- 1Basic and Molecular Epidemiology of Gastrointestinal Disorders Research Center, Research Institute for Gastroenterology and Liver Diseases, Shahid Beheshti University of Medical Sciences, Tehran, Iran
- 2Department 4 – Materials and the Environment, Bundesanstalt für Materialforschung und -prüfung (BAM), Berlin, Germany
- 3Department of Biology Chemistry Pharmacy, Freie Universität Berlin, Berlin, Germany
Vaccination is defined as the stimulation and development of the adaptive immune system by administering specific antigens. Vaccines' efficacy, in inducing immunity, varies in different societies due to economic, social, and biological conditions. One of the influential biological factors is gut microbiota. Cross-talks between gut bacteria and the host immune system are initiated at birth during microbial colonization and directly control the immune responses and protection against pathogen colonization. Imbalances in the gut microbiota composition, termed dysbiosis, can trigger several immune disorders through the activity of the adaptive immune system and impair the adequate response to the vaccination. The bacteria used in probiotics are often members of the gut microbiota, which have health benefits for the host. Probiotics are generally consumed as a component of fermented foods, affect both innate and acquired immune systems, and decrease infections. This review aimed to discuss the gut microbiota's role in regulating immune responses to vaccination and how probiotics can help induce immune responses against pathogens. Finally, probiotic-based oral vaccines and their efficacy have been discussed.
Introduction
Vaccination is defined as the stimulation and development of the adaptive immune system by the administration of specific antigens. Vaccines help prevent and eradicate the mortality and morbidity of numerous infectious diseases (1). Vaccine efficacy (VE) is described as the incidence proportion between the vaccinated and non-vaccinated populations (2) and varies in different societies based on economic, social, and biological conditions (3, 4). Several suggested economic and social determinants, such as country income status, living conditions and access to healthcare appear to act indirectly and non-specifically on VE. In contrast, many but not all biological factors, such as co-infections, malnutrition, and enteropathy, presumably, act directly and proximally on VE (5). Gut microbiota also plays a crucial role in the development and regulation of the immune system; hence, its composition might affect how individuals respond to vaccinations (6, 7).
Gut microbiota develops alongside host development and is affected by genetics and environmental factors, and is an integral part of the human body (8, 9). The microbiota interacts with the host in many ways. Cross-talks between gut bacteria and the host immune system are initiated at birth during microbial colonization (10). This interaction promotes the intestinal epithelial barrier, immune homeostasis, protects from pathogen colonization (11), and inhibits deleterious inflammatory reactions that would harm both the host and its gut microbiota (12). Gut lymph nodes, lamina propria, and epithelial cells (mucosal immune system) form a protective barrier for the integrity of the intestinal tract (13). Therefore the gut microbiota composition can affect the normal mucosal immune system (14).
During gut microbiota development, especially in early life, various factors can affect and alter its composition. For instance, the human gut changes considerably during the first 2 years of life as children grow from breast milk-dominated diets to solid foods and are exposed to vast numbers of bacterial species (15). Therefore undernourished children have been reported to have less mature gut microbiota compared to healthy children (16). Diet serves as a significant factor in gut microbiota composition in adults too. Various studies reported that a higher-fat diet in healthy adults appeared to be associated with unfavorable changes in gut microbiota, fecal metabolomics profiles, and plasma pro inflammatory factors, which might result in long-term adverse consequences for health (17–19). In addition, metabolic diseases such as diabetes can alter the gut microbiome and disrupt gut bacterial equilibrium (20). Other factors, including physical activity, mental health, and obesity may also affect gut microbiota composition (21–23).
Imbalances in the gut microbiota composition, termed dysbiosis, can trigger several immune disorders through the activity of the adaptive immune system (24). For example, recent studies on this subject reported that germ-free (GF) mice had a reduced number of Th1 and Th17 cells. Th17 cells, which are grouped as CD4+ effector T cells that secrete IL-17, play an important role in host defense against extracellular pathogens and the development of autoimmune diseases (25–27). Moreover, in dysbiotic gut microbiota, the number of inducible Foxp3 Helio-Tregs (iTregs) is reduced significantly in colonic lamina propria (28). Other studies indicate that excessive use of antibiotics disrupting gut microbiota hemostasis in young children might delay or impair the proper development of IgG response and immune memory that profoundly impacts adulthood (29). This review highlighted studies about the relationship between gut microbiota and related immune responses after vaccination and the impact of gut microbiota dysbiosis on VE.
Gut microbiota and vaccine efficacy
Cross-talk between the gut microbiome and the immune system by producing various metabolites and antimicrobial peptides directly regulates innate and adaptive immunity (30) and its failure to regulate inflammatory responses could increase the risk of developing inflammatory conditions in the gastrointestinal tract (31). Therefore the gut microbiota impacts the efficacy of various immune system-related interventions, including prevention of human immunodeficiency virus (HIV) infection (32, 33), cancer immunotherapy (34–36), and dysregulation in gut microbial composition associated with autoantibodies production and autoimmune diseases (37–40). Several studies were designed to evaluate the relationship between gut microbiota and immune responses to assess vaccine efficiency. A study by Pulendran et al. showed that antibiotic consumption resulted in a 10,000-fold reduction in gut bacterial composition and reduced specific neutralization and binding antibody responses against the influenza vaccine, and a significant association between bacterial species and metabolic phenotypes in the gut was displayed in this study (41). Furthermore, infants who received oral polio vaccine (OPV), intramuscular tetanus-hepatitis B, and intradermal Bacillus Calmette–Guérin (BCG) vaccines had detectable levels of Bifidobacterium longum (B. longum) and displayed higher specific T cell responses, serum IgG and fecal polio-specific IgA levels. In contrast, a higher relative abundance of Enterobacteriales and Pseudomonadales was associated with lower specific T cell responses and serum IgG levels (6, 42). Another study on infants receiving BCG, OPV, tetanus toxoid (TT), and hepatitis B virus confirmed the previous results that Bifidobacterium abundance in early infancy might increase the protective effects of vaccines by enhancing immunologic memory (7). The concurrent presence of non-polio enterovirus (NPEV) and oral polio vaccination can affect VE and reduce OPV seroconversion (43).
One of the critical factors in VE is the expression of Toll-like receptor 5 (TLR5) within 3 days after vaccination, which correlates to the amount of hemagglutination inhibition (HAI) titers 4 weeks after influenza vaccination (44, 45). TLR5 is a cell receptor for the recognition of flagellin and stimulates inflammatory signaling and immune responses (46). In addition, trivalent inactivated influenza vaccination of Trl5–/– mice resulted in reduced antibody titers. TLR5-mediated sensing of the microbiota also impacted antibody responses to the inactivated polio vaccine (47). NOD2 (Nucleotide-binding oligomerization domain 2), an intracellular pathogen recognition sensor, is associated with the immune system and VE stimulation (48, 49). Recognition of symbiotic bacteria by NOD2 in CD11c-expressing phagocytes helps the mucosal adjuvant activity of cholera toxin (CT), as confirmed by a study on mice (50).
One of the most influential factors that lead to dysregulation of gut microbiota dysbiosis is antibiotic exposure (51). In 1 study, it is demonstrated that antibiotics-induced dysbiosis in infant mice (but not adults) leads to impaired antibody responses and promotes ex vivo cytokine recall responses (52). Antibiotic-treated mice models also showed impaired oral immunization in response to cholera toxin (53) and dysregulation in the generation of anti-viral macrophages, virus-specific CD4 and CD8 T cells, and antibody responses following respiratory influenza virus infection (54, 55). Gut dysbiosis induced by antibiotics significantly decreased the activation of CD4+ T cells and CD8+ T cells and declined the level of memory of CD4+ T cells and CD8+ T cells in secondary lymphoid organs of the vaccinated animals (56). In a study on human adults with impaired microbiome induced by antibiotics, reduced antibody response to TIV in subjects with low pre-existing immunity to influenza virus was observed (41). However, adults receiving Rotavirus (RV), Pneumo23, and TT vaccines with antibiotics consumption showed increased fecal shedding of RV and changes in gut bacteria beta diversity which is associated with RV vaccine immunogenicity boosting (57). Although antibiotics consumption could not improve the immunogenicity of OPV in human infants, the reduction of enteropathy and pathogenic intestinal bacteria biomarkers were reported (58).
The composition of gut microbiota and its diversity are associated with the response of the immune system to vaccines. In this case, a study on specific pathogen-free layer chickens (SPF) showed that shifts in gut microbiota composition might result in changes in cell- and antibody-mediated immune responses to vaccination against influenza viruses (59, 60). Other experiments on adults receiving an HIV vaccine showed the immunogenicity of the vaccine was correlated with microbiota clusters (61). On the contrary, another study on human adults reported no differences in overall gut microbiota community diversity between humoral responders and non-responders to the oral Salmonella Typhi vaccine (62). Co-infection with porcine reproductive and respiratory syndrome virus (PRRSV) and porcine circovirus type 2 (PCV2) in pig models revealed that high growth outcomes were associated with several gut microbiome characteristics, such as increased bacterial diversity, increased relative abundance of Bacteroides pectinophilus, decreased Mycoplasmataceae species diversity, higher Firmicutes:Bacteroidetes ratios, increased relative abundance of the phylum Spirochaetes, reduced relative abundance of the family Lachnospiraceae, and increased Lachnospiraceae species (63). Diet is also influential on the gut microbiome and vaccine efficacy. A study showed that a gluten-free diet was associated with a reduced anti-tetanus IgG response, and it increased the relative abundance of the anti-inflammatory Bifidobacterium in the mice model (64).
Humans harbor several latent viruses, including cytomegalovirus (CMV) implicated in the modulation of host immunity (65). However, there is an insufficient understanding of the influence of lifelong persistent latent viral infections on the immune system (66). In a rhesus macaques model, subclinical CMV infection increased butyrate-producing bacteria and lower antibody responses to influenza vaccination (67).
Oral RV vaccines have the potential role in reducing the morbidity and mortality of RV infection that causes diarrhea-related death in children worldwide, but RV vaccines showed significantly lower efficacy in low-income countries (68, 69). A comparison between infants in India and Malawi and infants born in the UK showed that ORV immune response was significantly impaired among infants in the former. This result is linked with their gut microbiome composition, in which microbiota diversity was significantly higher among Malawian infants, while Indian infants had high Bifidobacterium abundance (70). Despite low RVV immunogenicity which was also reported in rural Zimbabwean infants, it was not associated with the composition or function of the early-life gut microbiome (71). Human gut microbiota transplanted pig models vaccinated with attenuated RVV showed significantly enhanced IFN-γ producing T cell responses and reduced regulatory T cells and cytokine production (72). Moreover, poor diet decreased total Ig and HRV-specific IgG and IgA antibody titers in serum or ileum and it increased fecal virus shedding titers in human infant microbiome transplanted pig models (57, 73, 74). In a study on rural Ghana's infants, RVV response was associated with an increased relative abundance of Streptococcus gallolyticus, decreased relative abundance of phylum Bacteroidetes and higher Enterobacteria/Bacteroides ratio (75). Another study reported that RVV response correlates with a higher relative abundance of bacteria belonging to Clostridium cluster XI and Proteobacteria (76). Bacteroides thetaiotaomicron is also associated with anti-rotavirus IgA titer (77). However, a study on Nicaraguan Infants reported a limited impact of gut microbial taxa on response to oral RVV (78).
Recent studies indicated that dysbiosis might be relevant in systemic severe acute respiratory syndrome coronavirus 2 (SARS-CoV-2) infections. Khan et al. indicated an association between dysbiosis and severe inflammatory response in coronavirus disease 2019 (COVID-19) patients. Decreased Firmicutes/Bacteroidetes ratio, induced by the depletion of Faecalibacterium prausnitzii (F. prausnitzii), Bacteroides plebeius (B. plebeius), and Prevotella, which utilize fiber, and a relative increase in Bacteroidetes species is associated with raised serum IL-21 levels and better prognosis (79). A study on a cohort of 100 patients revealed that the composition of the gut microbiome in patients with COVID-19 correlates with disease severity, plasma concentrations of several inflammatory cytokines, and tissue-damaged associated chemokines. Patients with COVID-19 are recommended to consume beneficial microorganisms with immunomodulatory potentials, such as F. prausnitzii, Eubacterium rectale, and several Bifidobacterium species, and the dysbiosis persisted after the clearance of the virus (80, 81). Currently, controlling and preventing the spread of SARS-CoV2 infection is one of the critical challenges in the healthcare system. Vaccination is the best strategy to overcome this challenge (82). Among all recently proposed vaccines, an important note is to balance the humoral (neutralizing antibody) and T cell responses (83). Mucosal immunity is the most influential factor in preventing viral respiratory infections and response to vaccination. In this regard, the intestinal immune system is as important as the respiratory system's mucosal immunity (84). Thus, the intestinal immune system might be a promising approach for improving current SARS-CoV2 vaccination strategies (85). On the other hand, risk factors that reduce the immune system's defenses against SARS-CoV-2 infections could also reduce their responses to vaccination and increase vaccination's adverse effects. Thus gut dysbiosis is one of the mechanisms that can cause a pathological and impaired immune response to SARS-CoV-2 vaccination (86).
So far, most studies around vaccine efficacy and gut microbiota composition demonstrated that gut microbiota can influence vaccines' immunogenicity and the mucosal and acquired immunity against pathogens.
The effects of probiotics on vaccine efficacy
Probiotics are live commensal microorganisms that have positive benefits for the host that are generally consumed as a component of fermented foods. They have an impact on both innate and adaptive immune systems and decrease infections (87, 88). A meta-analysis comprising 1,979 adults showed that probiotics and prebiotics effectively promote immunogenicity by influencing seroconversion and seroprotection rates in adults vaccinated with influenza vaccines (89).
Bifidobacteria (BIF) is one of the probiotics and beneficial bacteria for human and animal health, having roles in the prevention of infection, modulation of lipid metabolism, and reduction of allergic symptoms by stimulating the host's mucosal immune system and systemic immune response (90, 91). Consumption of B. longum BB536 in newborns showed an increase in the number of interferon-γ (IFN-γ), a representative cytokine for T helper 1 response, secretion cells, and the ratio of IFN-γ/IL-4 secretion cells (92). In addition, a combination of B. longum BL999 and Lactobacillus rhamnosus (L. rhamnosus) [LPR (CGMCC1.3724)] consumption after Hepatitis B vaccination resulted in improved antibody responses (93). The results of a study on adults who received seasonal influenza vaccines was the same. Probiotic consumption (B. longum bv. infantis CCUG 52,486, combined with a prebiotic gluco-oligosaccharide) could improve total antibody titers and seroprotection (94). Bifidobacterium lactis BB-12 and Lactobacillus paracasei (L. paracasei) 431 improved specific Antibody titers and seroconversion rates after influenza vaccination but there was no difference in INF-γ, IL-2, and IL-10 levels (95). In a randomized placebo-controlled, double-blinded prospective trial, the effect of probiotics [Bifidobacterium bifidum, B. infantis, B. longum, and Lactobacillus acidophilus (L. acidophilus)] on vaccination efficacy could not be proven statistically (96).
Strains of Lactobacillus are a subdominant component of the commensal human intestinal microbiota and are identified as a potential driving force in the development of the human immune system (97). They exert early immunostimulatory effects that may be directly linked to the initial inflammation responses in human macrophages (98). Chickens who received Lactobacillus spp as probiotics showed an increased major histocompatibility complex (MHC) II expression on macrophages and B cells. The number of CD4 + CD25 + T regulatory cells was also reduced in the spleen (99). In a study, the probiotic function of Lactobacillus plantarum (L. plantarum) was assessed and the results showed that fecal secretory immunoglobulin A (sIgA) titer significantly increased in the probiotic group infants (100). Another study on chicken models showed that a mixture of probiotic Lactobacillus spp can enhance IFN-γ gene expression but does not influence antibody production after influenza vaccination (101). Consumption of probiotics containing Lactobacillus acidophilus; Lactobacillus plantarum; Pediococcus pentosaceus; Saccharomyces cerevisiae; Bacillus subtilis, and Bacillus licheniformis in broiler chickens resulted in the diminished adverse effect of live vaccine, reduced mortality rate, fecal shedding, and re-isolation of Salmonella Enteritidis (SE) from liver, spleen, heart, and cecum against SE vaccine (102). On this subject, oral administration of L.plantarum GUANKE (LPG) on mice models acted as a booster for COVID-19 vaccination and boosted >8-fold specific neutralization antibodies in bronchoalveolar lavage (BAL) and >2-fold in serum (103). An in-vitro and in-silico study showed that L.plantarum could reduce inflammatory markers such as IFN-α, IFN-β, and IL-6 and block virus replication by interaction with SARS-CoV-2 helicase (104). L. acidophilus W37 (LaW37) with long-chain inulin (lcITF) was also used as a probiotic in a study on piglets and increased two-folded vaccine efficacy against Salmonella Typhimurium strains (STM) (105).
A pilot study on adults who received the influenza vaccine reported that L. rhamnosus GG (LGG) could be an influential adjuvant to improve influenza vaccine immunogenicity (106). LGG also improves T cell responses but not antibody production on human gut microbiota (HGM) transplanted gnotobiotic (Gn) pig model vaccinated with AttHRV (72). However, specific RV antibody production was stimulated in infants who received LGG (107). Another study confirms that the combination of L. acidophilus CRL431 and LGG enhanced IgA and IgM (but not IgG) production after OP vaccination (108).
Other types of probiotics have been studied on this subject as well. For example, Escherichia coli Nissle (EcN) 1917 was used to colonize antibiotic-treated and human infant fecal microbiota transplanted Gn piglets and immune response was evaluated to human Rotavirus (HRV). As a result, the humoral and cellular immune responses were enhanced, and EcN biofilm increased the frequencies of systemic memory and IgA + B cells (109, 110). Likewise, the Lactococcus lactis strain decreased severity and symptoms in volunteers with Dengue fever (DF) compared to the placebo group, promoted IFN-γ and TGF-β cytokines secretion, and reduced serum IgE and IL-4 cytokine levels in mice models (111, 112). Bacillus toyonensis (B. toyonensis) BCT-7112 was also enabled to improve the humoral immune response of ewes against the clostridium perfringens epsilon toxin (rETX) vaccine and boost higher neutralizing antibody titers (113). B. toyonensis and Saccharomyces boulardii also successfully boosted antibody production and expression of IFN-γ, IL2, and Bcl6 genes in Clostridium chauvoei vaccinated sheep (114). Likewise, Bacillus velezensis significantly reduced the pigeon circovirus (PiCV) viral load in the feces and spleen of pigeons and promoted TLR 2&4 expression (115). Fecal microbiome transplantation with Clostridium butyricum and Saccharomyces boulardii treatment in piglets not only improved plasma concentrations of IL-23, IL-17, IL-22 and specific antibodies against Mycoplasma hyopneumoniae (M. hyo) and Porcine Circovirus Type 2 (PCV2), but also decreased the inflammation levels and oxidative stress injury, and improved intestinal barrier function (116).
Although several studies reported a positive effect of Lactobacillus on VE, some studies yielded different results. For example, maternal LGG supplementation showed decreased specific antibody responses in tetanus, Haemophilus influenza type b (Hib), and pneumococcal conjugate (PCV7) vaccinated infants (117). Also, probiotic consumption containing Lactobacillus strains (L. paracasei and Lactobacillus casei (L. casei) 431 showed no effects on the immune response to the influenza vaccine but shortened the duration of respiratory symptoms (118). Another study on L. paracasei and MoLac-1 (heat-killed) supplemented diet reported the same results, and these probiotics could not boost immune responses after vaccination (119). A recent study also assessed LGG consumption impact on influenza vaccine efficacy in type 1 diabetic (T1D) children and reported no significant improvement in humoral response in the probiotic group (120). In conclusion, although some studies show that probiotics are inefficient in boosting the immune system and increasing vaccine efficacy, most studies demonstrated the positive effects of probiotics on promoting vaccine immunity and protecting the gut barrier simultaneously (Table 1).
Probiotic-based vaccines
One efficient way to increase VE, produce a better immune response to an antigen, and reduce attenuated vaccine risk is to utilize recombinant antigens in gut microbiota vectors. Based on this idea, several probiotic-based vaccines were developed (Figure 1). For instance, the recombinant Streptococcus gordonii RJM4 vector has been used to express the N-terminal fragment of the S1 subunit of pertussis toxin (PT) as a SpaP/S1 fusion protein in mice. SIgA in saliva and IgG were detected, and long-term oral colonization and maintenance of recombinant protein were observed in these animal models (121). The B subunit of the heat-labile toxin (LTB) was one of the antigen targets that colonized Bacillus subtilis (B. subtilis) with episomal expression systems. Vaccinated mice with engineered B. subtilis via the oral route could be recognized and neutralize the native toxin, produced by enterotoxigenic Escherichia coli (ETEC) strains in vitro (122). B. subtilis was also used as a shuttle for Clonorchis sinensis antigen. Compared with control groups, the results indicated that the vaccinated group could induce humoral and cellular immune responses successfully (123). Furthermore, another vaccine against ETEC strains, the probiotic E. coli Nissle 1917 (EcN) was used to express Stx B-subunits, OspA, and OspG protein antigens. This system could elicit hormonal responses but could not trigger selective T-cell responses against selected antigens (124). On the other hand, EcN 1917 expressing heterologous F4 or F4 and F18 fimbriae of ETEC improved anti-F4 and both anti-F4 and anti-F18 IgG immune responses (125).
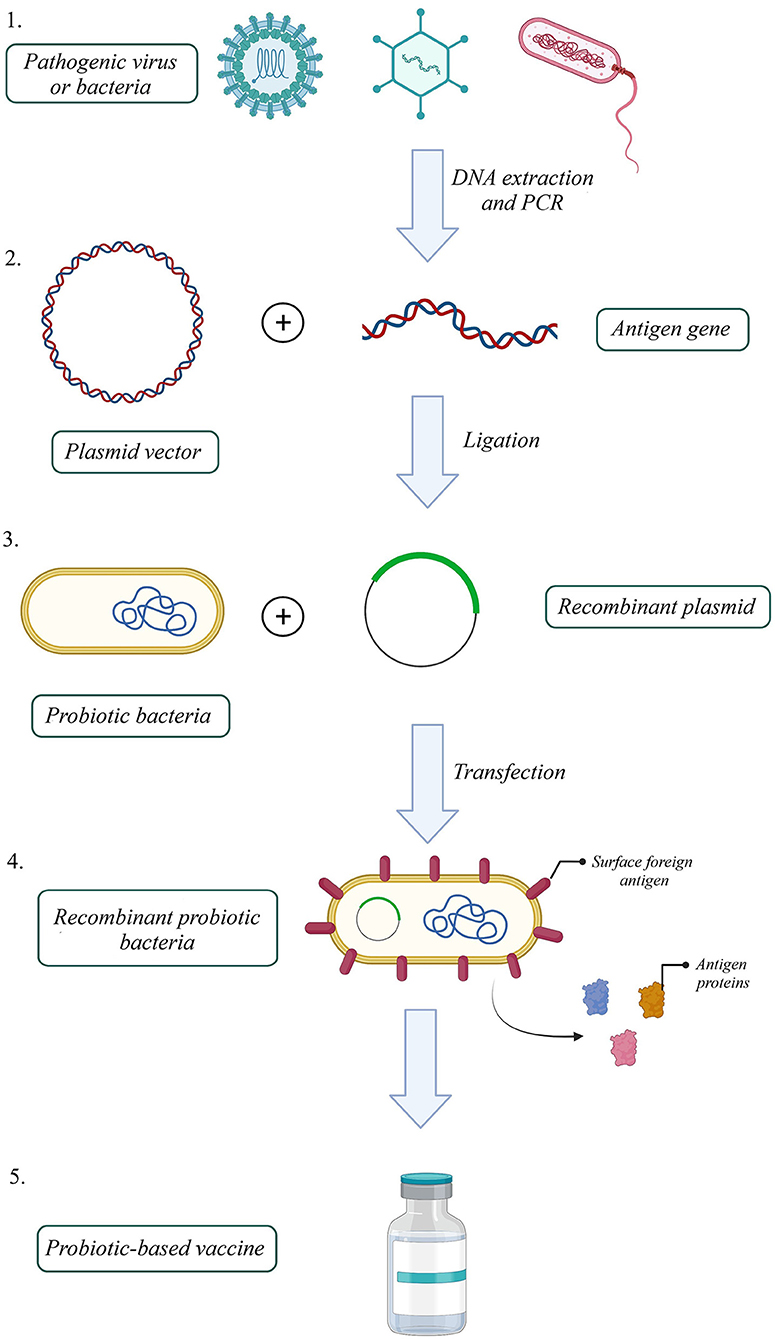
Figure 1. How to build a probiotic-based vaccine: 1. Extract the antigen gene from the pathogen, 2. Amplify the gene by polymerase chain reaction (PCR), 3. Build a recombinant expression plasmid by ligating antigen gene into a proper plasmid, 4. Transfect recombinant plasmid into a probiotic host, 5. Select successfully transfected recombinant probiotic bacteria 6. Probiotic-based oral vaccines could be manufactured with a recombinant probiotic host expressing the pathogenic antigen (Created with BioRender.com).
Lactococcus lactis is a commonly used food-grade probiotic. To develop a vaccine against Helicobacter pylori, L. lactis expressing Helicobacter pylori urease subunit B (UreB) was used and results demonstrated that orally vaccinated mice elicited significant humoral immunity against gastric Helicobacter infection (126). Tang et al., designed a recombinant L. lactis expressing TGEV spike glycoprotein. Results on mice revealed induction of local mucosal immune responses and IgG and IgA antibodies production against TGEV spike glycoprotein (127). On this subject, L. lactis PppA (LPA+) recombinant strain containing pneumococcal protective protein A (LPA) in oral immunized mice showed mucosal and systemic antibody production against different serotypes of Streptococcus pneumonia (128). L. lactis was likewise used to deliver rotavirus spike-protein subunit VP8 in the mouse model. The serum of animals that received L. lactis with cell wall-anchored RV VP8 antigen could inhibit viral infection in vitro by 100% and vaccinated mice developed significant levels of intestinal IgA antibodies in vivo (129). The oral vaccine with L. Lactis expressing a recombinant fusion protein of M1 and HA2 proteins derived from the H9N2 virus successfully induces protective mucosal and systemic immunity in eighty 1-day-old chickens (130). Mohseni et al. employed L. lactis as a vector for expressing the codon-optimized human papillomavirus (HPV) - 16 E7 oncogenes, and it showed cytotoxic T lymphocytes (CTL), and humoral responses after vaccination in healthy women volunteers with this probiotic-based vaccine (131). Similarly, another study on L. lactis expressing HPV codon-optimized E6 protein reported induction of humoral and cellular immunity and significantly increased intestinal mucosal lymphocytes, splenocytes, and vaginal lymphocytes in the vaccinated group compared to controls (132).
Lactobacillus casei strains are known for their immune stimulatory effect and have been used as probiotics for many years. A genetically engineered L. casei oral vaccine expressing dendritic cell (DC)-targeting peptide for Porcine epidemic diarrhea (PED) resulted in significantly elevated levels of anti-PEDV specific IgG and IgA antibody responses in mice and piglets (133, 134). Yoon et al. expressed poly-glutamic acid synthetase A (pgsA) protein from HPV-16 L2 in L. casei, and interestingly, L2-specific antibodies had cross-neutralizing activity against diverse HPV types in the mouse model (135). Recombinant L. casei was also used for immunizing piglets against TGEV. As a result, solid cellular response, switching from Th1 to Th2-based immune responses, and IL-17 expression in systemic and mucosal immunity was reported (136). In another study, α, ε, β1, and β2 toxoids of Clostridium perfringens expressed in L. casei ATCC 393 vector and elevated the levels of antigen-specific mucosa sIgA and sera IgG antibodies with exotoxin-neutralizing activity were seen in rabbit models (137). A different study used this probiotic expressing the VP2 protein of infectious pancreatic necrosis virus (IPNV) and reported induction of local mucosal and systemic immune responses in rainbow trout juveniles (138).
Other strains of lactobacillus are used in this technique as well. Oral recombinant Lactobacillus vaccine containing VP7 antigen of porcine rotavirus (PRV) showed stimulation in the differentiation of dendritic cells (DCs) in Peyer's patches (PPs) significantly, increased serum levels of IL-4 and IFN-γ and production of B220+ B cells in mesenteric lymph nodes (MLNs). Also, it increased the titer levels of the VP7-specific antibodies in mice models (139). Recombinant L. Plantarum expressing H9N2 avian influenza virus used for specific pathogen-free (SPF) 3-week-old chickens and could elicit humoral and cellular immunity (140). Shi et al. showed excessive serum titers of hemagglutination-inhibition (HI) antibodies in mice, and robust T cell immune responses in both mouse and chicken H9N2 vaccinated models by Recombinant L. Plantarum (141). L. Plantarum NC8, expressing oral rabies vaccine G protein fused with a DC-targeting peptide (DCpep), resulted in more functional maturation of DCs and a strong Th1-biased immune response in mice (142). A recent study utilized L. Plantarum for developing SARS-CoV-2 food-grade oral vaccine. The results indicated that the spike gene could be efficiently expressed on the surface of recombinant L. Plantarum and displayed high antigenicity (143). As a novel approach for vaccination against SARS-Cov2, L. plantarum strain expressing the SARS-CoV-2 spike protein was used, and high yields for S protein were obtained in an engineered probiotic group in vitro (143). In murine models, Lactobacillus pentosus expressing D antigenic site of spike glycoprotein transmissible gastroenteritis coronavirus (TGEV) could induce IgG and sIgA against this virus (144). Recombinant Lactobacillus rhamnosus that contains Koi herpesvirus (KHV) ORF81 protein in vaccinated fish was also successfully generated antigen-specific IgM with KHV-neutralizing activity (145). Another study used Lactobacillus acidophilus vector with the membrane-proximal external region from HIV-1 (MPER) and secreted interleukin-1ß (IL-1ß) or expressed the surface flagellin subunit C (FliC) as adjuvants, and reported as an improved vaccine efficacy and immune response against HIV-1 in mice (146). These studies demonstrated that probiotics have a potential role in acting as a shuttle for recombinant oral vaccines and successfully promoting the immune system against pathogens, and improving intestinal condition simultaneously.
Future perspective
There is no doubt that gut microbiota significantly impacts human metabolism and the immune system. Even further, some scientists consider gut microbiota as an endocrine organ in the human body. Probiotics are part of gut microbiota that have health benefits and promote immune responses. Based on the impact of gut mucosal immunity in the humoral immune response to vaccination, using probiotics as an immune booster next to oral vaccines can lead to better immunity, and probiotic-based recombinant vaccines promise a better generation of recombinant vaccines. Although a few human studies were performed on this subject, probiotics and probiotic-based recombinant vaccines' efficacy on immunity against pathogens is promising. Such a new oral vaccine against SARS-CoV-2 infection was developed by Symvivo Corporation (a Vancouver-based Biotech Company) using Bifidobacteria longum, for expressing spike protein (named bacTRL-Spike), and it is under investigation in phase 1 clinical trials (NCT04334980). However, more studies need to be performed to detect the effectiveness of probiotics and engineered probiotic vaccines in clinical trials and investigate their role in human immunological pathways to ensure their safety and durable immunity.
Author contributions
NK: literature search, writing, and drawing of figures. AD and SB: literature search. All authors contributed to the article and approved the submitted version.
Conflict of interest
The authors declare that the research was conducted in the absence of any commercial or financial relationships that could be construed as a potential conflict of interest.
Publisher's note
All claims expressed in this article are solely those of the authors and do not necessarily represent those of their affiliated organizations, or those of the publisher, the editors and the reviewers. Any product that may be evaluated in this article, or claim that may be made by its manufacturer, is not guaranteed or endorsed by the publisher.
References
1. Kocourkova A, Honegr J, Kuca K, Danova J. Vaccine ingredients: components that influence vaccine efficacy. Mini Rev Med Chem. (2017) 17:451–66. doi: 10.2174/1389557516666160801103303
2. Carpenter TE. Evaluation of effectiveness of a vaccination program against an infectious disease at the population level. Am J Vet Res. (2001) 62:202–5. doi: 10.2460/ajvr.2001.62.202
3. Desselberger U. Differences of rotavirus vaccine effectiveness by country: likely causes and contributing factors. Pathogens. (2017) 6:65. doi: 10.3390/pathogens6040065
4. Jiang V, Jiang B, Tate J, Parashar UD, Patel MM. Performance of rotavirus vaccines in developed and developing countries. Hum Vaccin. (2010) 6:532–42. doi: 10.4161/hv.6.7.11278
5. Kaslow DC. Force of infection: a determinant of vaccine efficacy? NPJ Vaccines. (2021) 6:51. doi: 10.1038/s41541-021-00316-5
6. Huda MN, Lewis Z, Kalanetra KM, Rashid M, Ahmad SM, Raqib R, et al. Stool microbiota and vaccine responses of infants. Pediatrics. (2014) 134:e362–72. doi: 10.1542/peds.2013-3937
7. Huda MN, Ahmad SM, Alam MJ, Khanam A, Kalanetra KM, Taft DH, et al. Bifidobacterium abundance in early infancy and vaccine response at 2 years of age. Pediatrics. (2019) 143:1489. doi: 10.1542/peds.2018-1489
8. David LA, Maurice CF, Carmody RN, Gootenberg DB, Button JE, Wolfe BE, et al. Diet rapidly and reproducibly alters the human gut microbiome. Nature. (2014) 505:559–63. doi: 10.1038/nature12820
9. Zhang C, Yin A, Li H, Wang R, Wu G, Shen J, et al. Dietary modulation of gut microbiota contributes to alleviation of both genetic and simple obesity in children. EBioMedicine. (2015) 2:968–84. doi: 10.1016/j.ebiom.2015.07.007
10. Adak A, Khan MR. An insight into gut microbiota and its functionalities. Cell Mol Life Sci. (2019) 76:473–93. doi: 10.1007/s00018-018-2943-4
11. Pickard JM, Zeng MY, Caruso R, Núñez G. Gut microbiota: Role in pathogen colonization, immune responses, and inflammatory disease. Immunol Rev. (2017) 279:70–89. doi: 10.1111/imr.12567
12. Gaboriau-Routhiau V, Cerf-Bensussan N. Gut microbiota and development of the immune system. Med Sci (Paris). (2016) 32:961–7. doi: 10.1051/medsci/20163211011
13. Richards JL, Yap YA, McLeod KH, Mackay CR, Mariño E. Dietary metabolites and the gut microbiota: an alternative approach to control inflammatory and autoimmune diseases. Clin Transl Immunol. (2016) 5:e82. doi: 10.1038/cti.2016.29
14. Shi N, Li N, Duan X, Niu H. Interaction between the gut microbiome and mucosal immune system. Mil Med Res. (2017) 4:14. doi: 10.1186/s40779-017-0122-9
15. Olszak T, An D, Zeissig S, Vera MP, Richter J, Franke A, et al. Microbial exposure during early life has persistent effects on natural killer T cell function. Science. (2012) 336:489–93. doi: 10.1126/science.1219328
16. Kortekangas E, Kamng'ona AW, Fan YM, Cheung YB, Ashorn U, Matchado A, et al. Environmental exposures and child and maternal gut microbiota in rural Malawi. Paediatr Perinat Epidemiol. (2020) 34:161–70. doi: 10.1111/ppe.12623
17. Wan Y, Wang F, Yuan J, Li J, Jiang D, Zhang J, et al. Effects of dietary fat on gut microbiota and faecal metabolites, and their relationship with cardiometabolic risk factors: a 6-month randomised controlled-feeding trial. Gut. (2019) 68:1417–29. doi: 10.1136/gutjnl-2018-317609
18. Bibbò S, Ianiro G, Giorgio V, Scaldaferri F, Masucci L, Gasbarrini A, et al. The role of diet on gut microbiota composition. Eur Rev Med Pharmacol Sci. (2016) 20:4742–9.
19. Zhang M, Yang XJ. Effects of a high fat diet on intestinal microbiota and gastrointestinal diseases. World J Gastroenterol. (2016) 22:8905–9. doi: 10.3748/wjg.v22.i40.8905
20. Karusheva Y, Koessler T, Strassburger K, Markgraf D, Mastrototaro L, Jelenik T, et al. Short-term dietary reduction of branched-chain amino acids reduces meal-induced insulin secretion and modifies microbiome composition in type 2 diabetes: a randomized controlled crossover trial. Am J Clin Nutr. (2019) 110:1098–107. doi: 10.1093/ajcn/nqz191
21. Uemura M, Hayashi F, Ishioka K, Ihara K, Yasuda K, Okazaki K, et al. Obesity and mental health improvement following nutritional education focusing on gut microbiota composition in Japanese women: a randomised controlled trial. Eur J Nutr. (2019) 58:3291–302. doi: 10.1007/s00394-018-1873-0
22. Motiani KK, Collado MC, Eskelinen JJ, Virtanen KA, LÖyttyniemi E, Salminen S, et al. Exercise Training Modulates Gut Microbiota Profile and Improves Endotoxemia. Med Sci Sports Exerc. (2020) 52:94–104. doi: 10.1249/MSS.0000000000002112
23. Pasini E, Corsetti G, Assanelli D, Testa C, Romano C, Dioguardi FS, et al. Effects of chronic exercise on gut microbiota and intestinal barrier in human with type 2 diabetes. Minerva Med. (2019) 110:3–11. doi: 10.23736/S0026-4806.18.05589-1
24. Honda K, Littman DR. The microbiota in adaptive immune homeostasis and disease. Nature. (2016) 535:75–84. doi: 10.1038/nature18848
25. Hirota K, Ahlfors H, Duarte JH, Stockinger B. Regulation and function of innate and adaptive interleukin-17-producing cells. EMBO Rep. (2012) 13:113–20. doi: 10.1038/embor.2011.248
26. Dutzan N, Kajikawa T, Abusleme L, Greenwell-Wild T, Zuazo CE, Ikeuchi T, et al. A dysbiotic microbiome triggers T(H)17 cells to mediate oral mucosal immunopathology in mice and humans. Sci Transl Med. (2018) 10:eaat0797. doi: 10.1126/scitranslmed.aat0797
27. Ji J, Ge X, Chen Y, Zhu B, Wu Q, Zhang J, et al. Daphnetin ameliorates experimental colitis by modulating microbiota composition and T(reg)/T(h)17 balance. FASEB J. (2019) 33:9308–22. doi: 10.1096/fj.201802659RR
28. Smith PM, Howitt MR, Panikov N, Michaud M, Gallini CA, Bohlooly YM, et al. The microbial metabolites, short-chain fatty acids, regulate colonic Treg cell homeostasis. Science. (2013) 341:569–73. doi: 10.1126/science.1241165
29. Zeng MY, Inohara N, Nuñez G. Mechanisms of inflammation-driven bacterial dysbiosis in the gut. Mucosal Immunol. (2017) 10:18–26. doi: 10.1038/mi.2016.75
30. Baradaran Ghavami S, Pourhamzeh M, Farmani M, Raftar SKA, Shahrokh S, Shpichka A, et al. Cross-talk between immune system and microbiota in COVID-19. Expert Rev Gastroenterol Hepatol. (2021) 15:1281–94. doi: 10.1080/17474124.2021.1991311
31. Vitetta L, Briskey D, Alford H, Hall S, Coulson S. Probiotics, prebiotics and the gastrointestinal tract in health and disease. Inflammopharmacology. (2014) 22:135–54. doi: 10.1007/s10787-014-0201-4
32. Liu H, Gu R, Li W, Zhou W, Cong Z, Xue J, et al. Lactobacillus rhamnosus GG attenuates tenofovir disoproxil fumarate-induced bone loss in male mice via gut-microbiota-dependent anti-inflammation. Ther Adv Chronic Dis. (2019) 10:2040622319860653. doi: 10.1177/2040622319860653
33. Klatt NR, Cheu R, Birse K, Zevin AS, Perner M, Noël-Romas L, et al. Vaginal bacteria modify HIV tenofovir microbicide efficacy in African women. Science. (2017) 356:938–45. doi: 10.1126/science.aai9383
34. Gopalakrishnan V, Spencer CN, Nezi L, Reuben A, Andrews MC, Karpinets TV, et al. Gut microbiome modulates response to anti-PD-1 immunotherapy in melanoma patients. Science. (2018) 359:97–103.
35. Routy B, Le Chatelier E, Derosa L, Duong CPM, Alou MT, Daillère R, et al. Gut microbiome influences efficacy of PD-1-based immunotherapy against epithelial tumors. Science. (2018) 359:91–7. doi: 10.1126/science.aan3706
36. Baruch EN, Youngster I, Ben-Betzalel G, Ortenberg R, Lahat A, Katz L, et al. Fecal microbiota transplant promotes response in immunotherapy-refractory melanoma patients. Science. (2021) 371:602–9. doi: 10.1126/science.abb5920
37. Ruff WE, Dehner C, Kim WJ, Pagovich O, Aguiar CL, Yu AT, et al. Pathogenic autoreactive T and B cells cross-react with mimotopes expressed by a common human gut commensal to trigger autoimmunity. Cell Host Microbe. (2019) 26:100–13.e8. doi: 10.1016/j.chom.2019.05.003
38. López P, de Paz B, Rodríguez-Carrio J, Hevia A, Sánchez B, Margolles A, et al. Th17 responses and natural IgM antibodies are related to gut microbiota composition in systemic lupus erythematosus patients. Sci Rep. (2016) 6:24072. doi: 10.1038/srep24072
39. Qian X, Liu YX, Ye X, Zheng W, Lv S, Mo M, et al. Gut microbiota in children with juvenile idiopathic arthritis: characteristics, biomarker identification, and usefulness in clinical prediction. BMC Genomics. (2020) 21:286. doi: 10.1186/s12864-020-6703-0
40. Miyake S, Kim S, Suda W, Oshima K, Nakamura M, Matsuoka T, et al. Dysbiosis in the gut microbiota of patients with multiple sclerosis, with a striking depletion of species belonging to clostridia XIVa and IV clusters. PLoS ONE. (2015) 10:e0137429. doi: 10.1371/journal.pone.0137429
41. Hagan T, Cortese M, Rouphael N, Boudreau C, Linde C, Maddur MS, et al. Antibiotics-driven gut microbiome perturbation alters immunity to vaccines in humans. Cell. (2019) 178:1313–28.e13. doi: 10.1016/j.cell.2019.08.010
42. Mullié C, Yazourh A, Thibault H, Odou MF, Singer E, Kalach N, et al. Increased poliovirus-specific intestinal antibody response coincides with promotion of Bifidobacterium longum-infantis and Bifidobacterium breve in infants: a randomized, double-blind, placebo-controlled trial. Pediatr Res. (2004) 56:791–5. doi: 10.1203/01.PDR.0000141955.47550.A0
43. Praharaj I, Parker EPK, Giri S, Allen DJ, Silas S, Revathi R, et al. Influence of Nonpolio Enteroviruses and the Bacterial Gut Microbiota on Oral Poliovirus Vaccine Response: A Study from South India. J Infect Dis. (2019) 219:1178–86. doi: 10.1093/infdis/jiy568
44. Huleatt JW, Nakaar V, Desai P, Huang Y, Hewitt D, Jacobs A, et al. Potent immunogenicity and efficacy of a universal influenza vaccine candidate comprising a recombinant fusion protein linking influenza M2e to the TLR5 ligand flagellin. Vaccine. (2008) 26:201–14. doi: 10.1016/j.vaccine.2007.10.062
45. Nakaya HI, Wrammert J, Lee EK, Racioppi L, Marie-Kunze S, Haining WN, et al. Systems biology of vaccination for seasonal influenza in humans. Nat Immunol. (2011) 12:786–95. doi: 10.1038/ni.2067
46. Tahoun A, Jensen K, Corripio-Miyar Y, McAteer S, Smith DGE, McNeilly TN, et al. Host species adaptation of TLR5 signalling and flagellin recognition. Sci Rep. (2017) 7:17677. doi: 10.1038/s41598-017-17935-5
47. Oh JZ, Ravindran R, Chassaing B, Carvalho FA, Maddur MS, Bower M, et al. TLR5-mediated sensing of gut microbiota is necessary for antibody responses to seasonal influenza vaccination. Immunity. (2014) 41:478–92. doi: 10.1016/j.immuni.2014.08.009
48. Lupfer C, Thomas PG, Kanneganti TD. Nucleotide oligomerization and binding domain 2-dependent dendritic cell activation is necessary for innate immunity and optimal CD8+ T Cell responses to influenza A virus infection. J Virol. (2014) 88:8946–55. doi: 10.1128/JVI.01110-14
49. Deng W, Xie J. NOD2 signaling and role in pathogenic mycobacterium recognition, infection and immunity. Cell Physiol Biochem. (2012) 30:953–63. doi: 10.1159/000341472
50. Kim D, Kim YG, Seo SU, Kim DJ, Kamada N, Prescott D, et al. Nod2-mediated recognition of the microbiota is critical for mucosal adjuvant activity of cholera toxin. Nat Med. (2016) 22:524–30. doi: 10.1038/nm.4075
51. Vangay P, Ward T, Gerber Jeffrey S, Knights D. Antibiotics, pediatric dysbiosis, and disease. Cell Host Microbe. (2015) 17:553–64. doi: 10.1016/j.chom.2015.04.006
52. Lynn MA, Tumes DJ, Choo JM, Sribnaia A, Blake SJ, Leong LEX, et al. Early-life antibiotic-driven dysbiosis leads to dysregulated vaccine immune responses in mice. Cell Host Microbe. (2018) 23:653–60.e5. doi: 10.1016/j.chom.2018.04.009
53. Kim D, Kim YM, Kim WU, Park JH, Núñez G, Seo SU. Recognition of the microbiota by Nod2 contributes to the oral adjuvant activity of cholera toxin through the induction of interleukin-1β. Immunology. (2019) 158:219–29. doi: 10.1111/imm.13105
54. Ichinohe TI, Pang K, Kumamoto Y, Peaper DR, Ho JH, Murray TS, et al. Microbiota regulates immune defense against respiratory tract influenza A virus infection. Proc Natl Acad Sci USA. (2011) 108:5354–9. doi: 10.1073/pnas.1019378108
55. Abt MC, Osborne LC, Monticelli LA, Doering TA, Alenghat T, Sonnenberg GF, et al. Commensal bacteria calibrate the activation threshold of innate antiviral immunity. Immunity. (2012) 37:158–70. doi: 10.1016/j.immuni.2012.04.011
56. Nadeem S, Maurya SK, Das DK, Khan N, Agrewala JN. Gut dysbiosis thwarts the efficacy of vaccine against Mycobacterium tuberculosis. Front Immunol. (2020) 11:726. doi: 10.3389/fimmu.2020.00726
57. Michael H, Langel SN, Miyazaki A, Paim FC, Chepngeno J, Alhamo MA, et al. Malnutrition decreases antibody secreting cell numbers induced by an oral attenuated human rotavirus vaccine in a human infant fecal microbiota transplanted gnotobiotic pig model. Front Immunol. (2020) 11:196. doi: 10.3389/fimmu.2020.00196
58. Grassly NC, Praharaj I, Babji S, Kaliappan SP, Giri S, Venugopal S, et al. The effect of azithromycin on the immunogenicity of oral poliovirus vaccine: a double-blind randomised placebo-controlled trial in seronegative Indian infants. Lancet Infect Dis. (2016) 16:905–14. doi: 10.1016/S1473-3099(16)30023-8
59. Yitbarek A, Astill J, Hodgins DC, Parkinson J, Nagy É, Sharif S. Commensal gut microbiota can modulate adaptive immune responses in chickens vaccinated with whole inactivated avian influenza virus subtype H9N2. Vaccine. (2019) 37:6640–7. doi: 10.1016/j.vaccine.2019.09.046
60. Yitbarek A, Alkie T, Taha-Abdelaziz K, Astill J, Rodriguez-Lecompte JC, Parkinson J, et al. Gut microbiota modulates type I interferon and antibody-mediated immune responses in chickens infected with influenza virus subtype H9N2. Benef Microbes. (2018) 9:417–27. doi: 10.3920/BM2017.0088
61. Cram JA, Fiore-Gartland AJ, Srinivasan S, Karuna S, Pantaleo G, Tomaras GD, et al. Human gut microbiota is associated with HIV-reactive immunoglobulin at baseline and following HIV vaccination. PLoS ONE. (2019) 14:e0225622. doi: 10.1371/journal.pone.0225622
62. Eloe-Fadrosh EA, McArthur MA, Seekatz AM, Drabek EF, Rasko DA, Sztein MB, et al. Impact of oral typhoid vaccination on the human gut microbiota and correlations with s. Typhi-specific immunological responses. PLoS ONE. (2013) 8:e62026. doi: 10.1371/journal.pone.0062026
63. Constance LA, Thissen JB, Jaing CJ, McLoughlin KS, Rowland RR, Serão NV, et al. Gut microbiome associations with outcome following co-infection with porcine reproductive and respiratory syndrome virus (PRRSV) and porcine circovirus type 2 (PCV2) in pigs immunized with a PRRS modified live virus vaccine. Vet Microbiol. (2021) 254:109018. doi: 10.1016/j.vetmic.2021.109018
64. Kihl P, Krych L, Deng L, Hansen LH, Buschard K, Skov S, et al. Effect of gluten-free diet and antibiotics on murine gut microbiota and immune response to tetanus vaccination. PLoS ONE. (2022) 17:e0266719. doi: 10.1371/journal.pone.0266719
65. Cannon MJ, Schmid DS, Hyde TB. Review of cytomegalovirus seroprevalence and demographic characteristics associated with infection. Rev Med Virol. (2010) 20:202–13. doi: 10.1002/rmv.655
66. Brodin P, Jojic V, Gao T, Bhattacharya S, Angel CJ, Furman D, et al. Variation in the human immune system is largely driven by non-heritable influences. Cell. (2015) 160:37–47. doi: 10.1016/j.cell.2014.12.020
67. Santos Rocha C, Hirao LA, Weber MG, Méndez-Lagares G, Chang WLW, Jiang G, et al. Subclinical Cytomegalovirus Infection Is Associated with Altered Host Immunity, Gut Microbiota, and Vaccine Responses. J Virol. (2018) 92:e00167–18. doi: 10.1128/JVI.00167-18
68. Tate JE, Burton AH, Boschi-Pinto C, Steele AD, Duque J, Parashar UD. 2008 estimate of worldwide rotavirus-associated mortality in children younger than 5 years before the introduction of universal rotavirus vaccination programmes: a systematic review and meta-analysis. Lancet Infect Dis. (2012) 12:136–41. doi: 10.1016/S1473-3099(11)70253-5
69. Fischer Walker CL, Black RE. Rotavirus vaccine and diarrhea mortality: quantifying regional variation in effect size. BMC Public Health. (2011) 11 Suppl 3:S16. doi: 10.1186/1471-2458-11-S3-S16
70. Parker EP, Bronowski C, Sindhu KNC, Babji S, Benny B, Carmona-Vicente N, et al. Impact of maternal antibodies and microbiota development on the immunogenicity of oral rotavirus vaccine in African, Indian, and European infants. Nat Commun. (2021) 12:1–14. doi: 10.1038/s41467-021-27074-1
71. Robertson RC, Church JA, Edens TJ, Mutasa K, Geum HM, Baharmand I, et al. The fecal microbiome and rotavirus vaccine immunogenicity in rural Zimbabwean infants. Vaccine. (2021) 39:5391–400. doi: 10.1016/j.vaccine.2021.07.076
72. Wen K, Tin C, Wang H, Yang X, Li G, Giri-Rachman E, et al. Probiotic Lactobacillus rhamnosus GG enhanced Th1 cellular immunity but did not affect antibody responses in a human gut microbiota transplanted neonatal gnotobiotic pig model. PLoS ONE. (2014) 9:e94504. doi: 10.1371/journal.pone.0094504
73. Miyazaki A, Kandasamy S, Michael H, Langel SN, Paim FC, Chepngeno J, et al. Protein deficiency reduces efficacy of oral attenuated human rotavirus vaccine in a human infant fecal microbiota transplanted gnotobiotic pig model. Vaccine. (2018) 36:6270–81. doi: 10.1016/j.vaccine.2018.09.008
74. Srivastava V, Deblais L, Huang H-C, Miyazaki A, Kandasamy S, Langel S, et al. Reduced rotavirus vaccine efficacy in protein malnourished human-faecal-microbiota-transplanted gnotobiotic pig model is in part attributed to the gut microbiota. Benef Microbes. (2020) 11:733–51. doi: 10.3920/BM2019.0139
75. Harris VC, Armah G, Fuentes S, Korpela KE, Parashar U, Victor JC, et al. Significant correlation between the infant gut microbiome and rotavirus vaccine response in rural ghana. J Infect Dis. (2017) 215:34–41. doi: 10.1093/infdis/jiw518
76. Harris V, Ali A, Fuentes S, Korpela K, Kazi M, Tate J, et al. Rotavirus vaccine response correlates with the infant gut microbiota composition in Pakistan. Gut Microbes. (2018) 9:93–101. doi: 10.1080/19490976.2017.1376162
77. Robertson RC, Church JA, Edens TJ, Mutasa K, Geum HM, Baharmand I, et al. The gut microbiome and rotavirus vaccine immunogenicity in rural Zimbabwean infants. medRxiv. 2021:2021.03.24.21254180. (2021). doi: 10.1101/2021.03.24.21254180
78. Fix J, Chandrashekhar K, Perez J, Bucardo F, Hudgens MG, Yuan L, et al. Association between Gut Microbiome Composition and Rotavirus Vaccine Response among Nicaraguan Infants. Am J Trop Med Hyg. (2020) 102:213–9. doi: 10.4269/ajtmh.19-0355
79. Khan M, Mathew BJ, Gupta P, Garg G, Khadanga S, Vyas AK, et al. Gut Dysbiosis and IL-21 Response in Patients with Severe COVID-19. Microorganisms. (2021) 9:1292. doi: 10.3390/microorganisms9061292
80. Yeoh YK, Zuo T, Lui GC, Zhang F, Liu Q, Li AY, et al. Gut microbiota composition reflects disease severity and dysfunctional immune responses in patients with COVID-19. Gut. (2021) 70:698–706. doi: 10.1136/gutjnl-2020-323020
81. Shenoy S. Gut microbiome, Vitamin D, ACE2 interactions are critical factors in immune-senescence and inflammaging: key for vaccine response and severity of COVID-19 infection. Inflamm Res. (2022) 71:13–26. doi: 10.1007/s00011-021-01510-w
82. Cao X. COVID-19: immunopathology and its implications for therapy. Nat Rev Immunol. (2020) 20:269–70. doi: 10.1038/s41577-020-0308-3
83. Cohen J. Vaccine designers take first shots at COVID-19. In: American Association for the Advancement of Science. (2020). doi: 10.1126/science.368.6486.14
84. Fu Y, Cheng Y, Wu Y. Understanding SARS-CoV-2-mediated inflammatory responses: from mechanisms to potential therapeutic tools. Virol Sin. (2020) 35:266–71. doi: 10.1007/s12250-020-00207-4
85. Moradi-Kalbolandi S, Majidzadeh AK, Abdolvahab MH, Jalili N, Farahmand L. The role of mucosal immunity and recombinant probiotics in SARS-CoV2 vaccine development. Probiotics Antimicrob Proteins. (2021) 13:1239–53. doi: 10.1007/s12602-021-09773-9
86. Chen J, Vitetta L, Henson JD, Hall S. The intestinal microbiota and improving the efficacy of COVID-19 vaccinations. J Funct Foods. (2021) 87:104850. doi: 10.1016/j.jff.2021.104850
87. Hill C, Guarner F, Reid G, Gibson GR, Merenstein DJ, Pot B, et al. Expert consensus document. The International Scientific Association for Probiotics and Prebiotics consensus statement on the scope and appropriate use of the term probiotic. Nat Rev Gastroenterol Hepatol. (2014) 11:506–14. doi: 10.1038/nrgastro.2014.66
88. Kanauchi O, Andoh A, AbuBakar S, Yamamoto N. Probiotics and paraprobiotics in viral infection: clinical application and effects on the innate and acquired immune systems. Curr Pharm Des. (2018) 24:710–7. doi: 10.2174/1381612824666180116163411
89. Lei WT, Shih PC, Liu SJ, Lin CY, Yeh TL. Effect of probiotics and prebiotics on immune response to influenza vaccination in adults: a systematic review and meta-analysis of randomized controlled trials. Nutrients. (2017) 9:1175. doi: 10.3390/nu9111175
90. Majamaa H, Isolauri E. Probiotics: a novel approach in the management of food allergy. J Allergy Clin Immunol. (1997) 99:179–85. doi: 10.1016/S0091-6749(97)70093-9
91. Makioka Y, Tsukahara T, Ijichi T, Inoue R. Oral supplementation of Bifidobacterium longum strain BR-108 alters cecal microbiota by stimulating gut immune system in mice irrespectively of viability. Biosci Biotechnol Biochem. (2018) 82:1180–7. doi: 10.1080/09168451.2018.1451738
92. Wu BB, Yang Y, Xu X, Wang WP. Effects of Bifidobacterium supplementation on intestinal microbiota composition and the immune response in healthy infants. World J Pediatr. (2016) 12:177–82. doi: 10.1007/s12519-015-0025-3
93. Soh SE, Ong DQ, Gerez I, Zhang X, Chollate P, Shek LP, et al. Effect of probiotic supplementation in the first 6 months of life on specific antibody responses to infant Hepatitis B vaccination. Vaccine. (2010) 28:2577–9. doi: 10.1016/j.vaccine.2010.01.020
94. Przemska-Kosicka A, Childs CE, Enani S, Maidens C, Dong H, Dayel IB, et al. Effect of a synbiotic on the response to seasonal influenza vaccination is strongly influenced by degree of immunosenescence. Immun Ageing. (2016) 13:6. doi: 10.1186/s12979-016-0061-4
95. Rizzardini G, Eskesen D, Calder PC, Capetti A, Jespersen L, Clerici M. Evaluation of the immune benefits of two probiotic strains Bifidobacterium animalis ssp. lactis, BB-12® and Lactobacillus paracasei ssp paracasei, L casei 431® in an influenza vaccination model: a randomised, double-blind, placebo-controlled study. Br J Nutr. (2012) 107:876–84. doi: 10.1017/S000711451100420X
96. Youngster I, Kozer E, Lazarovitch Z, Broide E, Goldman M. Probiotics and the immunological response to infant vaccinations: a prospective, placebo controlled pilot study. Arch Dis Child. (2011) 96:345–9. doi: 10.1136/adc.2010.197459
97. O'Callaghan J, O'Toole PW. Lactobacillus: host-microbe relationships. Curr Top Microbiol Immunol. (2013) 358:119–54. doi: 10.1007/82_2011_187
98. Rocha-Ramírez LM, Pérez-Solano RA, Castañón-Alonso SL, Moreno Guerrero SS, Ramírez Pacheco A, García Garibay M, et al. Probiotic lactobacillus strains stimulate the inflammatory response and activate human macrophages. J Immunol Res. (2017) 2017:4607491. doi: 10.1155/2017/4607491
99. Bavananthasivam J, Alizadeh M, Astill J, Alqazlan N, Matsuyama-Kato A, Shojadoost B, et al. Effects of administration of probiotic lactobacilli on immunity conferred by the herpesvirus of turkeys vaccine against challenge with a very virulent Marek's disease virus in chickens. Vaccine. (2021) 39:2424–33. doi: 10.1016/j.vaccine.2021.03.046
100. Kusumo PD, Bela B, Wibowo H, Munasir Z, Surono IS. Lactobacillus plantarum IS-10506 supplementation increases faecal sIgA and immune response in children younger than two years. Benef Microbes. (2019) 10:245–52. doi: 10.3920/BM2017.0178
101. Alqazlan N, Astill J, Taha-Abdelaziz K, Nagy É, Bridle B, Sharif S. Probiotic lactobacilli enhance immunogenicity of an inactivated H9N2 influenza virus vaccine in chickens. Viral Immunol. (2021) 34:86–95. doi: 10.1089/vim.2020.0209
102. El-Shall NA, Awad AM, El-Hack MEA, Naiel MA, Othman SI, Allam AA, et al. The simultaneous administration of a probiotic or prebiotic with live Salmonella vaccine improves growth performance and reduces fecal shedding of the bacterium in Salmonella-challenged broilers. Animals. (2019) 10:70. doi: 10.3390/ani10010070
103. Xu J, Ren Z, Cao K, Li X, Yang J, Luo X, et al. Boosting Vaccine-Elicited Respiratory Mucosal and Systemic COVID-19 Immunity in Mice With the Oral Lactobacillus plantarum. Front Nutr. (2021) 8:789242. doi: 10.3389/fnut.2021.789242
104. Rather IA, Choi S-B, Kamli MR, Hakeem KR, Sabir JS, Park Y-H, et al. Potential Adjuvant Therapeutic Effect of Lactobacillus plantarum Probio-88 Postbiotics against SARS-CoV-2. Vaccines. (2021) 9:1067. doi: 10.3390/vaccines9101067
105. Lépine AF, Konstanti P, Borewicz K, Resink J-W, de Wit NJ, Vos Pd, et al. Combined dietary supplementation of long chain inulin and Lactobacillus acidophilus W37 supports oral vaccination efficacy against Salmonella typhimurium in piglets. Sci Rep. (2019) 9:1–13. doi: 10.1038/s41598-019-54353-1
106. Davidson LE, Fiorino AM, Snydman DR, Hibberd PL. Lactobacillus GG as an immune adjuvant for live-attenuated influenza vaccine in healthy adults: a randomized double-blind placebo-controlled trial. Eur J Clin Nutr. (2011) 65:501–7. doi: 10.1038/ejcn.2010.289
107. Isolauri E, Joensuu J, Suomalainen H, Luomala M, Vesikari T. Improved immunogenicity of oral D x RRV reassortant rotavirus vaccine by Lactobacillus casei GG. Vaccine. (1995) 13:310–2. doi: 10.1016/0264-410X(95)93319-5
108. de Vrese M, Rautenberg P, Laue C, Koopmans M, Herremans T, Schrezenmeir J. Probiotic bacteria stimulate virus-specific neutralizing antibodies following a booster polio vaccination. Eur J Nutr. (2005) 44:406–13. doi: 10.1007/s00394-004-0541-8
109. Michael H, Paim FC, Langel SN, Miyazaki A, Fischer DD, Chepngeno J, et al. Escherichia coli Nissle 1917 Enhances Innate and Adaptive Immune Responses in a Ciprofloxacin-Treated Defined-Microbiota Piglet Model of Human Rotavirus Infection. mSphere. (2021) 6:e00074–21. doi: 10.1128/mSphere.00074-21
110. Michael H, Paim FC, Miyazaki A, Langel SN, Fischer DD, Chepngeno J, et al. Escherichia coli Nissle 1917 administered as a dextranomar microsphere biofilm enhances immune responses against human rotavirus in a neonatal malnourished pig model colonized with human infant fecal microbiota. PLoS ONE. (2021) 16:e0246193. doi: 10.1371/journal.pone.0246193
111. Khor C-S, Tsuji R, Lee H-Y. Nor'e S-S, Sahimin N, Azman A-S, et al. Lactococcus lactis strain plasma intake suppresses the incidence of dengue fever-like symptoms in healthy malaysians: a randomized, double-blind, placebo-controlled trial. Nutrients. (2021) 13:4507. doi: 10.3390/nu13124507
112. Vasiee A, Falah F, Sankian M, Tabatabaei-Yazdi F, Mortazavi SA. Oral immunotherapy using probiotic ice cream containing recombinant food-grade Lactococcus lactis which inhibited allergic responses in a BALB/c mouse model. J Immunol Res. (2020) 1–12. doi: 10.1155/2020/2635230
113. Santos FDS, Ferreira MRA, Maubrigades LR, Gonçalves VS, de Lara APS, Moreira C, et al. Bacillus toyonensis BCT-7112(T) transient supplementation improves vaccine efficacy in ewes vaccinated against Clostridium perfringens epsilon toxin. J Appl Microbiol. (2021) 130:699–706. doi: 10.1111/jam.14814
114. Santos FDS, Maubrigades LR, Gonçalves VS, Alves Ferreira MR, Brasil CL, Cunha RC, et al. Immunomodulatory effect of short-term supplementation with Bacillus toyonensis BCT-7112(T) and Saccharomyces boulardii CNCM I-745 in sheep vaccinated with Clostridium chauvoei. Vet Immunol Immunopathol. (2021) 237:110272. doi: 10.1016/j.vetimm.2021.110272
115. Tsai CY, Hu SY, Santos H, Catulin G, Tayo L, Chuang K. Probiotic supplementation containing Bacillus velezensis enhances expression of immune regulatory genes against pigeon circovirus in pigeons (Columba livia). J Appl Microbiol. (2021) 130:1695–704. doi: 10.1111/jam.14893
116. Xiang Q, Wu X, Pan Y, Wang L, Cui C, Guo Y, et al. Early-life intervention using fecal microbiota combined with probiotics promotes gut microbiota maturation, regulates immune system development, and alleviates weaning stress in piglets. Int J Mol Sci. (2020) 21:503. doi: 10.3390/ijms21020503
117. Licciardi PV, Ismail IH, Balloch A, Mui M, Hoe E, Lamb K, et al. Maternal Supplementation with LGG Reduces Vaccine-Specific Immune Responses in Infants at High-Risk of Developing Allergic Disease. Front Immunol. (2013) 4:381. doi: 10.3389/fimmu.2013.00381
118. Jespersen L, Tarnow I, Eskesen D, Morberg CM, Michelsen B, Bügel S, et al. Effect of Lactobacillus paracasei subsp. paracasei, L casei 431 on immune response to influenza vaccination and upper respiratory tract infections in healthy adult volunteers: a randomized, double-blind, placebo-controlled, parallel-group study. Am J Clin Nutr. (2015) 101:1188–96. doi: 10.3945/ajcn.114.103531
119. Akatsu H, Arakawa K, Yamamoto T, Kanematsu T, Matsukawa N, Ohara H, et al. Lactobacillus in jelly enhances the effect of influenza vaccination in elderly individuals. J Am Geriatr Soc. (2013) 61:1828–30. doi: 10.1111/jgs.12474
120. Bianchini S, Orabona C, Camilloni B, Berioli MG, Argentiero A, Matino D, et al. Effects of probiotic administration on immune responses of children and adolescents with type 1 diabetes to a quadrivalent inactivated influenza vaccine. Hum Vaccin Immunother. (2020) 16:86–94. doi: 10.1080/21645515.2019.1633877
121. Lee SF, Halperin SA, Wang H, MacArthur A. Oral colonization and immune responses to Streptococcus gordonii expressing a pertussis toxin S1 fragment in mice. FEMS Microbiol Lett. (2002) 208:175–8. doi: 10.1111/j.1574-6968.2002.tb11078.x
122. Paccez JD, Nguyen HD, Luiz WB, Ferreira RC, Sbrogio-Almeida ME, Schuman W, et al. Evaluation of different promoter sequences and antigen sorting signals on the immunogenicity of Bacillus subtilis vaccine vehicles. Vaccine. (2007) 25:4671–80. doi: 10.1016/j.vaccine.2007.04.021
123. Wang X, Chen W, Tian Y, Mao Q, Lv X, Shang M, et al. Surface display of Clonorchis sinensis enolase on Bacillus subtilis spores potentializes an oral vaccine candidate. Vaccine. (2014) 32:1338–45. doi: 10.1016/j.vaccine.2014.01.039
124. Remer KA, Bartrow M, Roeger B, Moll H, Sonnenborn U, Oelschlaeger TA. Split immune response after oral vaccination of mice with recombinant Escherichia coli Nissle 1917 expressing fimbrial adhesin K88. Int J Med Microbiol. (2009) 299:467–78. doi: 10.1016/j.ijmm.2009.03.003
125. Ou B, Jiang B, Jin D, Yang Y, Zhang M, Zhang D, et al. Engineered recombinant Escherichia coli probiotic strains integrated with F4 and F18 fimbriae cluster genes in the chromosome and their assessment of immunogenic efficacy in vivo. ACS Synth Biol. (2020) 9:412–26. doi: 10.1021/acssynbio.9b00430
126. Gu Q, Song D, Zhu M. Oral vaccination of mice against Helicobacter pylori with recombinant Lactococcus lactis expressing urease subunit B. FEMS Immunol Med Microbiol. (2009) 56:197–203. doi: 10.1111/j.1574-695X.2009.00566.x
127. Tang L, Li Y. Oral immunization of mice with recombinant Lactococcus lactis expressing porcine transmissible gastroenteritis virus spike glycoprotein. Virus Genes. (2009) 39:238–45. doi: 10.1007/s11262-009-0390-x
128. Villena J, Medina M, Racedo S, Alvarez S. Resistance of young mice to pneumococcal infection can be improved by oral vaccination with recombinant Lactococcus lactis. J Microbiol Immunol Infect. (2010) 43:1–10. doi: 10.1016/S1684-1182(10)60001-1
129. Marelli B, Perez AR, Banchio C, de Mendoza D, Magni C. Oral immunization with live Lactococcus lactis expressing rotavirus VP8 subunit induces specific immune response in mice. J Virol Methods. (2011) 175:28–37. doi: 10.1016/j.jviromet.2011.04.011
130. Sha Z, Shang H, Miao Y, Huang J, Niu X, Chen R, et al. Recombinant Lactococcus Lactis Expressing M1-HA2 Fusion Protein Provides Protective Mucosal Immunity Against H9N2 Avian Influenza Virus in Chickens. Front Vet Sci. (2020) 7:153. doi: 10.3389/fvets.2020.00153
131. Mohseni AH, Taghinezhad SS, Keyvani H. The First Clinical Use of a Recombinant Lactococcus lactis Expressing Human Papillomavirus Type 16 E7 Oncogene Oral Vaccine: A Phase I Safety and Immunogenicity Trial in Healthy Women Volunteers. Mol Cancer Ther. (2020) 19:717–27. doi: 10.1158/1535-7163.MCT-19-0375
132. Taghinezhad SS, Mohseni AH, Keyvani H, Razavilar V. Protection against human papillomavirus type 16-induced tumors in C57BL/6 mice by mucosal vaccination with Lactococcus lactis NZ9000 expressing E6 oncoprotein. Microb Pathog. (2019) 126:149–56. doi: 10.1016/j.micpath.2018.10.043
133. Wang X, Wang L, Huang X, Ma S, Yu M, Shi W, et al. Oral delivery of probiotics expressing dendritic cell-targeting peptide fused with porcine epidemic diarrhea virus COE antigen: a promising vaccine strategy against PEDV. Viruses. (2017) 9:312. doi: 10.3390/v9110312
134. Hou X, Jiang X, Jiang Y, Tang L, Xu Y, Qiao X, et al. Oral immunization against PEDV with recombinant lactobacillus casei expressing dendritic cell-targeting peptide fusing COE protein of PEDV in piglets. Viruses. (2018) 10:106. doi: 10.3390/v10030106
135. Yoon SW, Lee TY, Kim SJ, Lee IH, Sung MH, Park JS, et al. Oral administration of HPV-16 L2 displayed on Lactobacillus casei induces systematic and mucosal cross-neutralizing effects in Balb/c mice. Vaccine. (2012) 30:3286–94. doi: 10.1016/j.vaccine.2012.03.009
136. Jiang X, Hou X, Tang L, Jiang Y, Ma G, Li Y, et al. phase trial of the oral Lactobacillus casei vaccine polarizes Th2 cell immunity against transmissible gastroenteritis coronavirus infection. Appl Microbiol Biotechnol. (2016) 100:7457–69. doi: 10.1007/s00253-016-7424-9
137. Bai J, Qiao X, Ma Y, Han M, Jia S, Huang X, et al. Protection efficacy of oral bait probiotic vaccine constitutively expressing tetravalent toxoids against Clostridium perfringens exotoxins in livestock (rabbits). Vaccines. (2020) 8:17. doi: 10.3390/vaccines8010017
138. Duan K, Hua X, Wang Y, Wang Y, Chen Y, Shi W, et al. Oral immunization with a recombinant Lactobacillus expressing CK6 fused with VP2 protein against IPNV in rainbow trout (Oncorhynchus mykiss). Fish Shellfish Immunol. (2018) 83:223–31. doi: 10.1016/j.fsi.2018.09.034
139. Yao J, Li Z, Wang X, Xu P, Zhao L, Qian J. MiR-125a regulates chemo-sensitivity to gemcitabine in human pancreatic cancer cells through targeting A20. Acta Biochim Biophys Sin. (2016) 48:202–8. doi: 10.1093/abbs/gmv129
140. Yang WT, Yang GL, Yang X, Shonyela SM, Zhao L, Jiang YL, et al. Recombinant Lactobacillus plantarum expressing HA2 antigen elicits protective immunity against H9N2 avian influenza virus in chickens. Appl Microbiol Biotechnol. (2017) 101:8475–84. doi: 10.1007/s00253-017-8600-2
141. Shi SH, Yang WT, Yang GL, Zhang XK, Liu YY, Zhang LJ, et al. Lactobacillus plantarum vaccine vector expressing hemagglutinin provides protection against H9N2 challenge infection. Virus Res. (2016) 211:46–57. doi: 10.1016/j.virusres.2015.09.005
142. Wang J, Jiang H, Yang R, Zhang S, Zhao W, Hu J, et al. Construction and evaluation of recombinant Lactobacillus plantarum NC8 delivering one single or two copies of G protein fused with a DC-targeting peptide (DCpep) as novel oral rabies vaccine. Vet Microbiol. (2020) 251:108906. doi: 10.1016/j.vetmic.2020.108906
143. Wang M, Fu T, Hao J, Li L, Tian M, Jin N, et al. A recombinant Lactobacillus plantarum strain expressing the spike protein of SARS-CoV-2. Int J Biol Macromol. (2020) 160:736–40. doi: 10.1016/j.ijbiomac.2020.05.239
144. Di-Qiu L, Xin-Yuan Q, Jun-Wei G, Li-Jie T, Yan-Ping J, Yi-Jing L. Construction and characterization of Lactobacillus pentosus expressing the D antigenic site of the spike protein of Transmissible gastroenteritis virus. Can J Microbiol. (2011) 57:392–7. doi: 10.1139/w11-027
145. Huang X, Ma Y, Wang Y, Niu C, Liu Z, Yao X, et al. Oral Probiotic Vaccine Expressing Koi Herpesvirus (KHV) ORF81 Protein Delivered by Chitosan-Alginate Capsules Is a Promising Strategy for Mass Oral Vaccination of Carps against KHV Infection. J Virol. (2021) 95:e00415–21. doi: 10.1128/JVI.00415-21
Keywords: probiotics, vaccine, vaccine efficacy, probiotic-based vaccines, gut microbiota, adaptive immunity
Citation: Kazemifard N, Dehkohneh A and Baradaran Ghavami S (2022) Probiotics and probiotic-based vaccines: A novel approach for improving vaccine efficacy. Front. Med. 9:940454. doi: 10.3389/fmed.2022.940454
Received: 10 May 2022; Accepted: 07 September 2022;
Published: 13 October 2022.
Edited by:
Aldert Zomer, Utrecht University, NetherlandsReviewed by:
Ana Isabel Alvarez-Mercado, University of Granada, SpainNirmal Kumar Ganguly, Indraprastha Apollo Hospitals, India
Copyright © 2022 Kazemifard, Dehkohneh and Baradaran Ghavami. This is an open-access article distributed under the terms of the Creative Commons Attribution License (CC BY). The use, distribution or reproduction in other forums is permitted, provided the original author(s) and the copyright owner(s) are credited and that the original publication in this journal is cited, in accordance with accepted academic practice. No use, distribution or reproduction is permitted which does not comply with these terms.
*Correspondence: Shaghayegh Baradaran Ghavami, c2guYmdoYXZhbWlAeWFob28uY29t
†These authors share first authorship