroidal neovascularization
Choroidal neovascularization (CNV) is characterized by an atypical choroidal vasculature growth into the retinal pigment epithelium potentially leading to fluid and blood accumulation in the macula (50). Eyes with lower foveolar choroidal blood volume and flow have been identified to be at a higher risk of developing CNV. This reduced choroidal blood supply appears greater than any changes observed in eyes without CNV, suggesting that alterations in the foveal choroidal circulation might precede be part of CNV etiology (193). In addition, the choroidal thinning and capillary density reduction observed in degenerative pathological myopia is believed to trigger RPE and glial cells hypoxia, resulting in an upregulation of VEGF expression (194).
8.4. Retinal detachment
The most prevalent form of neurosensory retina separation from the retinal pigment epithelium (RPE) is the rhegmatogenous retinal detachment. This type of detachment disrupts the retinal vasculature leading to smaller vascular diameters, tortuosity, and vascular density (195). Capillary dilatation, hyperpermeability and fluorescein leakage have also been observed with fluorescein angiography on the detached retina, and have been proposed to originate from tissue hypoxia (196, 197).
8.5. Posterior staphyloma
Peripapillary posterior staphyloma (PPS) is one of six types of posterior staphylomas identified in degenerative myopia (198). Eyes with PPS have an increased macular vessel density in the deep plexus, reduced macular choriocapillaris and radial peripapillary capillary density, and thinner choroids (199). In addition, a retrospective study also identified reduced choriocapillaris flow and thinner subfoveal choroidal thickness, confirming that eyes with posterior staphyloma have thinner choroids and lower perfusion (200).
8.6. Cataract
A relationship between lens opacity and hypertension was identified in the initial cross-sectional phase of the Beaver Dam Eye Study (201). However, this relationship was not confirmed once the longitudinal 5-year Beaver Dam Eye Study was completed (202). There is, however, evidence of lower ocular blood velocity in cataract patients that requires further evaluation to understand the nature of the changes (203).
9. Conclusion
Adequate blood flow is fundamental for tissue homeostasis (204). In view of the findings described in the literature and discussed in this review, studying the haemodynamics and vascular autoregulation features of healthy myopic eyes may be crucial to identify early markers of associated degeneration and help develop novel vascular interventions to preserve the health of myopic eyes.
Author contributions
AB-P conceived, designed, wrote and reviewed the manuscript.
Acknowledgments
The author would like to acknowledge William Bourassa for his invaluable assistance with graphics and manuscript editing.
Conflict of interest
The author declares that the research was conducted in the absence of any commercial or financial relationships that could be construed as a potential conflict of interest.
Publisher’s note
All claims expressed in this article are solely those of the authors and do not necessarily represent those of their affiliated organizations, or those of the publisher, the editors and the reviewers. Any product that may be evaluated in this article, or claim that may be made by its manufacturer, is not guaranteed or endorsed by the publisher.
References
1. Ohno-Matsui, K, Akiba, M, Ishibashi, T, and Moriyama, M. Observations of vascular structures within and posterior to sclera in eyes with pathologic myopia by swept-source optical coherence tomography. Invest Ophthalmol Vis Sci. (2012) 53:7290–8. doi: 10.1167/iovs.12-10371
2. Vongphanit, J, Mitchell, P, and Wang, JJ. Prevalence and progression of myopic retinopathy in an older population. Ophthalmology. (2002) 109:704–11. doi: 10.1016/S0161-6420(01)01024-7
3. Saw, SM . How blinding is pathological myopia? Br J Ophthalmol. (2006) 90:525–6. doi: 10.1136/bjo.2005.087999
4. Ruiz-Moreno, JM, Arias, L, Montero, JA, Carneiro, A, and Silva, R. Intravitreal anti-Vegf therapy for choroidal neovascularisation secondary to pathological myopia: 4-year outcome. Br J Ophthalmol. (2013) 97:1447–50. doi: 10.1136/bjophthalmol-2012-302973
5. Neelam, K, Cheung, CM, Ohno-Matsui, K, Lai, TY, and Wong, TY. Choroidal neovascularization in pathological myopia. Prog Retin Eye Res. (2012) 31:495–525. doi: 10.1016/j.preteyeres.2012.04.001
6. Mutti, DO, Semina, E, Marazita, M, Cooper, M, Murray, JC, and Zadnik, K. Genetic loci for pathological myopia are not associated with juvenile myopia. Am J Med Genet. (2002) 112:355–60. doi: 10.1002/ajmg.10683
7. Morgan, IG, Ohno-Matsui, K, and Saw, SM. Myopia. Lancet. (2012) 379:1739–48. doi: 10.1016/s0140-6736(12)60272-4
8. Ohno-Matsui, K, Kawasaki, R, Jonas, JB, Cheung, CMG, Saw, S-M, Verhoeven, VJM, et al. International photographic classification and grading system for myopic maculopathy. Am J Ophthalmol. (2015) 159:877–83.e7. doi: 10.1016/j.ajo.2015.01.022
9. Curtin, BJ . The Myopias: Basic Science and Clinical Management. Philadelphia: Harper and Row (1985).
10. Vutipongsatorn, K, Yokoi, T, and Ohno-Matsui, K. Current and emerging pharmaceutical interventions for myopia. Br J Ophthalmol. (2019) 103:1539. doi: 10.1136/bjophthalmol-2018-313798
11. Holden, BA, Fricke, TR, Wilson, DA, Jong, M, Naidoo, KS, Sankaridurg, P, et al. Global prevalence of myopia and high myopia and temporal trends from 2000 through 2050. Ophthalmology. (2016) 123:1036–42. doi: 10.1016/j.ophtha.2016.01.006
12. Smith, EL III . Prentice award lecture 2010: a case for peripheral optical treatment strategies for myopia. Optom Vis Sci. (2011) 88:1029–44. doi: 10.1097/OPX.0b013e3182279cfa
13. Faria-Ribeiro, M, Queirós, A, Lopes-Ferreira, D, Jorge, J, and González-Méijome, JM. Peripheral refraction and retinal contour in stable and progressive myopia. Optom Vis Sci. (2013) 90:9–15. doi: 10.1097/OPX.0b013e318278153c
14. Ashby, RS, and Schaeffel, F. The effect of ambient illuminance on the development of deprivation myopia in chicks. Invest Ophthalmol Vis Sci. (2009) 50:5348–54. doi: 10.1167/iovs.09-3419
15. Smith, EL III, Hung, LF, and Huang, J. Effects of high ambient lighting on the development of form-deprivation myopia in infant Rhesus monkeys. Invest Ophthalmol Vis Sci. (2012) 53:421–8. doi: 10.1167/iovs.11-8652
16. Rose, KA, Morgan, IG, Ip, J, Kifley, A, Huynh, S, Smith, W, et al. Outdoor activity reduces the prevalence of myopia in children. Ophthalmol. (2008) 115:1279–85. doi: 10.1016/j.ophtha.2007.12.019
17. Dirani, M, Tong, L, Gazzard, G, Zhang, X, Chia, A, Young, TL, et al. Outdoor activity and myopia in Singapore teenage children. Br J Ophthalmol. (2009) 93:997–1000. doi: 10.1136/bjo.2008.150979
18. Tkatchenko, TV, Troilo, D, Benavente-Perez, A, and Tkatchenko, AV. Gene expression in response to optical defocus of opposite signs reveals bidirectional mechanism of visually guided eye growth. PLoS Biol. (2018) 16:e2006021. doi: 10.1371/journal.pbio.2006021
19. Chakraborty, R, Park, HN, Hanif, AM, Sidhu, CS, Iuvone, PM, and Pardue, MT. On pathway mutations increase susceptibility to form-deprivation myopia. Exp Eye Res. (2015) 137:79–83. doi: 10.1016/j.exer.2015.06.009
20. Forrester, JV, Dick, AD, McMenamin, PG, Roberts, F, and Pearlman, E. The Eye: Basic Sciences in Practice. Edinburgh, United Kingdom: Elsevier (2001).
21. Yu, DY, and Cringle, SJ. Oxygen distribution and consumption within the retina in vascularised and avascular retinas and in animal models of retinal disease. Prog Retin Eye Res. (2001) 20:175–208. doi: 10.1016/S1350-9462(00)00027-6
22. Hayreh, SS In: D Dartt , editor. Encyclopedia of the Eye. United States: Academic Press (2010). 43:1–8.
23. Delaey, C, and Van de Voorde, J. Regulatory mechanisms in the retinal and choroidal circulation. Ophthalmic Res. (2000) 32:249–56. doi: 10.1159/000055622
24. Flammer, J, Orgüla, S, Costab, V, Orzalesic, N, Krieglsteind, G, Metzner Serrae, K, et al. The impact of ocular blood flow in glaucoma. Prog Retin Eye Res. (2002) 21:359–93. doi: 10.1016/S1350-9462(02)00008-3
25. Bron, A, Tripathi, R, and Tripathi, B. Wolff's Anatomy of the Eye and Orbit. 8th ed. London: Chapman & Hall Medical (1997).
26. Hayreh, SS . Blood flow in the optic nerve head and factors that may influence it. Prog Retin Eye Res. (2001) 20:595–624. doi: 10.1016/S1350-9462(01)00005-2
27. Akyol, N, Kukner, A, Ozdemir, T, and Esmerligil, S. Choroidal and retinal blood flow changes in degenerative myopia. Can J Ophthalmol. (1996) 31:113–9.
28. Dimitrova, G, Tamaki, Y, Kato, S, and Nagahara, M. Retrobulbar circulation in myopic patients with or without myopic choroidal neovascularisation. Br J Ophthalmol. (2002) 86:771–3. doi: 10.1136/bjo.86.7.771
29. Wu, H, Seet, B, Yap, E, Saw, S, Lim, T, and Chia, K. Does education explain ethnic differences in myopia prevalence? A population-based study of Young adult males in Singapore. Optom Vis Sci. (2001) 78:234–9. doi: 10.1097/00006324-200104000-00012
30. Fitzgerald, M, Wildsoe, C, and Anton, R. Temporal relationship of choroidal blood flow and thickness changes during recovery from form deprivation myopia in chicks. Exp Eye Res. (2001) 74:561–70. doi: 10.1006/exer.2002.1142
31. Fitzgerald, MEC, Tolley, E, Jackson, B, Zagvazdin, YS, Cuthbertson, SL, Hodos, W, et al. Anatomical and functional evidence for progressive age-related decline in parasympathetic control of choroidal blood flow in pigeons. Exp Eye Res. (2005) 81:478–91. doi: 10.1016/j.exer.2005.03.008
32. Wildsoet, C, and Wallman, J. Choroidal and scleral mechanisms of compensation for spectacle lenses in chicks. Vis Res. (1995) 35:1175–94. doi: 10.1016/0042-6989(94)00233-C
33. Jin, N, and Stjernschantz, J. Regional blood flow in the myopic Chick eye during and after form deprivation: a study with radioactively-labelled microspheres. Exp Eye Res. (2000) 71:233–8. doi: 10.1006/exer.2000.0871
34. Riva, C, Ross, B, and Benedek, GB. Laser Doppler measurements of blood flow in capillary tubes and retinal arteries. Investig Ophthalmol. (1972) 11:936–44.
35. Olafsdottir, OB, Eliasdottir, TS, Kristjansdottir, JV, Hardarson, SH, and Stefánsson, E. Retinal vessel oxygen saturation during 100% oxygen breathing in healthy individuals. PLoS One. (2015) 10:e0128780. doi: 10.1371/journal.pone.0128780
36. de Carlo, TE, Romano, A, Waheed, NK, and Duker, JS. A review of optical coherence tomography angiography (Octa). Int J Retina Vitreous. (2015) 1:5. doi: 10.1186/s40942-015-0005-8
37. Mo, S, Krawitz, B, Efstathiadis, E, Geyman, L, Weitz, R, Chui, TY, et al. Imaging foveal microvasculature: optical coherence tomography angiography versus adaptive optics scanning light ophthalmoscope fluorescein angiography. Invest Ophthalmol Vis Sci. (2016) 57:130–40. doi: 10.1167/iovs.15-18932
38. Senarathna, J, Rege, A, Li, N, and Thakor, NV. Laser speckle contrast imaging: theory, instrumentation and applications. IEEE Rev Biomed Eng. (2013) 6:99–110. doi: 10.1109/RBME.2013.2243140
39. Zion, IB, Harris, A, Siesky, B, Shulman, S, McCranor, L, and Garzozi, HJ. Pulsatile ocular blood flow: relationship with flow velocities in vessels supplying the retina and choroid. Br J Ophthalmol. (2007) 91:882–4. doi: 10.1136/bjo.2006.108340
40. Garhofer, G, Werkmeister, R, Dragostinoff, N, and Schmetterer, L. Retinal blood flow in healthy Young subjects. Invest Ophthalmol Vis Sci. (2012) 53:698–703. doi: 10.1167/iovs.11-8624
41. Riva, CE, Grunwald, JE, Sinclair, SH, and Petrig, BL. Blood velocity and volumetric flow rate in human retinal vessels. Invest Ophthalmol Vis Sci. (1985) 26:1124–32.
42. Leitgeb, RA, Werkmeister, RM, Blatter, C, and Schmetterer, L. Doppler optical coherence tomography. Prog Retin Eye Res. (2014) 41:26–43. doi: 10.1016/j.preteyeres.2014.03.004
43. Emeterio Nateras, OS, Harrison, JM, Muir, ER, Zhang, Y, Peng, Q, Chalfin, S, et al. Choroidal blood flow decreases with age: an Mri study. Curr Eye Res. (2014) 39:1059–67. doi: 10.3109/02713683.2014.892997
44. Burgansky-Eliash, Z, Lowenstein, A, Neuderfer, M, Kesler, A, Barash, H, Nelson, DA, et al. The correlation between retinal blood flow velocity measured by the retinal function imager and various physiological parameters. Ophthalmic Surg Lasers Imaging Retina. (2013) 44:51–8. doi: 10.3928/23258160-20121221-13
45. Yu, J, Jiang, C, Wang, X, Zhu, L, Gu, R, Xu, H, et al. Macular perfusion in healthy Chinese: an optical coherence tomography angiogram study. Invest Ophthalmol Vis Sci. (2015) 56:3212–7. doi: 10.1167/iovs.14-16270
46. Yu, J, Gu, R, Zong, Y, Xu, H, Wang, X, Sun, X, et al. Relationship between retinal perfusion and retinal thickness in healthy subjects: an optical coherence tomography angiography study. Invest Ophthalmol Vis Sci. (2016) 57:204–10. doi: 10.1167/iovs.15-18630
47. Lin, Y, Jiang, H, Liu, Y, Rosa Gameiro, G, Gregori, G, Dong, C, et al. Age-related alterations in retinal tissue perfusion and volumetric vessel density. Invest Ophthalmol Vis Sci. (2019) 60:685–93. doi: 10.1167/iovs.18-25864
48. Wei, Y, Jiang, H, Shi, Y, Qu, D, Gregori, G, Zheng, F, et al. Age-related alterations in the retinal microvasculature, microcirculation, and microstructure. Invest Ophthalmol Vis Sci. (2017) 58:3804–17. doi: 10.1167/iovs.17-21460
49. Fitzgerald, MEC, Tolley, E, Frase, S, Zagvazdin, Y, Miller, RF, Hodos, W, et al. Functional and morphological assessment of age-related changes in the choroid and outer retina in pigeons. Vis Neurosci. (2001) 18:299–317. doi: 10.1017/S0952523801182143
50. Chirco, KR, Sohn, EH, Stone, EM, Tucker, BA, and Mullins, RF. Structural and molecular changes in the aging choroid: implications for age-related macular degeneration. Eye (Lond). (2017) 31:10–25. doi: 10.1038/eye.2016.216
51. Kur, J, Newman, EA, and Chan-Ling, T. Cellular and physiological mechanisms underlying blood flow regulation in the retina and choroid in health and disease. Prog Retin Eye Res. (2012) 31:377–406. doi: 10.1016/j.preteyeres.2012.04.004
52. Avetisov, ES, and Savitskaya, NF. Some features of ocular microcirculation in myopia. Ann Ophthalmol. (1977) 9:1261–4.
53. Bal'zhanova, AB . Fluorescence angiography in assessing choroidal circulation in highly progressive myopia. Vestn oftalmol. (1978) 1:25–30.
54. Lim, LS, Cheung, CY, Lin, X, Mitchell, P, Wong, TY, and Mei-Saw, S. Influence of refractive error and axial length on retinal vessel geometric characteristic. Invest Ophthalmol Vis Sci. (2011) 52:669–78s. doi: 10.1167/iovs.10-6184
55. Sun, C, Chen, T, Cong, J, Wu, X, Wang, J, and Yuan, Y. Changes in retinal vascular bifurcation in eyes with myopia. BMC Ophthalmol. (2022) 22:408. doi: 10.1186/s12886-022-02629-y
56. Benavente-Perez, A, Hosking, SL, Logan, NS, and Broadway, DC. Ocular blood flow measurements in healthy human myopic eyes. Graefes Arch Clin Exp Ophthalmol. (2010) 248:1587–94. doi: 10.1007/s00417-010-1407-9
57. Shimada, N, Ohno-Matsui, K, Harino, S, Yoshida, T, Yasuzumi, K, Kojima, A, et al. Reduction of retinal blood flow in high myopia. Graefes Arch Clin Exp Ophthalmol. (2004) 242:284–8. doi: 10.1007/s00417-003-0836-0
58. Karczewicz, D, and Modrzejewska, M. Blood flow in eye arteries assessed by Doppler ultrasound in patients with myopia. Klin Ocz. (2004) 106:211–3.
59. Mamikonian, VR, Shmeleva-Demir, OA, Kharlap, SI, Andzhelova, DV, Kazarian, EE, Makashova, NV, et al. Hemodynamic changes in myopia of different degrees. Vestn oftalmol. (2013) 129:24–7.
60. Mo, J, Duan, A, Chan, S, Wang, X, and Wei, W. Vascular flow density in pathological myopia: an optical coherence tomography angiography study. BMJ Open. (2017) 7:e013571. doi: 10.1136/bmjopen-2016-013571
61. Yang, Y, Wang, J, Jiang, H, Yang, X, Feng, L, Hu, L, et al. Retinal microvasculature alteration in high myopia. Invest Ophthalmol Vis Sci. (2016) 57:6020–30. doi: 10.1167/iovs.16-19542
62. Li, Y, Miara, H, Ouyang, P, and Jiang, B. The comparison of regional Rnfl and fundus vasculature by Octa in Chinese myopia population. J Ophthalmol. (2018) 2018:10. doi: 10.1155/2018/3490962
63. Su, L, Ji, YS, Tong, N, Sarraf, D, He, X, Sun, X, et al. Quantitative assessment of the retinal microvasculature and Choriocapillaris in myopic patients using swept-source optical coherence tomography angiography. Graefes Arch Clin Exp Ophthalmol. (2020) 258:1173–80. doi: 10.1007/s00417-020-04639-2
64. Min, CH, Al-Qattan, HM, Lee, JY, Kim, J-G, Yoon, YH, and Kim, YJ. Macular microvasculature in high myopia without pathologic changes: an optical coherence tomography angiography study. Korean J Ophthalmol. (2020) 34:106–12. doi: 10.3341/kjo.2019.0113
65. Wang, X, Kong, X, Jiang, C, Li, M, Yu, J, and Sun, X. Is the Peripapillary retinal perfusion related to myopia in healthy eyes? A prospective comparative study. BMJ Open. (2016) 6:e010791. doi: 10.1136/bmjopen-2015-010791
66. Al-Sheikh, M, Phasukkijwatana, N, Dolz-Marco, R, Rahimi, M, Iafe, NA, Freund, KB, et al. Quantitative Oct angiography of the retinal microvasculature and the Choriocapillaris in myopic eyes. Invest Ophthalmol Vis Sci. (2017) 58:2063–9. doi: 10.1167/iovs.16-21289
67. Wang, T, Li, H, Zhang, R, Yu, Y, Xiao, X, and Wu, C. Evaluation of retinal vascular density and related factors in youth myopia without maculopathy using Octa. Sci Rep. (2021) 11:15361. doi: 10.1038/s41598-021-94909-8
68. Qu, D, Lin, Y, Jiang, H, Shao, Y, Shi, Y, Airen, S, et al. Retinal nerve Fiber layer (Rnfl) integrity and its relations to retinal microvasculature and microcirculation in myopic eyes. Eye Vis. (2018) 5:25. doi: 10.1186/s40662-018-0120-3
69. Lim, LS, Cheung, CY, Lin, X, Mitchell, P, Wong, TY, and Mei-Saw, S. Influence of refractive error and axial length on retinal vessel geometric characteristics. Invest Ophthalmol Vis Sci. (2011) 52:669–78. doi: 10.1167/iovs.10-6184
70. Man, RE, Sasongko, MB, Xie, J, Best, WJ, Noonan, JE, Lo, TC, et al. Decreased retinal capillary flow is not a mediator of the protective myopia-diabetic retinopathy relationship. Invest Ophthalmol Vis Sci. (2014) 55:6901–7. doi: 10.1167/iovs.14-15137
71. Wallman, J, Wildsoet, C, Xu, A, Gottlieb, MD, Nickla, D, Marran, L, et al. Moving the retina: choroidal modulation of refractive state. VR. (1995) 35:37–70.
72. Hirata, A, and Negi, A. Morphological changes of Choriocapillaris in experimentally induced Chick Myopa. Graefes Arch Clin Exp Ophthalmol. (1998) 236:132–7.
73. Fitzgerald, MEC, Wildsoet, CF, and Reiner, A. Choroidal blood flow and thickness changes during recovery from form deprivation myopia in chicks – are they causally related? ARVO. (1998) 39:S868.
74. Troilo, D, Nickla, DL, and Wildsoet, CW. Choroidal thickness changes during altered eye growth and refractive state in a primate. Investig Ophthalmol Vis Sci. (2000) 41:1249–58.
75. Shih, YF, Fitzgerald, MEC, Norton, TT, Gamlin, PDR, Hodos, W, and Reiner, A. Reduction in choroidal blood flow occurs in chicks wearing goggles that induce eye growth toward myopia. Curr Eye Res. (1993) 12:219–27.
76. Hirata, A, and Negi, A. Lacquer crack lesions in experimental Chick myopia. Graefes Arch Clin Exp Ophthalmol. (1998) 236:138–45.
77. Fitzgerald, MEC, Wildsoet, CF, and Reiner, A. Temporal relationship of choroidal blood flow and thickness changes during recovery from form deprivation myopia in chicks. Exp Eye Res. (2002) 74:561–70. doi: 10.1006/exer.2002.1142
78. Harb, E, Hyman, L, Gwiazda, J, Marsh-Tootle, W, Zhang, Q, Hou, W, et al. Choroidal thickness profiles in myopic eyes of Young adults in the correction of myopia evaluation trial cohort. Am J Ophthalmol. (2015) 160:62–71.e2. doi: 10.1016/j.ajo.2015.04.018
79. Hung, LF, Wallman, J, and Smith, EL. Vision-dependent changes in the choroidal thickness of macaque monkeys. Invest Ophthalmol Vis Sci. (2000) 41:1259–69.
80. Howlett, MH, and McFadden, SA. Spectacle Lens compensation in the pigmented Guinea pig. Vis Res. (2009) 49:219–27. doi: 10.1016/j.visres.2008.10.008
81. Read, SA, Alonso-Caneiro, D, Vincent, SJ, and Collins, MJ. Longitudinal changes in choroidal thickness and eye growth in childhood. Invest Ophthalmol Vis Sci. (2015) 56:3103–12. doi: 10.1167/iovs.15-16446
82. Read, SA, Alonso-Caneiro, D, Vincent, SJ, and Collins, MJ. Peripapillary choroidal thickness in childhood. Exp Eye Res. (2015) 135:164–73. doi: 10.1016/j.exer.2015.03.002
83. Liu, L, Zhu, C, Yuan, Y, Hu, X, Chen, C, Zhu, H, et al. Three-dimensional choroidal vascularity index in high myopia using swept-source optical coherence tomography. Curr Eye Res. (2022) 47:484–92. doi: 10.1080/02713683.2021.2006236
84. Alshareef, RA, Khuthaila, MK, Goud, A, Vupparaboina, KK, Jana, S, and Chhablani, J. Subfoveal choroidal vascularity in myopia: evidence from spectral-domain optical coherence tomography. Ophthalmic Surg Lasers Imaging Retina. (2017) 48:202–7. doi: 10.3928/23258160-20170301-02
86. Berisha, F, Findl, O, Lasta, M, Kiss, B, and Schmetterer, L. A study comparing ocular pressure pulse and ocular fundus pulse in dependence of axial eye length and ocular volume. Acta Ophthalmol. (2010) 88:766–72. doi: 10.1111/j.1755-3768.2009.01577.x
87. James, CB, Trew, DR, Clark, K, and Smith, SE. Factors influencing the ocular pulse – axial length. Graefes Arch Clin Exp Ophthalmol. (1991) 229:341–4. doi: 10.1007/bf00170692
88. Zhang, S, Zhang, G, Zhou, X, Xu, R, Wang, S, Guan, Z, et al. Changes in choroidal thickness and choroidal blood perfusion in Guinea pig myopia. Invest Ophthalmol Vis Sci. (2019) 60:3074–83. doi: 10.1167/iovs.18-26397
89. Scherm, P, Pettenkofer, M, Maier, M, Lohmann, CP, and Feucht, N. Choriocapillary blood flow in myopic patients measured with Oct angiography. Invest Ophthalmol Vis Sci. (2018) 59:2821.
90. Ning, J, Joshi, N, Franchi-Pereira, R, and Benavente-Perez, A. Longitudinal evaluation of choroidal thickness and ocular perfusion pressure in progressing Myopes, baseline data. Invest Ophthalmol Vis Sci. (2017) 58:1106.
91. Cheng, W, Song, Y, Gao, X, Lin, F, Li, F, Wang, P, et al. Axial length and Choriocapillaris flow deficits in non-pathological high myopia. Am J Ophthalmol. (2022) 244:68–78. doi: 10.1016/j.ajo.2022.08.005
92. Wang, Y, Chen, S, Lin, J, Chen, W, Huang, H, Fan, X, et al. Vascular changes of the choroid and their correlations with visual acuity in pathological myopia. Invest Ophthalmol Vis Sci. (2022) 63:20. doi: 10.1167/iovs.63.12.20
93. Zhang, Z, Qi, Y, Wei, W, Jin, Z-B, Wang, W, Duan, A, et al. Investigation of macular choroidal thickness and blood flow change by optical coherence tomography angiography after posterior scleral reinforcement. Front Med. (2021):8. doi: 10.3389/fmed.2021.658259
94. Lim, LS, Lim, XH, and Tan, L. Retinal vascular oxygen saturation and its variation with refractive error and axial length. Transl Vis Sci Technol. (2019) 8:22. doi: 10.1167/tvst.8.4.22
95. Liu, X, He, X, Yin, Y, Zhang, B, Sun, S, Zhu, J, et al. Retinal oxygen saturation in 1461 healthy children aged 7-19 and its associated factors. Acta Ophthalmol. (2019) 97:287–95. doi: 10.1111/aos.14043
96. Zheng, Q, Zong, Y, Li, L, Huang, X, Lin, L, Yang, W, et al. Retinal vessel oxygen saturation and vessel diameter in high myopia. Ophthalmic Physiol Opt. (2015) 35:562–9. doi: 10.1111/opo.12223
97. Liu, Y, Wang, L, Xu, Y, Pang, Z, and Mu, G. The influence of the choroid on the onset and development of myopia: from perspectives of choroidal thickness and blood flow. Acta Ophthalmol. (2021) 99:730–8. doi: 10.1111/aos.14773
98. Brown, DM, Mazade, R, Clarkson-Townsend, D, Hogan, K, Datta Roy, PM, and Pardue, MT. Candidate pathways for retina to scleral signaling in refractive eye growth. Exp Eye Res. (2022) 219:109071. doi: 10.1016/j.exer.2022.109071
99. Wu, H, Chen, W, Zhao, F, Zhou, Q, Reinach, PS, Deng, L, et al. Scleral hypoxia is a target for myopia control. Proc Natl Acad Sci. (2018) 115:E7091–100. doi: 10.1073/pnas.1721443115
100. Zhao, F, Zhang, D, Zhou, Q, Zhao, F, He, M, Yang, Z, et al. Scleral Hif-1α is a prominent regulatory candidate for genetic and environmental interactions in human myopia pathogenesis. EBioMedicine. (2020) 57:102878. doi: 10.1016/j.ebiom.2020.102878
101. Guo, L, Frost, MR, Siegwart, JT Jr, and Norton, TT. Gene expression signatures in tree shrew sclera during recovery from minus-Lens Wear and during plus-Lens Wear. Mol Vis. (2019) 25:311–28.
102. Guo, L, Frost, MR, He, L, Siegwart, JT Jr, and Norton, TT. Gene expression signatures in tree shrew sclera in response to three Myopiagenic conditions. Invest Ophthalmol Vis Sci. (2013) 54:6806–19. doi: 10.1167/iovs.13-12551
103. Benavente-Perez, A, Hosking, SL, Logan, NS, and Bansal, D. Reproducibility-repeatability of choroidal thickness calculation using Oct. Optom Vis Sci. (2010) 87:867–72. doi: 10.1097/OPX.0b013e3181f3eced
104. Goldenberg, D, Moisseiev, E, Goldstein, M, Loewenstein, A, and Barak, A. Enhanced depth imaging optical coherence tomography: choroidal thickness and correlations with age, refractive error, and axial length. Ophthalmic Surg Lasers Imaging. (2012) 43:296–301. doi: 10.3928/15428877-20120426-02
105. Li, XQ, Larsen, M, and Munch, IC. Subfoveal choroidal thickness in relation to sex and axial length in 93 Danish university students. Invest Ophthalmol Vis Sci. (2011) 52:8438–41. doi: 10.1167/iovs.11-8108
106. Agawa, T, Miura, M, Ikuno, Y, Makita, S, Fabritius, T, Iwasaki, T, et al. Choroidal thickness measurement in healthy Japanese subjects by three-dimensional high-penetration optical coherence tomography. Graefes Arch Clin Exp Ophthalmol. (2011) 249:1485–92.
107. Ohno-Matsui, K, Akiba, M, Modegi, T, Tomita, M, Ishibashi, T, Tokoro, T, et al. Association between shape of sclera and myopic Retinochoroidal lesions in patients with pathologic myopia. Invest Ophthalmol Vis Sci. (2012) 53:6046–61. doi: 10.1167/iovs.12-10161
108. Ohno-Matsui, K, Akiba, M, Moriyama, M, Ishibashi, T, Tokoro, T, and Spaide, RF. Imaging Retrobulbar subarachnoid space around optic nerve by swept-source optical coherence tomography in eyes with pathologic myopia. Invest Ophthalmol Vis Sci. (2011) 52:9644–50. doi: 10.1167/iovs.11-8597
109. Riva, C . Rhythmic changes in velocity, volume and blood flow in the optic nerve head tissue. Microvasc Res. (1990) 40:36–45. doi: 10.1016/0026-2862(90)90005-C
110. Hafez, A, Bizzarro, R, Rivard, M, Trabut, I, Lovasik, J, Kergoat, H, et al. Reproducibility of retinal and optic nerve head perfusion measurements using scanning laser Doppler Flowmetry. Ophthalmic Surg Lasers Imaging. (2003) 34:422–32. doi: 10.3928/1542-8877-20030901-18
111. Schemetterer, L, Lexer, F, Findl, LO, Graselli, U, Eichler, H, and Woltz, M. The effect of inhalation of different mixtures of O2and Co2on ocular fundus pulsations. Exp Eye Res. (1996) 63:351–5. doi: 10.1006/exer.1996.0125
112. Schmetterer, L . Noninvasive investigations of the Normal ocular circulation in humans. Invest Ophthalmol Vis Sci. (1998) 39:1210.
113. Hosking, SL, Harris, A, Chung, HS, Jonescu-Cuypers, CP, Kagemann, L, Roff Hilton, EJ, et al. Ocular Haemodynamic responses to induced hypercapnia and Hyperoxia in Glaucoma. Br J Ophthalmol. (2004) 88:406–11. doi: 10.1136/bjo.2002.008995
114. Embleton, S, Hosking, S, Roff Hilton, E, and Cunliffe, I. Effect of senescence on ocular blood flow in the retina, neuroretinal rim and lamina cribrosa, using scanning laser Doppler flowmetry. Eye (Lond). (2002) 16:156–62. doi: 10.1038/sj.eye.6700100
115. Logan, N, Gilmartin, B, and Cox, W. Ocular Volume and Blood Flow in Human Anisomyopia. Invest Ophthalmol Vis Sci. (2002) 43:199.
116. Guyton, AC . Evidence for tissue oxygen demand as the major factor causing autoregulation. Circ Res. (1964) 15:60–9.
117. Riva, CE, Grunwald, JE, and Petrig, BL. Autoregulation of human retinal blood flow. An investigation with laser Doppler velocimetry. Invest Ophthalmol Vis Sci. (1986) 27:1706–12.
118. Michelson, G, Groh, M, and Gründler, A. Regulation of ocular blood flow during increases of arterial blood pressure. Br J Ophthalmol. (1994) 78:461–5. doi: 10.1136/bjo.78.6.461
119. Kergoat, H, and Faucher, C. Effects of oxygen and Carbogen breathing on choroidal hemodynamics in humans. Invest Ophthalmol Vis Sci. (1999) 40:2906–11.
120. Schumann, J, Orgül, S, Gugleta, K, Dubler, B, and Flammer, J. Interocular difference in progression of Glaucoma correlates with Interocular differences in Retrobulbar circulation. Am J Ophthalmol. (2000) 129:728–33. doi: 10.1016/S0002-9394(99)00481-X
121. Aaslid, R, Lindegaard, KF, Sorteberg, W, and Nornes, H. Cerebral autoregulation dynamics in humans. Stroke. (1989) 20:45–52. doi: 10.1161/01.STR.20.1.45
122. Riva, C, Hero, M, Titze, P, and Petrig, B. Autoregulation of human optic nerve head blood flow in response to acute changes in ocular perfusion pressure. Graefes Arch Clin Exp Ophthalmol. (1997) 235:618–26. doi: 10.1007/BF00946937
123. Longo, A, Geiser, M, and Riva, C. Posture changes and subfoveal choroidal blood flow. Investig Ophthalmol Vis Sci. (2004) 45:546–51. doi: 10.1167/iovs.03-0757
124. Riva, CE, Logean, E, and Falsini, B. Visually evoked Hemodynamical response and assessment of neurovascular coupling in the optic nerve and retina. Prog Retin Eye Res. (2005) 24:183–215. doi: 10.1016/j.preteyeres.2004.07.002
125. Benavente-Perez, A, Hosking, SL, and Logan, NS. Defective haemodynamic autoregulation associated with autonomic dysregulation in human myopia. Invest Ophthalmol Vis Sci. (2007) 48:1041.
126. Benavente-Perez, A, Hosking, SL, and Logan, NS. Myopes exhibit reduced choroidal blood velocity which is highly responsive to hypercapnia. Invest Ophthalmol Vis Sci. (2008) 49:3581.
127. Fan, X, Xu, H, Zhai, R, Sheng, Q, Sun, Y, Shao, T, et al. Peripapillary vascular reactivity in primary open-angle Glaucoma with high myopia by using optical coherence tomography angiography. Front Med. (2022) 9:850483. doi: 10.3389/fmed.2022.850483
128. La Spina, C, Corvi, F, Bandello, F, and Querques, G. Static characteristics and dynamic functionality of retinal vessels in longer eyes with or without pathologic myopia. Graefes Arch Clin Exp Ophthalmol. (2016) 254:827–34. doi: 10.1007/s00417-015-3122-z
129. Tedja, MS, Wojciechowski, R, Hysi, PG, Eriksson, N, Furlotte, NA, Verhoeven, VJM, et al. Genome-wide association Meta-analysis highlights light-induced signaling as a driver for refractive error. Nat Genet. (2018) 50:834–48. doi: 10.1038/s41588-018-0127-7
130. Tedja, MS, Haarman, AEG, Meester-Smoor, MA, Kaprio, J, Mackey, DA, Guggenheim, JA, et al. Imi–myopia genetics report. Invest Ophthalmol Vis Sci. (2019) 60:M89–M105. doi: 10.1167/iovs.18-25965
131. Verhoeven, VJ, Hysi, PG, Wojciechowski, R, Fan, Q, Kiefer, AK, Klaver, CCW, et al. Genome-wide mega-analysis on myopia and refractive error in Cream and 23andme. Invest Ophthalmol Vis Sci. (2014) 55:839.
132. Verhoeven, VJM, Hysi, PG, Wojciechowski, R, Fan, Q, Guggenheim, JA, Höhn, R, et al. Genome-wide Meta-analyses of multiancestry cohorts identify multiple new susceptibility loci for refractive error and myopia. Nat Genet. (2013) 45:314–8. doi: 10.1038/ng.2554
133. Kiefer, AK, Tung, JY, Do, CB, Hinds, DA, Mountain, JL, Francke, U, et al. Genome-wide analysis points to roles for extracellular matrix remodeling, the visual cycle, and neuronal development in myopia. PLoS Genet. (2013) 9:e1003299. doi: 10.1371/journal.pgen.1003299
134. Haarman, AEG, Tedja, MS, Meester-Smoor, MA, Kaprio, J, Mackey, DA, Guggenheim, JA, et al. Consortium for refractive error and myopia (Cream): vision, Mission, and accomplishments In: G Prakash and T Iwata, editors. Advances in Vision Research, Volume III: Genetic Eye Research Around the Globe. Singapore: Springer Singapore (2021). 381–407.
135. Al-Shabrawey, M, Hussein, K, Wang, F, Wan, M, Elmasry, K, Elsherbiny, N, et al. Bone morphogenetic Protein-2 induces non-canonical inflammatory and oxidative pathways in human retinal endothelial cells. Front Immunol. (2021):11. doi: 10.3389/fimmu.2020.568795
136. Nasrabadi, D, Rezaeiani, S, Eslaminejad, MB, and Shabani, A. Improved protocol for Chondrogenic differentiation of bone marrow derived mesenchymal stem cells-effect of Pthrp and Fgf-2 on Tgfβ1/Bmp2-induced chondrocytes hypertrophy. Stem Cell Rev Rep. (2018) 14:755–66. doi: 10.1007/s12015-018-9816-y
137. Simões Sato, AY, Bub, GL, and Campos, AH. Bmp-2 and-4 produced by vascular smooth muscle cells from atherosclerotic lesions induce monocyte chemotaxis through direct Bmprii activation. Atherosclerosis. (2014) 235:45–55. doi: 10.1016/j.atherosclerosis.2014.03.030
138. Helbing, T, Rothweiler, R, Ketterer, E, Goetz, L, Heinke, J, Grundmann, S, et al. Bmp activity controlled by Bmper regulates the Proinflammatory phenotype of endothelium. Blood. (2011) 118:5040–9. doi: 10.1182/blood-2011-03-339762
139. Xu, J, Zhu, D, Sonoda, S, He, S, Spee, C, Ryan, SJ, et al. Over-expression of Bmp4 inhibits experimental choroidal neovascularization by modulating Vegf and Mmp-9. Angiogenesis. (2012) 15:213–27. doi: 10.1007/s10456-012-9254-4
140. Siemerink, MJ, Hughes, MR, Dallinga, MG, Gora, T, Cait, J, Vogels, IM, et al. Cd34 promotes pathological epi-retinal neovascularization in a mouse model of oxygen-induced retinopathy. PLoS One. (2016) 11:e0157902. doi: 10.1371/journal.pone.0157902
141. Zauhar, R, Biber, J, Jabri, Y, Kim, M, Hu, J, Kaplan, L, et al. As in real estate, location matters: cellular expression of complement varies between macular and peripheral regions of the retina and supporting tissues. Front Immunol. (2022):13. doi: 10.3389/fimmu.2022.895519
142. Kim, C, Smith, KE, Castillejos, A, Diaz-Aguilar, D, Saint-Geniez, M, and Connor, KM. The alternative complement pathway aids in vascular regression during the early stages of a murine model of proliferative retinopathy. FASEB J. (2016) 30:1300–5. doi: 10.1096/fj.15-280834
143. Suchting, S, Freitas, C, le Noble, F, Benedito, R, Bréant, C, Duarte, A, et al. The notch Ligand Delta-like 4 negatively regulates endothelial tip cell formation and vessel branching. Proc Natl Acad Sci U S A. (2007) 104:3225–30. doi: 10.1073/pnas.0611177104
144. Lobov, IB, Renard, RA, Papadopoulos, N, Gale, NW, Thurston, G, Yancopoulos, GD, et al. Delta-like ligand 4 (Dll4) is induced by Vegf as a negative regulator of Angiogenic sprouting. Proc Natl Acad Sci U S A. (2007) 104:3219–24. doi: 10.1073/pnas.0611206104
145. Kappas, NC, Zeng, G, Chappell, JC, Kearney, JB, Hazarika, S, Kallianos, KG, et al. The Vegf receptor Flt-1 spatially modulates Flk-1 signaling and blood vessel branching. J Cell Biol. (2008) 181:847–58. doi: 10.1083/jcb.200709114
146. Chappell, JC, Cluceru, JG, Nesmith, JE, Mouillesseaux, KP, Bradley, VB, Hartland, CM, et al. Flt-1 (Vegfr-1) coordinates discrete stages of blood vessel formation. Cardiovasc Res. (2016) 111:84–93. doi: 10.1093/cvr/cvw091
147. Nesmith, JE, Chappell, JC, Cluceru, JG, and Bautch, VL. Blood vessel anastomosis is spatially regulated by Flt1 during angiogenesis. Development. (2017) 144:889–96. doi: 10.1242/dev.145672
148. Chappell, JC, Mouillesseaux, KP, and Bautch, VL. Flt-1 (vascular endothelial growth factor receptor-1) is essential for the vascular endothelial growth factor-notch feedback loop during angiogenesis. Arterioscler Thromb Vasc Biol. (2013) 33:1952–9. doi: 10.1161/ATVBAHA.113.301805
149. Tosi, GM, Orlandini, M, and Galvagni, F. The controversial role of Tgf-Β in Neovascular age-related macular degeneration pathogenesis. Int J Mol Sci. (2018) 19:3363. doi: 10.3390/ijms19113363
150. Zarkada, G, Howard, JP, Xiao, X, Park, H, Bizou, M, Leclerc, S, et al. Specialized endothelial tip cells guide Neuroretina vascularization and blood-retina-barrier formation. Dev Cell. (2021) 56:2237–2251.e6. doi: 10.1016/j.devcel.2021.06.021
151. Goumans, MJ, Valdimarsdottir, G, Itoh, S, Rosendahl, A, Sideras, P, and ten Dijke, P. Balancing the activation state of the endothelium via two distinct Tgf-Beta type I receptors. EMBO J. (2002) 21:1743–53. doi: 10.1093/emboj/21.7.1743
152. Hu, J, Li, T, Du, X, Wu, Q, and Le, YZ. G protein-coupled receptor 91 signaling in diabetic retinopathy and hypoxic retinal diseases. Vis Res. (2017) 139:59–64. doi: 10.1016/j.visres.2017.05.001
153. DeGraff, AC, Frieden, J, and Ahlquist, RP. Present state of alpha-and Beta-adrenergic drugs I. Adrenerg Recept Am Heart J. (1976) 92:661–4. doi: 10.1016/S0002-8703(76)80086-5
154. Dal Monte, M, Martini, D, Latina, V, Pavan, B, Filippi, L, and Bagnoli, P. Beta-Adrenoreceptor Agonism influences retinal responses to hypoxia in a model of retinopathy of prematurity. Invest Ophthalmol Vis Sci. (2012) 53:2181–92. doi: 10.1167/iovs.11-9408
155. Lindgren, I, and Altimiras, J. Chronic prenatal hypoxia sensitizes Beta-adrenoceptors in the embryonic heart but causes postnatal desensitization. Am J Physiol Regul Integr Comp Physiol. (2009) 297:R258–64. doi: 10.1152/ajpregu.00167.2009
156. Casini, G, Dal Monte, M, Fornaciari, I, Filippi, L, and Bagnoli, P. The Β-adrenergic system as a possible new target for pharmacologic treatment of Neovascular retinal diseases. Prog Retin Eye Res. (2014) 42:103–29. doi: 10.1016/j.preteyeres.2014.06.001
157. Martinez-Camarillo, JC, Spee, CK, Trujillo-Sanchez, GP, Rodriguez, A, Hinton, DR, Giarola, A, et al. Blocking ocular sympathetic activity inhibits choroidal neovascularization. Front Neurosci. (2021) 15:780841. doi: 10.3389/fnins.2021.780841
158. Steinle, JJ . Review: role of camp signaling in diabetic retinopathy. Mol Vis. (2020) 26:355–8.
159. Biesecker, KR, Srienc, AI, Shimoda, AM, Agarwal, A, Bergles, DE, Kofuji, P, et al. Glial cell calcium signaling mediates capillary regulation of blood flow in the retina. J Neurosci. (2016) 36:9435–45. doi: 10.1523/JNEUROSCI.1782-16.2016
160. Newman, EA . Functional hyperemia and mechanisms of neurovascular coupling in the retinal vasculature. J Cereb Blood Flow Metab. (2013) 33:1685–95. doi: 10.1038/jcbfm.2013.145
161. Novak, J, Parry, LJ, Matthews, JE, Kerchner, LJ, Indovina, K, Hanley-Yanez, K, et al. Evidence for local Relaxin ligand-receptor expression and function in arteries. FASEB J. (2006) 20:2352–62. doi: 10.1096/fj.06-6263com
162. Jelinic, M, Leo, CH, Post Uiterweer, ED, Sandow, SL, Gooi, JH, Wlodek, ME, et al. Localization of Relaxin receptors in arteries and veins, and region-specific increases in compliance and bradykinin-mediated relaxation after in vivo Serelaxin treatment. FASEB J. (2014) 28:275–87. doi: 10.1096/fj.13-233429
163. Ng, HH, Jelinic, M, Parry, LJ, and Leo, CH. Increased superoxide production and altered nitric oxide-mediated relaxation in the aorta of Young but not old male Relaxin-deficient mice. Am J Phys Heart Circ Phys. (2015) 309:H285–96. doi: 10.1152/ajpheart.00786.2014
164. Madaan, A, Chaudhari, P, Nadeau-Vallée, M, Hamel, D, Zhu, T, Mitchell, G, et al. Müller cell-localized G-protein-coupled receptor 81 (Hydroxycarboxylic acid receptor 1) regulates inner retinal vasculature via Norrin/Wnt pathways. Am J Pathol. (2019) 189:1878–96. doi: 10.1016/j.ajpath.2019.05.016
165. Ando, A, Yang, A, Mori, K, Yamada, H, Yamada, E, Takahashi, K, et al. Nitric oxide is proangiogenic in the retina and choroid*. J Cell Physiol. (2002) 191:116–24. doi: 10.1002/jcp.10083
166. Lin, C, Toychiev, A, Ablordeppey, R, Slavi, N, Srinivas, M, and Benavente-Perez, A. Myopia alters the structural Organization of the Retinal Vasculature, Gfap-positive glia and ganglion cell layer thickness. Int J Mol Sci. (2022) 23:6202. doi: 10.3390/ijms23116202
167. Haarman, AEG, Enthoven, CA, Tideman, JWL, Tedja, MS, Verhoeven, VJM, and Klaver, CCW. The complications of myopia: a review and Meta-analysis. Invest Ophthalmol Vis Sci. (2020) 61:49. doi: 10.1167/iovs.61.4.49
168. Sun, J, Wang, Y, and Wang, J. Choroidal arterial watershed zone topography and its relationship with maculopathy in highly myopic eyes. Eye. (2021) 35:2624–30. doi: 10.1038/s41433-021-01427-y
169. Wong, CW, Phua, V, Lee, SY, Wong, TY, and Cheung, CMG. Is choroidal or scleral thickness related to myopic macular degeneration? Invest Ophthalmol Vis Sci. (2017) 58:907–13. doi: 10.1167/iovs.16-20742
170. Hsia, Y, Wang, S-W, Huang, C-J, Hung, K-C, Chen, M-S, and Ho, T-C. Clinical characteristics of highly myopic patients with asymmetric myopic atrophic maculopathy–analysis using multimodal imaging. Invest Ophthalmol Vis Sci. (2021) 62:21. doi: 10.1167/iovs.62.3.21
171. Li, J, Zhou, H, Feinstein, M, Wong, J, Wang, RK, Chan, L, et al. Choriocapillaris changes in myopic macular degeneration. Transl Vis Sci Technol. (2022) 11:37. doi: 10.1167/tvst.11.2.37
172. Ohno-Matsui, K, Lai, TY, Lai, CC, and Cheung, CM. Updates of pathologic myopia. Prog Retin Eye Res. (2016) 52:156–87. doi: 10.1016/j.preteyeres.2015.12.001
173. Weinreb, RN, Aung, T, and Medeiros, FA. The pathophysiology and treatment of Glaucoma: a review. JAMA. (2014) 311:1901–11. doi: 10.1001/jama.2014.3192
174. Chan, KKW, Tang, F, Tham, CCY, Young, AL, and Cheung, CY. Retinal vasculature in Glaucoma: a review. BMJ Open Ophthalmol. (2017) 1:e000032. doi: 10.1136/bmjophth-2016-000032
175. Mitchell, P, Hourihan, F, Sandbach, J, and Wang, JJ. The relationship between Glaucoma and myopia - the Blue Mountains eye study. Ophthalmology. (1999) 106:2010–5. doi: 10.1016/S0161-6420(99)90416-5
176. Cedrone, C, Mancino, R, Ricci, F, Cerulli, A, Culasso, F, and Nucci, C. The 12-year incidence of Glaucoma and Glaucoma-related visual field loss in Italy: the Ponza eye study. J Glaucoma. (2012) 21:1–6. doi: 10.1097/IJG.0b013e3182027796
177. Czudowska, MA, Ramdas, WD, Wolfs, RCW, Hofman, A, De Jong, PTVM, Vingerling, JR, et al. Incidence of glaucomatous visual field loss: a ten-year follow-up from the Rotterdam study. Ophthalmology. (2010) 117:1705–12. doi: 10.1016/j.ophtha.2010.01.034
178. Kuzin, AA, Varma, R, Reddy, HS, Torres, M, and Azen, SP, Los Angeles Latino Eye Study G. Ocular biometry and open-angle Glaucoma: the Los Angeles Latino eye study. Ophthalmology. (2010) 117:1713–9. doi: 10.1016/j.ophtha.2010.01.035
179. Marcus, MW, de Vries, MM, Montolio, FGJ, and Jansonius, NM. Myopia as a risk factor for open-angle Glaucoma: a systematic review and Meta-analysis. Ophthalmology. (2011) 118:1989–94.e2. doi: 10.1016/j.ophtha.2011.03.012
180. Xu, L, Wang, Y, Wang, S, Wang, Y, and Jonas, JB. High myopia and Glaucoma susceptibility: the Beijing eye study. Ophthalmology. (2007) 114:216–20. doi: 10.1016/j.ophtha.2006.06.050
181. Memarzadeh, F, Ying-Lai, M, Chung, J, Azen, SP, and Varma, R. Blood pressure, perfusion pressure, and open-angle Glaucoma: the Los Angeles Latino eye study. Invest Ophthalmol Vis Sci. (2010) 51:2872–7. doi: 10.1167/iovs.08-2956
182. Leske, MC, Wu, SY, Hennis, A, Honkanen, R, and Nemesure, B. Risk factors for incident open-angle Glaucoma: the Barbados eye studies. Ophthalmology. (2008) 115:85–93. doi: 10.1016/j.ophtha.2007.03.017
183. Fuchsjäger-Mayrl, G, Wally, B, Georgopoulos, M, Rainer, G, Kircher, K, Buehl, W, et al. Ocular blood flow and systemic blood pressure in patients with primary open-angle Glaucoma and ocular hypertension. Invest Ophthalmol Vis Sci. (2004) 45:834–9. doi: 10.1167/iovs.03-0461
184. Suwan, Y, Fard, MA, Geyman, LS, Tantraworasin, A, Chui, TY, Rosen, RB, et al. Association of Myopia with Peripapillary perfused capillary density in patients with Glaucoma: an optical coherence tomography angiography study. JAMA Ophthalmol. (2018) 136:507–13. doi: 10.1001/jamaophthalmol.2018.0776
185. Mammo, Z, Heisler, M, Balaratnasingam, C, Lee, S, Yu, DY, Mackenzie, P, et al. Quantitative optical coherence tomography angiography of radial Peripapillary capillaries in Glaucoma, Glaucoma suspect, and Normal eyes. Am J Ophthalmol. (2016) 170:41–9. doi: 10.1016/j.ajo.2016.07.015
186. Ye, J, Lin, J, Shen, M, Chen, W, Zhang, R, Lu, F, et al. Reduced radial Peripapillary capillary in pathological myopia is correlated with visual acuity. Front Neurosci. (2022) 16:818530. doi: 10.3389/fnins.2022.818530
187. Yamazaki, Y, and Drance, SM. The relationship between progression of visual field defects and retrobulbar circulation in patients with Glaucoma. Am J Ophthalmol. (1997) 124:287–95. doi: 10.1016/S0002-9394(14)70820-7
188. Findl, O, Rainer, G, Dallinger, S, Dorner, G, Polak, K, Kiss, B, et al. Assessment of optic disk blood flow in patients with open-angle Glaucoma. Am J Ophthalmol. (2000) 130:589–96. doi: 10.1016/S0002-9394(00)00636-X
189. Venkataraman, ST, Flanagan, JG, and Hudson, C. Vascular reactivity of optic nerve head and retinal blood vessels in glaucoma – a review. Microcirculation. (2010) 17:568–81. doi: 10.1111/j.1549-8719.2010.00045.x
190. Németh, J, Michelson, G, and Harazny, J. Retinal microcirculation correlates with Ocular Wall thickness, axial eye length, and refraction in Glaucoma patients. J Glaucoma. (2001) 10:390–5. doi: 10.1097/00061198-200110000-00005
191. Samra, WA, Pournaras, C, Riva, C, and Emarah, M. Choroidal hemodynamic in myopic patients with and without primary open-angle Glaucoma. Acta Ophthalmol. (2013) 91:371–5. doi: 10.1111/j.1755-3768.2012.02386.x
192. Lin, F, Li, F, Gao, K, He, W, Zeng, J, Chen, Y, et al. Longitudinal changes in macular optical coherence tomography angiography metrics in primary open-angle Glaucoma with high myopia: a prospective study. Invest Ophthalmol Vis Sci. (2021) 62:30. doi: 10.1167/iovs.62.1.30
193. Metelitsina, TI, Grunwald, JE, DuPont, JC, Ying, GS, Brucker, AJ, and Dunaief, JL. Foveolar choroidal circulation and choroidal neovascularization in age-related macular degeneration. Invest Ophthalmol Vis Sci. (2008) 49:358–63. doi: 10.1167/iovs.07-0526
194. Wakabayashi, T, Ikuno, Y, Oshima, Y, Hamasaki, T, and Nishida, K. Aqueous concentrations of vascular endothelial growth factor in eyes with high myopia with and without choroidal neovascularization. J Ophthalmol. (2013) 2013:257381. doi: 10.1155/2013/257381
195. Feng, B, Su, W, Chen, Q, Gan, R, Wang, M, Wang, J, et al. Quantitative analysis of retinal vasculature in rhegmatogenous retinal detachment based on ultra-Widefield fundus imaging. Front Med. (2021) 8:797479. doi: 10.3389/fmed.2021.797479
196. Tolentino, FI, Lapus, JV, Novalis, G, Trempe, CL, Gutow, GS, and Ahmad, A. Fluorescein angiography of degenerative lesions of the peripheral fundus and Rhegmatogenous retinal detachment. Int Ophthalmol Clin. (1976) 16:13–29. doi: 10.1097/00004397-197601610-00005
197. Cardillo, PF . Vascular changes in Rhegmatogenous retinal detachment. Ophthalmologica. (1983) 186:17–24. doi: 10.1159/000309255
198. Ohno-Matsui, K . Proposed classification of posterior staphylomas based on analyses of eye shape by three-dimensional magnetic resonance imaging and wide-field fundus imaging. Ophthalmology. (2014) 121:1798–809. doi: 10.1016/j.ophtha.2014.03.035
199. Nie, F, Zhang, L, Cao, M, Zhou, D, Liu, K, Ouyang, J, et al. Impact of Peripapillary staphylomas on the vascular and structural characteristics in myopic eyes: a propensity score matching analysis. Graefes Arch Clin Exp Ophthalmol. (2023) 2013:257381. doi: 10.1007/s00417-022-05966-2
200. Nie, F, Ouyang, J, Tang, W, Luo, L, Cao, M, Zhang, L, et al. Posterior staphyloma is associated with the microvasculature and microstructure of myopic eyes. Graefes Arch Clin Exp Ophthalmol. (2021) 259:2119–30. doi: 10.1007/s00417-020-05057-0
201. Klein, BE, Klein, R, Jensen, SC, and Linton, KL. Hypertension and Lens opacities from the beaver dam eye study. Am J Ophthalmol. (1995) 119:640–6. doi: 10.1016/S0002-9394(14)70223-5
202. Klein, BE, Klein, R, and Lee, KE. Diabetes, cardiovascular disease, selected cardiovascular disease risk factors, and the 5-year incidence of age-related cataract and progression of Lens opacities: the beaver dam eye study. Am J Ophthalmol. (1998) 126:782–90. doi: 10.1016/S0002-9394(98)00280-3
203. Grieshaber, MC, Koçak, I, Dubler, B, Flammer, J, and Orgül, S. Retrobulbar blood flow in patients with cataract. Br J Ophthalmol. (2006) 90:1512–5. doi: 10.1136/bjo.2006.101261
Keywords: myopia, vascular, structure, function, evidence
Citation: Benavente-Perez A (2023) Evidence of vascular involvement in myopia: a review. Front. Med. 10:1112996. doi: 10.3389/fmed.2023.1112996
Edited by:
Doina Gherghel, Aston University, United KingdomCopyright © 2023 Benavente-Perez. This is an open-access article distributed under the terms of the Creative Commons Attribution License (CC BY). The use, distribution or reproduction in other forums is permitted, provided the original author(s) and the copyright owner(s) are credited and that the original publication in this journal is cited, in accordance with accepted academic practice. No use, distribution or reproduction is permitted which does not comply with these terms.
*Correspondence: Alexandra Benavente-Perez, YWJlbmF2ZW50ZUBzdW55b3B0LmVkdQ==