- 1Regulative Physiology and Prevention, Department of Sports and Health Sciences, University Potsdam, Potsdam, Germany
- 2Practice of Integrative Medicine Bittmann, Potsdam, Germany
The increasing prevalence of Long COVID is an imminent public health disaster, and established approaches have not provided adequate diagnostics or treatments. Recently, anesthetic blockade of the stellate ganglion was reported to improve Long COVID symptoms in a small case series, purportedly by “rebooting” the autonomic nervous system. Here, we present a novel diagnostic approach based on the Adaptive Force (AF), and report sustained positive outcome for one severely affected Long COVID patient using individualized pulsed electromagnetic field (PEMF) at the area C7/T1. AF reflects the capacity of the neuromuscular system to adapt adequately to external forces in an isometric holding manner. In case, maximal isometric AF (AFisomax) is exceeded, the muscle merges into eccentric muscle action. Thereby, the force usually increases further until maximal AF (AFmax) is reached. In case adaptation is optimal, AFisomax is ~99–100% of AFmax. This holding capacity (AFisomax) was found to be vulnerable to disruption by unpleasant stimulus and, hence, was regarded as functional parameter. AF was assessed by an objectified manual muscle test using a handheld device. Prior to treatment, AFisomax was considerably lower than AFmax for hip flexors (62 N = ~28% AFmax) and elbow flexors (71 N = ~44% AFmax); i.e., maximal holding capacity was significantly reduced, indicating dysfunctional motor control. We tested PEMF at C7/T1, identified a frequency that improved neuromuscular function, and applied it for ~15 min. Immediately post-treatment, AFisomax increased to ~210 N (~100% AFmax) at hip and 184 N (~100% AFmax) at elbow. Subjective Long COVID symptoms resolved the following day. At 4 weeks post-treatment, maximal holding capacity was still on a similarly high level as for immediately post-treatment (~100% AFmax) and patient was symptom-free. At 6 months the patient's Long COVID symptoms have not returned. This case report suggests (1) AF could be a promising diagnostic for post-infectious illness, (2) AF can be used to test effective treatments for post-infectious illness, and (3) individualized PEMF may resolve post-infectious symptoms.
1. Introduction
“Long COVID” receives increasing attention due to the high number of affected persons during SARS-CoV-2 pandemic. Six month post-infection 57% of COVID-19 survivors show one or more sequelae, after 1 year still half of them present at least one symptom (1, 2), regardless of infection severity (3). Long COVID shows similarities to myalgic encephalomyelitis/chronic fatigue syndrome (ME/CFS) (4–9), which is known since decades and can arise after viral infections (7–12). For post-infectious syndromes a dysfunction of the autonomous nervous system (ANS) was discussed to be the cause or at least a component (4, 7–9). The underlying mechanisms, the causality and the influence of pre-existing health conditions are not sufficiently known (1, 13). Innovative diagnostics and efficient causal therapies are urgently needed (14, 15).
Recently, Liu and Duricka reported sustained positive clinical outcomes for two Long COVID patients after stellate ganglion block (SGB), i.e., injecting local anesthetics near the stellate ganglion (4). Based on the rapid resolution of symptoms the authors concluded the “system needs to ‘reboot' to produce functional recovery” (4). The positive effect of SGB was suggested to be based on “sympathectomy,” which “produces its beneficial effects… by attenuating chronic sympathetic hyper responsiveness, improving cerebral and regional blood flow, and recalibrating the autonomic nervous system toward pre-COVID homeostasis” or “rebalancing the interaction between the nervous and immune system” (4).
Despite of delaying broad acceptance as valid treatment (4), therapeutic local anesthesia to sympathetic ganglia is supposed to be a promising approach for relieving severe conditions (16–20). It is applied since decades to treat several conditions, e.g., acute/chronic pain, functional disorders, dysautonomia, and chronic inflammation (16, 21). SGB, e.g., reduced the symptoms in patients with posttraumatic stress disorders (22, 23), may modulate the immune response (24), or stabilized ventricular rhythm (25). The local injection is claimed to be safe (4, 21), however, it is invasive and involves some risks (21, 26).
Another approach to influence the ANS is the use of weak, low-frequency pulsatile electromagnetic fields (PEMF) (27). Animal studies support the hypothesis that PEMF can be useful in therapy (27–30), e.g., in cardiac diseases (27, 30, 31). In humans, PEMF could normalize dysautonomia in children (32, 33) and was found to be effective to treat neuropathic/postsurgical pain and edema as well as several other indications (34–37). PEMF acupuncture of BL15 (bladder meridian and paravertebral T5) was found to activate the parasympathetic nervous system (38). Moreover, PEMF showed positive effects in cancer treatment (39). It modulated the physiology and electrochemistry of cancer cells and had immunomodulatory and systematic effects (39–41). PEMF was suggested to be a “suitable therapeutic approach with neuroimmunomodulatory, anti-inflammatory, anti-hyperglycemic, anti-hyperalgesic, and anti-allodynic actions” (35). Despite of those findings, development of PEMF therapy is slow due to the lack of scientifical evidence-based knowledge (36). Furthermore, the application parameters of PEMF were claimed to be “quite diverse, with no clear rationale for why particular parameters are chosen” (35).
Based on the above-mentioned knowledge and own clinical experience, we hypothesize (1) individualized PEMF in the sense of non-invasive neural therapy can be useful for treatment of dysautonomia in Long COVID; (2) the appropriate and helpful application parameters of PEMF can be tested by Adaptive Force (AF); (3) The AF can serve as biomarker (diagnostic/follow-up).
The AF characterizes the holding capacity of the neuromuscular system, which can be assessed, e.g., by a manual muscle test (MMT) objectified by a handheld device (42–44). During MMT, the tester applies a smoothly increasing force on the patient's limb in direction of muscle lengthening up to a considerably high force level. In case, the patient can adapt the muscle tension maintaining the isometric position during the entire force increase, the MMT is rated as “stable” and the maximal AF (AFmax) is reached under isometric conditions [AFmax = maximal isometric AF (AFisomax)]. An “unstable” adaptation is characterized by yielding of the limb during force increase. The patient is not able to adapt adequately. AFisomax is considerably low and AFmax is reached during eccentric muscle action (43–45).
Healthy persons usually show stable adaptation ( 99%) (43–45). Based on own practical experience, patients with, e.g., post-infectious syndromes show unstable adaptation. Common measurements of maximal strength (e.g., hand grip force) usually do not show a significant difference between patients and controls (46, 47). Two studies revealed a significantly reduced force in ME/CFS (48, 49). However, one did not describe sex effects. Females were overrepresented in ME/CFS group (96 vs. 62% in controls) (49), which might explain the lower strength. The findings are inconclusive and highlight that common maximal strength assessments might not be appropriate to investigate motor function in post-infectious states. We hypothesize AFisomax might be a decisive motor function to investigate and uncover clear differences between patients and controls. Moreover, AFisomax can react immediately to positive and negative inputs (43–45). A proposed neurophysiological explanation was given previously (42–45). Hence, the AF might be a useful biomarker to investigate patients and to determine helpful treatments, such as the individual PEMF.
This case report presents the positive clinical outcome for one Long COVID patient after a single treatment with individualized PEMF using the AF as biomarker.
2. Patient information
A 24-year-old female (168 cm, 65 kg; student since 2016; student assistant since 2020) presented herself in our practice of integrative medicine in August 2021. She reported a non-critical course of COVID-19 infection in December 2020 which lasted 2–3 weeks with symptoms as fever, loss of smell/taste, muscle pain and headache.
Afterwards she felt quite good for ~8 weeks. In March 2021 a state of Long COVID arose with severe symptoms as pronounced fatigue, fast exhaustion, post-exertional “crashs,” weakness, concentration problems, loss of speaking abilities, headache, muscle pain/cramps, sensitivity to stimuli (light/noise) and loss of smell. Less pronounced were nausea, nerve tingling, visual disturbances, memory, and sleeping problems and heavy perspiration. She was not able to proceed her Bachelor thesis, work as assistant or participate in social life. She appeared to be emotionally strong with good family bonding, although she naturally perceived her condition as very burdensome, especially because of the prospect of the clinicians she had to be patient, wait and pace herself.
She had a borreliosis infection in 2016. No other pre-existing health issues were reported (infections/hormonal/digestive/psychological). She always was sportive but sometimes not able to climb stairs in the current condition.
She already received exercise and physiotherapy, reflective breathing massage, tried supplements/vitamins and melatonin pills for sleeping problems. None of them led to a considerable condition improvement. Pacing herself resulted in a state in which she partly could resume work/studies. However, as soon as she went beyond her (low) limits (physically/cognitive/emotionally), a crash resulted (recovery: few days).
3. Clinical findings
The intensity of common Long COVID symptoms was inquired on a numerical scale [0-no to 10-very strong; according to Liu and Duricka (4)] retrospectively for pre-COVID baseline, during Long COVID (post-COVID) as well as 1-day, 4-weeks, and ~6-month post-treatment (Figure 1). Fatigue, memory/concentration issues, headache, muscle pain, loss of smell/taste, depression/anxiety, dizziness, and post-exertion malaise were rated by ≥ 9 post-COVID.
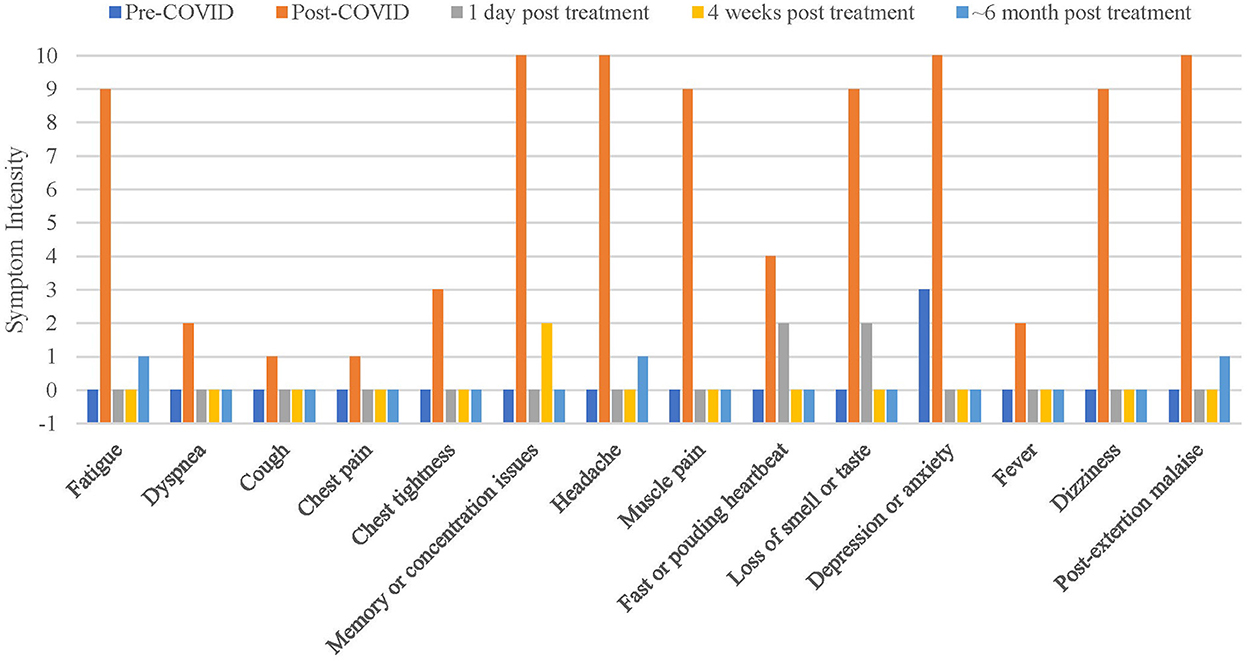
Figure 1. Intensity of Long COVID symptoms over time. Data was collected retrospectively for pre-COVID. Y-axis was set to −1 to visualize a score of zero [visualization was chosen following Liu and Duricka (4)].
For physical examination, the AF of nine different muscles/muscle groups was assessed on both sides by the MMT [hip flexors/adductors/abductors/extensors, foot dorsiflexors, pectoralis major (sternal and clavicular part), deltoid, and elbow flexors]. For left elbow/hip flexors, the AF was objectified (see below, Figure 2). All tested muscles showed a clearly unstable behavior in MMTs pre-treatment.
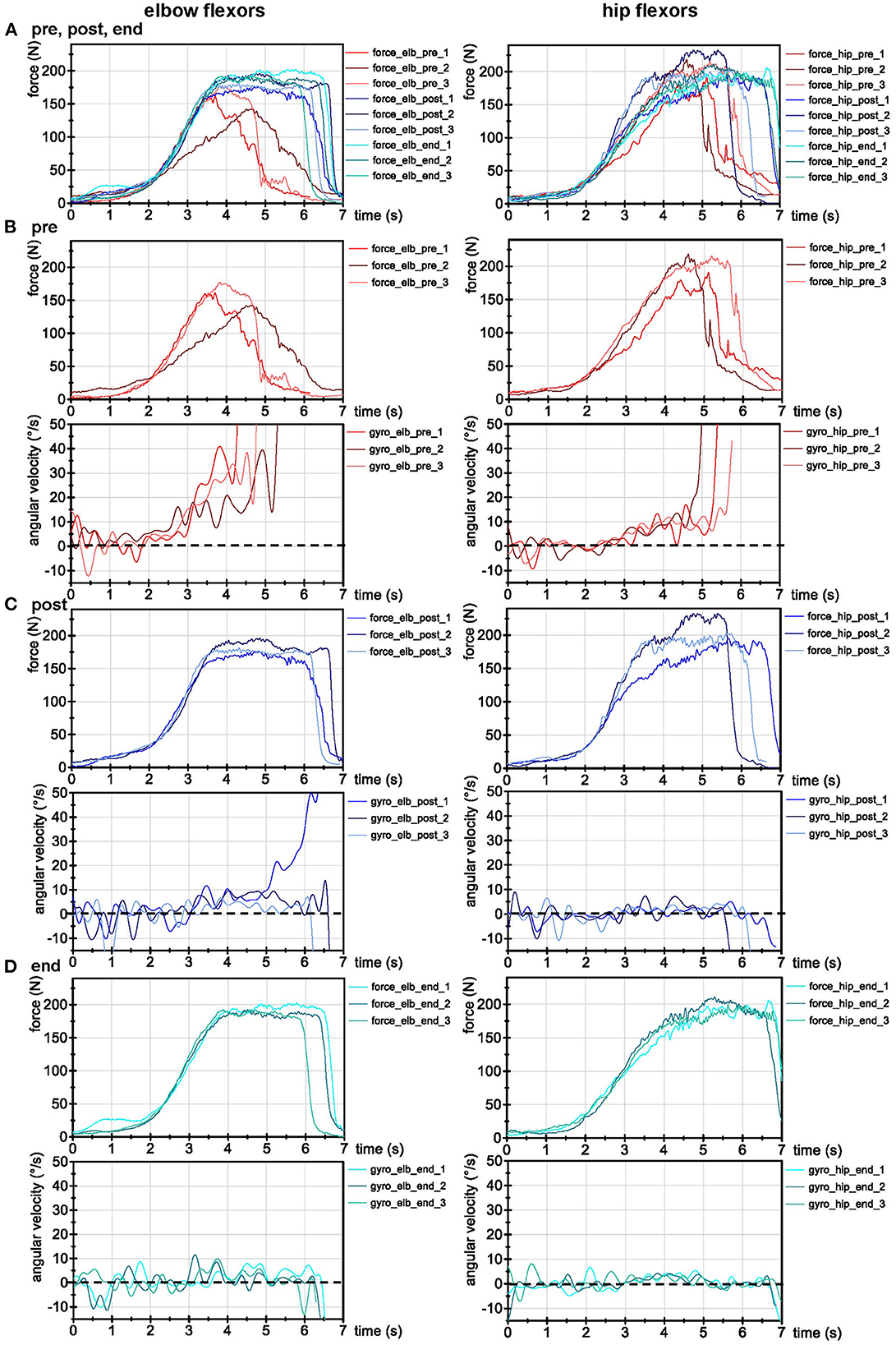
Figure 2. AF recordings of left elbow and hip flexors. (A) Force (N) of all trials before (pre), directly after (post) and 4 weeks after treatment (end). (B) Force (N) and angular velocity (°/s) of AF recordings pre-treatment, (C) directly post-treatment, and (D) 4-weeks post-treatment (end). All signals were filtered (butterworth; force: filter degree 5, cut-off frequency: 20 Hz; angular velocity: filter degree: 10, cut-off: 3 Hz). Dotted lines indicate zero for angular velocity.
4. Timeline
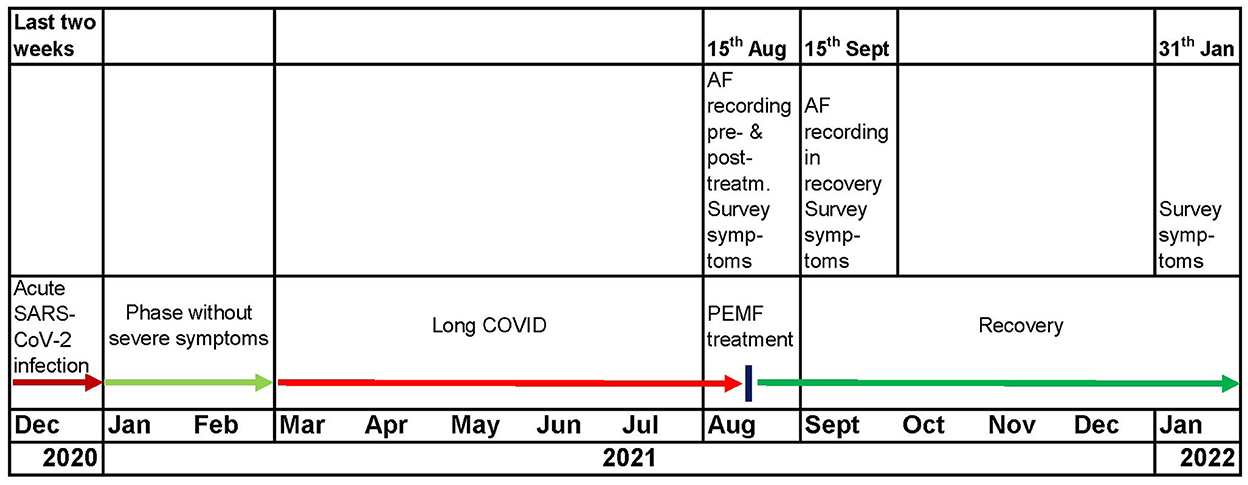
Figure 3. Timeline from acute SARS-CoV-infection over the ~6-month Long COVID period until the individualized PEMF treatment resulting into sustained recovery (~6-month post-treatment).
5. Diagnostic assessment
Diagnostic challenges for Long COVID appear because diagnosis is currently based on exclusion (15, 50). The patient provided documentation of a received extensive diagnostic assessment from a medical clinic (diagnosis: Long COVID). All other possible causes were excluded therein.
Besides the symptom intensity at five timepoints (Figure 1), the AF of left elbow and hip flexors was objectified by a handheld device which records reaction force (N) between tester and patient as well as limb position [angular velocity (°/s)]. It consists of strain gauges (co. Sourcing map, model: a14071900ux0076, precision: 1.0 ± 0.1%, sensitivity: 0.3 mV/V) and kinematic sensor technology (Bosch BNO055, 9-axis absolute orientation sensor, sensitivity: ± 1%) (42–45). Data were AD converted, buffered (sampling rate: 180 Hz) and sent (Bluetooth 5.0) to a tablet with measuring software (sticky notes). Data processing and evaluation were performed according to Schaefer et al. (43–45) in NI DIAdem 20.0 (National Instruments, Austin, TX, USA). Signals were interpolated (1 kHz) and filtered (Butterworth, filter degree 5, cut-off frequency 20 Hz). For visualization (Figure 2) the angular velocity was additionally filtered (degree: 3, cut-off: 10 Hz) to smoothen the oscillations (note: this leads to slightly different results between visual inspection in Figure 2 and results given below).
The following parameters were extracted: (1) AFmax (N): peak value of the whole trial. This can be reached either during isometric or eccentric muscle action. (2) AFisomax (N): the maximal isometric AF refers to the highest force under isometric conditions. This was defined as the force at the moment in which the gyrometer signal increased above zero, indicating a yielding of the limb (breaking point). In case the gyrometer signal oscillated ~0 during the entire trial, AFmax = AFisomax. (3) Slope: the slope of force rise before AFisomax of all trials was calculated by the difference quotient to control the increase. Reference points (time, force) were 70% and 100% of averaged AFisomax of all as unstable assessed MMTs. The decadic logarithm was taken from values [lg(N/s)] since force rise is exponential. Arithmetic means (M) and standard deviations (SD) of each parameter were calculated of the three trials for each muscle and timepoint (Table 1).
Figure 2 shows the signals of the three trials of left elbow/hip flexors at each timepoint (pre, post, and end), Table 1 shows the respective values. The entry MMTs were clearly unstable, indicated by low AFisomax ≈ ~71 N (elbow) and ~62 N (hip). The muscle started to lengthen at ~44 ± 25% of AFmax (elbow) and ~28 ± 6% (hip). The slope was slightly smoother for pre vs. post vs. end (Table 1). Thus, the conditions for adaptation should have been even better in pre-tests.
After initial AF assessment, we tested the individual supportive PEMF frequency. For that, we placed the coil anteriorly centered to the area of stellate ganglion (C7/T1) and performed the MMT repeatedly whereby before each test we adjusted the frequency. As soon as the muscle showed stability, we used this frequency for treatment. The stabilized holding capacity indicates that exactly this configuration is supportive for the patient's system. Hence, the motor output leads us to the helpful PEMF frequency by instantaneously gaining stability. The PEMF has a reach of ~20 cm and, therefore, it had no special lateral effect.
Immediately after PEMF application (see below), all muscles were clearly stable in MMT. Results of AF values are given (Figure 2C, Table 1). The first trial of elbow flexors was not fully stable, indicated by a deviation of gyrometer signal above zero. However, the breaking point (AFisomax) was on a high force (174 N ≈ 99% of AFmax). All other trials post-treatment showed full stability with high AFmax reached during isometric conditions [M ± SD: = 99.6 ± 0.7% (elbow); 100 ± 0% (hip)]. The isometric holding capacity was immediately increased by 2.6 (elbow) and 3.4-fold (hip) force compared to pre-treatment. The patient was able to maintain the isometric position of muscles during the entire force increase in contrast to pre-state. Those results support the manually assessed motor function as immediate reaction to the individual PEMF therapy.
6. Therapeutic intervention
Individualized PEMF therapy using bioMATRIX driver (Roland Pechan GmbH & Co.KG; sinusoidal signal, 100–1,000 Hz, max. 3 mT) was applied via coil to the area of C7/T1 assuming that it affects the stellate ganglion in order to “reboot” the ANS in the sense of a non-invasive neural therapy. The individual PEMF frequency of 550 Hz (flux density 1 mT) was tested by the AF and was applied for ~15 min. Established PEMF devices work with up to 10 mT (51). Only one treatment was performed since the condition improved immediately.
7. Follow-up and outcomes
The symptoms intensity improved immediately 1-day post-treatment and sustained until now (6-month post-treatment; Figure 1). The day after treatment she gave feedback (e-mail; translated): “I woke up this morning for the first time since months without a feeling of hangover. I don't have headache; my head feels broad and open (…). An incredible feeling. I don't have any nausea, I feel as 1,000 kg burden were removed from my body. I feel totally easy and energetic. I had no problems to fall asleep yesterday and slept through without melatonin pills. This morning I got out of bed without any difficulties, directly felt like doing Yoga and went for a bicycle trip.” She also felt like having “drunk 10 cups of coffee. I don't know where to go with my energy. It almost feels uncomfortable since my body is so twitchy.” It appears that the treatment led to sympathetic hyper activation. However, this adverse unanticipated reaction dissolved the next day.
Two weeks post-treatment she reported she still feels physically and mentally healthy. She was able to exercise as intensive as before COVID-infection (85 km bicycle trip without problems), she had no concentration issues and meetings with several persons were no problem anymore. “I am grateful and happy to have my life back.”
At follow-up appointment 4-weeks post-treatment, she felt well and healthy (Figure 1). All above-mentioned muscles showed stability in MMT, supported by AF recordings (Figure 2D, Table 1). The patient was able to stabilize the muscles in isometric holding conditions despite of the external increase until a considerably high AFisomax = AFmax = 195.7 ± 5.7 N (elbow) and 205.6 ± 5.7 N (hip).
Approximately 6-weeks post-treatment she received a lymph drainage (head and shoulder girdle) independent of our intervention and reported of headache, fatigue, concentration problems and sensitivity to stimuli afterwards for 3 days. She had another appointment in our practice ~1 week later. The muscles were still stable in entry MMTs. They became unstable after lymph drainage performed in our practice indicating that it irritated her system. By applying an individually newly tested PEMF frequency (590 Hz) the muscles were stabilized again. After the next lymph drainage independent of our intervention, she perceived headache for 1 hour but felt well afterwards. Approximately 10-weeks post-treatment she reported “I feel currently wonderful”. The sustainability was underpinned by the last assessment (January 2022; Figure 1). She reported, she is physically completely on the level before COVID, “if not better.” However, after emotional stress fatigue sometimes returns, but not in the previous extent.
8. Discussion
This case report suggests that low-frequency PEMF to the area of stellate ganglion with individually tested frequency using the AF might be an effective therapy in Long COVID patients. Since Liu and Duricka found a similar outcome after SGB (4), we assume that PEMF to the area C7/T1 affect the stellate ganglion. Based on our case and their suggestion that “cervical sympathetic chain activity can be blocked with local anesthetic, allowing the regional autonomic nervous system to ‘reboot”' (4), we propose the same effect might be gained by individualized PEMF therapy. A rationale for the mechanisms behind the hypothesis rebooting the ANS was given by Liu and Duricka (4).
The benefit of PEMF is that it is non-invasive, the patient does not feel anything of the intervention (see below) and no side effects are known (36, 39, 52). However, a successful treatment will not be that easy in every Long COVID patient. Some will have more severe pre-existing health issues which might hinder the positive outcome of a single treatment. From our current experience, three main factors in Long COVID occur: dysautonomia, pre-existing, and/or current mental stress and previous infections affecting the lymphatic system, which might lead to lymphatic entrapments post-COVID. Based on psychoneuroimmunology it is known that those factors interact (53). This is underpinned by the present case, since the patient relapsed after lymph drainage but could be switched back by one re-treatment. The switching between both states as immediate responses to disturbing or helping interventions speaks for a regulative character of Long COVID condition, at least in part. This would explain the instant reversibility observed in some cases. It is suggested that the complex psychoneuroimmunological network might still be vulnerable after “rebooting” the ANS. Lymphatic and mental stress might impede an immediate positive outcome or lead to a relapse. Consequently, such conditions must be treated, too. However, the ANS dysfunction—presumably triggered by SARS-CoV-2 infection—could benefit from individualized PEMF therapy determined by the holding capacity (AFisomax) of the neuromuscular system.
AFisomax was suggested to be especially sensitive regarding interfering inputs entering the complex motor control processes. At least the thalamus, cerebellum, inferior olivary nucleus, red nucleus, basal ganglia, cingulate cortex, and the sensorimotor cortex are involved in processing adaptive motor control (54–95). Due to the strong interconnections between those areas (73, 84) and since they also process other inputs (e.g., emotions/nociception) (63, 65, 68, 69, 73, 96–99), it was proposed that the motor output in the sense of AFisomax can be modified by different stimuli—positive and negative ones. The pro-inflammatory cytokine/chemokine profile (100), organ damage, lymphatic stress and/or the dysautonomia in Long COVID might impair that motor function. In case this is based on malfunction, it can be resolved immediately by applying the helpful therapy, e.g., individualized PEMF. The instantaneous improvement of AFisomax by 2.6 and 3.4-fold by applying the individualized PEMF frequency clearly demonstrated this. This effect cannot be reached by training. It must be the result of a functional readjustment of the patient's system. In contrast to maximal forces (as AFmax or MVIC), which can be reached also in dysfunctional state [as found here or in other studies (46, 47)], the holding capacity might uncover the dysfunction. In case the patient must adapt in an isometric holding manner to an increasing external force, the maximal force cannot be demanded under isometric conditions anymore. The adjustment of tension under stable muscle length fails and the limb gives way on significantly low forces. The AFisomax improved though immediately by applying the helpful PEMF. As was postulated by Mert (35), there is no rationale which PEMF parameters should be applied. So, why not “ask” the patient's system? The holding capacity seems to lead the way to the individual helpful parameters. Applying any frequency would not have this positive effect. Therefore, it is necessary to test the PEMF frequency individually by adequate biomarkers, as the neuromuscular holding capacity.
9. Conclusion
In conclusion, we suggest (1) to include pre-existing health issues of Long COVID individuals, especially concerning mental stress and previous infections and to examine the lymphatic system regarding flow restrictions. (2) The AF provides a valuable biomarker which can be used as functional diagnostic parameter for patients in post-infectious states, to determine the individual appropriate cause-related therapy and to monitor follow-up, since it seems to correlate with the patient's condition. (3) Soft, low-frequency PEMF with an individually tested frequency for each patient at the actual timepoint seems to be useful to “reboot” the dysfunctional ANS and might be an alternative non-invasive neural therapy. Further research is needed to verify and pursue this approach.
Data availability statement
The original contributions presented in the study are included in the article/supplementary material, further inquiries can be directed to the corresponding author.
Ethics statement
Ethical review and approval was not required for the study on human participants in accordance with the local legislation and institutional requirements. The patients/participants provided their written informed consent to participate in this study. The patient gave written informed consent to publish her case.
Author contributions
FB performed the measurements. LS analyzed the data and wrote the manuscript. All authors planned and designed the treatment process and measurements of the patient. All authors critically revised the manuscript and approved it for publication.
Funding
The publication of this article was funded by the Deutsche Forschungsgemeinschaft (DFG, German Research Foundation) - Project number 491466077.
Conflict of interest
The authors declare that the research was conducted in the absence of any commercial or financial relationships that could be construed as a potential conflict of interest.
Publisher's note
All claims expressed in this article are solely those of the authors and do not necessarily represent those of their affiliated organizations, or those of the publisher, the editors and the reviewers. Any product that may be evaluated in this article, or claim that may be made by its manufacturer, is not guaranteed or endorsed by the publisher.
References
1. Taquet M, Dercon Q, Luciano S, Geddes JR, Husain M, Harrison PJ. Incidence, co-occurrence, and evolution of long-COVID features: A 6-month retrospective cohort study of 273,618 survivors of COVID-19. PLoS Med. (2021) 18:e1003773. doi: 10.1371/journal.pmed.1003773
2. Huang L, Yao Q, Gu X, Wang Q, Ren L, Wang Y, et al. 1-year outcomes in hospital survivors with COVID-19: A longitudinal cohort study. Lancet. (2021) 398:747–58. doi: 10.1016/S0140-6736(21)01755-4
3. Solve ME/CFS Initiative,. Long Covid Alliance (LCA). (2021). Available online at: https://solvecfs.org/long-covid-alliance/
4. Liu LD, Duricka DL. Stellate ganglion block reduces symptoms of Long COVID: A case series. J Neuroimmunol. (2022) 362:577784. doi: 10.1016/j.jneuroim.2021.577784
5. Jason LA, Islam M, Conroy K, Cotler J, Torres C, Johnson M, et al. COVID-19 symptoms over time: comparing long-haulers to ME/CFS. Fatigue Biomed Health Behav. (2021) 9:59–68. doi: 10.1080/21641846.2021.1922140
6. Mantovani E, Mariotto S, Gabbiani D, Dorelli G, Bozzetti S, Federico A, et al. Chronic fatigue syndrome: An emerging sequela in COVID-19 survivors? J Neurovirol. (2021) 27:631–7. doi: 10.1007/s13365-021-01002-x
7. Wirth KJ, Scheibenbogen C, Paul F. An attempt to explain the neurological symptoms of Myalgic Encephalomyelitis/Chronic Fatigue Syndrome. J Transl Med. (2021) 19:471. doi: 10.1186/s12967-021-03143-3
8. Petracek LS, Suskauer SJ, Vickers RF, Patel NR, Violand RL, Swope RL, et al. Adolescent and young adult ME/CFS after confirmed or probable COVID-19. Front Med. (2021) 8:668944. doi: 10.3389/fmed.2021.668944
9. Wirth KJ, Scheibenbogen C. Pathophysiology of skeletal muscle disturbances in myalgic encephalomyelitis/chronic fatigue syndrome (ME/CFS). J Transl Med. (2021) 19:162. doi: 10.1186/s12967-021-02833-2
10. Hickie I, Davenport T, Wakefield D, Vollmer-Conna U, Cameron R, Vernon SD, et al. Post-infective and chronic fatigue syndromes precipitated by viral and non-viral pathogens: Prospective cohort study. Br Med J. (2006) 333:575. doi: 10.1136/bmj.38933.585764.AE
11. Shikova E, Reshkova V, Kumanova A, Raleva S, Alexandrova D, Capo N, et al. Cytomegalovirus, Epstein-Barr virus, and human herpesvirus-6 infections in patients with myalgic encephalomyelitis/chronic fatigue syndrome. J Med Virol. (2020) 92:3682–8. doi: 10.1002/jmv.25744
12. Estévez-López F, Mudie K, Wang-Steverding X, Bakken IJ, Ivanovs A, Castro-Marrero J, et al. Systematic review of the epidemiological burden of myalgic encephalomyelitis/chronic fatigue syndrome across Europe: Current evidence and EUROMENE research recommendations for epidemiology. J Clin Med. (2020) 9:1557. doi: 10.3390/jcm9051557
13. Morris G, Maes M, Berk M, Puri BK. Myalgic encephalomyelitis or chronic fatigue syndrome: how could the illness develop? Metab Brain Dis. (2019) 34:385–415. doi: 10.1007/s11011-019-0388-6
14. Augustin M, Schommers P, Stecher M, Dewald F, Gieselmann L, Gruell H, et al. Post-COVID syndrome in non-hospitalised patients with COVID-19: a longitudinal prospective cohort study. Lancet Reg Health Eur. (2021) 6:100122. doi: 10.1016/j.lanepe.2021.100122
15. Sisó-Almirall A, Brito-Zerón P, Ferrín LC, Kostov B, Moreno AM, Mestres J, et al. Long COVID-19: Proposed primary care clinical guidelines for diagnosis and disease management. Int J Environ Res Public Health. (2021) 18:4350. doi: 10.3390/ijerph18084350
16. Weinschenk S. Neural therapy—A review of the therapeutic use of local anesthetics. Acupunct Relat Ther. (2012) 1:5–9. doi: 10.1016/j.arthe.2012.12.004
17. Mak AKY, Hu Z, Zhang JX, Xiao Z, Lee TMC. Neural correlates of regulation of positive and negative emotions: An fMRI study. Neurosci Lett. (2009) 457:101–6. doi: 10.1016/j.neulet.2009.03.094
18. Pfister M, Fischer L. Die Behandlung des komplexen regionalen Schmerzsyndroms der oberen Extremität mit wiederholter Lokalanästhesie des Ganglion stellatum. Praxis. (2009) 98:247–57. doi: 10.1024/1661-8157.98.5.247
19. van Eijs F, Stanton-Hicks M, Van Zundert J, Faber CG, Lubenow TR, Mekhail N, et al. 16. Complex Regional Pain Syndrome: 16 CRPS. Pain Pract. (2011) 11:70–87. doi: 10.1111/j.1533-2500.2010.00388.x
20. Hey M, Wilson I, Johnson MI. Stellate ganglion blockade (SGB) for refractory index finger pain – A case report. Ann Phys Rehabil Med. (2011) 54:181–8. doi: 10.1016/j.rehab.2011.03.001
21. Narouze S. Ultrasound-guided stellate ganglion block: Safety and efficacy. Curr Pain Headache Rep. (2014) 18:424. doi: 10.1007/s11916-014-0424-5
22. Lipov E, Navaie M, Stedje-Larsen ET, Burkhardt K, Smith JC, Sharghi LH, et al. A novel application of stellate ganglion block: Preliminary observations for the treatment of post-traumatic stress disorder. Mil Med. (2012) 177:125–7. doi: 10.7205/MILMED-D-11-00328
23. Mulvaney SW, Lynch JH, Hickey MJ, Rahman-Rawlins T, Schroeder M, Kane S, et al. Stellate ganglion block used to treat symptoms associated with combat-related post-traumatic stress disorder: A case series of 166 patients. Mil Med. (2014) 179:1133–40. doi: 10.7205/MILMED-D-14-00151
24. Yokoyama M, Nakatsuka H, Itano Y, Hirakawa M. Stellate ganglion block modifies the distribution of lymphocyte subsets and natural-killer cell activity. Anesthesiology. (2000) 92:109–109. doi: 10.1097/00000542-200001000-00021
25. Tian Y, Wittwer ED, Kapa S, McLeod CJ, Xiao P, Noseworthy PA, et al. Effective use of percutaneous stellate ganglion blockade in patients with electrical storm. Circ Arrhythm Electrophysiol. (2019) 12:7118. doi: 10.1161/CIRCEP.118.007118
26. Brobyn TL. Neural therapy: An overlooked game changer for patients suffering chronic pain? J Pain Relief. (2015) 4:184. doi: 10.4172/2167-0846.1000184
27. Wang S, Zhou X, Huang B, Wang Z, Zhou L, Wang M, et al. Noninvasive low-frequency electromagnetic stimulation of the center stellate ganglion reduces myocardial infarction-induced ventricular arrhythmia. Sci Rep. (2016) 6:30783. doi: 10.1038/srep30783
28. Krayukhina K, Yu A, Lobkaeva EP, Devyatkova NS. Effect of low-frequency pulsatile AC magnetic field on the state of the autonomic nervous system in rats. Biophysics. (2010) 55:637–41. doi: 10.1134/S0006350910040226
29. Kholodov K, Yu A. The Effect of Electromagnetic and Magnetic Fields on the Central Nervous System. NASA Technical Translation, TTF-465. Washington, DC: National Aeronautics and Space Administration (1967).
30. Scherlag BJ, Yamanashi WS, Hou Y, Jacobson JI, Jackman WM, Lazzara R. Magnetism and cardiac arrhythmias. Cardiol Rev. (2004) 12:85–96. doi: 10.1097/01.crd.0000094029.10223.2f
31. Yu L, Dyer JW, Scherlag BJ, Stavrakis S, Sha Y, Sheng X, et al. The use of low-level electromagnetic fields to suppress atrial fibrillation. Heart Rhythm. (2015) 12:809–17. doi: 10.1016/j.hrthm.2014.12.022
32. Bolotova NV, Raigorodsky YM, Dronova EG, Posokhova NV. The use of magnetic sympathocor-rection for the treatment of vegetative disorders in the children with obesity. Russ J Physiother Balneology Rehabil. (2013) 12:30–4. Available online at: https://rjpbr.com/1681-3456/article/view/41416
33. Yermishev OV. The levels of functional-vegetative homeostasis as criteria for magnetotherapy efficacy. Int J Med Med Res. (2018) 2018:8902. doi: 10.11603/ijmmr.2413-6077.2018.1.8902
34. Shupak NM, Prato FS, Thomas AW. Therapeutic uses of pulsed magnetic-field exposure: A review. URSI Radio Sci Bull. (2003) 307:9–32. doi: 10.23919/URSIRSB.2003.7909506
35. Mert T. Pulsed magnetic field treatment as antineuropathic pain therapy. Rev Neurosci. (2017) 28:751–8. doi: 10.1515/revneuro-2017-0003
36. Strauch B, Herman C, Dabb R, Ignarro LJ, Pilla AA. Evidence-based use of pulsed electromagnetic field therapy in clinical plastic surgery. Aesthet Surg J. (2009) 29:135–43. doi: 10.1016/j.asj.2009.02.001
37. Nelson FR, Zvirbulis R, Pilla AA. Non-invasive electromagnetic field therapy produces rapid and substantial pain reduction in early knee osteoarthritis: A randomized double-blind pilot study. Rheumatol Int. (2013) 33:2169–73. doi: 10.1007/s00296-012-2366-8
38. Lee JW, Kim JY, Hyun JH, Lee YH. Analysis of HRV and body temperature variation for manual acupuncture and PEMF (Pulsed Electro-Magnetic Field) acupuncture stimulation. Acupunct Electrother Res. (2021) 47:91–9. doi: 10.3727/036012921X16287835103390
39. Vadalà M, Morales-Medina JC, Vallelunga A, Palmieri B, Laurino C, Iannitti T. Mechanisms and therapeutic effectiveness of pulsed electromagnetic field therapy in oncology. Cancer Med. (2016) 5:3128–39. doi: 10.1002/cam4.861
40. Yamaguchi S, Ogiue-Ikeda M, Sekino M, Ueno S. Effects of pulsed magnetic stimulation on tumor development and immune functions in mice. Bioelectromagnetics. (2006) 27:64–72. doi: 10.1002/bem.20177
41. Barbault A, Costa FP, Bottger B, Munden RF, Bomholt F, Kuster N, et al. Amplitude-modulated electromagnetic fields for the treatment of cancer: Discovery of tumor-specific frequencies and assessment of a novel therapeutic approach. J Exp Clin Cancer Res. (2009) 28:51. doi: 10.1186/1756-9966-28-51
42. Bittmann FN, Dech S, Aehle M, Schaefer LV. Manual muscle testing—Force profiles and their reproducibility. Diagnostics. (2020) 10:996. doi: 10.3390/diagnostics10120996
43. Schaefer LV, Dech S, Bittmann FN. Adaptive Force and emotionally related imaginations – preliminary results suggest a reduction of the maximal holding capacity as reaction to disgusting food imagination. Heliyon. (2021) 7:e07827. doi: 10.1016/j.heliyon.2021.e07827
44. Schaefer LV, Dech S, Aehle M, Bittmann FN. Disgusting odours affect the characteristics of the Adaptive Force in contrast to neutral and pleasant odours. Sci Rep. (2021) 11:16410. doi: 10.1038/s41598-021-95759-0
45. Schaefer LV, Dech S, Wolff LL, Bittmann FN. Emotional imagery influences the adaptive force in young women: Unpleasant imagery reduces instantaneously the muscular holding capacity. Brain Sci. (2022) 12:1318. doi: 10.3390/brainsci12101318
46. Lloyd AR, Hales JP, Gandevia SC. Muscle strength, endurance and recovery in the post-infection fatigue syndrome. J Neurol Neurosurg Psychiatry. (1988) 51:1316–22. doi: 10.1136/jnnp.51.10.1316
47. Rutherford OM, White PD. Human quadriceps strength and fatiguability in patients with post viral fatigue. J Neurol Neurosurg Psychiatry. (1991) 54:961–4. doi: 10.1136/jnnp.54.11.961
48. Jäkel B, Kedor C, Grabowski P, Wittke K, Thiel S, Scherbakov N, et al. Hand grip strength and fatigability: Correlation with clinical parameters and diagnostic suitability in ME/CFS. J Transl Med. (2021) 19:159. doi: 10.1186/s12967-021-02774-w
49. Meeus M, Ickmans K, Struyf F, Kos D, Lambrecht L, Willekens B, et al. What is in a name? Comparing diagnostic criteria for chronic fatigue syndrome with or without fibromyalgia. Clin Rheumatol. (2016) 35:191–203. doi: 10.1007/s10067-014-2793-x
50. NICE. COVID-19 Rapid Guideline: Managing the Long-Term Effects of COVID-19. (2022). p. 106. Available online at: https://www.nice.org.uk/guidance/ng188/resources/covid19-rapid-guideline-managing-the-longterm-effects-of-covid19-pdf-51035515742.
51. Quittan M. Magnetfeldtherapie—klinische Wirksamkeiten. Trauma Berufskrankh. (2004) 6:4. doi: 10.1007/s10039-003-0758-4
52. Hedén P, Pilla AA. Effects of pulsed electromagnetic fields on postoperative pain: A double-blind randomized pilot study in breast augmentation patients. Aesthetic Plast Surg. (2008) 32:660–6. doi: 10.1007/s00266-008-9169-z
53. Wright AGC, Aslinger EN, Bellamy B, Edershile EA, Woods WC. Psychoneuroimmunology of stress and mental health. In: KL Harkness, EP Hayden, editors, The Oxford Handbook of Stress and Mental Health. Oxford: Oxford University Press (2020). p. 26–44. doi: 10.1093/oxfordhb/9780190681777.013.2
54. Pflüger HJ, Sillar K. Motor control. In: Neurosciences - From Molecule to Behavior: A University Textbook. Berlin/?Heidelberg?: Springer. (2013). p. 479–524. doi: 10.1007/978-3-642-10769-6_23
55. Huggenberger S, Moser N, Schröder H, Cozzi B, Granato A, Merighi A. Neuroanatomie des Menschen: mit 202 größtenteils farbigen Abbildungen. Berlin/?Heidelberg?: Springer (2019). doi: 10.1007/978-3-662-56461-5
56. Albus JS. A theory of cerebellar function. Math Biosci. (1971) 10:25–61. doi: 10.1016/0025-5564(71)90051-4
57. Ashe J, Bushara K. The olivo-cerebellar system as a neural clock. In: H Merchant, V de Lafuente, editors, Neurobiology of Interval Timing. vol. 829. New York, NY: Springer. (2014). p. 155–65. doi: 10.1007/978-1-4939-1782-2_9
58. Lawrenson C, Bares M, Kamondi A, Kovács A, Lumb B, Apps R, et al. The mystery of the cerebellum: Clues from experimental and clinical observations. Cerebellum Ataxias. (2018) 5:8. doi: 10.1186/s40673-018-0087-9
59. Shadmehr R, Smith MA, Krakauer JW. Error correction, sensory prediction, and adaptation in motor control. Annu Rev Neurosci. (2010) 33:89–108. doi: 10.1146/annurev-neuro-060909-153135
60. Ivry RB. Exploring the role of the cerebellum in sensory anticipation and timing: Commentary on Tesche and Karhu. Hum Brain Mapp. (2000) 9:115–8. doi: 10.1002/(SICI)1097-0193(200003)9:3<115::AID-HBM1>3.0.CO;2-5
61. Bengtsson F, Ekerot CF, Jörntell H. In vivo analysis of inhibitory synaptic inputs and rebounds in deep cerebellar nuclear neurons. PLoS ONE. (2011) 6:e18822. doi: 10.1371/journal.pone.0018822
62. Lang EJ, Apps R, Bengtsson F, Cerminara NL, De Zeeuw CI, Ebner TJ, et al. The roles of the olivocerebellar pathway in motor learning and motor control. A consensus paper. Cerebellum. (2017) 16:230–52. doi: 10.1007/s12311-016-0787-8
63. Groenewegen HJ. The basal ganglia and motor control. Neural Plast. (2003) 10:107–20. doi: 10.1155/NP.2003.107
64. Jörntell H. Cerebellar physiology: Links between microcircuitry properties and sensorimotor functions: Cerebellar physiology. J Physiol. (2017) 595:11–27. doi: 10.1113/JP272769
65. Vogt BA, Finch DM, Olson CR. Functional heterogeneity in cingulate cortex: The anterior executive and posterior evaluative regions. Cereb Cortex. (1992) 2:435–43. doi: 10.1093/cercor/2.6.435-a
67. Galizia CG. Neurosciences—From Molecule to Behavior: A University Textbook. Berlin Heidelberg: Springer (2013).
68. Rothwell JC. Control of Human Voluntary Movement. Gaithersburg, MD: Aspen Publishers (1987). doi: 10.1007/978-1-4684-7688-0
69. Doya K. Complementary roles of basal ganglia and cerebellum in learning and motor control. Curr Opin Neurobiol. (2000) 10:732–9. doi: 10.1016/S0959-4388(00)00153-7
70. Wu T, Hallett M. The cerebellum in Parkinson's disease. Brain. (2013) 136:696–709. doi: 10.1093/brain/aws360
71. Caligiore D, Pezzulo G, Baldassarre G, Bostan AC, Strick PL, Doya K, et al. Consensus paper: Towards a systems-level view of cerebellar function: The interplay between cerebellum, basal ganglia, and cortex. Cerebellum. (2017) 16:203–29. doi: 10.1007/s12311-016-0763-3
72. Pelzer EA, Hintzen A, Goldau M, von Cramon DY, Timmermann L, Tittgemeyer M. Cerebellar networks with basal ganglia: Feasibility for tracking cerebello-pallidal and subthalamo-cerebellar projections in the human brain. Eur J Neurosci. (2013) 38:3106–14. doi: 10.1111/ejn.12314
73. Morecraft RJ, Tanjii J. Cingulofrontal interactions and the cingulate motor areas. In: Vogt BA, editor, Cingulate Neurobiology and Disease. Oxford; New York, NY: Oxford University Press (2009) pp. 113–144.
74. Gerfen CR, Wilson CJ. The basal ganglia. In: Swanson LW, Bjorklund A, Hokfelt T, editors. Handbook of Chemical Neuroanatomy, Vol. 12. Amsterdam: Elsevier (1996). p. 371–468. doi: 10.1016/S0924-8196(96)80004-2
75. Wise SP, Murray EA, Gerfen CR. The frontal cortex-basal ganglia system in primates. Crit Rev Neurobiol. (1996) 10:317–56. doi: 10.1615/CritRevNeurobiol.v10.i3-4.30
76. O'Halloran CJ, Kinsella GJ, Storey E. The cerebellum and neuropsychological functioning: A critical review. J Clin Exp Neuropsychol. (2012) 34:35–56. doi: 10.1080/13803395.2011.614599
77. Schlerf JE, Galea JM, Bastian AJ, Celnik PA. Dynamic modulation of cerebellar excitability for abrupt, but not gradual, visuomotor adaptation. J Neurosci. (2012) 32:11610–7. doi: 10.1523/JNEUROSCI.1609-12.2012
78. Jueptner M, Ottinger S, Fellows SJ, Adamschewski J, Flerich L, Müller SP, et al. The relevance of sensory input for the cerebellar control of movements. Neuroimage. (1997) 5:41–8. doi: 10.1006/nimg.1996.0249
79. Welsh JP, Lang EJ, Suglhara I, Llinás R. Dynamic organization of motor control within the olivocerebellar system. Nature. (1995) 374:453–7. doi: 10.1038/374453a0
80. Vitek JL, Ashe J, DeLong MR, Alexander GE. Physiologic properties and somatotopic organization of the primate motor thalamus. J Neurophysiol. (1994) 71:1498–513. doi: 10.1152/jn.1994.71.4.1498
82. Haber SN, Calzavara R. The cortico-basal ganglia integrative network: The role of the thalamus. Brain Res Bull. (2009) 78:69–74. doi: 10.1016/j.brainresbull.2008.09.013
83. Sommer MA. The role of the thalamus in motor control. Curr Opin Neurobiol. (2003) 13:663–70. doi: 10.1016/j.conb.2003.10.014
84. Vogt BA, Nimchinsky EA, Vogt LJ, Hof PR. Human cingulate cortex: Surface features, flat maps, and cytoarchitecture. J Comp Neurol. (1995) 359:490–506. doi: 10.1002/cne.903590310
85. Pruszynski JA, Scott SH. Optimal feedback control and the long-latency stretch response. Exp Brain Res. (2012) 218:341–59. doi: 10.1007/s00221-012-3041-8
87. Bueti D, Walsh V, Frith C, Rees G. Different brain circuits underlie motor and perceptual representations of temporal intervals. J Cogn Neurosci. (2008) 20:204–14. doi: 10.1162/jocn.2008.20017
88. D'Angelo E. Physiology of the cerebellum. In: Manto M, Huisman TAGM, editors. Handbook of Clinical Neurology, Vol. 154. Amsterdam: Elsevier (2018). p. 85–108. doi: 10.1016/B978-0-444-63956-1.00006-0
89. Rao SM, Harrington DL, Haaland KY, Bobholz JA, Cox RW, Binder JR. Distributed neural systems underlying the timing of movements. J Neurosci. (1997) 17:5528–35. doi: 10.1523/JNEUROSCI.17-14-05528.1997
90. Lang EJ, Sugihara I, Welsh JP, Llinás R. Patterns of spontaneous purkinje cell complex spike activity in the awake rat. J Neurosci. (1999) 19:2728–39. doi: 10.1523/JNEUROSCI.19-07-02728.1999
91. Manning CD, Tolhurst SA, Bawa P. Proprioceptive reaction times and long-latency reflexes in humans. Exp Brain Res. (2012) 221:155–66. doi: 10.1007/s00221-012-3157-x
92. Gross J, Timmermann L, Kujala J, Dirks M, Schmitz F, Salmelin R, et al. The neural basis of intermittent motor control in humans. Proc Natl Acad Sci USA. (2002) 99:2299–302. doi: 10.1073/pnas.032682099
93. Bays PM, Wolpert DM. Computational principles of sensorimotor control that minimize uncertainty and variability: Computational principles of sensorimotor control. J Physiol. (2007) 578:387–96. doi: 10.1113/jphysiol.2006.120121
94. Johansson RS. How is grasping modified by somatosensory input? In: Humphrey DR, Freund HJ, editors. Motor Control: Concepts and Issues. New York, NY: John Wiley (1991). p. 331–55.
95. Todorov E. Optimality principles in sensorimotor control. Nat Neurosci. (2004) 7:907–15. doi: 10.1038/nn1309
96. Gire DH, Restrepo D, Sejnowski TJ, Greer C, De Carlos JA, Lopez-Mascaraque L. Temporal processing in the olfactory system: Can we see a smell? Neuron. (2013) 78:416–32. doi: 10.1016/j.neuron.2013.04.033
98. Kugelberg E, Eklund K, Grimby L. An electromyographic study of the nociceptive reflexes of the lower limb. Mechanism of the plantar responses. Brain. (1960) 83:394–410. doi: 10.1093/brain/83.3.394
99. Nijs J, Daenen L, Cras P, Struyf F, Roussel N, Oostendorp RAB. Nociception affects motor output: A review on sensory-motor interaction with focus on clinical implications. Clin J Pain. (2012) 28:175–81. doi: 10.1097/AJP.0b013e318225daf3
Keywords: individualized pulsed electromagnetic field, Adaptive Force, muscular holding capacity, case report, Long COVID, post-COVID syndrome, muscle weakness, fatigue
Citation: Schaefer LV and Bittmann FN (2023) Case report: Individualized pulsed electromagnetic field therapy in a Long COVID patient using the Adaptive Force as biomarker. Front. Med. 9:879971. doi: 10.3389/fmed.2022.879971
Received: 25 March 2022; Accepted: 22 December 2022;
Published: 11 January 2023.
Edited by:
Mohamed Emara, Qatar University, QatarReviewed by:
Luís Carlos Lopes-Júnior, Federal University of Espirito Santo, BrazilDeborah Duricka, Neuroversion Inc., United States
Copyright © 2023 Schaefer and Bittmann. This is an open-access article distributed under the terms of the Creative Commons Attribution License (CC BY). The use, distribution or reproduction in other forums is permitted, provided the original author(s) and the copyright owner(s) are credited and that the original publication in this journal is cited, in accordance with accepted academic practice. No use, distribution or reproduction is permitted which does not comply with these terms.
*Correspondence: Laura V. Schaefer, bHNjaGFlZmUmI3gwMDA0MDt1bmktcG90c2RhbS5kZQ==