- 1Department of Biomedical Sciences and Public Health, Università Politecnica delle Marche, Ancona, Italy
- 2Anesthesia and Intensive Care Unit, Azienda Ospedaliera Universitaria “Ospedali Riuniti Umberto I-Lancisi-Salesi”, Ancona, Italy
Background: In COVID-19 patients requiring mechanical ventilation, the administration of high oxygen (O2) doses for prolonged time periods may be necessary. Although life-saving in most cases, O2 may exert deleterious effects if administered in excessive concentrations. We aimed to describe the prevalence of hyperoxemia and excessive O2 administration in mechanically ventilated patients with SARS-CoV-2 pneumonia and determine whether hyperoxemia is associated with mortality in the Intensive Care Unit (ICU) or the onset of ventilator-associated pneumonia (VAP).
Materials and methods: Retrospective single-center study on adult patients with SARS-CoV-2 pneumonia requiring invasive mechanical ventilation for ≥48 h. Patients undergoing extracorporeal respiratory support were excluded. We calculated the excess O2 administered based on the ideal arterial O2 tension (PaO2) target of 55–80 mmHg. We defined hyperoxemia as PaO2 > 100 mmHg and hyperoxia + hyperoxemia as an inspired O2 fraction (FiO2) > 60% + PaO2 > 100 mmHg. Risk factors for ICU-mortality and VAP were assessed through multivariate analyses.
Results: One hundred thirty-four patients were included. For each day of mechanical ventilation, each patient received a median excess O2 of 1,121 [829–1,449] L. Hyperoxemia was found in 38 [27–55]% of arterial blood gases, hyperoxia + hyperoxemia in 11 [5–18]% of cases. The FiO2 was not reduced in 69 [62–76]% of cases of hyperoxemia. Adjustments were made more frequently with higher PaO2 or initial FiO2 levels. ICU-mortality was 32%. VAP was diagnosed in 48.5% of patients. Hyperoxemia (OR 1.300 95% CI [1.097–1.542]), time of exposure to hyperoxemia (OR 2.758 [1.406–5.411]), hyperoxia + hyperoxemia (OR 1.144 [1.008–1.298]), and daily excess O2 (OR 1.003 [1.001–1.005]) were associated with higher risk for ICU-mortality, independently of age, Sequential Organ failure Assessment score at ICU-admission and mean PaO2/FiO2. Hyperoxemia (OR 1.033 [1.006–1.061]), time of exposure to hyperoxemia (OR 1.108 [1.018–1.206]), hyperoxia + hyperoxemia (OR 1.038 [1.003–1.075]), and daily excess O2 (OR 1.001 [1.000–1.001]) were identified as risk factors for VAP, independently of body mass index, blood transfusions, days of neuromuscular blocking agents (before VAP), prolonged prone positioning and mean PaO2/FiO2 before VAP.
Conclusion: Excess O2 administration and hyperoxemia were common in mechanically ventilated patients with SARS-CoV-2 pneumonia. The exposure to hyperoxemia may be associated with ICU-mortality and greater risk for VAP.
Introduction
Supplemental oxygen (O2) is a life-saving therapy in hypoxemic patients in order to guarantee adequate tissue O2 delivery. Nonetheless, excessive O2 administration may also exert deleterious effects (1). In recent years, several studies supported the use of more conservative oxygenation strategies in Intensive Care Units (ICUs), whereas liberal O2 therapy and the exposure to arterial hyperoxia in critically ill patients were associated with adverse outcomes (2–4). The lung is the first organ affected by O2 toxicity. Hyperoxia induces oxidative stress and inflammation in the lung (1) and may impair the surfactant system, thus causing alveolar collapse and the reduction in pulmonary compliance (5). Excess O2 administration may also compromise muco-ciliary clearance and the anti-microbial capacity of the immune cells, thus contributing to the development of ventilator-associated pneumonia (VAP) (6).
In moderate or severe acute respiratory distress syndrome (ARDS), the administration of high inspired O2 fractions (FiO2) is frequently required to maintain normoxemia (arterial O2 tension [PaO2] 80–100 mmHg) and this may predispose to additional hyperoxia-induced lung injury. In order to limit the exposure to hyperoxia, the ARDS Network recommends using a PaO2 target of 55–80 mmHg in mechanically ventilated patients (7). Nonetheless, ARDS patients are frequently exposed to excessive FiO2 levels (8) and even undergo a condition of hyperoxemia (PaO2 > 100 mmHg) in a substantial number of cases (9). In a meta-analysis of randomized controlled trials (RCTs), excessive O2 administration (FiO2 > 50%) resulting in PaO2 levels above the protocol goal (>80 mmHg) was associated with mortality and lower ventilator- and hospital-free days (10). A recent multicentre RCT was aimed to compare a conservative (target PaO2 55–70 mmHg, SpO2 88–92%) with a liberal oxygenation strategy (target PaO2 90–105 mmHg, peripheral O2 saturation [SpO2] ≥ 96%) in ARDS patients, however, this study was prematurely stopped due to safety concerns (higher mortality and five mesenteric ischemic events in the conservative O2 group) (11). Therefore, which is the safest oxygenation target for mechanically ventilated patients with ARDS remains an open question.
This is a problem of major importance for patients with severe acute respiratory syndrome due to novel Coronavirus (SARS-CoV-2). A large Italian cohort study showed that 12% of these patients received FiO2 up to 100% and an FiO2 ≥ 50% was necessary in 89% of total (12). Hyperoxia-induced lung injury may add to the inflammatory process caused by the viral infection. Moreover, these patients often require long periods of mechanical ventilation and are at risk of exposure to high O2 concentrations for several days (13). Despite the key role of O2 in the treatment of SARS-CoV-2, the potential adverse effects of a prolonged exposure to hyperoxia in this patient category remain unexplored.
The primary aim of this study was to explore whether mechanically ventilated patients with SARS-CoV-2 pneumonia in our ICU received an excessive amount of O2 and were exposed to hyperoxemia. In addition, we evaluated if hyperoxemia was associated with mortality or the onset of VAP.
Materials and methods
This retrospective observational study was conducted in the “General, respiratory and major trauma Intensive care Unit” of the Azienda Ospedaliera Universitaria “Ospedali Riuniti Umberto I-Lancisi-Salesi” of Ancona, Italy. During the pandemic phase, this ICU provided 18 beds for COVID-19 patients. The study protocol was approved by the local Ethics Committee (Comitato Etico Regionale Marche). Written informed consent was not requested due to the retrospective study design. This study included all consecutive adult (>18 year old) patients with SARS-CoV-2 pneumonia admitted to the ICU between February 2020 and May 2021, who required endotracheal intubation and invasive mechanical ventilation for at least 48 consecutive hours. COVID-19 infection was confirmed by means of real time polymerase chain reaction on nasopharyngeal swab or bronchoalveolar lavage. Exclusion criteria were: duration of mechanical ventilation < 48 h; ICU-discharge or death within 48 h; use of extracorporeal membrane oxygenation (ECMO) or extracorporeal carbon dioxide removal (ECCO2R); admission from another ICU with a length of stay > 48 h; re-admissions after a previous ICU admission for SARS-CoV-2 pneumonia; COVID-19+ patients without pneumonia admitted to the ICU for different reasons.
Patient management
According to the most recent guidelines for the management of ARDS and COVID-19 (7, 14, 15), all patients received a lung protective ventilation strategy with a tidal volume (TV) of 4–6 ml/kg of ideal body weight, while maintaining a plateau pressure (Pplat) ≤ 30 cmH2O and a driving pressure ≤ 15 cmH2O. A positive end-expiratory pressure (PEEP) ≥ 5 cmH2O was applied in all patients, using an open-lung strategy. Neuromuscular blocking agents (NMBA) (continuous infusion of cisatracurium or rocuronium) were used in the early phase of mechanical ventilation in cases of refractory hypoxemia despite deep sedation to facilitate lung protective ventilation, during prone positioning, in cases of patient-ventilator dyssynchrony and/or in presence of high respiratory drive despite optimal sedation (15). Prone positioning was used in patients with a PaO2/FiO2 < 150 mmHg for a duration of at least 16 h per session (15). During the COVID-19 pandemic, we implemented and applied a protocol of prolonged prone positioning with a duration of 36 consecutive hours per session, which showed to be feasible and potentially more effective in improving oxygenation (16). Inhaled nitric oxide was administered as a rescue therapy in cases of refractory hypoxemia despite the use of NMBA and prone ventilation. Veno-venous ECMO was used in patients with refractory hypoxemia despite all other therapies (17).
A VAP prevention strategy was routinely applied, including: oral care with chlorhexidine three times a day; head of bed elevation (30° whenever possible); check (3 times a day) and maintenance of the cuff pressure of the endotracheal tube (25 cmH2O); routine aspiration of tracheal secretions by means of a closed suction system; frequent check of the correct positioning and function of the nasogastric tube; weaning from mechanical ventilation as soon as possible; tracheostomy in cases of anticipated difficult weaning (18). During prone ventilation, the administration of enteral nutrition was discontinued or, alternatively, continued at low dosage (10–20 ml/h) with frequent check of the gastric residual volume and possible episodes of regurgitation. Stress ulcer prophylaxis (pantoprazole 40 mg/day) was administered in patients with risk factors for gastro-intestinal bleeding (including coagulopathy, mechanical ventilation >48 h, hypotension), who represented the vast majority of the patients enrolled. Deep venous thrombosis prophylaxis was applied in all patients with low molecular weight heparin (at least 4,000 UI/day). In patients with VAP, antibiotic therapy was based on local written protocols in accordance with the most recent guidelines (19).
Data collection
For each included patient we collected: age, gender, body mass index (BMI), comorbidities (obesity, arterial hypertension, diabetes mellitus, chronic obstructive pulmonary disease, ischemic cardiomyopathy), the Sequential Organ Failure Assessment (SOFA) score at ICU admission, arterial lactate levels at ICU admission, PaO2/FiO2 at ICU admission, ICU length-of-stay, duration of invasive mechanical ventilation, ICU mortality, antibiotics prior to ICU admission, steroids prior to ICU admission, total steroid dose administered in the ICU (adjusted for body weight, mg/kg/day), episodes of VAP (early/late onset, causal pathogen, antibiotic resistance), use of NMBA, prone positioning (number of sessions, prolonged prone ventilation), use of proton pump inhibitors, blood transfusions (number of units).
The onset of VAP was defined by the detection of a causative agent in a respiratory sample (tracheal aspirate or bronchoalveolar lavage) associated with a new or progressive lung infiltrate, plus at least two clinical criteria (fever or hypothermia, leukocytosis or leukopenia, purulent secretions), after at least 48 h of invasive mechanical ventilation (18). In cases of multiple VAP episodes, only the first episode was considered. An episode of VAP occurring within 4 days after endotracheal intubation was defined as “early onset VAP” (18).
Starting from endotracheal intubation and for the whole duration of invasive mechanical ventilation, we collected data from all arterial blood gas (ABG) analyses, including PaO2, SaO2, pH, PaCO2 with date and time. In our ICU, ABG are generally performed at least every 8 h in all patients. For each ABG we recorded data on mechanical ventilation: TV (ml), respiratory rate (RR), minute ventilation (l/min), PEEP, FiO2, and PaO2/FiO2. In addition, for each ABG we noted if the patient was on NMBA, prone ventilation, and measures of respiratory mechanics if available (Pplat, driving pressure, static compliance [Cstat]).
Based on the ARDS Network protocol, we considered a PaO2 of 80 mmHg as the upper limit of the oxygenation target. For each ABG showing a PaO2 > 80 mmHg, we estimated the “ideal FiO2” that would be sufficient to obtain a PaO2 of 80 mmHg based on the PaO2/FiO2 ratio, according to the following formula:
We then estimated the excess O2 being administered as follows:
We then multiplied the value obtained for the time (minutes) elapsed since the previous ABG and calculated:
- Total excess O2 (L): total cumulative amount of excess O2 administered during the whole duration of invasive mechanical ventilation;
- Daily excess O2 (L/day): average amount of excess O2 administered in each day of invasive mechanical ventilation;
- Three-days excess O2 (L): cumulative amount of excess O2 administered during the first 3 days of invasive mechanical ventilation;
- Daily excess O2 before VAP (L/day): average amount of excess O2 administered in each day of invasive mechanical ventilation before the first diagnosis of VAP.
Episodes of “hyperoxemia” were defined by a PaO2 > 100 mmHg. We defined as “uncorrected hyperoxemia” any episode of hyperoxemia that was not followed by a reduction in the FiO2. In addition, we defined as “hyperoxia + hyperoxemia” any episode of FiO2 > 60% in presence of a PaO2 > 100 mmHg. Herein, the term “hyperoxia” (generally defined as the administration of any O2 dose > 21%) was used to indicate a high FiO2 (>60%). We then calculated the prevalence of hyperoxemia and hyperoxia + hyperoxemia for the whole duration of invasive mechanical ventilation, in the first 3 days of mechanical ventilation and for the days prior to VAP diagnosis. The duration of exposure to hyperoxemia was estimated as follows:
1. Daily time of exposure to hyperoxemia (hours per day of MV): whenever an ABG showed a PaO2 > 100 mmHg, we considered as time of exposure to hyperoxemia the time elapsed since the previous ABG; the total sum of hours was then divided by the number of days of MV.
2. Time of exposure to hyperoxemia in the first 3 days of MV: total sum of hours of hyperoxemia in the first 3 days of MV.
3. Daily time of exposure to hyperoxemia before VAP: average amount of hours of hyperoxemia in each day of invasive mechanical ventilation before the first diagnosis of VAP.
In addition, we calculated: mean FiO2, mean PaO2, mean PaO2/FiO2, highest FiO2, highest PaO2.
Statistical analysis
Statistics was performed with GraphPad Prism version 6 (GraphPad Software, La Jolla, CA, United States) and Statistical Package for Social Science software, version 17.0 (SPSS Inc., Chicago, IL, United States). Normality of distribution was checked with the Shapiro–Wilk test. Continuous variables were expressed as mean ± standard deviation or median [1st–3rd quartile], as appropriate. Unpaired t-test or Mann Whitney U-test were used for comparisons of two groups. The chi-square test was used for nominal variables. We constructed multivariate binary logistic regression models in order to evaluate the independent association between the exposure to hyperoxemia (prevalence of hyperoxemia, prevalence of hyperoxia + hyperoxemia, daily excess O2) and the outcomes of interest (ICU mortality, diagnosis of VAP). Separate models were constructed for each index of exposure to hyperoxemia in order to avoid multi-collinearity. The basic assumptions for conducting logistic regression analyses were verified, including the absence of multi-collinearity and the linearity of the logit for each continuous independent variable (20). Covariates included in the models were selected based on their well-established association with the outcome of interest (6, 20). A p-value < 0.05 was used to indicate statistical significance.
Results
Between February 27, 2020 and May 12, 2021, a total of 207 COVID-19 patients was admitted to our ICU. Of these, exclusions were: 43 patients who underwent ECMO or ECCO2R; 7 patients who had an ICU length of stay < 48 h; 1 patient who was extubated before 48 h; 1 non-intubated patient; 13 patients transferred from a different ICU in which they stayed for more than 48 h; 6 re-admissions; 2 COVID-19+ patients without pneumonia. Therefore, we included 134 patients in total. The vast majority of patients was male, and the most frequent comorbities were obesity and arterial hypertension (Table 1). ICU-mortality was 32%. ICU Non-survivors were older, had a higher prevalence of ischemic cardiomyopathy and worse PaO2/FiO2 and SOFA score at ICU-admission (Table 1).
Excess O2 and hyperoxemia
We analyzed 9,583 ABGs in total. A PaO2 > 80 mmHg was found in 68.8% of cases. All the analyzed patients received an excessive amount of O2 in relation to the oxygenation target indicated by the guidelines (55–80 mmHg). We estimated that, for the whole duration of invasive mechanical ventilation, each patient received an average excess O2 of 17,741 [8,950–27,248] L, corresponding to a daily excess O2 of 1,121 [829–1,449] L (minimum: 319 L; maximum: 3,818 L). In each patient, hyperoxemia (PaO2 > 100 mmHg) was present in 38 [27–55]% of ABGs, and 11 [5–18]% of ABGs showed a condition of hyperoxia + hyperoxemia (FiO2 > 60% + PaO2 > 100 mmHg). Hyperoxemia was more frequently associated with higher PaO2/FiO2 and possible indicators of less severe pulmonary dysfunction (lower Pplat and driving pressure) (Table 2). Figure 1 show the prevalence of hyperoxemia stratified by the FiO2 and PaO2/FiO2.
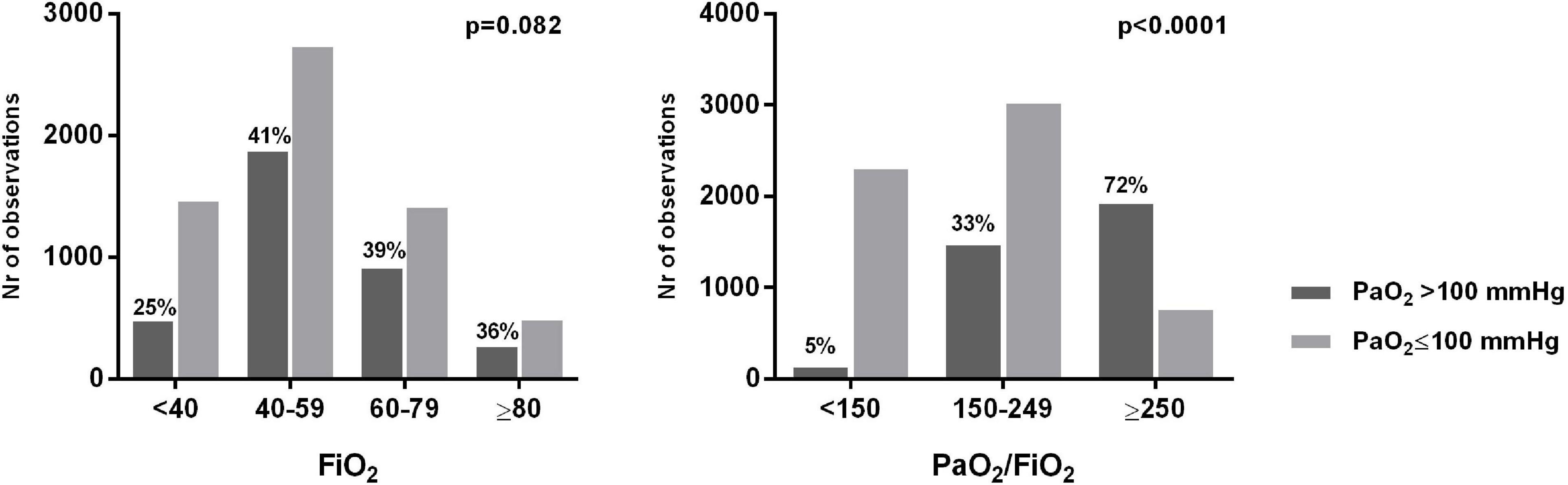
Figure 1. Number and percentage of arterial blood gases showing hyperoxemia stratified by PaO2 and FiO2 levels.
In 69 [62–76]% of cases, an ABG showing hyperoxemia was not followed by a reduction in the FiO2 (uncorrected hyperoxemia). The FiO2 was more likely to be reduced in presence of higher PaO2 and initial FiO2 levels, although even in presence of a PaO2 ≥ 150 mmHg or an initial FiO2 ≥ 80% no change was made in about 40% of cases (Figure 2). The choice of reducing the FiO2 was not influenced by PaO2/FiO2 or PEEP levels (Figure 2).
Hyperoxemia and intensive care unit-mortality
Intensive care unit-survivors showed higher mean PaO2/FiO2, mean PaO2 and maximum PaO2 as compared to Non-survivors, however mean and maximum FiO2 were significantly lower (Table 3). Hyperoxemia was more frequent among ICU-survivors, and the daily time of exposure was higher, even if Non-survivors showed a higher prevalence of hyperoxia + hyperoxemia (both for the whole duration of mechanical ventilation and in the first 3 days) (Table 3). After adjusting for age, SOFA score at ICU-admission and mean PaO2/FiO2, the prevalence of hyperoxemia, the duration of exposure, the prevalence of hyperoxia + hyperoxemia and daily excess O2 were independently associated with ICU-mortality (Table 4). The total excess O2 and the excess O2 in the first 3 days were not significantly associated with mortality in the logistic regression analysis.
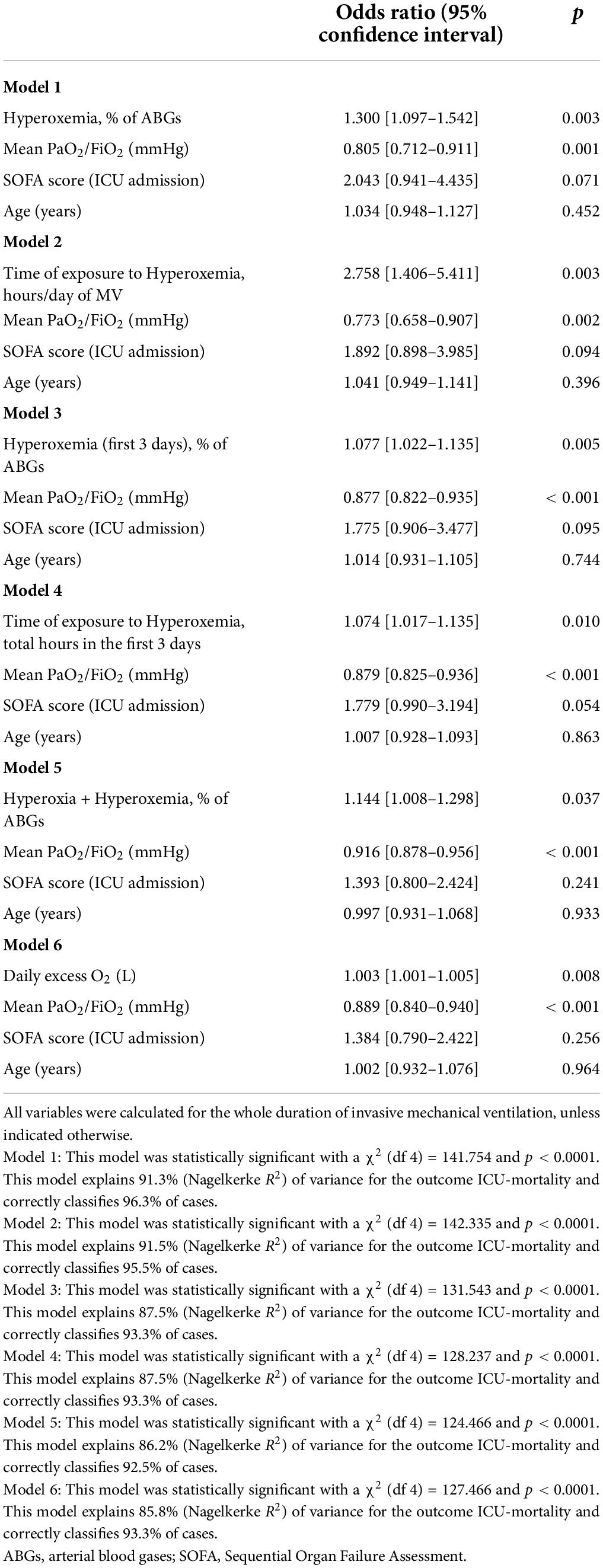
Table 4. Binomial logistic regression models for the association between hyperoxemia and ICU-mortality.
Hyperoxemia and ventilator-associated pneumonia
Sixty-five patients (48.5% of total) had at least one episode of VAP during the ICU-stay. The vast majority (91%) of VAP episodes was late-onset. The most frequently isolated pathogens were: Staphylococcus Aureus (29 cases); Acinetobacter Baumanii (20 cases); Enterobacteriaceae (Klebsiella spp., Escherichia Coli, Serratia spp., Enterobacter spp., 34 cases); Pseudomonas Aeruginosa (14 cases); Corynebacterium Striatum (10 cases); Proteus Mirabilis (10 cases); Streptococcus Pneumoniae (3 cases); Aspergillus spp. (7 cases). Multi-drug resistant pathogens were isolated in 33% of cases.
Patients with VAP had longer ICU-stay, more frequently underwent prolonged prone positioning, and showed lower PaO2/FiO2 than those with no episodes of VAP (Table 5). Patients with at least one episode of VAP showed higher prevalence of hyperoxia + hyperoxemia (before VAP and in the first 3 days of mechanical ventilation) and received a higher excess O2 (daily and in the first 3 days of mechanical ventilation) (Table 5).
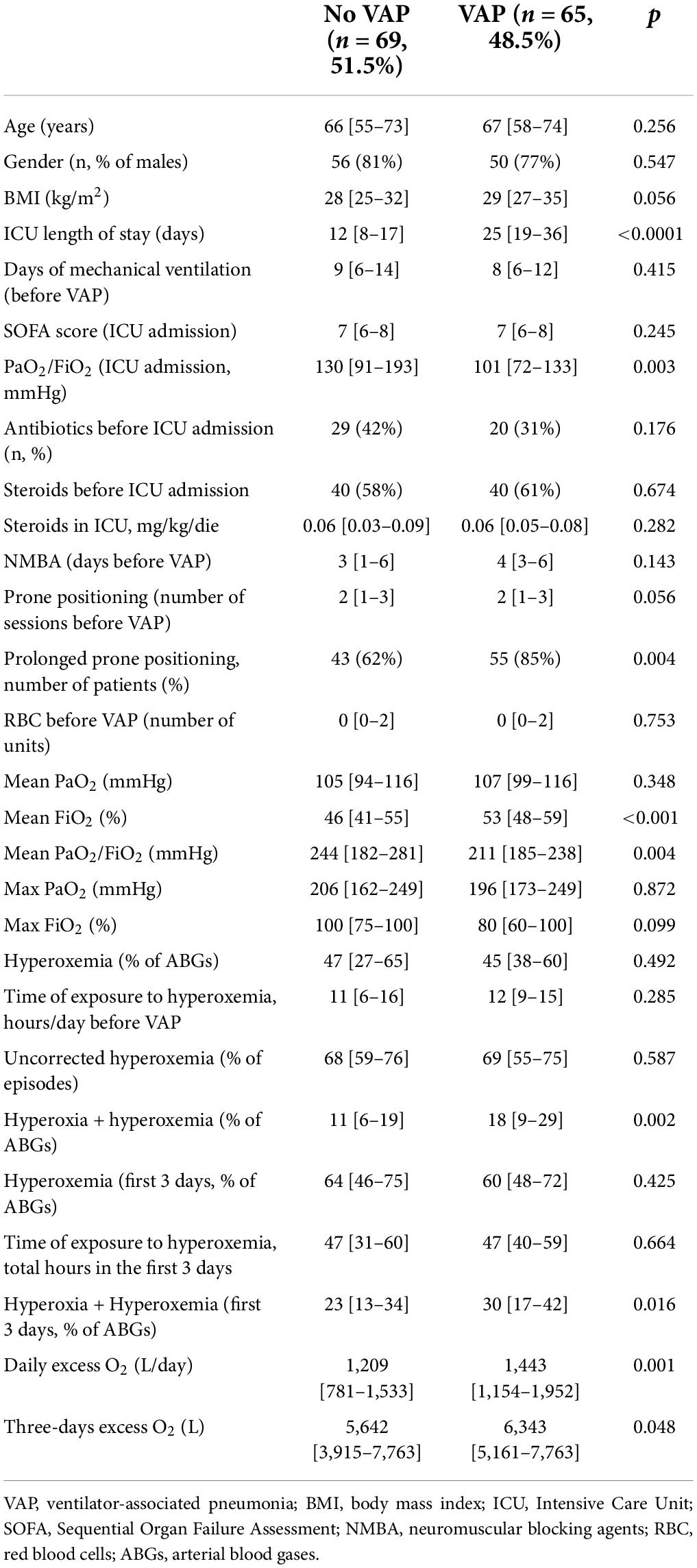
Table 5. Comparison between patients whit at least one episode of VAP and those without any episode of VAP.
After adjusting for BMI, blood transfusions, days of NMBA (before VAP), prolonged prone positioning and mean PaO2/FiO2 before VAP, a higher prevalence of hyperoxemia was associated with a higher risk of VAP (Table 6): the adjusted risk for VAP increased by 3.3% for each unitary increase in the percentage of ABGs with hyperoxemia. Similarly, the time of exposure to hyperoxemia before the diagnosis of VAP, the prevalence of hyperoxia + hyperoxemia, as well as the daily excess O2, were independently associated with the risk of VAP (Table 6). Moreover, patients in the highest tertile of daily excess O2 showed a 4.3 times greater adjusted risk of developing VAP as compared to those in the lowest tertile. The total amount of excess O2 received before the diagnosis of VAP was not significantly associated with the onset of VAP, nor were the prevalence of hyperoxemia or hyperoxia + hyperoxemia in the first 3 days of mechanical ventilation and the duration of hyperoxemia in the first 3 days.
Discussion
This retrospective single-centre study on 134 mechanically ventilated patients with SARS-CoV-2 pneumonia showed that: first, the dose of supplemental O2 administered was often excessive in comparison to the oxygenation target indicated by current guidelines and the exposure to hyperoxemia was frequent; second, in most cases of hyperoxemia the FiO2 was not varied, adjustments in O2 dose were made more frequently in presence of higher PaO2 or higher initial FiO2; third, the exposure to hyperoxemia was independently associated with higher risks of ICU-mortality and VAP.
Our data are consistent with those of previous studies showing that clinicians generally tolerate higher PaO2 values than those commonly recommended. In a retrospective study in critically ill mechanically ventilated patients, de Graaf et al. showed a prevalence of hyperoxemia (PaO2 > 120 mmHg) of 22% and adjustments in the FiO2 were rarely made, especially if this was ≤40% (21). Suzuki et al. calculated that an excess O2 dose of 3,472 L per patient was administered on average during mechanical ventilation and no change in the FiO2 was made in most cases of hyperoxemia if the initial level was 30–40% (22). In a recent study in COVID-19 mechanically ventilated patients, the prevalence of hyperoxemia in the ICU using a standard oxygenation protocol was 75.9% in the first day (23). Similarly in our study, the FiO2 was more likely to be reduced in presence of more severe hyperoxemia, whereas PaO2 values of 100–119 mmHg were accepted in more than 80% of cases. The initial FiO2 also determined the clinicians’ behavior against hyperoxemia: if this was <40% no change was made in almost 90% of cases, while an initial FiO2 ≥ 80% was corrected in more than 60% of cases.
A possible explanation of this too liberal attitude toward O2 therapy is a lack of perception of the risks associated with hyperoxemia. A survey by Helmerhorst et al. showed that most clinicians recognize the potential deleterious effects of a prolonged exposure to excessive O2 concentrations, including hyperoxia-induced lung injury, and show little tolerance toward even mild hyperoxemia (24). Nonetheless, a large proportion of their ICU patients was exposed to higher arterial O2 levels than self-reported target ranges (24). These data suggest that, in actual clinical practice, clinicians tend to tolerate a certain degree of hyperoxia, as perceived as a safety buffer against hypoxemia. In the context of the COVID-19 pandemic, such a liberal attitude may be partly justified by a greater fear against episodes of hypoxemia in a clinical scenario in which a ready accessibility to the patient is not always guaranteed due to the isolation precautions, and an SpO2 of 98–100% on the monitor could appear more reassuring than an SpO2 of 90–92%.
As a matter of fact, O2 toxicity and hyperoxia-induced lung injury are relatively slow processes in comparison to more acute conditions that can rapidly induce pulmonary oedema, respiratory failure and shock (such as aspiration pneumonia or sepsis-induced ARDS). A continuous exposure to hyperoxia for days/weeks can lead to diffuse alveolar damage, respiratory failure and high risk of death, while prolonged exposures to sub-lethal O2 doses generally induce pulmonary fibrosis (1). In healthy volunteers, breathing 95% O2 for 17 h caused a significant alveolar-capillary leak, due to a progressive destruction of the alveolar-capillary membrane, alveolar hemorrhage, formation of microthrombi, and intrapulmonary shunt (25). Moreover, oxidative stress compromises the surfactant system, thus causing atelectasis and reduction in lung compliance (26). Patients with ARDS may be particularly susceptible to O2 toxicity, since the ongoing inflammatory process may already compromise their adaptive and anti-oxidant capacities. Moreover, hyperoxia may aggravate and predispose the lung to the deleterious effects of positive pressure ventilation (5).
A recent meta-analysis of RCTs showed that the use of higher oxygenation targets in the critically ill may increase mortality, even if with a very-low level of evidence (27). The LOCO2 trial failed to prove the safety and efficacy of a more restrictive oxygen therapy in patients with ARDS (11), however, these results cannot be conclusive. Similarly, the multi-center HOT-ICU trial did not show any difference in 90-day mortality with a lower oxygenation target as compared to higher PaO2 target in patients with acute hypoxemic respiratory failure (28). Our study showed that the exposure to hyperoxemia in mechanically ventilated patients with SARS-CoV-2 pneumonia may be associated with higher ICU mortality, independently of other risk factors such as the mean PaO2/FiO2, SOFA score at admission and age. Moreover, a higher prevalence of hyperoxemia, as well as an excess O2 administration, was independently associated with a greater risk of VAP.
Ventilator-associated pneumonia is the most common ICU-acquired infection among mechanically ventilated patients, leading to higher mortality, longer need for mechanical ventilation, and an increase in healthcare cost (18). For the implementation of effective preventive strategies, it is crucial to gain a deep understanding of the pathophysiology and risk factors for VAP. There is ample evidence that a long-term exposure to hyeroxia can impair pulmonary innate immunity and bacterial phagocytosis capacity (29). In a retrospective study on mechanically ventilated critically ill patients, the presence of hyperoxemia (defined as PaO2 > 120 mmHg) at ICU-admission and the number of days of exposure to hyperoxemia were independently associated with the onset of VAP (6). Preclinical studies in animal models also supported a role of hyperoxia in the pathogenesis of VAP. Entezari et al. showed that a prolonged exposure to hyperoxia can compromise the ability of alveolar macrophages to phagocytose Pseudomonas Aeruginosa (30) and increased mortality in infected mice (31). Hyperoxia increased mortality in mice with Acinetobacter pneumonia, in which the administration of procysteine was able to improve survival by increasing the phagocytic activity of alveolar macrophages under hyperoxic conditions (32). By applying a too liberal O2 therapy, we may be losing the opportunity to control for a significant risk factor for VAP, a complications that occurred in almost 50% of patients in our cohort.
In our study, the use of prolonged prone positioning (sessions of up to 36 consecutive hours) was also associated with an increased risk of VAP. From a theoretical point of view, prone positioning could prevent the onset of VAP by facilitating the drain of respiratory secretions and limiting ventilator-induced lung injury, although most clinical studies showed no significant impact on the actual incidence of VAP (33). Several factors may explain the association observed, including the need for prolonged administration of NMBA and deep sedation, the increase in abdominal pressure with a higher risk of aspiration of gastric content, the need to limit the dose of enteral nutrition. ICU-acquired weakness is a frequent complication in COVID-19 patients, especially in those who received higher doses of NMBA and sedatives and lower caloric/protein intake, and may be responsible for a longer duration of mechanical ventilation and prolonged exposure to the risk of VAP (34).
Our study has several limitations. First, the retrospective design that does not allow to define a cause-effect relationship between the exposure to hyperoxia and mortality or VAP, but only enables to describe associations. Second, the relatively low sample size and the involvement of a single center, which limits the generalizability of our results. The sample size limited the number of confounders that could be included in multivariate regression models, since the inclusion of too many independent variables would lead to a mathematically unstable outcome (20). Third, we based our analysis on ABG data that can only provide a partial picture of the exposure to hyperoxemia, limited to the moment in which the ABG was made. Unfortunately, we could not collect SpO2 data, which could have been useful for a more continuative evaluation of the oxygenation status and the responses of clinicians to SpO2 values above the target. Fourth, the calculation of the “ideal FiO2” and “excess O2” can only provide an imprecise estimate of the amount of O2 administered in excess. In fact, factors such as PEEP, prone positioning or use of iNO will determine the most appropriate FiO2 of the patient by influencing gas exchange: therefore, calculating the ideal FiO2 merely on the basis of the PaO2/FiO2 ratio may be reductive. Despite its limitations, to the best of our knowledge, this is the first study that describes the prevalence of hyperoxemia in mechanically ventilated COVID-19 patients and explores the potential effects of excess O2 doses on outcome. Future larger studies are needed to confirm our findings.
Conclusion
In mechanically ventilated patients with SARS-CoV-2 pneumonia admitted to our ICU, the administration of O2 was often excessive in comparison to the PaO2 target indicated by the guidelines, and the exposure to hyperoxemia was frequent. In addition, most episodes of hyperoxemia were not followed by a reduction in the FiO2; changes were made more frequently in the presence of higher PaO2 or higher initial FiO2. The prevalence of hyperoxemia was independently associated with a greater risk of ICU-mortality, as well as with a greater risk of developing VAP. The retrospective nature of our study does not allow to draw conclusions on a possible cause-effect relationship between the exposure to excessive amounts of O2 and outcome. However, these data add to the ample literature that warns against the possible deleterious effects of a too liberal O2 therapy. In the absence of strong evidence of the safety of hyperoxemia in critically ill patients (in particular in those with ARDS and SARS-CoV-2 pneumonia), more efforts should be made to avoid the exposure to excessive amounts of supplemental O2. Further studies are needed to define the best oxygenation target for this patient category.
Data availability statement
The raw data supporting the conclusions of this article will be made available by the authors, without undue reservation.
Ethics statement
The studies involving human participants were reviewed and approved by Comitato Etico Regionale Marche. Written informed consent for participation was not required for this study in accordance with the national legislation and the institutional requirements.
Author contributions
ED contributed to the study design, analysis, and interpretation of data and drafted the manuscript. EC, AC, EA, and AD contributed to the study design and interpretation of the data and revised the manuscript critically. GM, SV, RG, RD, and CS participated in the acquisition and analysis of the data. All authors have agreed both to be personally accountable for the author’s own contributions and to ensure that questions related to the accuracy or integrity of any part of the work, even ones in which the author was not personally involved, are appropriately investigated, resolved, and the resolution documented in the literature and approved the submitted version of the manuscript.
Conflict of interest
The authors declare that the research was conducted in the absence of any commercial or financial relationships that could be construed as a potential conflict of interest.
Publisher’s note
All claims expressed in this article are solely those of the authors and do not necessarily represent those of their affiliated organizations, or those of the publisher, the editors and the reviewers. Any product that may be evaluated in this article, or claim that may be made by its manufacturer, is not guaranteed or endorsed by the publisher.
Acknowledgments
We wish to thank the medical and nurse staff for their help in the realization of this study and their end-less efforts during the COVID-19 pandemic.
References
1. Damiani E, Donati A, Girardis M. Oxygen in the critically ill: friend or foe? Curr Opin Anaesthesiol. (2018) 31:129–35. doi: 10.1097/ACO.0000000000000559
2. Chu DK, Kim LH, Young PJ, Zamiri N, Almenawer SA, Jaeschke R, et al. Mortality and morbidity in acutely ill adults treated with liberal versus conservative oxygen therapy (IOTA): a systematic review and meta-analysis. Lancet. (2018) 391:1693–705. doi: 10.1016/S0140-6736(18)30479-3
3. Girardis M, Busani S, Damiani E, Donati A, Rinaldi L, Marudi A, et al. Effect of conservative vs conventional oxygen therapy on mortality among patients in an intensive care unit: the oxygen-ICU randomized clinical trial. JAMA. (2016) 316:1583–9. doi: 10.1001/jama.2016.11993
4. Panwar R, Hardie M, Bellomo R, Barrot L, Eastwood GM, Young PJ, et al. Conservative versus liberal oxygenation targets for mechanically ventilated patients – a pilot multicenter randomized controlled trial. Am J Resp Crit Care Med. (2016) 193:43–51. doi: 10.1164/rccm.201505-1019OC
5. Bailey TC, Martin EL, Zhao L, Veldhuizen RAW. High oxygen concentrations predispose mouse lungs to the deleterious effects of high stretch ventilation. J Appl Physiol. (2003) 94:975–82. doi: 10.1152/japplphysiol.00619.2002
6. Six S, Jaffal K, Ledoux G, Jaillette E, Wallet F, Nseir S. Hyperoxemia as a risk factor for ventilator-associated pneumonia. Crit Care. (2016) 20:195. doi: 10.1186/s13054-016-1368-4
7. ARDSnet. NIH NHLBI ARDS Clinical Network Mechanical Ventilation Protocol Summary (2008). Available online at: http://www.ardsnet.org/files/ventilator_protocol_2008-07.pdf (accessed March 2022).
8. Rachmale S, Li G, Wilson G, Malinchoc M, Gajic O. Practice of excessive F(IO(2)) and effect on pulmonary outcomes in mechanically ventilated patients with acute lung injury. Respir Care. (2012) 57:1887–93. doi: 10.4187/respcare.01696
9. Madotto F, Rezoagli E, Pham T, Schmidt M, McNicholas B, Protti A, et al. Hyperoxemia and excess oxygen use in early acute respiratory distress syndrome: insights from the LUNG SAFE study. Crit Care. (2020) 24:125. doi: 10.1186/s13054-020-2826-6
10. Aggarwal NR, Brower RG, Hager DN, Thompson BT, Netzer G, Shanholtz G, et al. Oxygen exposure resulting in arterial oxygen tensions above the protocol goal was associated with worse clinical outcomes in acute respiratory distress syndrome. Crit Care Med. (2018) 46:517–24. doi: 10.1097/CCM.0000000000002886
11. Barrot L, Asfar P, Mauny F, Winiszewski H, Montini F, Badie J, et al. Liberal or conservative oxygen therapy for acute respiratory distress syndrome. N Engl J Med. (2020) 382:999–1008. doi: 10.1056/NEJMoa1916431
12. Grasselli G, Zangrillo A, Zanella A, Antonelli M, Cabrini L, Castelli A, et al. Baseline characteristics and outcomes of 1591 patients infected with SARS-CoV-2 admitted to ICUs of the Lombardy region, Italy. JAMA. (2020) 323:1574–81. doi: 10.1001/jama.2020.5394
13. Ziehr DR, Alladina J, Petri CR, Maley JH, Moskowitz A, Medoff BD, et al. Respiratory pathophysiology of mechanically ventilated patients with COVID-19: a cohort study. Am J Respir Crit Care Med. (2020) 201:1560–4. doi: 10.1164/rccm.202004-1163LE
14. Fan E, Brodie D, Slutsky AS. Acute respiratory distress syndrome: advances in diagnosis and treatment. JAMA. (2018) 319:698–710. doi: 10.1001/jama.2017.21907
15. Nasa P, Azoulay E, Khanna AK, Jain R, Gupta S, Javeri Y, et al. Expert consensus statements for the management of COVID-19-related acute respiratory failure using a Delphi method. Crit Care. (2021) 25:106. doi: 10.1186/s13054-021-03491-y
16. Carsetti A, Damia Paciarini A, Marini B, Pantanetti S, Adrario E, Donati A. Prolonged prone position ventilation for SARS-CoV-2 patients is feasible and effective. Crit Care. (2020) 24:225. doi: 10.1186/s13054-020-02956-w
17. Elso Guidelines for Cardiopulmonary Extracorporeal Life Support. Extracorporeal Life Support Organization, Version 1.4 August 2017 (2017). Available online at: www.elso.org (accessed March 2022).
18. Kalanuria AA, Zai W, Mirski M. Ventilator-associated pneumonia in the ICU. Crit Care. (2014) 18:208. doi: 10.1186/cc13775
19. American Thoracic Society, Infectious Diseases Society of America. Guidelines for the management of adults with hospital-acquired, ventilator-associated, and healthcare-associated pneumonia. Am J Respir Crit Care Med. (2005) 171:388–416. doi: 10.1164/rccm.200405-644ST
20. Stoltzfus JC. Logistic regression: a brief primer. Acad Emerg Med. (2011) 18:1099–104. doi: 10.1111/j.1553-2712.2011.01185.x
21. De Graaf AE, Dongelmans DA, Binnekade JM, de Jonge E. Clinicians’ response to hyperoxia in ventilated patients in a Dutch ICU depends on the level of FiO2. Intensive Care Med. (2011) 37:46–51. doi: 10.1007/s00134-010-2025-z
22. Suzuki S, Eastwood GM, Peck L, Glassford NJ, Bellomo R. Current oxygen management in mechanically ventilated patients: a prospective observational cohort study. J Crit Care. (2013) 28:647–54. doi: 10.1016/j.jcrc.2013.03.010
23. Gomes EP, Reboredo MM, Costa GB, Barros FS, Carvalho EV, Pinheiro BV. Impacts of a fraction of inspired oxygen adjustment protocol in covid-19 patients under mechanical ventilation: a prospective cohort study. Med Intensiva. (2022). doi: 10.1016/j.medin.2022.04.004
24. Helmerhorst HJF, Schultz MJ, van der Voort PHJ, Bosman RJ, Juffermans RP, de Jonge E, et al. Self-reported attitudes versus actual practice of oxygen therapy by ICU physicians and nurses. Ann Intensive Care. (2014) 4:23. doi: 10.1186/s13613-014-0023-y
25. Davis WB, Rennard SI, Bitterman PB, Crystal RG. Pulmonary oxygen toxicity. Early reversible changes in human alveolar structures induced by hyperoxia. N Engl J Med. (1983) 309:878–83. doi: 10.1056/NEJM198310133091502
26. Zenri H, Rodriquez-Capote K, McCaig L, Yao LJ, Brackenbury A, Possmayer F, et al. Hyperoxia exposure impairs surfactant function and metabolism. Crit Care Med. (2004) 32:1155–60.
27. Barbateskovic M, Schjørring OL, Russo Krauss S, Jakobsen JC, Meyhoff CS, Dahl RM, et al. Higher versus lower fraction of inspired oxygen or targets of arterial oxygenation for adults admitted to the intensive care unit. Cochrane Database Syst Rev. (2019) 2019:CD012631.
28. Schjørring OL, Klitgaard TL, Perner A, Wetterslev J, Lange T, Siegemund M, et al. Lower or higher oxygenation targets for acute hypoxemic respiratory failure. N Engl J Med. (2021) 384:1301–11.
29. Nußbaum B, Radermacher P, Asfar P, Hartmann C. Does hyperoxia enhance susceptibility to secondary pulmonary infection in the ICU? Crit Care. (2016) 20:239.
30. Entezari M, Weiss DJ, Sitapara R, Whittaker L, Wargo MJ, Li J, et al. Inhibition of high-mobility group box 1 protein (HMGB1) enhances bacterial clearance and protects against Pseudomonas aeruginosa pneumonia in cystic fibrosis. Mol Med. (2012) 18:477–85. doi: 10.2119/molmed.2012.00024
31. Patel VS, Sitapara RA, Gore A, Phan B, Sharma L, Sampat V, et al. High mobility group box-1 mediates hyperoxia-induced impairment of Pseudomonas aeruginosa clearance and inflammatory lung injury in mice. Am J Respir Cell Mol Biol. (2013) 48:280–7. doi: 10.1165/rcmb.2012-0279OC
32. Saito K, Kimura S, Saga T, Misonoo Y, Yoshizawa S, Akasaka Y, et al. Protective effect of procysteine on Acinetobacter pneumonia in hyperoxic conditions. J Antimicrob Chemother. (2013) 68:2305–10. doi: 10.1093/jac/dkt192
33. Alexiou VG, Ierodiakonou V, Dimopoulos G, Falagas ME. Impact of patient position on the incidence of ventilator-associated pneumonia: a meta-analysis of randomized controlled trials. J Crit Care. (2009) 24:515–22. doi: 10.1016/j.jcrc.2008.09.003
Keywords: COVID-19, SARS-CoV-2, ARDS, hyperoxia, oxygen, ventilator-associated pneumonia
Citation: Damiani E, Casarotta E, Carsetti A, Mariotti G, Vannicola S, Giorgetti R, Domizi R, Scorcella C, Adrario E and Donati A (2022) Too much tolerance for hyperoxemia in mechanically ventilated patients with SARS-CoV-2 pneumonia? Report from an Italian intensive care unit. Front. Med. 9:957773. doi: 10.3389/fmed.2022.957773
Received: 31 May 2022; Accepted: 06 July 2022;
Published: 28 July 2022.
Edited by:
Alfonso J. Rodriguez-Morales, Fundacion Universitaria Autónoma de las Américas, ColombiaReviewed by:
Ming Horng Tsai, Yunlin Chang Gung Memorial Hospital, TaiwanAlexandre Naime Barbosa, São Paulo State University, Brazil
Copyright © 2022 Damiani, Casarotta, Carsetti, Mariotti, Vannicola, Giorgetti, Domizi, Scorcella, Adrario and Donati. This is an open-access article distributed under the terms of the Creative Commons Attribution License (CC BY). The use, distribution or reproduction in other forums is permitted, provided the original author(s) and the copyright owner(s) are credited and that the original publication in this journal is cited, in accordance with accepted academic practice. No use, distribution or reproduction is permitted which does not comply with these terms.
*Correspondence: Erica Adrario, ZS5hZHJhcmlvQHVuaXZwbS5pdA==