- 1Departamento de Genética e Evolução, Universidade Federal de São Carlos, São Carlos, SP, Brazil
- 2Laboratório Central de Saúde Pública do Tocantins, Palmas, TO, Brazil
- 3Programa de Pós-graduação em Odontologia e Tecnologia Ambiental, Universidade de Ribeirão Preto, Ribeirão Preto, SP, Brazil
- 4Department of Vector Biology, Liverpool School of Tropical Medicine, Liverpool, United Kingdom
- 5Centro de Ciências Biológicas e da Saúde, Biodiversidade Tropical - BIOTROP, Universidade Federal de São Carlos, São Carlos, Brazil
Chryseobacterium indologenes is a non-glucose-fermenting Gram-negative bacillus. This emerging multidrug resistant opportunistic nosocomial pathogen can cause severe infections in neonates and immunocompromised patients. This study aimed to present the first detailed draft genome sequence of a multidrug-resistant C. indologenes strain isolated from the cerebrospinal fluid of an infant hospitalized at the Neonatal Intensive Care Unit of Brazilian Tertiary Hospital. We first analyzed the susceptibility of C. indologenes strain to different antibiotics using the VITEK 2 system. The strain demonstrated an outstanding resistance to all the antibiotic classes tested, including β-lactams, aminoglycosides, glycylcycline, and polymyxin. Next, C. indologenes was whole-genome-sequenced, annotated using Prokka and Rapid Annotation using Subsystems Technology (RAST), and screened for orthologous groups (EggNOG), gene ontology (GO), resistance genes, virulence genes, and mobile genetic elements using different software tools. The draft genome contained one circular chromosome of 4,836,765 bp with 37.32% GC content. The genomic features of the chromosome present numerous genes related to cellular processes that are essential to bacteria. The MDR C. indologenes revealed the presence of genes that corresponded to the resistance phenotypes, including genes to β-lactamases (blaIND–13, blaCIA–3, blaTEM–116, blaOXA–209, blaVEB–15), quinolone (mcbG), tigecycline (tet(X6)), and genes encoding efflux pumps which confer resistance to aminoglycosides (RanA/RanB), and colistin (HlyD/TolC). Amino acid substitutions related to quinolone resistance were observed in GyrA (S83Y) and GyrB (L425I and K473R). A mutation that may play a role in the development of colistin resistance was detected in lpxA (G68D). Chryseobacterium indologenes isolate harbored 19 virulence factors, most of which were involved in infection pathways. We identified 13 Genomic Islands (GIs) and some elements associated with one integrative and conjugative element (ICEs). Other elements linked to mobile genetic elements (MGEs), such as insertion sequence (ISEIsp1), transposon (Tn5393), and integron (In31), were also present in the C. indologenes genome. Although plasmids were not detected, a ColRNAI replicon type and the most resistance genes detected in singletons were identified in unaligned scaffolds. We provided a wide range of information toward the understanding of the genomic diversity of C. indologenes, which can contribute to controlling the evolution and dissemination of this pathogen in healthcare settings.
Introduction
Chryseobacterium indologenes is a non-motile, catalase-positive, oxidase-positive, indole-positive, non-glucose-fermenting Gram-negative bacilli (1) widely distributed in nature, but it is not normally present in the human microflora (2, 3). In humans, C. indologenes was isolated for the first time from a tracheal aspirate of a patient with ventilator-associated pneumonia (4). Although considered a relatively uncommon human pathogen, the number of hospital-acquired infections caused by C. indologenes has been increasing (5–8). Since C. indologenes can survive in inanimate objects, it may be isolated from hospital environments and cultured from specimens of sinks, indwelling vascular catheters, vials, feeding tubes, and other equipment that are in contact with fluids and water (9). Therefore, C. indologenes is considered a potential reservoir for various types of serious infections (8, 10), including pneumonia, meningitis, wound infection, intraabdominal infection, primary bacteremia, intravascular catheter–related bacteremia, and cellulitis (7, 11–14). Generally, major risk factors for C. indologene infection are patients with predisposing diseases, immunocompromised status, long-term broad-spectrum antibiotics treatment, and long-term hospitalization with indwelling devices (1, 15, 16).
Although the clinical significance and pathogenicity of C. indologenes are not well established (17, 18), C. indologenes is known to be naturally resistant to a wide variety of antibiotics including not only aminoglycosides, tetracyclines, chloramphenicol, macrolides, aminopenicillins, clindamycin, teicoplanin but also first-generation cephalosporins, aztreonam, ticarcillin-clavulanate, and carbapenems (10, 19–21). The most potent drugs reported against C. indologenes are quinolones (gatifloxacin and levofloxacin), minocycline, and trimethoprim-sulfamethoxazole (18, 22, 23); however, many studies show an alarming trend in resistance to those antibiotics (3, 9, 24).
Whole-genome sequencing (WGS) has become a well-established technique for high-resolution characterization of the genetic repertoire of bacterial pathogens, including antibiotic resistance, molecular epidemiology, and virulence (25). It is a promising technique for surveillance and monitoring infection control and outbreak cases of many microbial pathogens of interest in public health (26). Hence, we conducted the complete genome sequencing of MDR C. indologenes to understand the genomic diversity and genes responsible for antibiotic resistance and virulence. To the best of our knowledge, there is no published data on C. indologenes whole-genome sequencing from the cerebrospinal fluid sample of a hospitalized infant in Tocantins, Brazil.
Materials and methods
Patient and bacterial isolate
Chryseobacterium indologenes was isolated from the cerebrospinal fluid (CSF) of an infant hospitalized at the Neonatal Intensive Care Unit (NICU) of Hospital Geral de Palmas, Palmas, Tocantins, Brazil. This isolate was initially identified as C. indologenes by a clinical microbiology laboratory using conventional methods. It was sent to the Central Laboratory of Public Health of Tocantins (LACEN/TO) for species confirmation and drug susceptibility testing. LACEN is a healthcare facility in the Brazilian Ministry of Health that receives samples for the surveillance of antimicrobial resistance.
Bacteria information and antimicrobial susceptibility
Once the sample was received at LACEN, bacterial identification and drug susceptibility assays were performed by the Vitek 2 system (bioMérieux, Marcy-l’Etoile, France) and interpreted according to Clinical and Laboratory Standards Institute guidelines (27). Chryseobacterium indologenes isolate was tested for susceptibility against 16 antibiotics: ampicillin (AMP), ampicillin/sulbactam (SAM), piperacillin/tazobactam (TZP), cefuroximeaxetil (CXM-AX), cefoxitin (FOX), ceftazidime (CAZ), ceftriaxone (CRO), cefepime (FEP), ertapenem (ETP), imipenem (IPM), meropenem (MEM), amikacin (AMK), gentamicin (GEN), ciprofloxacin (CIP), tigecycline (TGC), and colistin (CST).
Minimum inhibitory concentration (MICs) of colistin and tigecycline was determined by the broth microdilution (BMD) method according to the EUCAST (28) recommendations. The results obtained with the VITEK 2 system were compared to those obtained by the BMD method.
Phenotypic detection for the production of carbapenemases was carried out by modified Hodge test, synergy test, and the ethylenediaminetetraacetic acid (EDTA) test under the CLSI guidelines (27) as described elsewhere (29–32). Multidrug-resistant (MDR) C. indologenes isolate was defined by non-susceptibility to at least one agent in three or more antibiotic categories (33).
DNA isolation and genome sequencing
Genomic DNA extraction was done in an overnight culture using the Wizard® Genomic DNA Purification Kit (Promega, Madison, WI, United States), according to the manufacturer’s instructions. The DNA extract concentration and purity were determined by measuring absorbance at wavelengths of 260 and 280 nm (NanoVue Plus; GE Healthcare Life Sciences, Marlborough, MA, United States). The integrity of genomic DNA was tested by way of electrophoresis. Bacterial DNA concentration was also measured fluorometrically (Qubit® 3.0, kit Qubit® dsDNA Broad Range Assay Kit, Life Technologies, Carlsbad, CA, United States). The sample from the isolate was prepared for sequencing using 1 ng of input genomic DNA. Nextera XT DNA Library Prep Kit (Illumina, San Diego, CA, United States) was used for library preparation. The libraries were amplified using a limited cycle PCR program. The PCR step adds Index 1(i7) adapters, Index 2 (i5) adapters, and sequences required for sequencing cluster generation. The purification of the amplified library was performed using 0.6x Agencourt AMPure XP beads (Beckman Coulter). For checking the library quality and size of fragmented DNA, the samples were evaluated on 1.5% electrophoresis agarose gel. The libraries were quantified with a fluorometric method Qubit® 3.0 using kit Qubit® dsDNA Broad Range Assay Kit, Life Technologies, Carlsbad, CA, United States) and normalized to 4 nM using a Standard Dilution Method. The libraries were pooled, denatured with 0.2 N NaOH, and diluted to the final concentration of 1.8 pM. A PhiX control was added to a final concentration of 1.5 pM. The run-length was a paired-end run of 75 cycles for each read (2 × 75), plus up to eight cycles each for two index reads.
Genome assembly and annotation
Raw reads were assessed for quality using FastQC v.0.11.9, a quality control tool for high throughput sequence data,1 and filtered for quality, length, and adapter regions using TrimGalore! v.0.6.5, a wrapper tool specific for FastQC.2 The de novo genome assembly was made with SPAdes v.3.15.3 (“careful” and “cov-cutoff auto” options selected) (34) and SSPACE software (35). Plasmid detection and assembly attempts were made using PlasmidFinder 2.13 (36) and PlasmidSPAdes (37), respectively. Scaffolds of less than 200 bp were discarded. We assessed the general statistics of the assembled genome using QUAST v5.0.2 (38). The graphical map of the circular genome was generated using CGView Server (39).
Genome annotations were conducted with Prokka v.1.14.5 annotation pipeline (40) and Rapid Annotation using Subsytems Technology (RAST) server v.2.04 (41). Orthologous groups were analyzed using eggNOG mapper v25 (42). Blast2GO (43) and Kyoto Encyclopedia of Genes and Genomes (KEGG)6 (44) were used for the determination of Gene Ontology (GO) annotation and gene role in metabolism, respectively.
Phylogenetic inferences using 16S rRNA gene, average nucleotide identity, and DNA–DNA hybridization
We identified the C. indologenes 16S rRNA gene sequence from our genome annotation. We considered reference sequences of the 16S rRNA gene from other 14 Chryseobacterium species available at the GenBank database, including another C. indologenes strain. Elizabethkingia miricola was used as the outgroup. The accession numbers of these sequences are shown in the phylogeny. Nucleotide sequences were aligned using MAFFT v.7 (45). With the software MEGA-X (46), we estimated the best-fitting nucleotide model of substitution. This information was used to construct the phylogenetic tree by the Maximum Likelihood (ML) method. The robustness of branches was assessed by bootstrap analysis of 1,000 replicates (47).
The average nucleotide identity (ANI) values among our C. indologenes genome and other twelve Chryseobacterium genomes (Supplementary Table 1) were calculated using OrthoANI7 (48). From these same genomes, we calculated the in silico DNA--DNA hybridization (DDH)-analogous values using the GGDC v.3.0 tool8 (49). Heat maps of ANI and DDH were generated using CIMminer.9
Comparative pan-genome analysis of Chryseobacterium indologenes strains
We constructed a whole-genome sequence-based phylogenetic tree considering our C. indologenes genome and other 15 Chryseobacterium indologenes genomes (Supplementary Table 2) using the online pipeline REALPHY v.1.13 (50) with default settings. The four closest strains of our C. indologenes were then analyzed with OrthoVenn210 (51), a webserver used for genome-wide annotation and comparison of orthologous gene clusters. Bacterial Pangenome Analysis Pipeline (BPGA) (52) was used for the identification of the core, accessory, and unique genes, and their functional distribution in KEGG categories.
Identification of antimicrobial resistance and virulence-associated genes
For the identification of antibiotic resistance genes, we used the AMRFinderPlus tool from NCBI (53), ResFinder 4.1 tool11 from DTU (54), and the Comprehensive Antibiotic Resistance Database (CARD; (55);12 RGI tool, with a 70% identity cutoff. We also performed BLASTp analysis against the ARG-ANNOT V6 database (56) with a 1E-5 e-value, > 50% identity, and > 90% query coverage cut-off. The results obtained from the KEGG functional annotation were also considered.
Putative virulence genes were predicted through BLASTp analysis against the Virulence Factor Database (VFDB) (57) using a 1E-5 e-value, > 50% identity, and > 90% coverage cut-off.
Identifications of mutations associated with quinolone and colistin resistance
Genes responsible for colistin and quinolone resistance were identified through the rapid prokaryotic genome annotation (PROKKA). The amino acid sequences obtained from lpxA, lpxC, lpxD, and pmrC were manually analyzed for known mutations conferring resistance to colistin. Acinetobacter multispecies (WP_196075311.1) and Acinetobacter baumanii (SUU42982.1) lpxA sequences were manually aligned with our C. indologenes sequence (58). Other genes associated with polymyxin resistance, such as pmrB, pmrA, phoP, and phoQ were not found in the annotation executed by PROKKA.
The presence of a known mutation responsible for quinolone resistance on the gyrA, gyrB, and parC genes was investigated using BLASTp software of NCBI.13 The deduced amino acid sequences of the C. indologenes gyrA were aligned with Chryseobacterium multispecies (WP_027372477.1) and Chryseobacterium indologenes (QPQ51520.1). Amino acid sequence alignment for the gyrB. of C. indologenes was made with Chryseobacterium aurantiacum (WP_106916365.1), Chryseobacterium joostei (WP_076354057.1), Chryseobacterium arachidis (WP_072953039.1), and Chryseobacterium taiwanense (WP_039365989.1). Substitutions of amino acids in parC sequence described in the literature were not found in our C. indologenes.
Genomic islands, insertion sequences, and other mobile genetic elements detection
IslandViewer 4 webserver14 (59) was used for the identification of the genomic island using FDAARGOS_379 as a reference strain. Insertion sequences, transposons, and integrons were predicted using ISFinder15 (60), TnCentral16 (61), and Integron Finder17 (62) webservers, respectively. CRISPR sequences were searched with CRISPRCas Finder18 (63) on default parameters. The PHASTER webserver19 (64) was used for the detection of phage-associated sequences in the genome. Integrative and conjugative elements (ICEs) and Integrative mobile elements (IMEs) were predicted with the ICEfinder tool20 (65) from the ICEberg 2.0 database using the default settings.
Sequences accession number
The raw reads were submitted to Sequence Reads Archives,21 and the Bioproject accession id is PRJNA830910. Moreover, all the sequence data that we analyzed are related to this Bioproject id.
Results
Characteristics of the patient and antibiotic resistance profile of strain
Our C. indologenes strain presented resistance to all tested antibiotics, including β-lactams (Ampicillin, Ampicillin-Sulbactam, Piperacillin-Tazobactam, Cefuroximeaxetil, Cefoxitin, Ceftazidime, Ceftriaxone, Cefepime, Ertapenem, Imipenem, Meropenem); aminoglycosides (Amikacin, Gentamicin); quinolones (Ciprofloxacin); glycylcycline (Tigecycline); and polymyxin (Colistin) (Table 1). The isolate was defined as multidrug-resistant (MDR). However, the following antibiotics were not included in the MDR classification: amikacin and gentamicin (aminoglycosides); ampicillin and ampicillin-sulbactam (aminopenicillins); imipenem, meropenem, and ertapenen (carbapenems). This was because C. indologenes is intrinsically resistant to these antibiotics (18, 19, 66, 67).
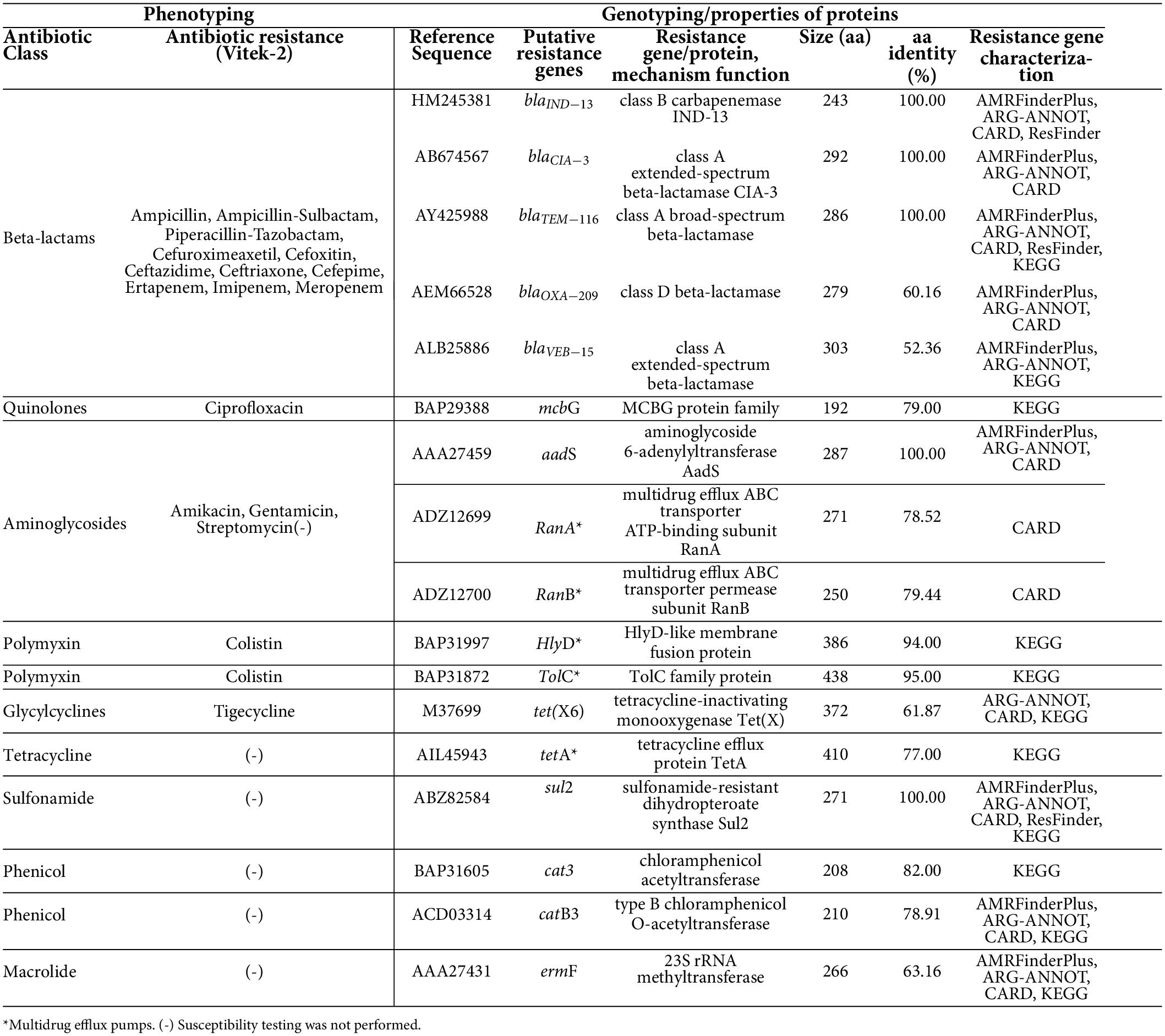
Table 1. Phenotyping and antibiotic resistance genes found within the Chryseobacterium indologenes genome.
General features of the Chryseobacterium indologenes genome
The total length of the draft genome assembled was 4,836,765 bp, comprising one circular chromosome, with 37.32% G + C content. We detected a COIRNAI plasmid replicon but could not assemble any plasmid. The statistics of assembly and annotation are shown in Figure 1A. The assembly comprised 58 scaffolds and 4,409 genes that covered 88.20% of the genome. Of these genes, 4,341 were predicted to be coding sequences (CDSs). Of the 68 RNA genes predicted, three were rRNAs, 64 transfer RNAs (tRNAs), and one transfer-messenger RNA (tmRNA) (Figures 1A,B). The RAST analysis showed that the genome of C. indologenes comprises 366 subsystems that could be classified into 27 categories. The five most significant categories in this genome were “amino acids and derivatives” that accounted for 384 genes, followed by “carbohydrates” (232 genes), “cofactors, vitamins, prosthetic groups, pigments” (224 genes), “protein metabolism” (222 genes), and “virulence, disease, and defense” (120). In the category of “virulence, disease, and defense,” 94 genes were found to be related to “resistance to antibiotics and toxic compounds,” including beta-lactamase (13 genes), multidrug resistance efflux pumps (13 genes), multidrug resistance, tripartite systems found in gram-negative bacteria (12 genes), resistance to fluoroquinolones (5 genes), and aminoglycoside adenylyltransferases (1 gene) (Figure 1C).
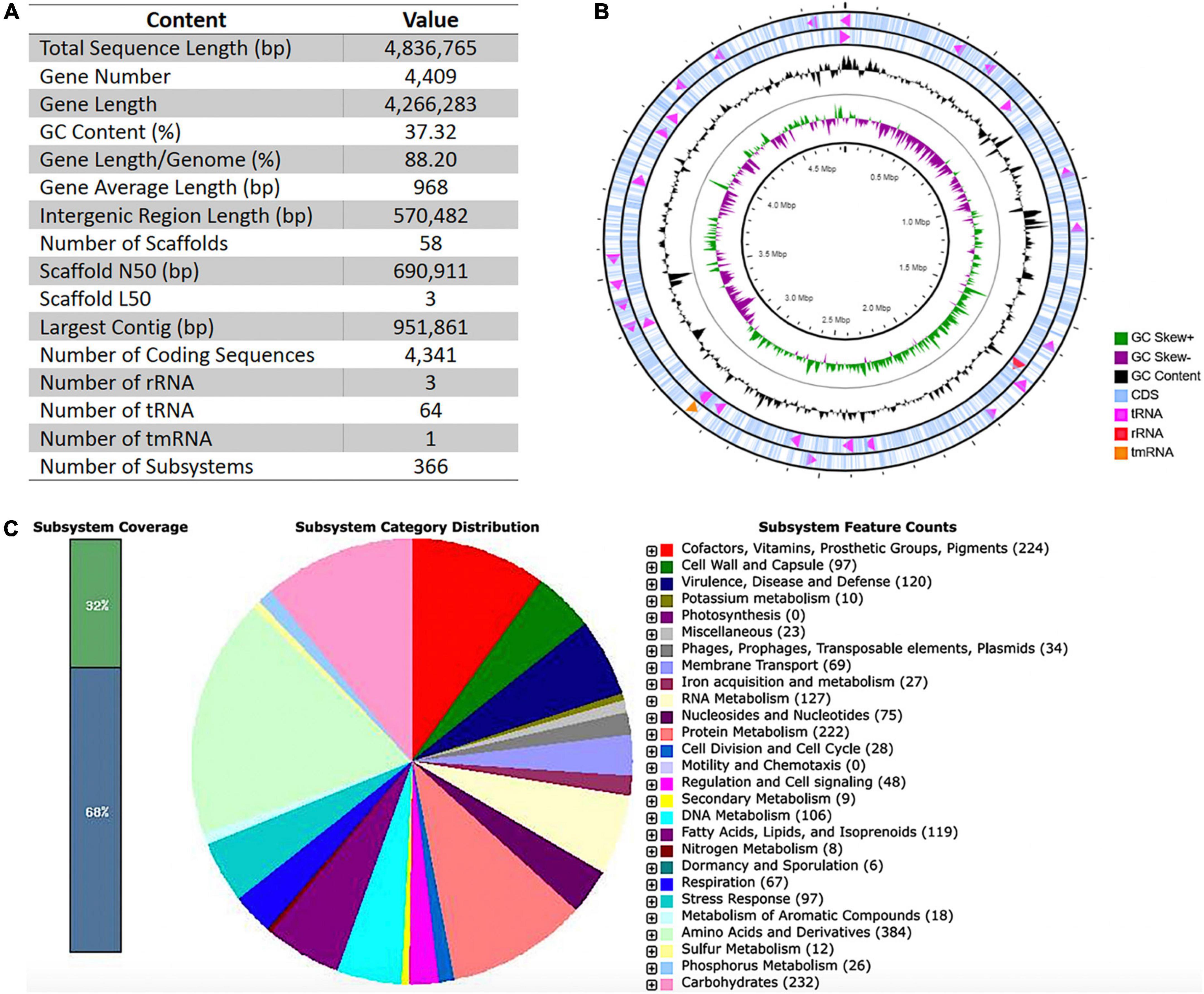
Figure 1. Basic data of Whole-Genome Sequencing, circular representations, and subsystem category distributions of Chryseobacterium indologenes. (A) Assembly and annotation statistics. (B) Circles are numbered from 1 (outer) to 4 (inner). The outer two circles represent the coding sequence (CDS), transfer ribonucleic acid (tRNA), ribosomal ribonucleic acid (rRNA), and transfer-messenger RNA (tmRNA). The third circle shows the GC content (black). The fourth circle demonstrates the GC skew curve (positive GC skew, green; negative GC skew, violet). (C) The genome of C. indologenes annotated by the Rapid Annotation System Technology (RAST) server was classified into subsystems and categories. The green part in the bar chart at the leftmost position corresponds to the percentage of proteins included. The pie chart and count of the subsystem features in the right panel show the percentage distribution and category of the subsystems.
Orthologous genes and gene ontology of Chryseobacterium indologenes
The distribution of protein-coding genes into the Cluster of Orthologous Groups (COG) functional category using EggNog resulted in a total of 3,759 genes. The majority of known protein-coding genes were related to “amino acid metabolism and transport” (n = 310; 8.25%), followed by those associated with “cell wall/membrane/envelope biogenesis” (n = 304; 8.09%), and “transcription” (n = 295; 7.85%). The number of genes associated with defense mechanisms was 70 (1.86%), and with “unknown functions” it was 973 (25.88%) (Figure 2A).
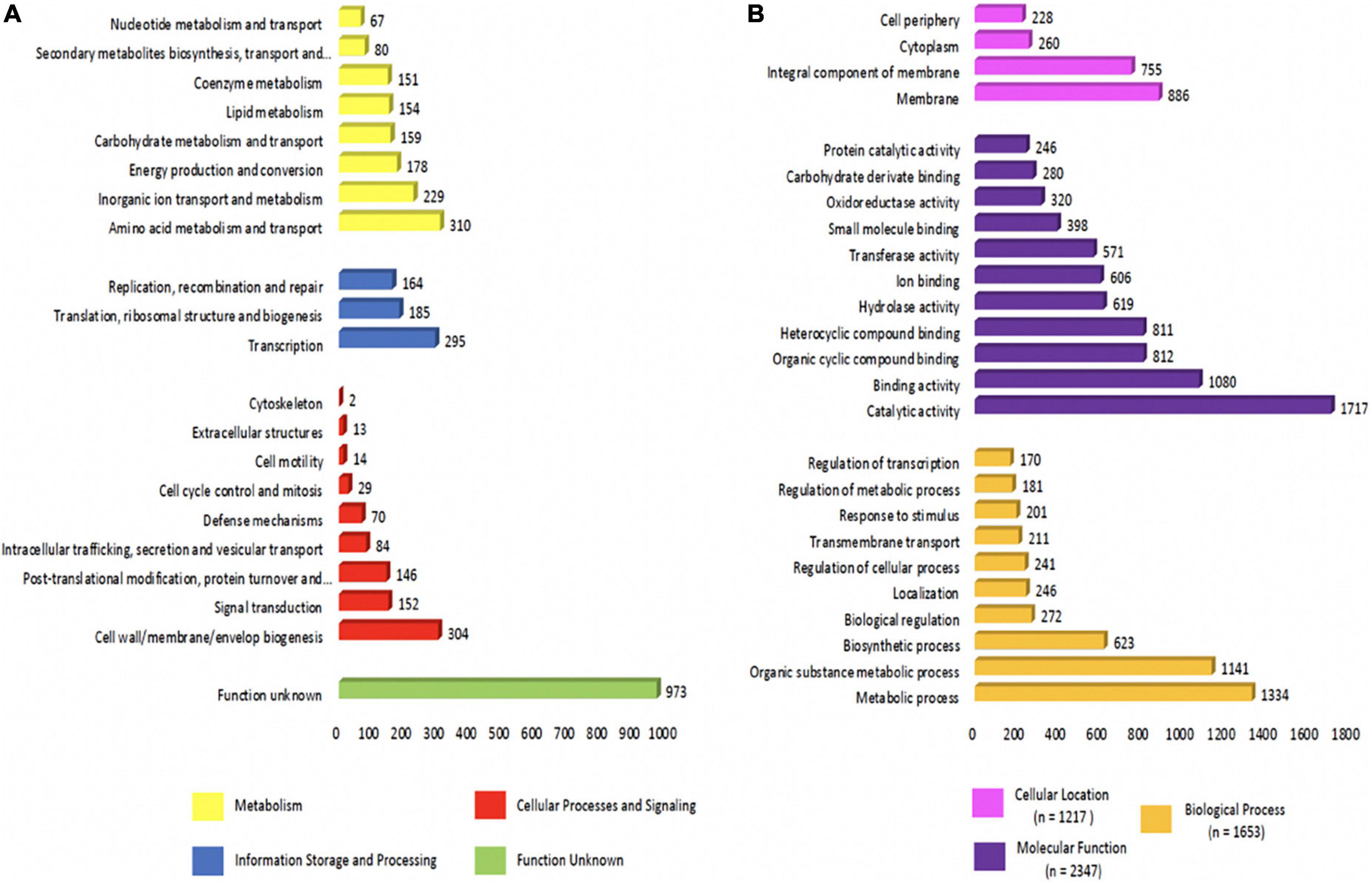
Figure 2. Functional annotations of Chryseobacterium indologenes strain. (A) Clusters of orthologous groups (COGs) based on functional annotation using eggNOG-mapper. (B) Distribution of gene ontology (GO).
The Gene Ontology (GO) distribution of our C. indologenes strain showed a total of 2,900 genes, which accounted for 65.77% of the entire encoded genes. Of these, 7,460 GO terms were associated with “molecular function,” 4,620 GO terms with “biological process,” and 2,129 GO terms with “cellular location.” The GO distribution showed that within the molecular function, the main subcategories were “catalytic activity (1.717 GO terms, 23.02%), “binding activity” (1.080 GO terms, 14.48%), and “organic cyclic compound binding” (812 GO terms, 10.88%). Among the biological process, most of the genes were characterized to the subcategories like “metabolic process” (1.334 GO terms, 28.87%), “organic substance metabolic process” (1.141 GO terms, 24.70%), and “biosynthetic process” (623 GO terms, 13.48%); Within the cellular location, the most highly assigned GO terms were “membrane” (n = 886, 41.62%), “integral component of membrane” (n = 755, 35.46%), and “cytoplasm” (n = 260, 12.21%) (Figure 2B).
Phylogenetic position and similarity of whole genomes
A phylogenetic tree based on the 16S rRNA sequence was constructed with fourteen 16S rRNA reference sequences related to Chryseobacterium and one 16S rRNA reference sequence of Elizabethkingia miricola as an outgroup. This aimed to define the evolutionary position of our C. indologenes strain. Analyses revealed that our C. indologenes was most closely related to Chryseobacterium indologenes (AM232813). Nevertheless, it also showed a similarity between different species, including Chryseobacterium cucumeris (KX146463), Chryseobacterium arthrosphaerae (FN398101), Chryseobacterium gleum (AM232812), and Chryseobacterium flavum (EF154516) (Figure 3A).
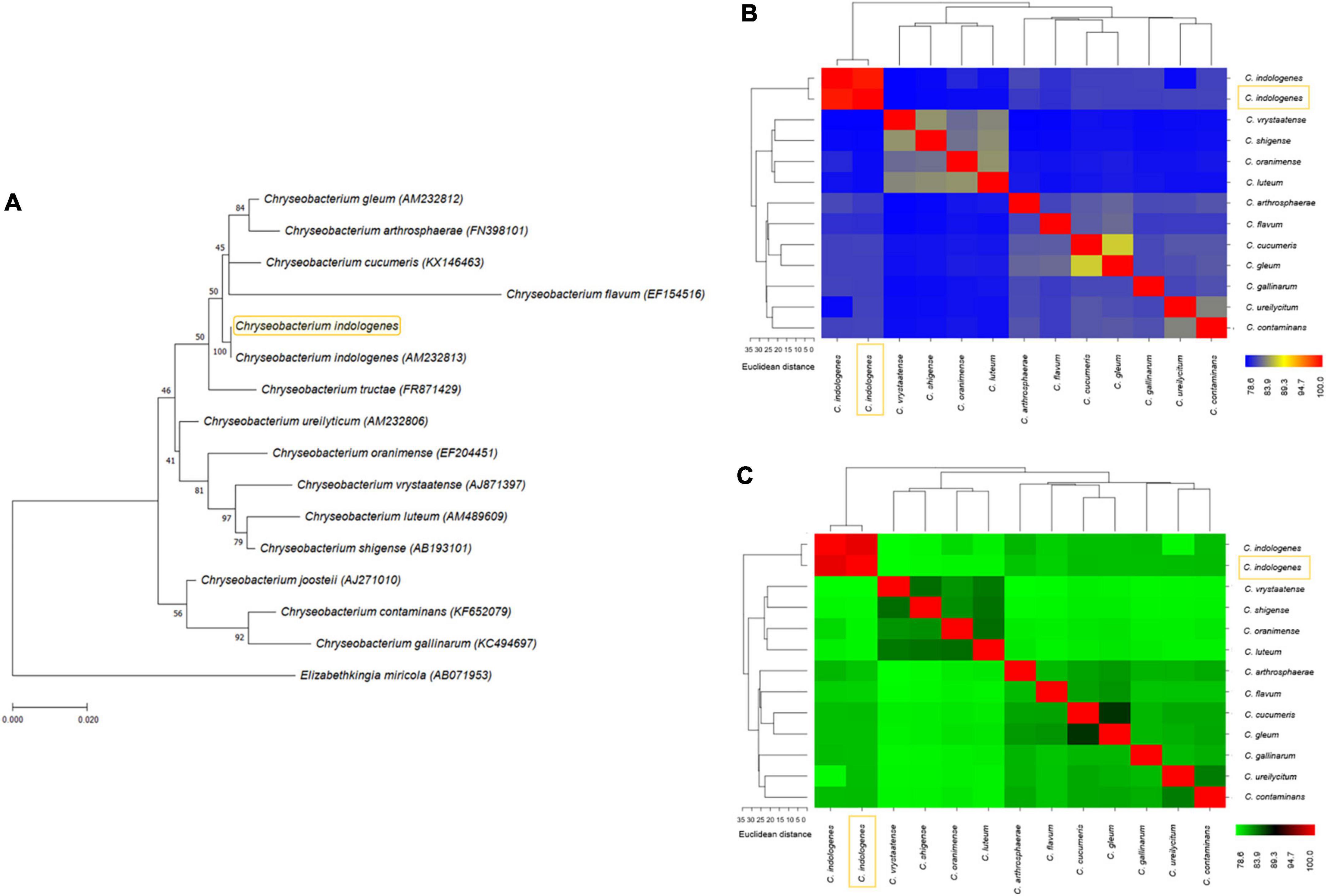
Figure 3. (A) Phylogenetic tree based on 16S rRNA showing the relationship among our C. indologenes strain and other reference sequences of 16S rRNA obtained from public databases. The number next to the node is the statistical bootstrap value. In brackets are GenBank accession numbers of the 16S rRNA genes. The scale bar indicates 0.020 substitutions per nucleotide position. The heat maps of average nucleotide identity (ANI) (B) and in silico DNA–DNA hybridization (DDH) (C) between our C. indologenes genome and twelve Chryseobacterium genomes. The yellow box represents our strain.
The genomic similarity between our sample and the twelve Chryseobacterium species that had complete genome sequences in the NCBI (Figure 3A) was evaluated using in silico ANI (Supplementary Table 3) and DDH (Supplementary Table 4) analyses (Figures 3B,C) to confirm the species relatedness inferred from the phylogenetic tree and ensure an accurate assignment at the species level. The ANI analysis found that our C. indologenes and C. indologenes (GCF_900460995.1) had a high similarity of 99.06% (Figure 3B). The DDH value between C. indologenes and C. indologenes (GCF_900460995.1) was 91.7% (Figure 3C). Therefore, both ANI and DDH analysis indicated that our C. indologenes strain belongs to the C. indologenes species.
PhyloGenetic tree and genetic relatedness
We performed a phylogenetic analysis based on C. indologenes genomes downloaded from the NCBI database. The resulting tree topology was assessed to identify genetic relatedness between our C. indologenes isolate and 15 C. indologenes strains (Figure 4A). Our analysis showed that our C. indologenes strains is more closely related to Chryseobacterium indologenes 742 (SAMN22445227), Chryseobacterium indologenes FDAARGOS_379 (SAMN07312423), Chryseobacterium indologenes FDAARGOS_537 (SAMN10163232), and Chryseobacterium indologenes FDAARGOS_648 (SAMN11056363) (Figure 4A). The characteristics of the 16 C. indologenes strains are shown in Figure 4B.
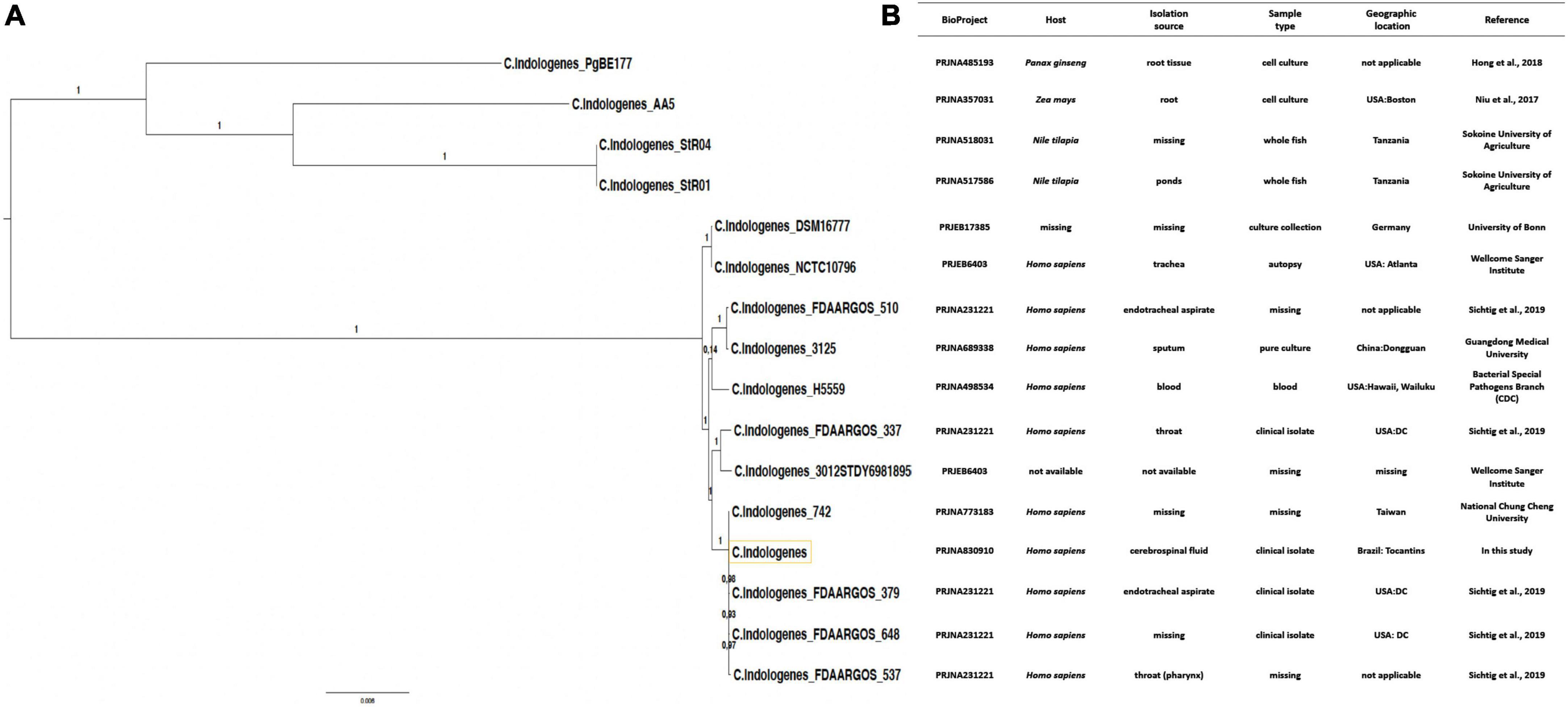
Figure 4. (A) Phylogenetic tree showing the evolutionary similarity between C. indologenes and other 15 selected strains of Chryseobacterium indologenes. (B) Genome Assembly and Annotation report (https://www.ncbi.nlm.nih.gov/genome/browse/#!/prokaryotes/14653/) of 16 strains of C. indologenes. The yellow box represents our strain.
Orthologous groups and Kyoto Encyclopedia of Genes and Genomes distribution in the closest Chryseobacterium indologenes genomes
Using OrthoVenn web server, comparison and annotation of orthologous gene clusters were performed between our C. indologenes and its four most closely related neighbors. Data indicates that there are 4,060 core-conserved genes shared by all five strains and a total of 6 strain-specific gene clusters in our C. indologenes strain. These unique genes were related to metal ion transport, response to cadmium ion, and copper ion transport (Figure 5A). The meanings of these unique gene clusters are not clear, and further studies are needed to better understand the functions of these singular genes. Our Chryseobacterium indologenes strain contained the highest number of singletons (n = 182), followed by C. indologenes 742 (n = 109), C. indologenes FDAARGOS_379 (n = 29), C. indologenes FDAARGOS_537 (n = 18), and C. indologenes FDAARGOS_648 (n = 11) (Figure 5B). We also found sul2, blaTEM–116, aadS, blaVEB–15, blaOXA–209, catB e ermF resistance genes in singletons of our C. indologenes strain.
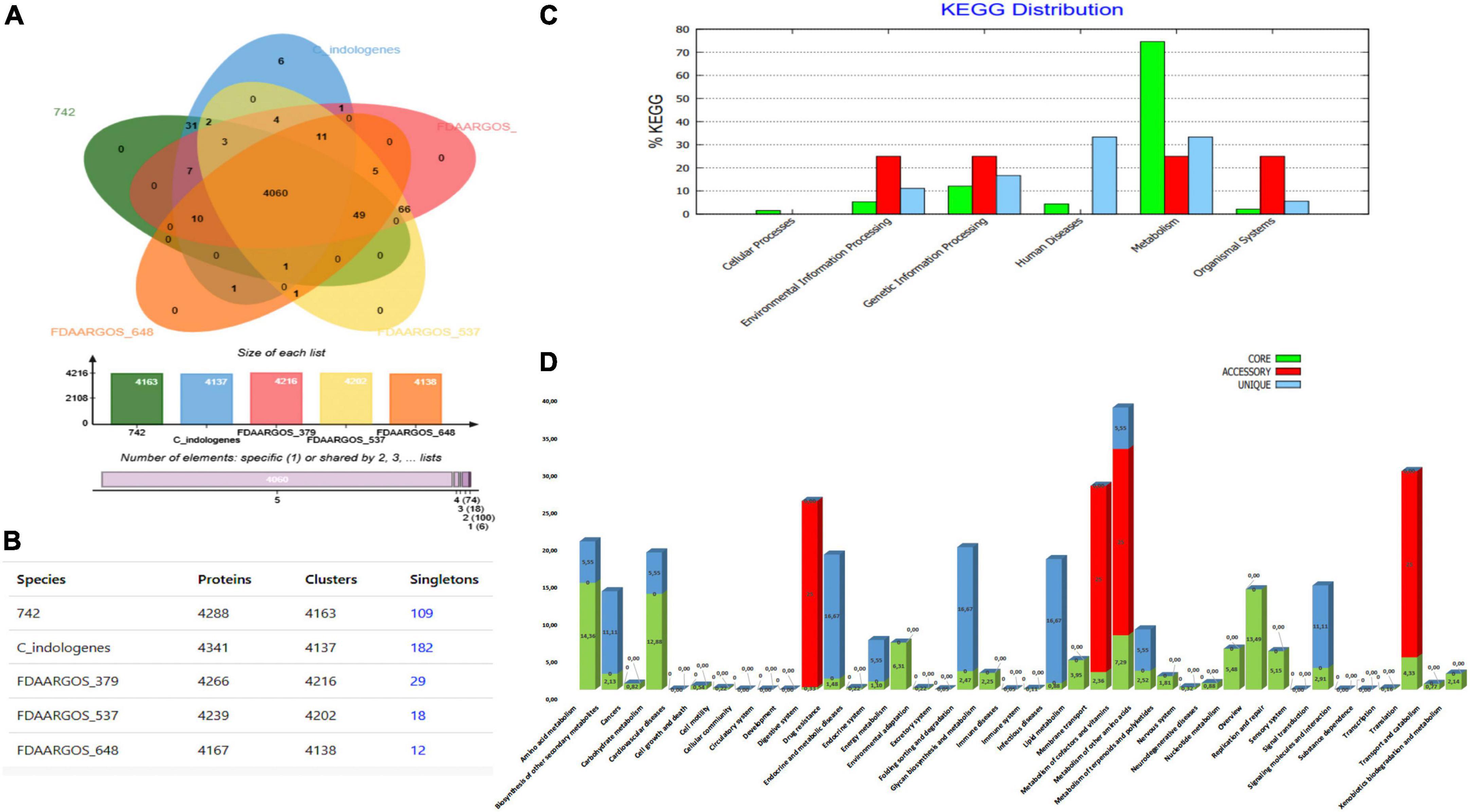
Figure 5. Comparative genomic analysis. (A) Venn diagram and bar chart showing the numbers of unique and shared orthologous genes in the five most closely related C. indologenes. (B) Number of proteins, clusters, and singletons. (C) KEGG pathway classification in core, accessory, and unique genomes. (D) Distribution of KEGG pathway classification.
The KEGG functional distribution showed that genes were associated mainly with “metabolism” and were the most abundant in core (74.62%) compared to unique (33.33%) and accessory (25%) genomes (Figure 5C). In the core genome, genes were mainly related to “amino acid metabolism” (14.36%), “overview” (13.49%), and “carbohydrate metabolism” (12.88%) (Figure 5D). In the accessory genome, “environmental information processing,” “genetic information processing,” “metabolism,” and “organismal systems” accounted for similar portions (25%) (Figure 5C). These genes were associated with “digestive system” (25%), “membrane transport” (25%), “metabolism of cofactors and vitamins” (25%), and “translation” (25%) (Figure 5D). In the unique genome, “metabolism” (33.33%), “human diseases” (33.33%), and “genetic information processing” (16.67%) accounted for most genes (Figure 5C). These genes were mainly associated with “folding, sorting, and degradation” (16.67%), “infectious diseases” (16.67%), and “drug-resistance” (16.67%) (Figure 5D). We found 27 genes related to “drug resistances” in the unique genome. Of these, 11 were related to beta-lactam resistance, 11 were associated with cationic antimicrobial peptide resistance, and 5 were vancomycin resistance genes. Our data suggest that antibiotic resistance plays an important function in all of the C. indologenes strains analyzed.
Resistome of Chryseobacterium indologenes
The whole-genome sequence analysis of C. indologenes corroborated with the phenotypic analyses, which revealed several antibiotic resistance-related genes (Table 1 and Figure 6). These antibiotic resistance genes included 5 β-lactamases (blaIND–13, blaCIA–3, blaTEM–116, blaOXA–209, blaVEB–15), 1 quinolone gene (mcbG), 1 tigecycline gene [tetracycline-inactivating monooxygenase tet(X6)], 1 RanA and 1 RanB genes that encode an efflux pump which confers resistance to aminoglycosides, 1 outer-membrane protein (TolC) and 1 gene of membrane fusion protein (HlyD) genes that mediate resistance to colistin antibiotic (Table 1). Additionally, our in silico analysis found genes that can confer resistance to tetracycline [tet(A)] (68), streptomycin (aadS) (69), phenicol (cat3, catB3) (70), sulfonamide (sul2) (71), and macrolides (ermF) (72); however, our strains were not tested phenotypically to these antibiotics (Table 1).
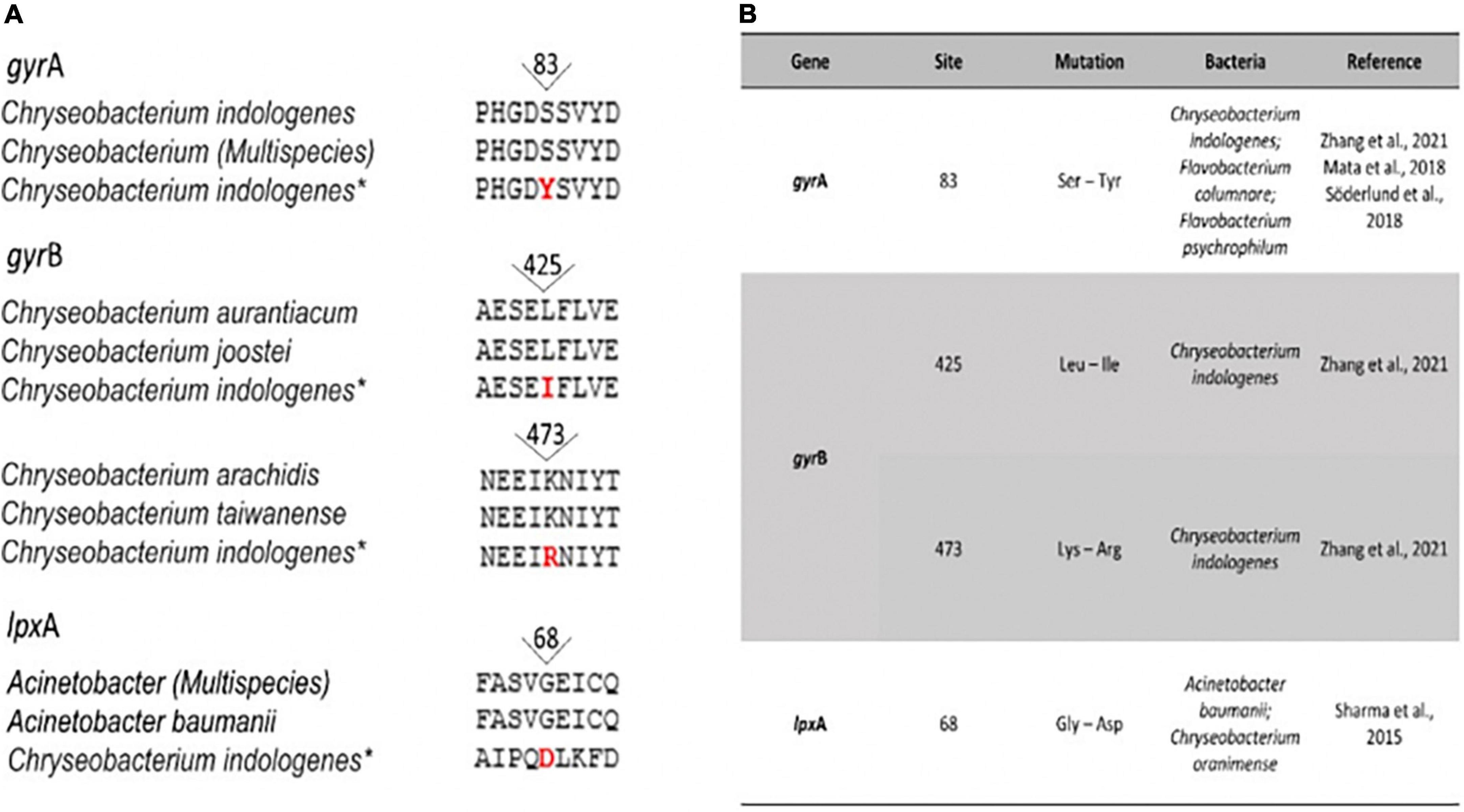
Figure 6. (A) Amino acid sequence alignment for the GyrA QRDR (Ser83Tyr) of Chryseobacterium ureilyticum, Chryseobacterium (Multispecies), Chryseobacterium indologenes*; GyrB QRDR (Leu425Ile) of Chryseobacterium aurantiacum, Chryseobacterium joostei, Chryseobacterium indologenes* and GyrB QRDR (Lys473Arg) of Chryseobacterium arachidis, Chryseobacterium taiwanense, Chryseobacterium indologenes*; and mutations in lpxA (Gly68Asp) of Chryseobacterium (Multispecies), Acinetobacter baumanii, Chryseobacterium indologenes*. (B) Known mutations in genes conferring resistance to quinolones (GyrA and GyrB QDDR) and colistin (lpxA). *Strain isolated in this study.
Gene functions annotated using the RAST/PROKKA tool identified gyrA, gyrB, and lpxA gene, in which amino acid substitution can also confer antibiotic resistance for fluoroquinolone and colistin, respectively. Amino acid substitutions related to quinolone resistance were also found at positions 83 of DNA gyrase subunit A (gyrA: Ser83Tyr) and at positions 425 and 473 of DNA gyrase subunit B (gyrB: Leu425Ile and Lys473Arg). Mutations that may play a role in the development of colistin resistance were found at lpxA (G68D) of C. indologenes (Figures 6A,B).
Virulence-associated genes detection
Chryseobacterium indologene strain revealed the presence of 19 virulence factorsthat were associated with adhesion (elongation factor Tu, ilpA), stress response (katA, katG, clpP, and groL), environmental adaptation (ureG, ureB), biofilm formation (clpP, galE), metabolism (clpE), motility (rffG), polysaccharide biosynthesis (tviB), O-antigen nucleotide sugar biosynthesis (wlbB), O-antigen-synthesis (rfbA), capsular polysaccharide synthesis and antiphagocytosis (cap8E, cap8G), survival and virulence (mgtB), cytokine production and cytotoxicity (ilpA), infection and immune evasion (icl1), and fatty acid biosynthesis (acpP) (Table 2).
Genomic islands and other mobile genetic elements genes in Chryseobacterium indologenes
Our analyses indicate that the C. indologenes genome contained 13 potential Genomic Islands (GI) (Figure 7A). These had some elements associated with one integrative and conjugative element (ICE), in the 8 and 12 GI regions, such as a copy of a TrbC, and a relaxase (xerC). The ICE, named ICECind1, contained further open reading frames (ORFs) encoding putative TrbL, TrbC (1 copy), TrwB, integrase, and it was bordered by a 16-bp direct repeat (attL; 5′-TTGTGGGTCCTGAGG-3′, and attR: 5′-TTGTGGGTCCTGAGGG-3′) at both ends. The attR was included in the 3’ end of the tRNA-Val(TAC). In GI, we also found a sul2 gene encoding for resistance to sulfonamide, flanked by IS91-like element (ISVsa3 family transposase); an IS91 family transposase ISTha3; 2 copies of xerC; and other “accessory” sequences (Figures 7B,C).
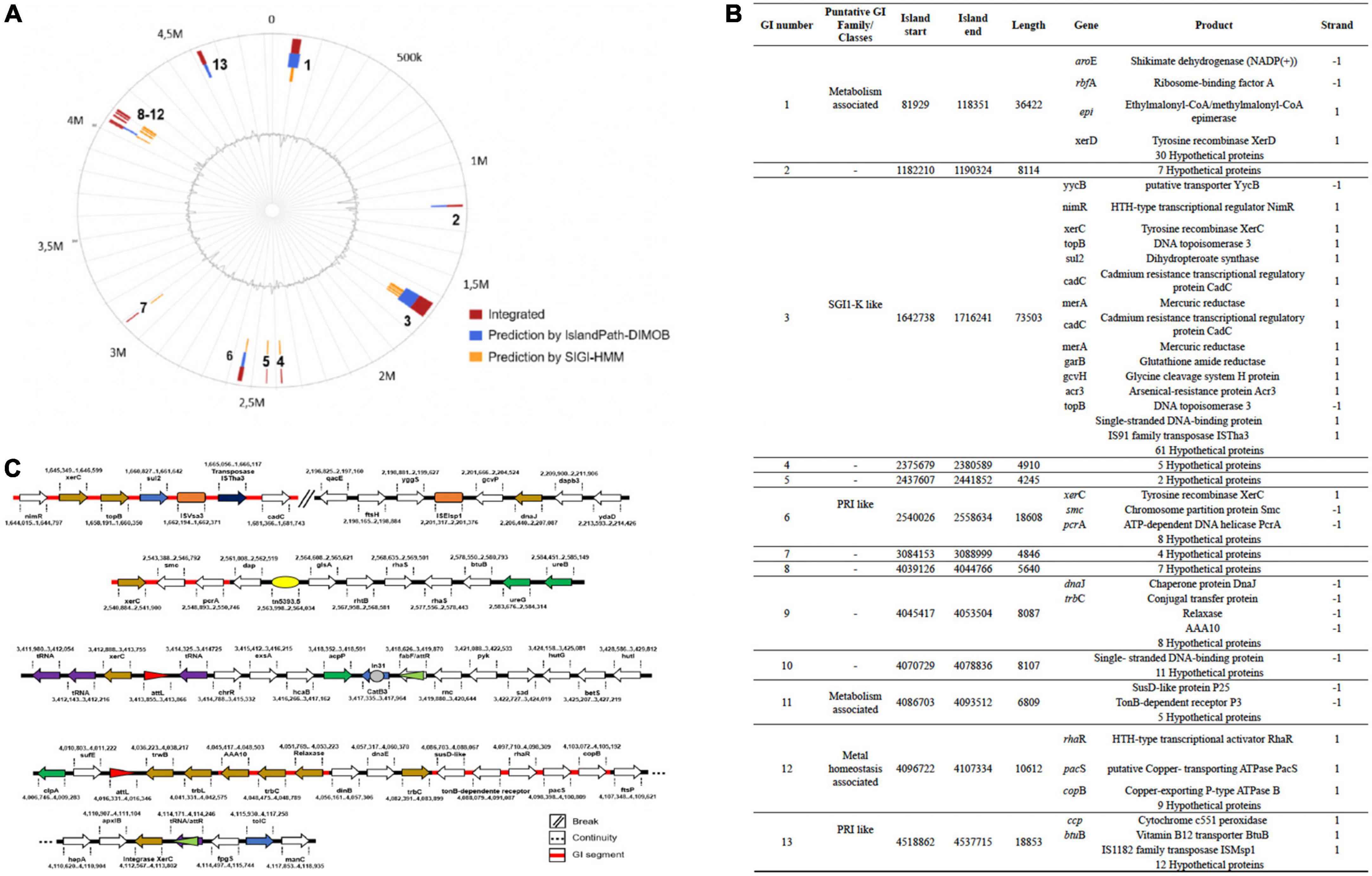
Figure 7. Schematic representation of the Genomic Islands (GIs) and Mobile Genetic Elements (MGEs) in C. indologenes strain. (A) Circular genomic representation of GIs. (B) Genetic composition of the GIs. (C) MGEs found in C. indologenes. Arrow: Light blue (resistance genes), dark blue (transposase genes), golden [elements associated with Integrative and Conjugative Element (ICE)], green (virulence genes), purple (tRNAs). Red/green triangles represent the attL and attR sequences, respectively. Orange squares represent Insertion Sequences. The yellow ellipse represents transposons. The gray circle represents integron.
Other elements linked to mobile genetic elements (MGEs) were present such as an insertion sequence (ISEIsp1), classified in the IS1595 family (ISPna2 group); and a transposon Tn5393. Sequence examination further indicated a region bordered by a 12-bp direct repeat (aatL 5′-ATTTTCTTAAAT-3 and attR 5′-ATTTTCTTAAAT-3) at both ends that contained the catB3, a chloramphenicol acetyltransferase gene inserted in integron In31; one gene associated with fatty acid biosynthesis (acpP); a 3-phenylpropionate-dihydrodiol/cinnamic acid-dihydrodiol dehydrogenase (hcaB) gene, a transcriptional regulator (exsA) gene; a quinone reductase (chrR) gene; and a tRNA (Figure 7B).
Through IslandViewer 4, we detected unaligned scaffolds that contained a ColRNAI type of replicons and genes associated with β-lactams (blaTEM–116, blaVEB–15, blaOXA–209), aminoglycosides (aadS), phenicol (catB3), and macrolide (ermF) antibiotic resistance. CRISPR and phage elements were not detected in the C. indologenes genome.
Discussion
Multidrug-resistant Gram-negative bacteria are usually associated with nosocomial infection and pose an important health problem for neonates admitted to neonatal intensive care units worldwide (9, 73). Chryseobacterium indologenes is an emerging nosocomial pathogen that has acquired clinical significance due to ubiquitous and intrinsical resistance to several antibiotics, life-threatening infection potential, and ability to persist in hospital settings (9, 74). The number of studies about C. indologenes is very limited, mainly in terms of their genomes. To build on current information, we performed a systematic genotypic characterization of a C. indologenes strain isolated from the cerebrospinal fluid of an infant.
Although there has been an increasing trend of C. indologenes bacteremia related to infants over the last few decades (5, 75, 76), most cases of infections caused by C. indologenes are still reported in adults (8, 10). The precise reason for fewer reports of C. indologenes infections in children remains unclear. Studies have suggested it may relate to the lower frequency of comorbidities in the pediatric population (77). Considering the pediatric population can present significant morbidity and immune dysfunction, we postulate that the relevance of C. indologenes isolated from clinical samples of infants can be challenging since novel molecular and phenotypic tests are providing rapid and accurate identification of many bacterial species, including glucose non-fermenting gram-negative bacilli (78, 79) such as C. indologenes.
Chryseobacterium indologenes often exhibit resistance to a wide variety of broad-spectrum antibiotics, including aminoglycosides, tetracyclines, chloramphenicol, macrolides, clindamycin, and teicoplanin; extended-spectrum penicillins; and first-, second- and third-generation cephalosporin, aztreonam, ticarcillin-clavulanate and the carbapenems (19, 23, 80). According to our phenotypic results, our C. indologenes strain was resistant to most of these antibiotics. It revealed a multidrug resistance profile, presenting in vitro resistance to a wide range of antibiotic classes including β-lactams, aminoglycosides, quinolone, glycylcycline, and polymyxin. Although some studies have reported that C. indologenes remains susceptible to trimethoprim/sulfamethoxazole, quinolones, minocycline, cefepime, ceftazidime, piperacillin-tazobactam, and rifampin (10, 18, 81, 82), our strain was resistant not only to piperacillin–tazobactam, ciprofloxacin, cefepime, and ceftazidime but also to colistin and tigecycline. Our findings are in accordance with other studies (3, 9, 24) that show an increasing trend in resistance of C. indologenes against most commonly used antimicrobial agents.
In recent years, whole-genome sequencing (WGS) has become an efficient method not only for understanding the evolution of a wide range of infectious pathogens, such as emerging bacteria, but also for outbreak surveillance and implementation of rapid infection control protocols (83). Thus, we decided to investigate the C. indologenes genome using WGS. Our draft genome revealed one circular chromosome that had a similar length (4,836 kb) to most of the sequenced genomes from C. indologenes deposited in NCBI. The genomic features of chromosomes annotated using RAST, eggNOG, and GO showed similar characteristics, presenting cellular processes that are essential to the bacteria (84). The genes related to the disease found in RAST and the defense mechanisms present in eggNOG analysis indicate our C. indologenes strain is associated with the multidrug resistance profile. Liang et al. (84) obtained similar results when they analyzed the genomic features and antimicrobial susceptibility patterns of the Chryseobacterium arthrosphaerae strain ED882-96 isolated from a patient in Taiwan.
To evaluate the taxonomic position and to confirm the species identification of our strain, we conducted a 16S rRNA analysis and found that our strain is affiliated with the species C. indologenes (GenBank: AM232813). Although 16S rRNA gene sequences are highly conserved among strains of the same bacterial species and are frequently used to identify and classify microorganisms, taxonomic classifications based only on the analysis of the 16S rRNA gene can lead to misclassifications in some cases (85). This happens because this analysis lacks sufficient discriminatory power to differentiate species in many genera, such as Aeromonas, Bacillus, Pseudomonas, Streptococcus, etc. (86, 87). Therefore, we used average nucleotide identity (ANI) and digital DNA–DNA hybridization (dDDH) to validate species identity, which confirmed that the most closely related species of our strain was C. indologenes (GCF_900460995.1).
The phylogenetic and corresponding taxonomic analysis is fundamental not only to establish the genetic novelty and the genotype–phenotype relationships of the isolates but also to identify the closest relatives of microorganisms within assembled genomes (88, 89). When we assessed the genetic relatedness between C. indologenes isolate and fifteen C. indologenes strains (90–92), we found that the five closest relatives of C. indologenes strains were isolated from clinical human samples. We then analyzed the distribution of shared gene families (sequence clusters) among the proteomes of our sample and four C. indologenes strains. Interestingly, our C. indologenes strain harbored the highest number of singleton genes compared to its closest four relatives. Some of the singleton genes were related to antibiotic resistance. The genomic variability and diversity among the isolates are highlighted by the analysis of singletons and non-core genes that may be acquired from distal lineages through horizontal gene transfer and represent the genetic source for the emergence of novel functions (89).
The biological functions of the KEGG pathway genes were analyzed to further characterize the genomic differences among five strains. KEGG pathway annotation revealed that most of the genes of the core genome are related to metabolism. Similar results were obtained in previous studies on Chryseobacterium genomes (93). Moreover, drug resistance genes that may contribute to the wide resistance of strain C. indologenes to antimicrobials were found in a unique genome. All these distinctive features of our C. indologenes isolate provide evidence of its genomic plasticity that may contribute to antibiotic resistance and environmental adaptation.
When investigating the genetic basis of multidrug resistance (MDR) profile in C. indologenes, we observed a high level of concordance between the phenotypic and the genotypic results. Our analysis showed that the resistance gene profile to β-lactams in C. indologenes isolate may be due to the carriage of blaCIA–3, blaIND–13, blaTEM–116, blaVEB, and blaOXA–209. The blaCIA and blaIND genes have previously been identified in C. indologenes (94), and they usually provide intrinsic resistance to carbapenems and cephalosporins due to their production of class A β-lactamase (CIA) and class B carbapenem-hydrolyzing β-lactamase (IND) (23, 95, 96). The blaTEM–116 gene was described for the first time in Korea in a clinical isolate of E. coli (97). In Brazil, it was previously found in Klebsiella pneumoniae (98), Vibrio parahaemolyticus (99), Aeromonas hydrophila, and Aeromonas jandaei (100). Studies have related that TEM-116 β-lactamase can confer resistance to ceftazidime, cefotaxime, and aztreonam (97, 101). The blaVEB–15 gene was first identified from genomic DNA of E. coli isolate that had reduced susceptibility to ceftazidime–avibactam (102). However, the blaVEB group has been identified in a variety of species of Enterobacteriaceae and non-fermenting species such as P. aeruginosa and Acinetobacter baumannii (102). The VEB enzymes confer a high level of resistance to an expanded-spectrum cephalosporin (103, 104). The blaOXA–209 is a β-lactamase gene that was first described in Riemerella anatipestifer strain isolated from ducks and geese (105). It was also described more recently in pan-drug-resistant Myroides odoratimimus PR63039 strain isolated from a patient presenting with post-injury urinary tract infection (106). Although there were a few reports on the substrate profile of beta-lactamase class D OXA-209, we suggest this enzyme can contribute to the resistance of C. indologenes to β-lactam antibiotics.
Our analysis revealed the presence of genes that can mediate resistance to tigecycline (tet(X)), quinolones (mcbG and mutation in the gyrA and gyrB genes), polymyxin (HlyD, TolC, and mutation in the lpxA gene), and aminoglycosides (RanA and RanB). Tet(X) is an enzyme capable of modifying the antibiotic tetracycline and its derivates, including tigecycline and glycylcycline (68, 107). Since the identification of the tet(X) gene from the obligately anaerobic Bacteroides spp. (108, 109), it has been reported among strains of Enterobacteriaceae, Comamonadaceae, Flavobacteriaceae, and Moraxellaceae (23, 110, 111). Resistance to quinolones can be conferred by mutations in quinolone-resistance-determining region (QRDRs) (gyrA, gyrB, parC, and parE subunits), efflux pumps (QepA and OqxAB), DNA topoisomerase protection protein Qnr, and quinolone acetyltransferase Aac (6′)-Ib-cr (23, 112, 113). Our strain displayed amino acid alternations at position 83 in GyrA (Ser83Tyr) (23, 114) and positions 425 and 473 in gyrB (Leu425 Ile and Lys473Arg) (23). These findings are in line with studies showing these mutations in C. indologenes (23). Furthermore, the mcbG gene, found in our C. indologenes strain, encodes a pentapeptide-repeat protein with 19.6% amino acid identity with QnrA (115). The mcbG protein protects DNA gyrase from the action of microcin B17 (MccB17) and some quinolones antibiotics (115, 116).
We also examined whether mutations in pmrC, lpxA, lpxC, and lpxD genes were associated with polymyxin resistance found in our strain. Our analysis revealed only a substitution in lpxA (Gly68Asp). Amino acid substitutions, frameshifts, or truncation of lpxD lpxA and lpxC have been demonstrated to lead to a complete loss of LPS (117). These changes may play a role in colistin resistance, as shown in clinical A. baumannii and Chryseobacterium oranimense isolates (117, 118).
Efflux systems have been described in several bacteria isolated from clinical specimens and can be related to multidrug resistance phenotypes (119). We identify genes associated with multidrug effux pumps, such as HlyD, TolC, RanA, and RanB. HlyD belongs to the membrane fusion protein family (120) and forms a continuous channel by docking to the TolC. HlyD has been shown to contribute to polymyxin resistance in A. baumannii (121, 122). TolC, an outer-membrane channel protein, is often the final portal in the pathways of protein toxin transport or export of unwanted molecules, such as antibiotics (123–125). RanARanB genes encode an efflux pump of the ABC efflux pump system. RanA alongside RanB mediates resistance to aminoglycosides in Riemerella anatipestifer (126), a member of the Flavobacteriaceae family.
Although some studies suggest that proteases and biofilm production may play an important role in the virulence of invasive infections caused by C. indologenes (8, 11), much remains unknown about virulence factors essential for pathogenicity and their mechanism during pathogenesis. Analyzing the virulence profiles of our strain, we found genes that encode conserved virulence factors, which have been previously identified in C. indologenes (127) and other pathogens (128). The virulence factors found were associated with oxidative stress such as catalase (katA, katG) (129, 130); adhesion to host cells and extracellular matrix components (elongation factor Tu) (131); colonization of a host organism and in maintenance of bacterial cells in tissues (ureB) (132); bacterial growth, stress tolerance, and biofilm formation (clpP) (133); modulating the expression of virulence determinants and metabolism-related factor (clpE) (134); cell envelope structure, swarmer cell elongation, and subsequent swarm motility (rffG) (135, 136); serum resistance and biofilm formation (galE) (137–139); O-antigen nucleotide sugar biosynthesis (wlbB) (140); O-antigen-synthesis (rfbA) (141); polysaccharide biosynthesis (tviB) (142); capsular polysaccharide synthesis and antiphagocytosis (cap8E, cap8G) (143, 144); survival and virulence (mgtB) (145, 146), stress response (groL) (147); induction of cytokine production, adhesion, and cytotoxicity (ilpA) (148, 149); and infection and immune evasion capacity (icl, acp) (150). Our data indicate that C. indologenes may be a highly virulent strain, presenting putative virulence factors related to structural functions, physiological activity, defense, or invasion that favor the course of pathogenesis.
Genomic Islands (GIs) are cluster genes in prokaryotic genomes of probable horizontal origin, which harbor components of mobile genetic elements (MGEs) that may be associated with mobilizing DNA (151, 152). GI regions often carry genes related to pathogenicity, symbiosis, metabolic, fitness, or resistance islands (153) that confer a selective advantage to the host bacterium (152). We searched the C. indologenes genome for the MGEs able to transfer genes between DNA molecules (insertion sequences, gene cassettes, integrons, and transposons) and for those able to transfer genes between cells (conjugative and mobilizable plasmids, and integrative and conjugative element (ICEs) (154). Our analysis showed that the most prevalent ORFs are hypothetical proteins, which are frequently found in GIs and ICEs (152). We also found a GI region with ICE features (ICECind1) that contained copies of a relaxase-encoding gene; genes related to a type IV secretion system such as three conjugative transfer protein-encoding genes (1 copy trbL and 2 copies of trbC) (155, 156); a gene related to essential integral membrane protein (TrwB), important for the conjugation process (157); and a gene encoding a site-specific integrase (xerC), which ensures the site-specific chromosomal integration of the ICE as well as effective excision of the element, where it may be aided by an excisionase or recombination directionality factor (158–160). In addition, we found a transposon Tn5393 that usually carries the strA and strB genes, responsible for the resistance to streptomycin (161, 162). However, these resistance genes were not present in this region. The sul2 gene, encoding for resistance to sulfonamide, was flanked by ISVsa3 family transposase (IS91-like element). This has been previously described in a plasmid (pEPMS-18199) from Edwardsiella piscicida (163). The cat variant genes usually participate in the composition of gene cassette or integron, and confer the ability of antibiotic resistance (164). Chryseobacterium indologenes contained a catB3, a chloramphenicol acetyltransferase gene, inserted in integron In31. Interestingly, Laraki et al. (165) showed that catB6, a chloramphenicol acetyltransferase−encoding allele of the catB family, may be inserted in this integron (In31). The authors also observed it decreased the in vitro antibiotic susceptibilities of Pseudomonas aeruginosa strains.
Although WGS allows the analysis of large datasets using in silico plasmid typing methods, short reads from popular high-throughput sequencers can be difficult to assemble. Therefore, complete plasmid sequences may not be accurately reconstructed (166). When antimicrobial resistance genes are localized on incomplete contigs, it is uncertain whether they are localized on plasmid or chromosome (167). In our C. indologenes strain, most resistance genes (blaTEM–116, blaVEB–15, blaOXA–209, aadS, catB3, ermF) detected in singletons and the replicon (ColRNAI_DQ298019) were located on unaligned scaffolds. These findings partially corroborate the results of Evans et al. (168), who found a ColRNAI plasmid harboring genes associated with β-lactams (blaOXA–9, blaTEM–1A), chloramphenicol (catA1) antibiotic resistance. Furthermore, studies have shown that catB3 is one member of the gene cassette aacA7-catB3-aadB-oxa2-orfD. It can be mobilized by the integron-encoded DNA integrase and plays a role in chloramphenicol resistance of plasmid pBWH301 (169, 170). However, this complete gene cassette was not found in our analysis.
The scarcity of data on the properties of clinical isolates of C. indologenes makes it challenging to characterize the transmission and evolution of this pathogen. Therefore, we believe that our detailed data of the WGS will contribute to the understanding of the genomic diversity, pathogenic potential, and multidrug resistance profile presented by C. indologenes. In addition, our data may guide future public health policy and MDR C. indologenes infection control.
Data availability statement
The datasets presented in this study can be found in online repositories. The names of the repository/repositories and accession number(s) can be found in the link: https://www.ncbi.nlm.nih.gov/bioproject/?term=PRJNA830910. Bioproject accession id is PRJNA830910.
Ethics statement
The studies involving human participants were reviewed and approved by the Committee of Ethics in Human Research of the Federal University of São Carlos (no. 1.595.268). In this work, C. indologenes and the anonymous archival data related patient age, gender, and sample type were obtained from the Central Laboratory of Public Health of Tocantins (LACEN/TO, data’s owner). Patient consent was not required since the data presented in this study do not relate to any specific person or persons. Written informed consent from the participants or their legal guardian/next of kin was not required to participate in this study in accordance with the national legislation and the institutional requirements. Permission to conduct the present study was obtained from the Health Department of the State of Tocantins (Secretaria da Saúde do Estado do Tocantins – SESAU) and LACEN/TO.
Author contributions
MSFD, RLF, EBC, GGS, LCC, PML, and ASC performed the experiments. CCMF, LTC, and AFC aided with the bioinformatics analysis. MCSP, MSFD, EBC, and AP-S wrote the manuscript and analyzed the data. MCSP conceived and supervised the study. All authors contributed to the article and approved the submitted version.
Funding
This work was supported by the Fundação de Amparo à Pesquisa do Estado de São Paulo-Brazil (FAPESP grants 2020/11964-4 to MCSP, and FAPESP grants 2018/20697-0 to AFC). This study was partially financed by the Fundação de Amparo à Pesquisa do Estado de São Paulo-Brazil (FAPESP) as a fellowship to MSFD (FAPESP fellowship 2018/24213-7), GGS (FAPESP fellowship 2021/08423-4), and PML (FAPESP fellowship 2021/00425-8).
Conflict of interest
The authors declare that the research was conducted in the absence of any commercial or financial relationships that could be construed as a potential conflict of interest.
Publisher’s note
All claims expressed in this article are solely those of the authors and do not necessarily represent those of their affiliated organizations, or those of the publisher, the editors and the reviewers. Any product that may be evaluated in this article, or claim that may be made by its manufacturer, is not guaranteed or endorsed by the publisher.
Supplementary material
The Supplementary Material for this article can be found online at: https://www.frontiersin.org/articles/10.3389/fmed.2022.931379/full#supplementary-material
Footnotes
- ^ https://www.bioinformatics.babraham.ac.uk/projects/fastqc/
- ^ https://www.bioinformatics.babraham.ac.uk/projects/trim_galore/
- ^ https://cge.cbs.dtu.dk/services/PlasmidFinder/
- ^ https://rast.nmpdr.org/
- ^ http://eggnog-mapper.embl.de/
- ^ https://www.genome.jp/kegg/
- ^ https://www.ezbiocloud.net/tools/orthoani
- ^ https://ggdc.dsmz.de/ggdc.php#
- ^ https://discover.nci.nih.gov/cimminer/
- ^ https://orthovenn2.bioinfotoolkits.net/
- ^ https://cge.cbs.dtu.dk/services/ResFinder/
- ^ https://card.mcmaster.ca/
- ^ http://blast.ncbi.nlm.nih.gov
- ^ https://www.pathogenomics.sfu.ca/islandviewer/upload/
- ^ https://www-is.biotoul.fr/
- ^ https://tncentral.ncc.unesp.br/
- ^ https://galaxy.pasteur.fr/
- ^ https://crisprcas.i2bc.paris-saclay.fr/CrisprCasFinder/Index
- ^ https://phaster.ca/
- ^ https://bioinfo-mml.sjtu.edu.cn/ICEfinder/ICEfinder.html
- ^ https://www.ncbi.nlm.nih.gov/sra
References
1. Vandamme P, Bernadet J-F, Segers P, Kersters K, Holmes B. New perspectives in the classification of the flavobacteria: description of Chryseobacterium gen. nov., Bergeyella gen. nov., and Empedobacter nom. rev. Int J Syst Bacteriol. (1994) 44:827–31. doi: 10.1099/00207713-44-4-827
2. Teke TA, Oz FN, Metin O, Bayhan GI, Gayretli Aydin ZG, Oguz M, et al. Chryseobacterium indologenes septicemia in an infant. Case Rep Infect Dis. (2014) 2014:270521. doi: 10.1155/2014/270521
3. Sud A, Chaudhary M, Baveja C, Pandey P. Rare case of meningitis due to an emerging pathogen Chryseobacterium indologenes. SAGE Open Med Case Rep. (2020) 8:2050313X20936098. doi: 10.1177/2050313x20936098
4. Bonten MJ, van Tiel FH, van der Geest S, Smeets HG, Stobberingh EE, Gaillard CA. Topical antimicrobial prophylaxis of nosocomial pneumonia in mechanically ventilated patients. Microbiological observations. Infection. (1993) 21:137–9. doi: 10.1007/BF01710529
5. Aykac K, Ozsurekci Y, Tuncer O, Sancak B, Cengiz AB, Kara A, et al. Six cases during 2012–2015 and literature review of Chryseobacterium indologenes infections in pediatric patients. Can J Microbiol. (2016) 62:812–9. doi: 10.1139/cjm-2015-0800
6. Mukerji R, Kakarala R, Smith SJ, Kusz HG. Chryseobacterium indologenes: an emerging infection in the USA. BMJ Case Rep. (2016) 2016:bcr2016214486. doi: 10.1136/bcr-2016-214486
7. Corbella M, Brandolini M, Cambieri P, Decembrino N, Pagani M, Bottazzi A, et al. A catheter-related bloodstream infection caused by Chryseobacterium indologenes successfully treated with antibiotic-lock rescue therapy. New Microbiol. (2017) 40:223–5.
8. Alon D, Karniel E, Zohar I, Stein GY. Chryseobacterium indologenes bacteremia: clinical and microbiological characteristics of an emerging infection. Int J Clin Med. (2018) 09:520–7. doi: 10.4236/ijcm.2018.96045
9. Mehta R, Pathak A. Emerging Chryseobacterium indologenes infection in indian neonatal intensive care units: a case report. Antibiotics. (2018) 7:109. doi: 10.3390/antibiotics7040109
10. Kirby JT, Sader HS, Walsh TR, Jones RN. Antimicrobial susceptibility and epidemiology of a worldwide collection of Chryseobacterium spp.: report from the SENTRY antimicrobial surveillance program (1997-2001). J Clin Microbiol. (2004) 42:445–8. doi: 10.1128/JCM.42.1.445-448.2004
11. Hsueh PR, Teng LJ, Ho SW, Hsieh WC, Luh KT. Clinical and microbiological characteristics of Flavobacterium indologenes infections associated with indwelling devices. J Clin Microbiol. (1996) 34:1908–13. doi: 10.1128/jcm.34.8.1908-1913.1996
12. Hsueh PR, Hsiue TR, Wu JJ, Teng LJ, Ho SW, Hsieh WC, et al. Flavobacterium indologenes bacteremia: clinical and microbiological characteristics. Clin Infect Dis. (1996) 23:550–5. doi: 10.1093/clinids/23.3.550
13. Hsueh PR, Teng LJ, Yang PC, Ho SW, Hsieh WC, Luh KT. Increasing incidence of nosocomial Chryseobacterium indologenes infections in Taiwan. Eur J Clin Microbiol Infect Dis. (1997) 16:568–74. doi: 10.1007/BF02447918
14. Celkan T, Ozkan A, Apak H, Diren S, Can G, Yuksel L, et al. Bacteremia in childhood cancer. J Trop Pediatr. (2002) 48:373–7. doi: 10.1093/tropej/48.6.373
15. Deng L, Li MF, Li YH, Yang JL, Zhou X. Chryseobacterium indologenes in four patients with leukemia. Transpl Infect Dis. (2015) 17:583–7. doi: 10.1111/tid.12400
16. Esposito S, Russo E, de Simone G, Gioia R, Noviello S, Vitolo M, et al. Transient bacteraemia due to Chryseobacterium indologenes in an immunocompetent patient: a case report and literature review. J Chemother. (2015) 27:324–9. doi: 10.1179/1973947814Y.0000000206
17. Calderón G, García E, Rojas P, García E, Rosso M, Losada A. Chryseobacterium indologenes infection in a newborn: a case report. J Med Case Rep. (2011) 5:10. doi: 10.1186/1752-1947-5-10
18. Izaguirre-Anariba DE, Sivapalan V. Chryseobacterium indologenes, an emerging bacteria: a case report and review of literature. Cureus. (2020) 12:e6720. doi: 10.7759/cureus.6720
19. Arif N, Khullar S, Kumar R, Choudhary SK, Kapil A, Dhawan B. Pleural effusion due to Chryseobacterium indologenes: case report and review of literature. J Lab Physicians. (2019) 11:284–6. doi: 10.4103/JLP.JLP_57_19
20. Palewar MS, Mudshingkar SS, Dohe V, Bharadwaj R. Infection by multidrug-resistant Chryseobacterium indologenes in cases of obstructive uropathy: case series with short review. Med J DY Patil Univ. (2017) 10:376–80. doi: 10.4103/MJDRDYPU.MJDRDYPU_201_16
21. Cunha V, Ferreira M, Fonseca AG, Diogo J. Community-acquired Chryseobacterium indologenes in an immuno-competent patient. JMM Case Rep. (2014) 1:e000588.
22. Fraser SL, Jorgensen JH. Reappraisal of the antimicrobial susceptibilities of chryseobacterium and flavobacterium species and methods for reliable susceptibility testing. Antimicrob Agents Chemother. (1997) 41:2738–41. doi: 10.1128/AAC.41.12.2738
23. Zhang Y, Li D, Yang Y, Su J, Xu X, Wang M, et al. Clinical and molecular characteristics of Chryseobacterium indologenes isolates at a teaching hospital in Shanghai, China. Ann Transl Med. (2021) 9:668–668. doi: 10.21037/atm-21-933
24. Vaneechoutte M, Dijkshoorn L, Nemec A, Kämpfer P, Wauters G. Acinetobacter, Chryseobacterium, Moraxella, and other nonfermentative gram-negative rods. In: R Patel editor. Manual of Clinical Microbiology. Washington, DC: ASM Press (2011). p. 714–31.
25. Gautam SS, Kc R, Leong KW, Mac Aogáin M, O’Toole RF. A step-by-step beginner’s protocol for whole genome sequencing of human bacterial pathogens. J Biol Methods. (2019) 6:e110. doi: 10.14440/jbm.2019.276
26. Revez J, Espinosa L, Albiger B, Leitmeyer KC, Struelens MJ. Survey on the use of whole-genome sequencing for infectious diseases surveillance: rapid expansion of European national capacities, 2015–2016. Front Public Health. (2017) 5:347. doi: 10.3389/fpubh.2017.00347
27. Clinical and Laboratory Standards Institute [CLSI]. Performance Standards for Antimicrobial Susceptibility Testing. 32nd ed. (2021). Available online at: https://clsi.org/standards/products/microbiology/documents/m100/ (Accessed April 27, 2022).
28. EUCAST. The European Committee on Antimicrobial Susceptibility Testing – (Valid From 2021-01-01). Version 11.0. (2022). Available online at: www.eucast.org (accessed April 21, 2022).
29. Miriagou V, Cornaglia G, Edelstein M, Galani I, Giske CG, Gniadkowski M, et al. Acquired carbapenemases in Gram-negative bacterial pathogens: detection and surveillance issues. Clin Microbiol Infect. (2010) 16:112–22. doi: 10.1111/j.1469-0691.2009.03116.x
30. Nordmann P, Naas T, Poirel L. Global spread of carbapenemase-producing enterobacteriaceae - volume 17, number 10—October 2011 - emerging infectious diseases journal - CDC. Emerg Infect Dis. (2011) 17:1791–8. doi: 10.3201/EID1710.110655
31. Ferreira RL, da Silva BCM, Rezende GS, Nakamura-Silva R, Pitondo-Silva A, Campanini EB, et al. High prevalence of multidrug-resistant Klebsiella pneumoniae harboring several virulence and β-lactamase encoding genes in a brazilian intensive care unit. Front Microbiol. (2019) 10:3198. doi: 10.3389/FMICB.2018.03198/BIBTEX
32. Ferreira RL, Rezende GS, Damas MSF, Oliveira-Silva M, Pitondo-Silva A, Brito MCA, et al. Characterization of KPC-producing Serratia marcescens in an intensive care unit of a Brazilian tertiary hospital. Front Microbiol. (2020) 11:956. doi: 10.3389/FMICB.2020.00956/BIBTEX
33. Magiorakos A-P, Srinivasan A, Carey RB, Carmeli Y, Falagas ME, Giske CG, et al. Multidrug-resistant, extensively drug-resistant and pandrug-resistant bacteria: an international expert proposal for interim standard definitions for acquired resistance. Clin Microbiol Infect. (2012) 18:268–81. doi: 10.1111/j.1469-0691.2011.03570.x
34. Bankevich A, Nurk S, Antipov D, Gurevich AA, Dvorkin M, Kulikov AS, et al. SPAdes: a new genome assembly algorithm and its applications to single-cell sequencing. J Comput Biol. (2012) 19:455–77. doi: 10.1089/cmb.2012.0021
35. Boetzer M, Henkel CV, Jansen HJ, Butler D, Pirovano W. Scaffolding pre-assembled contigs using SSPACE. Bioinformatics. (2011) 27:578–9. doi: 10.1093/bioinformatics/btq683
36. Carattoli A, Zankari E, García-Fernández A, Voldby Larsen M, Lund O, Villa L, et al. In silico detection and typing of plasmids using plasmidfinder and plasmid multilocus sequence typing. Antimicrob Agents Chemother. (2014) 58:3895–903. doi: 10.1128/AAC.02412-14
37. Antipov D, Hartwick N, Shen M, Raiko M, Lapidus A, Pevzner PA. plasmidSPAdes: assembling plasmids from whole genome sequencing data. Bioinformatics. (2016) 32:3380–7. doi: 10.1093/bioinformatics/btw493
38. Gurevich A, Saveliev V, Vyahhi N, Tesler G. QUAST: quality assessment tool for genome assemblies. Bioinformatics. (2013) 29:1072–5. doi: 10.1093/bioinformatics/btt086
39. Grant JR, Stothard P. The CGView server: a comparative genomics tool for circular genomes. Nucleic Acids Res. (2008) 36:W181–4. doi: 10.1093/nar/gkn179
40. Seemann T. Prokka: rapid prokaryotic genome annotation. Bioinformatics. (2014) 30:2068–9. doi: 10.1093/bioinformatics/btu153
41. Aziz RK, Bartels D, Best AA, DeJongh M, Disz T, Edwards RA, et al. The RAST Server: rapid annotations using subsystems technology. BMC Genomics. (2008) 9:75. doi: 10.1186/1471-2164-9-75
42. Cantalapiedra CP, Hernández-Plaza A, Letunic I, Bork P, Huerta-Cepas J. eggNOG-mapper v2: functional annotation, orthology assignments, and domain prediction at the metagenomic scale. Mol Biol Evol. (2021) 38:5825–9. doi: 10.1093/molbev/msab293
43. Conesa A, Gotz S, Garcia-Gomez JM, Terol J, Talon M, Robles M. Blast2GO: a universal tool for annotation, visualization and analysis in functional genomics research. Bioinformatics. (2005) 21:3674–6. doi: 10.1093/bioinformatics/bti610
44. Kanehisa M, Sato Y, Morishima K. BlastKOALA and GhostKOALA: KEGG tools for functional characterization of genome and metagenome sequences. J Mol Biol. (2016) 428:726–31. doi: 10.1016/j.jmb.2015.11.006
45. Katoh K, Standley DM. MAFFT multiple sequence alignment software version 7: improvements in performance and usability. Mol Biol Evol. (2013) 30:772–80. doi: 10.1093/molbev/mst010
46. Kumar S, Stecher G, Li M, Knyaz C, Tamura K. MEGA X: molecular evolutionary genetics analysis across computing platforms. Mol Biol Evol. (2018) 35:1547–9. doi: 10.1093/molbev/msy096
47. Felsenstein J. Confidence limits on phylogenies: an approach using the bootstrap. Evolution. (1985) 39:783–91. doi: 10.1111/j.1558-5646.1985.tb00420.x
48. Lee I, Ouk Kim Y, Park S-C, Chun J. OrthoANI: an improved algorithm and software for calculating average nucleotide identity. Int J Syst Evol Microbiol. (2016) 66:1100–3. doi: 10.1099/ijsem.0.000760
49. Meier-Kolthoff JP, Carbasse JS, Peinado-Olarte RL, Göker M. TYGS and LPSN: a database tandem for fast and reliable genome-based classification and nomenclature of prokaryotes. Nucleic Acids Res. (2022) 50:D801–7. doi: 10.1093/nar/gkab902
50. Bertels F, Silander OK, Pachkov M, Rainey PB, van Nimwegen E. Automated reconstruction of whole-genome phylogenies from short-sequence reads. Mol Biol Evol. (2014) 31:1077–88. doi: 10.1093/molbev/msu088
51. Xu L, Dong Z, Fang L, Luo Y, Wei Z, Guo H, et al. OrthoVenn2: a web server for whole-genome comparison and annotation of orthologous clusters across multiple species. Nucleic Acids Res. (2019) 47:W52–8. doi: 10.1093/nar/gkz333
52. Chaudhari NM, Gupta VK, Dutta C. BPGA - an ultra-fast pan-genome analysis pipeline. Sci Rep. (2016) 6:24373. doi: 10.1038/srep24373
53. Feldgarden M, Brover V, Gonzalez-Escalona N, Frye JG, Haendiges J, Haft DH, et al. AMRFinderPlus and the reference gene catalog facilitate examination of the genomic links among antimicrobial resistance, stress response, and virulence. Sci Rep. (2021) 11:12728. doi: 10.1038/s41598-021-91456-0
54. Bortolaia V, Kaas RS, Ruppe E, Roberts MC, Schwarz S, Cattoir V, et al. ResFinder 4.0 for predictions of phenotypes from genotypes. J Antimicrob Chemother. (2020) 75:3491–500. doi: 10.1093/jac/dkaa345
55. Alcock BP, Raphenya AR, Lau TTY, Tsang KK, Bouchard M, Edalatmand A, et al. CARD 2020: antibiotic resistome surveillance with the comprehensive antibiotic resistance database. Nucleic Acids Res. (2019) 48:D517–25. doi: 10.1093/nar/gkz935
56. Gupta SK, Padmanabhan BR, Diene SM, Lopez-Rojas R, Kempf M, Landraud L, et al. ARG-ANNOT, a new bioinformatic tool to discover antibiotic resistance genes in bacterial genomes. Antimicrob Agents Chemother. (2014) 58:212–20. doi: 10.1128/AAC.01310-13
57. Liu B, Zheng D, Jin Q, Chen L, Yang J. VFDB 2019: a comparative pathogenomic platform with an interactive web interface. Nucleic Acids Res. (2019) 47:D687–92. doi: 10.1093/nar/gky1080
58. Giles JA, Falconio J, Yuenger JD, Zenilman JM, Dan M, Bash MC. Quinolone resistance–determining region mutations and por type of Neisseria gonorrhoeae isolates: resistance surveillance and typing by molecular methodologies. J Infect Dis. (2004) 189:2085–93. doi: 10.1086/386312
59. Bertelli C, Laird MR, Williams KP, Lau BY, Hoad G, Winsor GL, et al. IslandViewer 4: expanded prediction of genomic islands for larger-scale datasets. Nucleic Acids Res. (2017) 45:W30–5. doi: 10.1093/nar/gkx343
60. Siguier P, Perochon J, Lestrade L, Mahillon J, Chandler M. ISfinder: the reference centre for bacterial insertion sequences. Nucleic Acids Res. (2006) 34:D32–6. doi: 10.1093/nar/gkj014
61. Ross K, Varani AM, Snesrud E, Huang H, Alvarenga DO, Zhang J, et al. TnCentral: a prokaryotic transposable element database and web portal for transposon analysis. mBio. (2021) 12:e0206021. doi: 10.1128/mBio.02060-21
62. Afgan E, Baker D, Batut B, van den Beek M, Bouvier D, Čech M, et al. The Galaxy platform for accessible, reproducible and collaborative biomedical analyses: 2018 update. Nucleic Acids Res. (2018) 46:W537–44. doi: 10.1093/nar/gky379
63. Couvin D, Bernheim A, Toffano-Nioche C, Touchon M, Michalik J, Néron B, et al. CRISPRCasFinder, an update of CRISRFinder, includes a portable version, enhanced performance and integrates search for Cas proteins. Nucleic Acids Res. (2018) 46:W246–51. doi: 10.1093/nar/gky425
64. Arndt D, Grant JR, Marcu A, Sajed T, Pon A, Liang Y, et al. PHASTER: a better, faster version of the PHAST phage search tool. Nucleic Acids Res. (2016) 44:W16–21. doi: 10.1093/nar/gkw387
65. Liu M, Li X, Xie Y, Bi D, Sun J, Li J, et al. ICEberg 2.0: an updated database of bacterial integrative and conjugative elements. Nucleic Acids Res. (2019) 47:D660–5. doi: 10.1093/nar/gky1123
66. Bellais S, Poirel L, Leotard S, Naas T. Genetic diversity of carbapenem-hydrolyzing metallo-beta-lactamases from Chryseobacterium (Flavobacterium) indologenes. Antimicrob Agents Chemother. (2000) 44:3028–34. doi: 10.1128/aac.44.11.3028-3034.2000
67. Matsumoto T, Nagata M, Ishimine N, Kawasaki K, Yamauchi K, Hidaka E, et al. Characterization of CIA-1, an Ambler class A extended-spectrum β-lactamase from Chryseobacterium indologenes. Antimicrob Agents Chemother. (2012) 56:588–90. doi: 10.1128/AAC.05165-11
68. Yang W, Moore IF, Koteva KP, Bareich DC, Hughes DW, Wright GD. TetX is a flavin-dependent monooxygenase conferring resistance to tetracycline antibiotics. J Biol Chem. (2004) 279:52346–52. doi: 10.1074/jbc.M409573200
69. Shaw KJ, Rather PN, Hare RS, Miller GH. Molecular genetics of aminoglycoside resistance genes and familial relationships of the aminoglycoside-modifying enzymes. Microbiol Rev. (1993) 57:138–63. doi: 10.1128/mr.57.1.138-163.1993
70. Wareth G, Brandt C, Sprague LD, Neubauer H, Pletz MW. WGS based analysis of acquired antimicrobial resistance in human and non-human Acinetobacter baumannii isolates from a German perspective. BMC Microbiol. (2021) 21:210. doi: 10.1186/s12866-021-02270-7
71. Antunes P, Machado J, Sousa JC, Peixe L. Dissemination of sulfonamide resistance genes (sul1, sul2, and sul3) in Portuguese Salmonella enterica strains and relation with integrons. Antimicrob Agents Chemother. (2005) 49:836–9. doi: 10.1128/AAC.49.2.836-839.2005
72. Halula MC, Manning S, Macrina FL. Nucleotide sequence of ermFU, a macrolide-lincosamide-streptogramin (MLS) resistance gene encoding an RNA methylase from the conjugal element of Bacteroides fragilis V503 (BF12256). Nucleic Acids Res. (1991) 19:3453.
73. van Duin D, Paterson DL. Multidrug-Resistant Bacteria in the Community. Infect Dis Clin North Am. (2016) 30:377–90. doi: 10.1016/j.idc.2016.02.004
74. Bhagawati G, Bhardwaj A, Sajikumar R, Singh SP, Prajapati S. Bacteraemia by chryseobacterium indologenes in a patient with lung cancer: a clinical and microbiological investigation. Indian J Crit Care Med. (2019) 23:157–9. doi: 10.5005/jp-journals-10071-23142
75. Atıcı S, Ünkar ZA, Erdem K, Kadayifci EK, Karaaslan A, Memişoğlu AÇ, et al. Ventilator-associated pneumonia caused by Chryseobacterium indologenes: a rare infant case and review of the literature. Springerplus. (2016) 5:1741. doi: 10.1186/s40064-016-3449-x
76. Mirza IA, Khalid A, Hameed F, Imtiaz A, Ashfaq A, Tariq A. Chryseobacterium indologenes as a novel cause of bacteremia in a neonate. J Coll Physicians Surg Pak. (2019) 29:375–8. doi: 10.29271/jcpsp.2019.04.375
77. Smith J, Han R, Mailman T, MacDonald N. Chryseobacterium indologenes: distinguishing pathogen from contaminant in a neonate. J Pediatr Infect Dis. (2015) 07:31–5. doi: 10.3233/JPI-2012-0337
78. Cimmino T, Rolain JM. Whole genome sequencing for deciphering the resistome of Chryseobacterium indologenes, an emerging multidrug-resistant bacterium isolated from a cystic fibrosis patient in Marseille, France. New Microbes New Infect. (2016) 12:35–42. doi: 10.1016/j.nmni.2016.03.006
79. Giacobbe DR, Giani T, Bassetti M, Marchese A, Viscoli C, Rossolini GM. Rapid microbiological tests for bloodstream infections due to multidrug resistant gram-negative bacteria: therapeutic implications. Clin Microbiol Infect. (2020) 26:713–22. doi: 10.1016/j.cmi.2019.09.023
80. Ozcan N, Dal T, Tekin A, Kelekci S, Can S, Ezin O, et al. Is Chryseobacterium indologenes a shunt-lover bacterium? A case report and review of the literature. Infez Med. (2013) 21:312–6.
81. Lin Y-T, Jeng Y-Y, Lin M-L, Yu K-W, Wang F-D, Liu C-Y. Clinical and microbiological characteristics of Chryseobacterium indologenes bacteremia. J Microbiol Immunol Infect. (2010) 43:498–505. doi: 10.1016/S1684-1182(10)60077-1
82. Chang Y-C, Lo H-H, Hsieh H-Y, Chang S-M. Identification, epidemiological relatedness, and biofilm formation of clinical Chryseobacterium indologenes isolates from central Taiwan. J Microbiol Immunol Infect. (2015) 48:559–64. doi: 10.1016/j.jmii.2014.04.004
83. NIHR Global Health Research Unit on Genomic Surveillance of AMR. Whole-genome sequencing as part of national and international surveillance programmes for antimicrobial resistance: a roadmap. BMJ Glob Health. (2020) 5:e002244. doi: 10.1136/bmjgh-2019-002244
84. Liang C-Y, Yang C-H, Lai C-H, Huang Y-H, Lin J-N. Genomic features, comparative genomic analysis, and antimicrobial susceptibility patterns of chryseobacterium arthrosphaerae strain ED882-96 isolated in Taiwan. Genes. (2019) 10:309. doi: 10.3390/genes10040309
85. Gomila M, Peña A, Mulet M, Lalucat J, García-Valdés E. Phylogenomics and systematics in Pseudomonas. Front Microbiol. (2015) 6:214. doi: 10.3389/fmicb.2015.00214
86. Janda JM, Abbott SL. 16S rRNA gene sequencing for bacterial identification in the diagnostic laboratory: pluses, perils, and pitfalls. J Clin Microbiol. (2007) 45:2761–4. doi: 10.1128/JCM.01228-07
87. Lalucat J, Mulet M, Gomila M, García-Valdés E. Genomics in bacterial taxonomy: impact on the genus Pseudomonas. Genes. (2020) 11:139. doi: 10.3390/genes11020139
88. Asnicar F, Thomas AM, Beghini F, Mengoni C, Manara S, Manghi P, et al. Precise phylogenetic analysis of microbial isolates and genomes from metagenomes using PhyloPhlAn 3.0. Nat Commun. (2020) 11:2500. doi: 10.1038/s41467-020-16366-7
89. Aulitto M, Martinez-Alvarez L, Fiorentino G, Limauro D, Peng X, Contursi P. A comparative analysis of Weizmannia coagulans genomes unravels the genetic potential for biotechnological applications. Int J Mol Sci. (2022) 23:3135. doi: 10.3390/ijms23063135
90. Hong CE, Kim JU, Bang KH, Jo I-H. Complete genome sequence of the endophytic bacterium Chryseobacterium indologenes PgBE177, Isolated from Panax quinquefolius. Microbiol Resour Announ. (2018) 7:e01234–18. doi: 10.1128/MRA.01234-18
91. Niu B, Paulson JN, Zheng X, Kolter R. Simplified and representative bacterial community of maize roots. Proc Natl Acad Sci USA. (2016) 114:E2450–9. doi: 10.1073/pnas.1616148114
92. Sichtig H, Minogue T, Yan Y, Stefan C, Hall A, Tallon L, et al. FDA-ARGOS is a database with public quality-controlled reference genomes for diagnostic use and regulatory science. Nat Commun. (2019) 10:3313. doi: 10.1038/s41467-019-11306-6
93. Kang D, Shoaie S, Jacquiod S, Sørensen SJ, Ledesma-Amaro R. Comparative genomics analysis of keratin-degrading chryseobacterium species reveals their keratinolytic potential for secondary metabolite production. Microorganisms. (2021) 9:1042. doi: 10.3390/microorganisms9051042
94. Rabenandrasana MAN, Rafetrarivony LF, Rivoarilala LO, Enouf V, Robinson AL, Rakotozanany A, et al. Draft genome sequence of a Chryseobacterium indologenes strain isolated from a blood culture of a hospitalized child in Antananarivo, Madagascar. Microbiol Resour Announc. (2019) 8:e00752–19. doi: 10.1128/MRA.00752-19
95. Bellais S, Léotard S, Poirel L, Naas T, Nordmann P. Molecular characterization of a carbapenem-hydrolyzing beta-lactamase from Chryseobacterium (Flavobacterium) indologenes. FEMS Microbiol Lett. (1999) 171:127–32. doi: 10.1111/j.1574-6968.1999.tb13422.x
96. Yum JH. Genetic diversity of metallo-β-lactamase genes of Chryseobacterium indologenes isolates from Korea. Biomed Sci Lett. (2019) 25:275–81. doi: 10.15616/BSL.2019.25.3.275
97. Jeong SH, Bae IK, Lee JH, Sohn SG, Kang GH, Jeon GJ, et al. Molecular characterization of extended-spectrum beta-lactamases produced by clinical isolates of Klebsiella pneumoniae and Escherichia coli from a Korean nationwide survey. J Clin Microbiol. (2004) 42:2902–6. doi: 10.1128/JCM.42.7.2902-2906.2004
98. Dropa M, Balsalobre LC, Lincopan N, Mamizuka EM, Cassettari VC, Matté GR, et al. Emergence of Klebsiella pneumoniae carrying the novel extended-spectrum β-lactamase gene variants blaSHV-40, blaTEM-116 and the class 1 integronassociated blaGES-7 in Brazil. Clin Microbiol Infect. (2010) 16:630–2. doi: 10.1111/j.1469-0691.2009.02944.x
99. Rojas MVR, Matté MH, Dropa M, da Silva ML, Matté GR. Characterization of Vibrio Parahaemolyticus isolated from oysters and mussels in São Paulo, Brazil. Rev Inst Med Trop São Paulo. (2011) 53:201–5. doi: 10.1590/S0036-46652011000400005
100. Balsalobre LC, Dropa M, de Oliveira DE, Lincopan N, Mamizuka EM, Matté GR, et al. Presence of blaTEM-116 gene in environmental isolates of Aeromonas hydrophila and Aeromonas jandaei from Brazil. Braz J Microbiol. (2010) 41:718–9. doi: 10.1590/S1517-83822010000300023
101. Lahlaoui H, Dahmen S, Moussa MB, Omrane B. First detection of TEM-116 extended-spectrum β-lactamase in a Providencia stuartii isolate from a Tunisian hospital. Indian J Med Microbiol. (2011) 29:258–61. doi: 10.4103/0255-0857.83909
102. Lahiri SD, Alm RA. Identification of novel VEB β-lactamase enzymes and their impact on avibactam inhibition. Antimicrob Agents Chemother. (2016) 60:3183–6. doi: 10.1128/AAC.00047-16
103. Brolund A. Overview of ESBL-producing Enterobacteriaceae from a Nordic perspective. Infect Ecol Epidemiol. (2014) 4:24555. doi: 10.3402/iee.v4.24555
104. Talebi G, Hashemi A. Metallo-β-lactamase, extended spectrum β-lactamase and mcr-1 gene as major therapeutic challenges. Rev Med Microbiol. (2022) 33:e40–7. doi: 10.1097/MRM.0000000000000249
105. Chen Y-P, Lee S-H, Chou C-H, Tsai H-J. Detection of florfenicol resistance genes in Riemerella anatipestifer isolated from ducks and geese. Vet Microbiol. (2012) 154:325–31. doi: 10.1016/j.vetmic.2011.07.012
106. Ming D, Chen Q, Chen X. Analysis of resistance genes in pan-resistant Myroides odoratimimus clinical strain PR63039 using whole genome sequencing. Microb Pathogen. (2017) 112:164–70. doi: 10.1016/j.micpath.2017.09.012
107. Moore IF, Hughes DW, Wright GD. Tigecycline is modified by the flavin-dependent monooxygenase TetX. Biochemistry. (2005) 44:11829–35. doi: 10.1021/bi0506066
108. Guiney DG, Hasegawa P, Davis CE. Expression in Escherichia coli of cryptic tetracycline resistance genes from bacteroides R plasmids. Plasmid. (1984) 11:248–52. doi: 10.1016/0147-619x(84)90031-3
109. Speer BS, Salyers AA. A tetracycline efflux gene on Bacteroides transposon Tn4400 does not contribute to tetracycline resistance. J Bacteriol. (1990) 172:292–8. doi: 10.1128/jb.172.1.292-298.1990
110. Walkiewicz K, Davlieva M, Wu G, Shamoo Y. Crystal structure of Bacteroides thetaiotaomicron TetX2: a tetracycline degrading monooxygenase at 2.8 Å resolution. Proteins. (2011) 79:2335–40. doi: 10.1002/prot.23052
111. Leski TA, Bangura U, Jimmy DH, Ansumana R, Lizewski SE, Stenger DA, et al. Multidrug-resistant tet(X)-containing hospital isolates in Sierra Leone. Int J Antimicrob Agents. (2013) 42:83–6. doi: 10.1016/j.ijantimicag.2013.04.014
112. Hooper DC, Jacoby GA. Topoisomerase inhibitors: fluoroquinolone mechanisms of action and resistance. Cold Spring Harb Perspect Med. (2016) 6:a025320. doi: 10.1101/cshperspect.a025320
113. Nordmann P, Poirel L. Emergence of plasmid-mediated resistance to quinolones in Enterobacteriaceae. J Antimicrob Chemother. (2005) 56:463–9. doi: 10.1093/jac/dki245
114. Lin JN, Lai CH, Yang CH, Huang YH. Differences in clinical manifestations, antimicrobial susceptibility patterns, and mutations of fluoroquinolone target genes between Chryseobacterium gleum and Chryseobacterium indologenes. Antimicrob Agents Chemother. (2019) 63:e02256–18. doi: 10.1128/AAC.02256-18
115. Robicsek A, Jacoby GA, Hooper DC. The worldwide emergence of plasmid-mediated quinolone resistance. Lancet Infect Dis. (2006) 6:629–40. doi: 10.1016/S1473-3099(06)70599-0
116. Garrido MC, Herrero M, Kolter R, Moreno F. The export of the DNA replication inhibitor Microcin B17 provides immunity for the host cell. EMBO J. (1988) 7:1853–62. doi: 10.1002/j.1460-2075.1988.tb03018.x
117. Moffatt JH, Harper M, Harrison P, Hale JDF, Vinogradov E, Seemann T, et al. Colistin resistance in Acinetobacter baumannii is mediated by complete loss of lipopolysaccharide production. Antimicrob Agents Chemother. (2010) 54:4971–7. doi: 10.1128/AAC.00834-10
118. Sharma P, Gupta SK, Diene SM, Rolain J-M. Whole-genome sequence of Chryseobacterium oranimense, a colistin-resistant bacterium isolated from a cystic fibrosis patient in France. Antimicrob Agents Chemother. (2015) 59:1696–706. doi: 10.1128/AAC.02417-14
119. Founou RC, Founou LL, Allam M, Ismail A, Essack SY. Whole Genome Sequencing of Extended Spectrum β-lactamase (ESBL)-producing Klebsiella pneumoniae Isolated from Hospitalized Patients in KwaZulu-Natal, South Africa. Sci Rep. (2019) 9:6266. doi: 10.1038/s41598-019-42672-2
120. Pimenta AL, Racher K, Jamieson L, Blight MA, Holland IB. Mutations in HlyD, part of the type 1 translocator for hemolysin secretion, affect the folding of the secreted toxin. J Bacteriol. (2005) 187:7471–80. doi: 10.1128/JB.187.21.7471-7480.2005
121. Cheah S-E, Johnson MD, Zhu Y, Tsuji BT, Forrest A, Bulitta JB, et al. Polymyxin Resistance in Acinetobacter baumannii: genetic mutations and transcriptomic changes in response to clinically relevant dosage regimens. Sci Rep. (2016) 6:26233. doi: 10.1038/srep26233
122. El-Sayed Ahmed MAE, Zhong L-L, Shen C, Yang Y, Doi Y, Tian G-B. Colistin and its role in the Era of antibiotic resistance: an extended review (2000–2019). Emerg Microbes Infect. (2020) 9:868–85. doi: 10.1080/22221751.2020.1754133
123. Koronakis V, Sharff A, Koronakis E, Luisi B, Hughes C. Crystal structure of the bacterial membrane protein TolC central to multidrug efflux and protein export. Nature. (2000) 405:914–9. doi: 10.1038/35016007
124. Sharff A, Fanutti C, Shi J, Calladine C, Luisi B. The role of the TolC family in protein transport and multidrug efflux. From stereochemical certainty to mechanistic hypothesis. Eur J Biochem. (2001) 268:5011–26. doi: 10.1046/j.0014-2956.2001.02442.x
125. Kim J-S, Song S, Lee M, Lee S, Lee K, Ha N-C. Crystal structure of a soluble fragment of the membrane fusion protein HlyD in a type I secretion system of gram-negative bacteria. Structure. (2016) 24:477–85. doi: 10.1016/j.str.2015.12.012
126. Li S, Chen Q, Gong X, Liu Y, Zheng F. RanB, a putative ABC-type multidrug efflux transporter contributes to aminoglycosides resistance and organic solvents tolerance in Riemerella anatipestifer. Vet Microbiol. (2020) 243:108641. doi: 10.1016/j.vetmic.2020.108641
127. Wang T, Jiang X, Feng C, Li A, Dong H, Wu S, et al. Whole genome sequencing uncovers a novel IND-16 metallo-β-lactamase from an extensively drug-resistant Chryseobacterium indologenes strain J31. Gut Pathogens. (2016) 8:47. doi: 10.1186/s13099-016-0130-4
128. Wang M, Gao H, Lin N, Zhang Y, Huang N, Walker ED, et al. The antibiotic resistance and pathogenicity of a multidrug-resistant Elizabethkingia anophelis isolate. Microbiologyopen. (2019) 8:e804. doi: 10.1002/mbo3.804
129. Kim J-C, Oh E, Kim J, Jeon B. Regulation of oxidative stress resistance in Campylobacter jejuni, a microaerophilic foodborne pathogen. Front Microbiol. (2015) 6:751. doi: 10.3389/fmicb.2015.00751
130. Zhang L, Alfano JR, Becker DF. Proline metabolism increases katG expression and oxidative stress resistance in Escherichia coli. J Bacteriol. (2015) 197:431–40. doi: 10.1128/JB.02282-14
131. Harvey KL, Jarocki VM, Charles IG, Djordjevic SP. The diverse functional roles of elongation factor Tu (EF-Tu) in microbial pathogenesis. Front Microbiol. (2019) 10:2351. doi: 10.3389/fmicb.2019.02351
132. Konieczna I, Zarnowiec P, Kwinkowski M, Kolesinska B, Fraczyk J, Kaminski Z, et al. Bacterial urease and its role in long-lasting human diseases. Curr Protein Pept Sci. (2012) 13:789–806. doi: 10.2174/138920312804871094
133. Deng DM, ten Cate JM, Crielaard W. The adaptive response of Streptococcus mutans towards oral care products: involvement of the ClpP serine protease. Eur J Oral Sci. (2007) 115:363–70. doi: 10.1111/j.1600-0722.2007.00477.x
134. Zhang Q, Xu S-X, Wang H, Xu W-C, Zhang X-M, Wu K-F, et al. Contribution of ClpE to virulence of Streptococcus pneumoniae. Can J Microbiol. (2009) 55:1187–94. doi: 10.1139/W09-078
135. Little K, Tipping MJ, Gibbs KA. Swarmer cell development of the bacterium Proteus mirabilis requires the conserved enterobacterial common antigen biosynthesis gene rffG. J Bacteriol. (2018) 200:e00230–18. doi: 10.1128/JB.00230-18
136. Bruchmann S, Feltwell T, Parkhill J, Short FL. Identifying virulence determinants of multidrug-resistant Klebsiella pneumoniae in Galleria mellonella. Pathog Dis. (2021) 79:ftab009. doi: 10.1093/femspd/ftab009
137. Nesper J, Lauriano CM, Klose KE, Kapfhammer D, Kraiss A, Reidl J. Characterization of Vibrio cholerae O1 El tor galU and galE mutants: influence on lipopolysaccharide structure, colonization, and biofilm formation. Infect Immun. (2001) 69:435–45. doi: 10.1128/IAI.69.1.435-445.2001
138. Zou Y, Feng S, Xu C, Zhang B, Zhou S, Zhang L, et al. The role of galU and galE of Haemophilus parasuis SC096 in serum resistance and biofilm formation. Vet Microbiol. (2013) 162:278–84. doi: 10.1016/j.vetmic.2012.08.006
139. Feng S, Chen A, Wang X, Pan Z, Xu S, Yu H, et al. The Glaesserella parasuis phosphoglucomutase is partially required for lipooligosaccharide synthesis. Vet Res. (2020) 51:97. doi: 10.1186/s13567-020-00822-9
140. Staton GJ, Clegg SR, Ainsworth S, Armstrong S, Carter SD, Radford AD, et al. Dissecting the molecular diversity and commonality of bovine and human treponemes identifies key survival and adhesion mechanisms. PLoS Pathog. (2021) 17:e1009464. doi: 10.1371/journal.ppat.1009464
141. Bank NC, Singh V, Rodriguez-Palacios A. Classification of Parabacteroides distasonis and other Bacteroidetes using O- antigen virulence gene: RfbA -Typing and hypothesis for pathogenic vs. probiotic strain differentiation. Gut Microbes. (2022) 14:1997293. doi: 10.1080/19490976.2021.1997293
142. Bian X, Liu X, Zhang X, Li X, Zhang J, Zheng H, et al. Epidemiological and genomic characteristics of Acinetobacter baumannii from different infection sites using comparative genomics. BMC Genomics. (2021) 22:530. doi: 10.1186/s12864-021-07842-5
143. Lim S, Lee D-H, Kwak W, Shin H, Ku H-J, Lee J, et al. Comparative genomic analysis of staphylococcus aureus FORC_001 and S. aureus MRSA252 reveals the characteristics of antibiotic resistance and virulence factors for human infection. J Microbiol Biotechnol. (2015) 25:98–108. doi: 10.4014/jmb.1410.10005
144. Chen S, Soehnlen M, Blom J, Terrapon N, Henrissat B, Walker ED. Comparative genomic analyses reveal diverse virulence factors and antimicrobial resistance mechanisms in clinical Elizabethkingia meningoseptica strains. PLoS One. (2019) 14:e0222648. doi: 10.1371/journal.pone.0222648
145. Ford DC, Joshua GWP, Wren BW, Oyston PCF. The importance of the magnesium transporter MgtB for virulence of Yersinia pseudotuberculosis and Yersinia pestis. Microbiology. (2014) 160:2710–7. doi: 10.1099/mic.0.080556-0
146. Park M, Kim H, Nam D, Kweon D-H, Shin D. The mgtCBR mRNA leader secures growth of Salmonella in both host and non-host environments. Front Microbiol. (2019) 10:2831. doi: 10.3389/fmicb.2019.02831
147. Duangurai T, Reamtong O, Rungruengkitkun A, Srinon V, Boonyuen U, Limmathurotsakul D, et al. In vitro passage alters virulence, immune activation and proteomic profiles of Burkholderia pseudomallei. Sci Rep. (2020) 10:8320. doi: 10.1038/s41598-020-64914-4
148. Lee K-J, Lee NY, Han Y-S, Kim J, Lee K-H, Park S-J. Functional characterization of the IlpA protein of Vibrio vulnificus as an adhesin and its role in bacterial pathogenesis. Infect Immun. (2010) 78:2408–17. doi: 10.1128/IAI.01194-09
149. Pérez-Duque A, Gonzalez-Muñoz A, Arboleda-Valencia J, Vivas-Aguas LJ, Córdoba-Meza T, Rodriguez-Rey GT, et al. Comparative genomics of clinical and environmental isolates of vibrio spp. of Colombia: implications of traits associated with virulence and resistance. Pathogens. (2021) 10:1605. doi: 10.3390/pathogens10121605
150. Pedrosa-Silva F, Matteoli FP, Passarelli-Araujo H, Olivares FL, Venancio TM. Genome sequencing of the vermicompost strain Stenotrophomonas maltophilia UENF-4GII and population structure analysis of the S. maltophilia Sm3 genogroup. Microbiol Res. (2022) 255:126923. doi: 10.1016/j.micres.2021.126923
151. Sato K, Naito M, Yukitake H, Hirakawa H, Shoji M, McBride MJ, et al. A protein secretion system linked to bacteroidete gliding motility and pathogenesis. Proc Natl Acad Sci USA. (2010) 107:276–81. doi: 10.1073/pnas.0912010107
152. Gonçalves OS, de Queiroz MV, Santana MF. Potential evolutionary impact of integrative and conjugative elements (ICEs) and genomic islands in the Ralstonia solanacearum species complex. Sci Rep. (2020) 10:12498. doi: 10.1038/s41598-020-69490-1
153. Juhas M, van der Meer JR, Gaillard M, Harding RM, Hood DW, Crook DW. Genomic islands: tools of bacterial horizontal gene transfer and evolution. FEMS Microbiol Rev. (2009) 33:376–93. doi: 10.1111/j.1574-6976.2008.00136.x
154. Partridge SR. Analysis of antibiotic resistance regions in Gram-negative bacteria. FEMS Microbiol Rev. (2011) 35:820–55. doi: 10.1111/j.1574-6976.2011.00277.x
155. Fronzes R, Christie PJ, Waksman G. The structural biology of type IV secretion systems. Nat Rev Microbiol. (2009) 7:703–14. doi: 10.1038/nrmicro2218
156. Souza RC, del Rosario Quispe Saji G, Costa MO, Netto DS, Lima NC, Klein CC, et al. AtlasT4SS: a curated database for type IV secretion systems. BMC Microbiol. (2012) 12:172. doi: 10.1186/1471-2180-12-172
157. Gomis-Rüth FX, Moncalián G, Pérez-Luque R, González A, Cabezón E, de la Cruz F. The bacterial conjugation protein TrwB resembles ring helicases and F1-ATPase. Nature. (2001) 409:637–41. doi: 10.1038/35054586
158. Burrus V, Marrero J, Waldor MK. The current ICE age: biology and evolution of SXT-related integrating conjugative elements. Plasmid. (2006) 55:173–83. doi: 10.1016/j.plasmid.2006.01.001
159. Wozniak RAF, Waldor MK. Integrative and conjugative elements: mosaic mobile genetic elements enabling dynamic lateral gene flow. Nat Rev Microbiol. (2010) 8:552–63. doi: 10.1038/nrmicro2382
160. de Maayer P, Chan W-Y, Martin DAJ, Blom J, Venter SN, Duffy B, et al. Integrative conjugative elements of the ICEPan family play a potential role in Pantoea ananatis ecological diversification and antibiosis. Front Microbiol. (2015) 6:576. doi: 10.3389/fmicb.2015.00576
161. Chiou CS, Jones AL. Nucleotide sequence analysis of a transposon (Tn5393) carrying streptomycin resistance genes in Erwinia amylovora and other gram-negative bacteria. J Bacteriol. (1993) 175:732–40. doi: 10.1128/jb.175.3.732-740.1993
162. Cain AK, Hall RM. Transposon Tn 5393 carrying the aphA1-containing transposon Tn 6023 upstream of strAB does not confer resistance to streptomycin. Microb Drug Resist. (2011) 17:389–94. doi: 10.1089/mdr.2011.0037
163. Abdelhamed H, Ramachandran R, Ozdemir O, Waldbieser G, Lawrence ML. Characterization of a novel conjugative plasmid in Edwardsiella piscicida strain MS-18-199. Front Cell Infect Microbiol. (2019) 9:404. doi: 10.3389/fcimb.2019.00404
164. Hu S, Cao L, Wu Y, Zhou Y, Jiang T, Wang L, et al. Comparative genomic analysis of Myroides odoratimimus isolates. Microbiologyopen. (2019) 8:e00634. doi: 10.1002/mbo3.634
165. Laraki N, Galleni M, Thamm I, Riccio ML, Amicosante G, Frère JM, et al. Structure of In31, a blaIMP-containing Pseudomonas aeruginosa integron phyletically related to In5, which carries an unusual array of gene cassettes. Antimicrob Agents Chemother. (1999) 43:890–901. doi: 10.1128/AAC.43.4.890
166. Orlek A, Stoesser N, Anjum MF, Doumith M, Ellington MJ, Peto T, et al. Plasmid classification in an era of whole-genome sequencing: application in studies of antibiotic resistance epidemiology. Front Microbiol. (2017) 8:182. doi: 10.3389/fmicb.2017.00182
167. Berbers B, Ceyssens P-J, Bogaerts P, Vanneste K, Roosens NHC, Marchal K, et al. Development of an NGS-based workflow for improved monitoring of circulating plasmids in support of risk assessment of antimicrobial resistance gene dissemination. Antibiotics. (2020) 9:503. doi: 10.3390/antibiotics9080503
168. Evans DR, Griffith MP, Sundermann AJ, Shutt KA, Saul MI, Mustapha MM, et al. Systematic detection of horizontal gene transfer across genera among multidrug-resistant bacteria in a single hospital. Elife. (2020) 9:e53886. doi: 10.7554/eLife.53886
169. Bunny KL, Hall RM, Stokes HW. New mobile gene cassettes containing an aminoglycoside resistance gene, aacA7, and a chloramphenicol resistance gene, catB3, in an integron in pBWH301. Antimicrob Agents Chemother. (1995) 39:686–93. doi: 10.1128/AAC.39.3.686
170. Houang ETS, Chu Y-W, Lo W-S, Chu K-Y, Cheng AFB. Epidemiology of rifampin ADP-ribosyltransferase (arr-2) and metallo-β-Lactamase (blaIMP-4) gene cassettes in class 1 integrons in acinetobacter strains isolated from blood cultures in 1997 to 2000. Antimicrob Agents Chemother. (2003) 47:1382–90. doi: 10.1128/AAC.47.4.1382-1390.2003
Keywords: Chryseobacterium indologenes, whole-genome sequencing, virulence and resistance genes, mobile genetic elements, neonatal intensive care unit, metabolic features
Citation: Damas MSF, Ferreira RL, Campanini EB, Soares GG, Campos LC, Laprega PM, Soares-Costa A, Freire CCM, Pitondo-Silva A, Cerdeira LT, Cunha AF and Pranchevicius MCS (2022) Whole genome sequencing of the multidrug-resistant Chryseobacterium indologenes isolated from a patient in Brazil. Front. Med. 9:931379. doi: 10.3389/fmed.2022.931379
Received: 05 June 2022; Accepted: 30 June 2022;
Published: 28 July 2022.
Edited by:
Xiaojiong Jia, Harvard Medical School, United StatesReviewed by:
Polly Soo Xi Yap, Monash University Malaysia, MalaysiaJoshy M. Easow, Sri Balaji Vidyapeeth University, India
Copyright © 2022 Damas, Ferreira, Campanini, Soares, Campos, Laprega, Soares da Costa, Freire, Pitondo-Silva, Cerdeira, Cunha and Pranchevicius. This is an open-access article distributed under the terms of the Creative Commons Attribution License (CC BY). The use, distribution or reproduction in other forums is permitted, provided the original author(s) and the copyright owner(s) are credited and that the original publication in this journal is cited, in accordance with accepted academic practice. No use, distribution or reproduction is permitted which does not comply with these terms.
*Correspondence: Maria-Cristina da Silva Pranchevicius, bWNzcHJhbmNAZ21haWwuY29t
†These authors have contributed equally to this work