- 1Institut für Anästhesiologische Pathophysiologie und Verfahrensentwicklung, Universitätsklinikum Ulm, Ulm, Germany
- 2Faculty of Science, York University, Toronto, ON, Canada
- 3Klinik für Anästhesiologie und Intensivmedizin, Universitätsklinikum Ulm, Ulm, Germany
Background: Sodium thiosulfate (STS) is a recognized drug with antioxidant and H2S releasing properties. We recently showed that STS attenuated organ dysfunction and injury during resuscitation from trauma-and-hemorrhage in CSE-ko mice, confirming its previously described organ-protective and anti-inflammatory properties. The role of H2S in diabetes mellitus type 1 (DMT1) is controversial: genetic DMT1 impairs H2S biosynthesis, which has been referred to contribute to endothelial dysfunction and cardiomyopathy. In contrast, development and severity of hyperglycemia in streptozotocin(STZ)-induced DMT1 was attenuated in CSE-ko mice. Therefore, we tested the hypothesis whether STS would also exert organ-protective effects in CSE-ko mice with STZ-induced DMT1, similar to our findings in animals without underlying co-morbidity.
Methods: Under short-term anesthesia with sevoflurane and analgesia with buprenorphine CSE-ko mice underwent DMT1-induction by single STZ injection (100 μg⋅g–1). Seven days later, animals underwent blast wave-induced blunt chest trauma and surgical instrumentation followed by 1 h of hemorrhagic shock (MAP 35 ± 5 mmHg). Resuscitation comprised re-transfusion of shed blood, lung-protective mechanical ventilation, fluid resuscitation and continuous i.v. norepinephrine together with either i.v. STS (0.45 mg⋅g–1) or vehicle (n = 9 in each group). Lung mechanics, hemodynamics, gas exchange, acid–base status, stable isotope-based metabolism, and visceral organ function were assessed. Blood and organs were collected for analysis of cytokines, chemokines, and immunoblotting.
Results: Diabetes mellitus type 1 was associated with more severe circulatory shock when compared to our previous study using the same experimental design in CSE-ko mice without co-morbidity. STS did not exert any beneficial therapeutic effect. Most of the parameters measured of the inflammatory response nor the tissue expression of marker proteins of the stress response were affected either.
Conclusion: In contrast to our previous findings in CSE-ko mice without underlying co-morbidity, STS did not exert any beneficial therapeutic effect in mice with STZ-induced DMT1, possibly due to DMT1-related more severe circulatory shock. This result highlights the translational importance of both integrating standard ICU procedures and investigating underlying co-morbidity in animal models of shock research.
Introduction
Sodium thiosulfate, Na2S2O3, (STS) is an H2S donor with minimal side effects and clinically approved for decades for the treatment of calciphylaxis, cis-Pt toxicity and cyanide poisoning (1). Along with its sulfide releasing properties it is a known antioxidant, and in various rodent models was shown to be organ-protective after ischemia reperfusion injury (2, 3), acute liver injury (4), endotoxemia (5, 6), and bacterial sepsis (7). However, none of these models integrated standard intensive care measures into the experimental design, and STS was mostly administered either before or simultaneously with the systemic challenge. Using a post-treatment approach in a long-term, large animal model of hemorrhage-and-resuscitation, we recently showed lung-protective properties (8) in “human-sized” swine with ubiquitous atherosclerosis and, hence, decreased expression of the H2S-producing enzyme cystathionine-γ-lyase (CSE) (9). We confirmed these beneficial effects of post-treatment STS administration under conditions of impaired endogenous H2S availability by attenuation of lung, liver and kidney injury in mice with genetic CSE deletion (CSE-ko) undergoing trauma-and-hemorrhage and subsequent intensive care-based resuscitation (10, 11).
Diabetes mellitus type 1 and 2 are disorders of glucose metabolism where the evidence points to the involvement of H2S and/or its endogenous producing enzymes (12, 13). In type 2 diabetes mellitus (i.e., diabetes due to insulin resistance), the endogenous availability of H2S or the exogenous administration play a special role with regard to the prevention and/or treatment of vascular complications of diabetes (14, 15). Type 2 diabetes mellitus per se significantly reduced CSE expression in the kidneys (16), while exogenous H2S improved insulin receptor sensitivity (17), reduced the hyperglycemia-induced oxidative stress and, subsequently, the activation of renin angiotensin system in the kidney (18). In addition, H2S administration prevented the progression of diabetic nephropathy (19, 20) and improved wound healing impaired by type 2 diabetes mellitus (21, 22). Inhibiting CSE, on the other hand, further worsened wound healing (21).
Limited data is only available regarding the importance of the H2S system in diabetes mellitus type 1 (DMT1), i.e., diabetes due to absolute insulin deficiency, and the little there is contradictory: While in CSE ko mice the development of diabetes mellitus type 1 induced by injection of streptozotocin (STZ) was both delayed (23) and attenuated, therapy with exogenous H2S prevented the development of diabetic nephropathy in a rat model of diabetes mellitus type 1 (24). Moreover, H2S treatment also attenuated STZ-induced diabetic retinopathy in rats (25).
Given the limited experimental evidence in diabetes mellitus type 1 (DMT1) and the fact that it may be an independent risk factor for mortality in critically ill surgical and trauma patients (26, 27), we therefore tested the hypothesis whether STS would also exert organ-protective effects in CSE-ko mice with STZ induced DMT1, similar to our recent findings in CSE-ko mice without underlying co-morbidity (10).
Materials and Methods
The study was approved by the University of Ulm Animal Care Committee and the Federal Authorities for Animal Research (Regierungspräsidium Tübingen, Baden-Württemberg, Germany, Reg.-Nr. 1387), and all experiments were performed in adherence with the National Institutes of Health Guidelines on the Use of Laboratory Animals and the European Union “Directive 2010/63 EU on the protection of animals used for scientific purposes.” The present experiments were part of a larger protocol including also wild type and diabetes type 2 animals. The federal authorities for animal protection, however, had only approved experiments in CSE-ko mice where the strongest effect of STS was expected. Thus, we were not allowed to include WT control mice in the present study as originally planned.
At the time of the experiment, a total of 23 homozygous (CSE-ko) deficient mice (C57BL/6J.129SvEv) (10) bred in house had been injected with STZ. Mice were 12–16 weeks old and weighed between 30 and 40 g. Five animals had to be excluded from the final data analysis due to hemothorax and pericardial tamponade subsequent to blunt chest trauma or uncontrollable bleeding during surgery. Thus, the subsequent data refer to 18 mice analyzed (vehicle group: n = 9; STS group: n = 9). This drop-out rate of 6/31 is similar to the previous study using the same model in CSE-ko mice without comorbidity (10) and is due to the extensive surgical instrumentation as well as the severe combined trauma injury mechanism of blunt chest trauma and hemorrhagic shock.
Figure 1 shows a flow chart of the experimental procedure. The procedures for anesthesia, blast wave-induced blunt chest trauma, surgical instrumentation, induction of hemorrhagic shock and subsequent resuscitation as well as the methods for immunoblotting, assessment of metabolism via stable isotope approach are only briefly outlined as they are identical to that in CSE-ko mice without comorbidity (10).
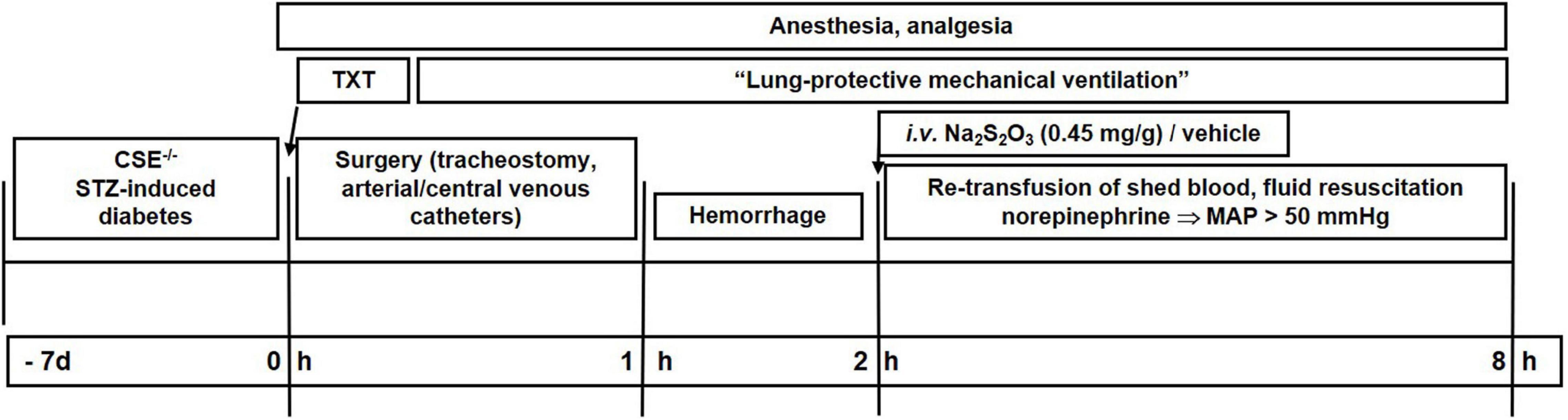
Figure 1. Flow chart of the experimental procedure. Txt, thoraxtrauma; MAP, mean arterial pressure; HR, heart rate, STZ, streptozotocin.
Induction of Diabetes Type1
To develop a DMT1 state, streptozotocin (STZ) administration was used, as it is a common method to induce DMT1 in mice (28–30). STZ is an antimicrobial and a chemotherapeutic alkylating agent that causes specific necrosis of the pancreatic β-cells. Female mice show a significantly lower sensitivity against STZ induced diabetes than male mice (30), so only male CSE-ko mice received a single intraperitoneal (IP) injection of 100 μg⋅g–1 streptozotocin 7 days before the experiment under short term inhalational anesthesia with sevoflurane and subcutaneous (s.c.) injection of 1.5 μg⋅g–1 buprenorphine as analgesia. This “single moderate-dose injection” model was used, because according to the literature it is associated with the least mortality and at the same time progressive hyperglycemia within a week post-STZ injection (30, 31).
Anesthesia, Blunt Chest Trauma, Surgical Instrumentation, and Hemorrhagic Shock
Briefly, mice were anesthetized, received a single blast wave-generated blunt chest trauma and subsequently underwent surgical instrumentation comprising tracheostomy, and placement of catheters in the jugular vein, in the carotid and the femoral artery and in the urinary bladder. After completion of surgical instrumentation, mice underwent 1 h of hemorrhagic shock by removal of 30 μL⋅g–1 of blood and titration of mean arterial pressure (MAP) to 35 ± 5 mmHg. After 1 h, resuscitation was started with the re-transfusion of shed blood, administration of hydroxyethyl starch and – if necessary – norepinephrine to maintain a MAP of 50 mmHg. Immediately upon initiation of resuscitation mice randomly received either a bolus injection of 0.45 mg⋅g–1 sodium thiosulfate [Na2S2O3, STS (Dr. Franz Köhler Chemie GmbH, Bensheim, Germany)] or the same amount of vehicle solution (NaCl 0.9%). After 6 h of lung-protective mechanical ventilation and intensive care (or if MAP could no longer be maintained >50 mmHg despite maximum norepinephrine infusion rates (i.e., ≤1 μg⋅g–1.min–1), mice were exsanguinated and blood and tissue samples were collected for further analysis (10).
Parameters of Lung Mechanics, Hemodynamics, Gas Exchange, and Acid-Base Status
Core temperature, hemodynamics and lung mechanics (10) were recorded hourly. Arterial blood samples were taken at baseline (i.e., immediately after insertion of the arterial catheter), at 3 h of resuscitation, and at the end of the experiment.
Metabolism and Organ Function
Arterial lactate and glucose levels were measured at baseline (i.e., immediately after insertion of the arterial catheter) and at the end of the experiment. Together with urine output, gas chromatography/mass spectrometry (GC/MS) measurement of plasma und urinary creatinine concentrations using 2H3-creatinine as internal standard allowed for calculation of creatinine clearance (10, 32). Endogenous glucose production and direct, aerobic glucose oxidation as well as the production rates of urea, glycerol, and leucine as markers of hepatic metabolic capacity, lipolysis, and protein degradation (10, 32), were derived from the measurement of the respective plasma isotope enrichment during continuous intravenous isotope infusion as described in detail previously (10, 32).
Cytokines and Chemokines
As described previously (10) cytokine and chemokine levels in lung and kidney tissue as well as plasma were determined by using a mouse multiplex cytokine kit (Bio-Plex Pro Cytokine Assay, Bio-Rad, Hercules, CA, United States) according to the manufacturers’ instructions.
Immunoblots
As described previously (10), expression of lung and kidney heme oxygenase-1 (HO-1; Abcam, Cambridge, United Kingdom), the inducible isoform of the nitric oxide synthase (iNOS; Thermo Fisher Scientific, Rockford, IL, United States), the endogenous inhibitor of the nuclear transcription factor NF-kB alpha (IκBα; Cell Signaling, Danvers, MA, United States), the second major H2S-producing enzyme cystathionine-β-synthase (CBS) (Signaling, Danvers, MA, United States caspase-3 (Signaling, Danvers, MA, United States), and the glucocorticoid receptor (GR; Cell Signaling, Danvers, MA, United States) were assessed by immunoblotting.
Statistical Analysis
All data are presented as medians and quartiles unless otherwise stated. Intergroup differences were analyzed using the Mann-Whitney rank sum test. A p-value of <0.05 was considered statistically significant. Survival curves were compared using the log-rank (Mantel Cox) test. Sample sizes were based on our previous experiments. A statistical power analysis using creatinine clearance (as the marker of acute kidney injury) and the norepinephrine infusion rate (as the marker of the severity of circulatory shock) as main criteria yielded a minimum number of 11 – 14 animals for two experimental groups based on two-sided testing, α = 0.05, power of 80%, and non-parametric analysis of variance. Since an interim analysis at n = 9 had not yielded any significant intergroup difference for the main criteria, the experiment was terminated due to a futile result and in order to comply with the “3R principle”. GraphPad Prism 9 software was used for statistical evaluation and graphical display.
Results
Table 1 summarizes the parameters recorded of systemic hemodynamic, lung mechanics, gas exchange, acid-base status and metabolic parameters at the end of the experiment. In this murine model of STZ-induced DMT1, which tested the drug effects using a post-treatment approach together with standard ICU measures, STS had no significant effect on any of these parameters. Furthermore, as shown in Table 2, the stable isotope-based quantification of assessment of whole-body and organ-specific metabolic pathways and kidney function confirmed the lacking effect of STS under these conditions: neither the parameters measured of hepatic metabolic capacity (i.e., urea production), lipolysis (i.e., glycerol production), and protein degradation (i.e., leucine rate of appearance), nor kidney function (i.e., creatinine clearance) showed any significant difference when compared to the vehicle group. Finally, as shown in Figure 2 and Table 3, STS did not affect inflammatory markers measured systemically nor in the organ tissue with the exception of limited anti-inflammatory properties in the lungs, where STS treatment significantly reduced interleukin-6 and monocyte-chemoattractant-protein-1 expression. In contrast, in the kidney, the STS group presented with increased interleukin-18 expression, again, with no apparent clinical impact. The overall unchanged organ cytokine and chemokine concentrations were supported by the results of the immunoblotting: neither lung nor kidney expression of HO-1, IκBα, CBS, caspase 3, or the GR, showed any significant intergroup difference (see Figure 2). Ultimately, all these findings coincided with similar survival times in the two experimental groups (see Figure 3).
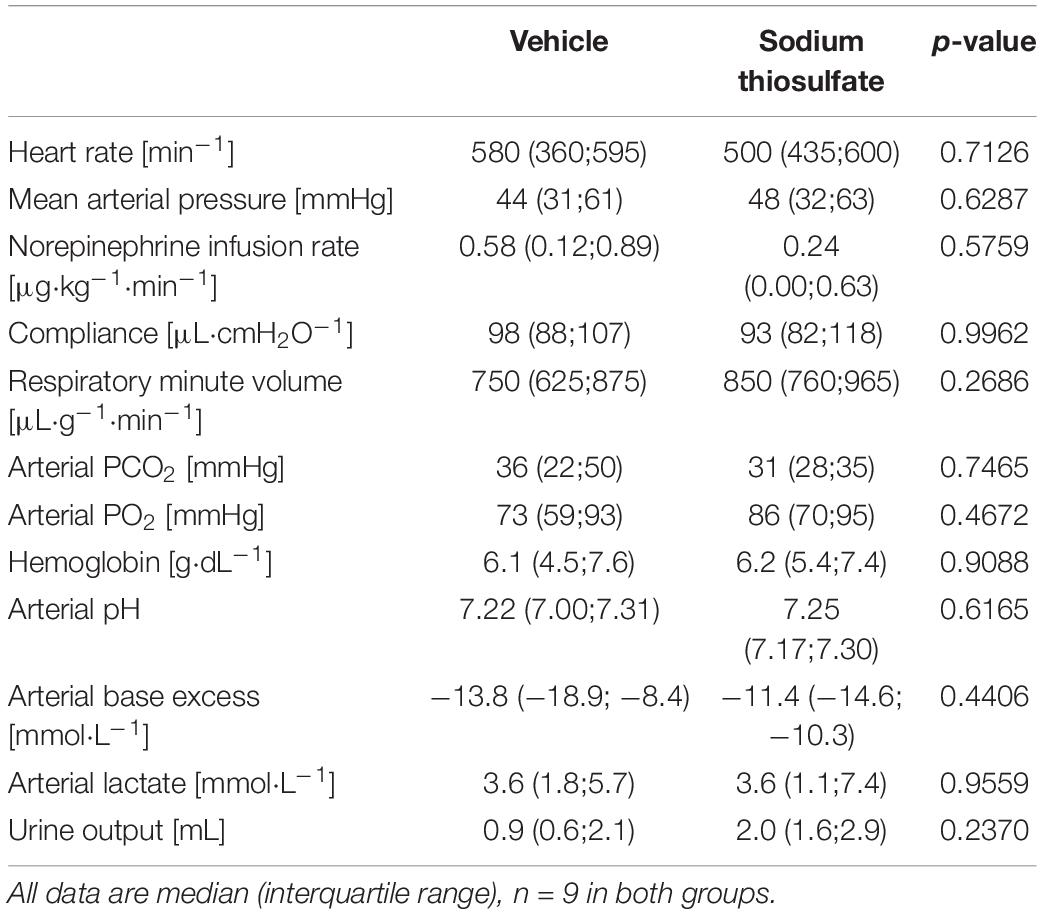
Table 1. Systemic hemodynamic, lung mechanics, gas exchange, acid-base status, and metabolic parameters at the end of the experiment.
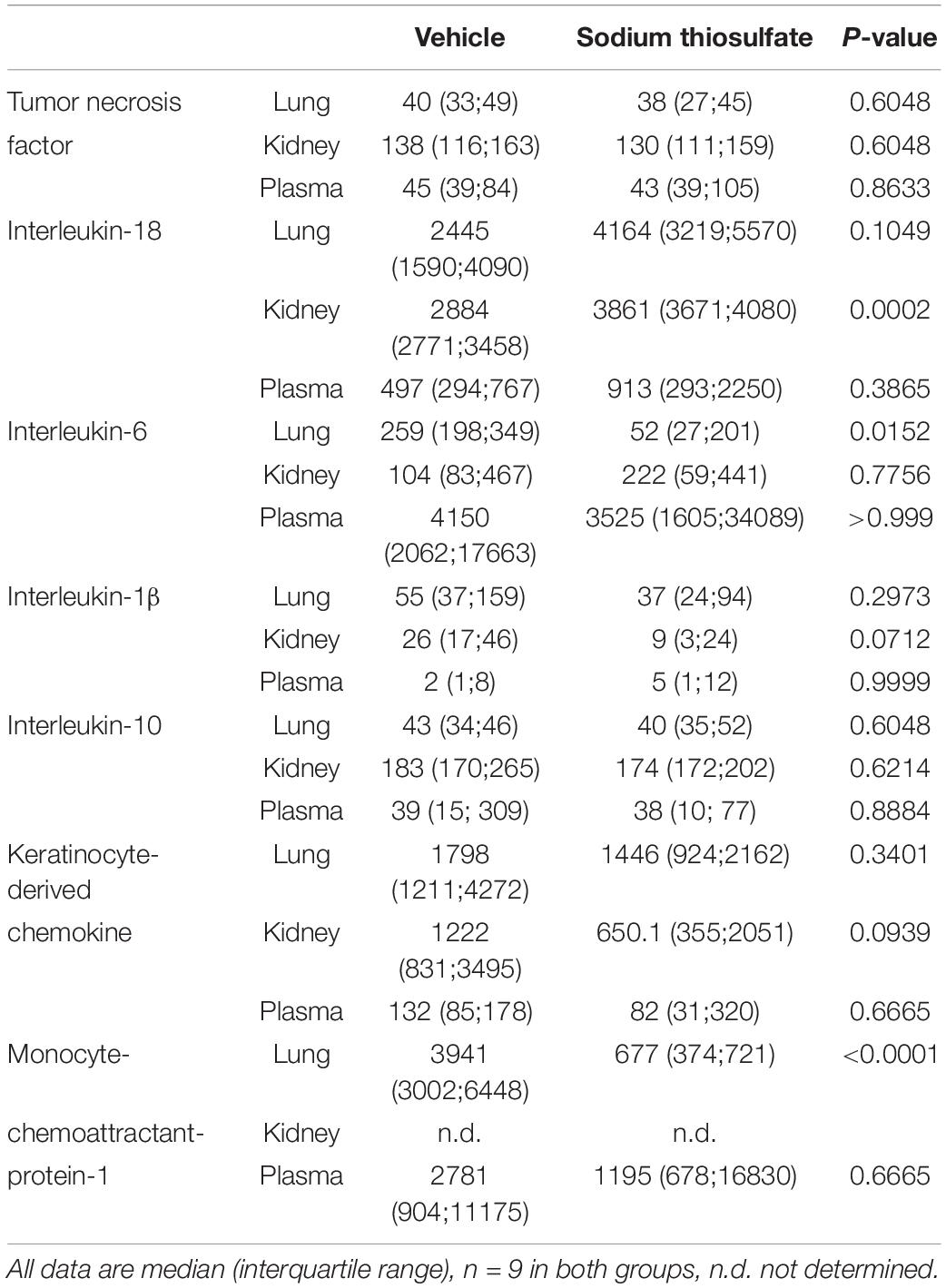
Table 3. Terminal plasma (in [pg⋅mL) and tissue (kidney and lung, [pg⋅mgprotein–1]) cytokine and chemokine concentrations.
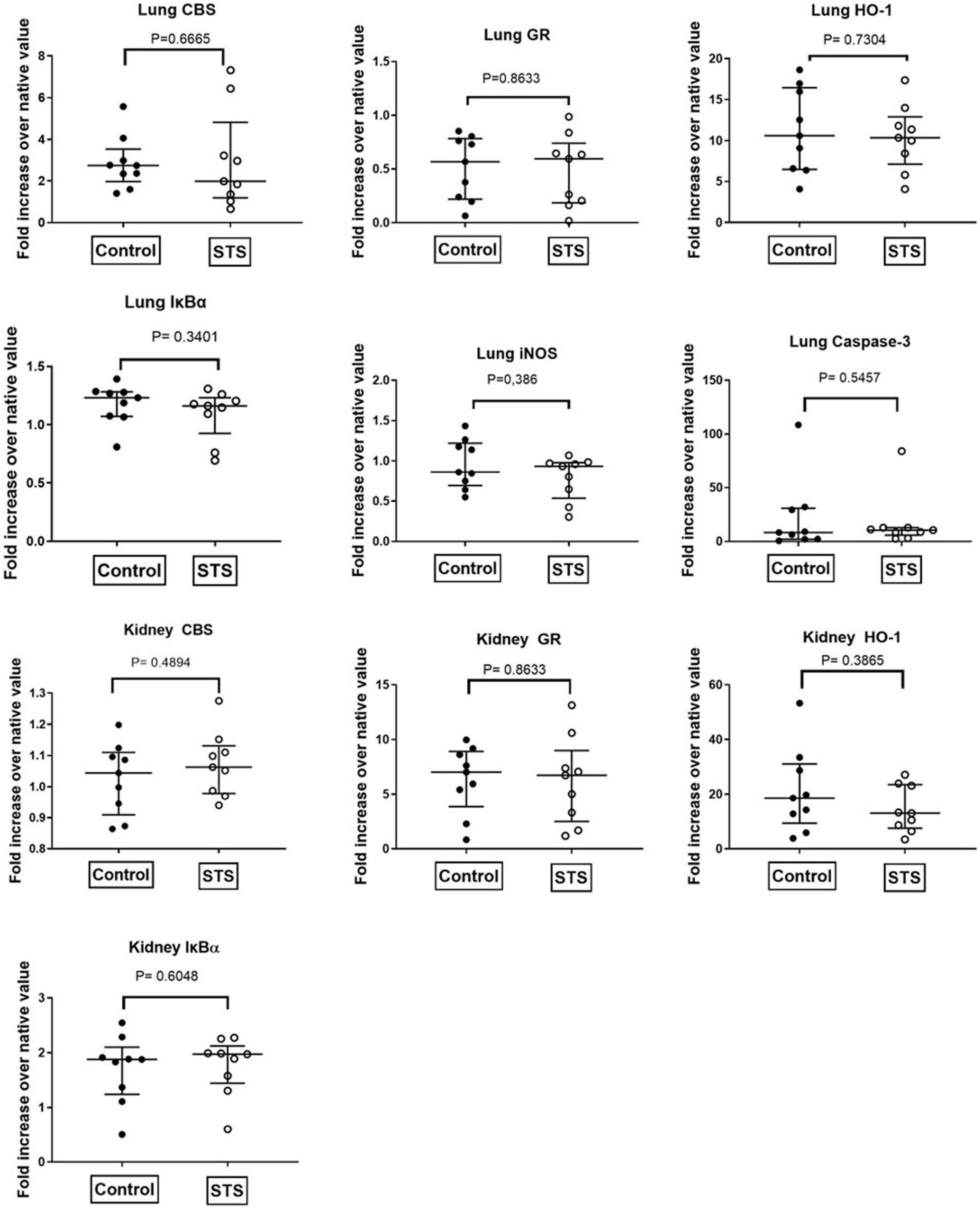
Figure 2. Quantitative analysis of immunoblots of lung and kidney tissue. CBS, Cystathionine-β-synthase; GR, glucocorticoid receptor; HO-1, heme-oxygenase 1; IκBα, nuclear factor of kappa light polypeptide gene enhancer in B-cells inhibitor, alpha; iNOS, inducible nitric oxide synthase.
Discussion
In this study, we tested the hypothesis whether STS would exert organ-protective effects in CSE-ko mice with STZ-induced DMT1. The main findings were that (i) in contrast to our previous findings in CSE-ko mice without underlying co-morbidity (10), STS did not exert any beneficial therapeutic effect under these conditions, (ii) possibly at least in part due to more severe circulatory shock. In addition, (iii) we confirmed previous findings (23) that metabolic derangements associated with STZ-induced DMT1 are less severe in CSE-ko mice, (iv) apparently in as a result of lower rates of endogenous glucose release (33).
Despite promising reports (34, 35) exogenous H2S administration produced equivocal results in murine models of hemorrhage-and-resuscitation, inasmuch undesired side effects were due to potentially high H2S peak concentrations (36) when the H2S-releasing salts NaSH and/or Na2S were used, or aggravation of shock due to the vasodilatory properties of so-called “slow-releasing H2S donors” (37). Therefore, STS was used, an H2S donor devoid of major undesired side effects and recognized for cyanide intoxication, cis-Pt-overdosing in oncology, as well as calciphylaxis in end stage kidney disease (1). STS has been shown to be beneficial in LPS- and polymicrobial sepsis-induced ALI (5), acute liver failure (4) and I/R injury (3), Pseudomonas aeruginosa-bacteremia (7) and colon ascendens stent peritonitis-induced sepsis (38) as well as both LPS- (6) and I/R-induced (2) brain injury. Moreover, STS also protected against arterial hypertension-induced congestive heart failure (39, 40) and kidney disease (41, 42). Finally, we have recently shown that post-treatment STS administration attenuated of lung, liver and kidney injury in non-comorbid CSE-ko mice undergoing trauma-and-hemorrhage and subsequent intensive care-based resuscitation (10).
We chose a single intraperitoneal STZ injection to induce DMT1 in in order to minimize any stress on the test animals from repeated i.p. administration. Moreover, this “single moderate-dose injection” has been referred to be associated with the least mortality, decreased renal toxicity, reduced “off target effects” and at the same time progressive hyperglycemia within a week post-STZ injection (30, 31, 43). Genetic CSE deletion attenuated this hyperglycemic response to the STZ injection: before the start of the actual trauma or MICU phase, all test animals showed hyperglycemia ≈260 mg⋅dL–1, in contrast to the blood glucose levels of approx. 100 mg⋅dL–1 reported in naïve CSE-ko mice (23). Hence, the degree of hyperglycemia was less than described in the literature for murine STZ-induced DMT1, i.e., in general ≥400 mg⋅dL–1 (30, 31) and e.g., at day 30 after five daily injections of 40 μg⋅g–1 STZ (508 ± 47 mg⋅dL–1 (23). Nevertheless, these values are in accordance with what is reported for STZ-DMT1 in CSE-ko mice, i.e., 259 ± 70 mg⋅dL–1 at the same time point (23). Strikingly, in both groups glycemia values returned to normal levels by the end of the experiment. Since glucose oxidation accounted for approx. 50% of the infused isotope-labeled substrate, i.e., was similar as previously shown in CSE-ko mice without DMT1 (10), this finding suggests a reduced rate of endogenous glucose release. Gluconeogenesis is a highly oxygen-dependent metabolic pathway, and both we and others previously showed that a lacking rise of glucose formation upon catecholamine stimulation and/or a fall in gluconeogenesis reflects impaired renal (44) and/or hepatic (45, 46) metabolic capacity. Moreover, even short-term (2 h only) (47) elevation of norepinephrine concentrations can compromise hepatocellular function. In addition, naïve CSE-ko mice per se have a reduced rate of gluconeogenesis, which was reversed by i.p. administration of the H2S releasing salt NaHS (33). In good agreement with these findings, we showed in CSE-ko mice undergoing trauma, hemorrhage and resuscitation that the “slow-releasing H2S donor” GYY4137 restored reduced blood glucose levels to the values found in wild type animals (48). Additionally, wild type mice undergoing a similar hemorrhage-and-resuscitation protocol showed a 20% higher stable isotope infusion-derived rate of gluconeogenesis (32) than both the non-diabetic CSE-ko mice in the previous (10) as well as the DMT1 animals in the present study (median 3.6 vs. 3.2 and 2.7 μmol⋅g–1⋅h–1, respectively). A limitation of this study was imposed by the Federal Authorities for Animal Research (Regierungspräsidium Tübingen Baden-Württemberg, Germany, Reg.-Nr. 1387, approval January 31, 2018), which only approved experiments for CSE-ko mice where the strongest effect of STS was expected. Thus, we would only be able to speculate on the results of STS treatment in DMT1 in WT mice when exposed to resuscitation from trauma-and-hemorrhage as in the current protocol, but the effects of hyperglycemia per se would imply more severe disturbance of mitochondrial respiration, possibly more profound oxidative stress and concomitant depressed liver metabolic capacity (49, 50). Furthermore, though we used single injection of a low dose of STZ known for its minimal collateral effects, Nørgaard et al. (43) found that all STZ doses they investigated, including ours, led to significant enlargement of the stomach with no pathological changes and not caused by food intake. Hence, albeit, they studied short-term effects (1 day post injection) while our animals had a “recovery” period of 7 days, nevertheless, we cannot rule out that these non-specific effects of STZ, a fundamental issue of the model per se, may have masked any beneficial STS effects. Although in rat STZ models of DMT1, with an intact endogenous H2S enzymes system, chronic administration of H2S prevented the development of diabetic nephropathy (24) and attenuated diabetic retinopathy (25). A very important piece of the puzzle was denied us by the authorities limiting our original experimental design (which included WT mice) to only CSEko mice. Thus we are unable to conclude if the lack of CSE or non-specific effects of STZ interfered with the potential benefits of acute administration of STS in our resuscitated circulatory shock model, as observed in our previous experiment without the DMT1 comorbidity (10).
The limited data available in CSE-ko mice in resuscitative circulatory shock models show increased local and systemic pro-inflammatory chemokine and cytokine concentrations, higher heart rate and MAP as well as lower glucose levels in comparison to WT. The administration of GYY4137, a slow releasing H2S donor, restored glucose levels to normal and slightly reduced MAP but had no effect on heart rate (48, 51).
In the present study compared to vehicle-treated animals, STS treatment affected neither systemic hemodynamics, lung mechanics, gas exchange, metabolism nor organ (dys)function. Most of the parameters measured of the systemic and organ inflammatory response nor the tissue expression of marker proteins of the stress response were affected either. These findings are in contrast to our previous study in CSE-ko mice without underlying co-morbidity (10). In fact, the role of H2S in DMT1 is controversial: on the one hand, DMT1 in patients has been reported to be associated with reduced H2S production as a result of decreased CSE activity (52), even suggesting a window for potential exogenous H2S therapy (53, 54). “Non-obese”, genetic DMT1 mice showed impaired H2S biosynthesis, the degree of which was directly related to the severity of glucosuria (55). In addition, reduced H2S availability has been referred to contribute to both diabetes-induced endothelial dysfunction (56) and cardiomyopathy (57). In contrast, increased H2S levels and/or CSE overexpression have been shown to at least partially inhibit high glucose-induced insulin secretion (58) due to activation of KATP channels in islet cells (59). Moreover, as mentioned-above, development and severity of hyperglycemia after STZ injection was attenuated in CSE-ko mice when compared to wild type littermates (23).
Irrespective of the lacking organ-protective effect of STS, DMT1 in CSE-ko mice was associated with aggravated severity of shock when compared to non-diabetic animals in our previous investigation (10): norepinephrine requirements to achieve hemodynamic targets were higher (median 0.58 vs. 0.27 μg⋅kg–1⋅min–1) and lactic acidosis more severe (arterial lactate and base excess median 3.6 vs. 2.5 and −13.8 vs. −10.3 mmol⋅L–1, respectively; arterial pH median 7.22 vs. 7.30). It is tempting to speculate that hyperglycemia per se plays a central role in this context: hyperglycemia >250 mg⋅dL–1 in mice with STZ-induced DMT1 was associated with aggravation of ventilator-induced acute lung injury (60), and blood glucose levels as high as 755 mg⋅dL–1 caused marked aggravation of acute kidney injury after hemorrhagic shock in 20-weeks old db/db mice, i.e., with diabetes type 2 (61). Finally, we had shown that mice with septic shock rendered hyperglycemic by i.v. glucose required the highest norepinephrine infusion rates in order to achieve pre-defined hemodynamic target values, which despite comparable parameters of microcirculatory perfusion and oxygen supply was associated with the most pronounced decrease in mitochondrial respiration and severity of lactic acidosis (49).
The above findings underscore the need to further investigate the treatment of the metabolic imbalance in DMT1 patients admitted to the ICU. In contrast to the general population adults with DMT1 have a higher admission and increased mortality in the ICU (62). Furthermore, the unexpected findings by Liou et al. (63) that a higher mortality rate after moderate to serious trauma is associated with DMT1 but not with DMT2 suggests a need to taper patient care specifically to the DMT1 trauma patients: early ICU admission, rapid hyperglycemia and related insulin therapy especially after moderate to severe trauma in an effort to increase survival (63). It is also noteworthy that in the current COVID-19 pandemic, caused by SARS-CoV-2, DMT1 patients are at higher risk for severe outcomes and ICU admission than those without diabetes and those with DMT2 (64). These results were echoed in a pediatric study where the strongest risk factors for hospitalization and severe COVID-19 was DMT1 (65). Interestingly, “a hallmark of COVID-19” are significantly reduced H2S levels in non-survivors. Therefore, the therapeutic potential for H2S donors in their ability to maintain physiological homeostasis attenuating endothelial dysfunction and kidney injury (common disorders in DMT1) in COVID-19 patients, especially STS, may assume particular importance under this condition. A separate discussion is beyond the scope of this paper (66–71).
Conclusion
In the present study and in contrast to our previous findings in CSE-ko mice without underlying co-morbidity (10) STS did not exert any beneficial therapeutic effect in mice with STZ-induced DMT1 under these conditions, possibly due to DMT1-related more severe circulatory shock and/or unspecific STZ-related effects. This result highlights the translational importance not only of integrating standard ICU procedures into the design of experimental models for shock research, but also the investigation of underlying co-morbidity, even when rodents are used because of the availability of genetically modified strains.
Data Availability Statement
All data is contained within the article and Supplementary Material.
Ethics Statement
The animal study was reviewed and approved by the University of Ulm Animal Care Committee and the Federal Authorities for Animal Research (Regierungspräsidium Tübingen, Baden-Württemberg, Germany, Reg. No. 1387, approval January 31, 2018).
Author Contributions
PR: conceptualization, funding, project administration, and supervision. MG, SK, EA, AH, and UW: surgical instrumentation and methodology. EA, EC, MG, AH, MH, TM, BS, JV, and UW: investigation. EA, MH, OM, BS, and JV: data curation. TM and OM: figures and visualization. OM and PR: writing – original draft preparation. PR, OM, RW, and TM: writing – review and editing. All authors have read and agreed to the published version of the manuscript.
Funding
PR and TM are supported by the Deutsche Forschungsgemeins chaft (CRC 1149, project no. 251293561). RW was supported by a Discovery Grant from Natural Science and Engineering Research Council of Canada.
Conflict of Interest
The authors declare that the research was conducted in the absence of any commercial or financial relationships that could be construed as a potential conflict of interest.
Publisher’s Note
All claims expressed in this article are solely those of the authors and do not necessarily represent those of their affiliated organizations, or those of the publisher, the editors and the reviewers. Any product that may be evaluated in this article, or claim that may be made by its manufacturer, is not guaranteed or endorsed by the publisher.
Acknowledgments
We are indebted to Rosemarie Mayer and Marina Fink for skillful technical assistance.
Supplementary Material
The Supplementary Material for this article can be found online at: https://www.frontiersin.org/articles/10.3389/fmed.2022.878823/full#supplementary-material
References
1. McGeer PL, McGeer EG, Lee M. Medical uses of sodium thiosulfate. J Neurol Neuromed. (2016) 1:28–30. doi: 10.1016/j.ekir.2018.10.002
2. Marutani E, Yamada M, Ida T, Tokuda K, Ikeda K, Kai S, et al. Thiosulfate mediates cytoprotective effects of hydrogen sulfide against neuronal ischemia. J Am Heart Assoc. (2015) 4:e002125. doi: 10.1161/JAHA.115.002125
3. Bauer M, Radermacher P, Wepler M. Sodium thiosulfate: a new player for circulatory shock and ischemia/reperfusion injury? In: JL Vincent editor. Annual Update in Intensive Care and Emergency Medicine. (Cham: Springer) (2019). p. 167–78.
4. Shirozu K, Tokuda K, Marutani E, Lefer D, Wang R, Ichinose F. Cystathionine γ-lyase deficiency protects mice from galactosamine/lipopolysaccharide-induced acute liver failure. Antioxid Red Signal. (2014) 20:204–16. doi: 10.1089/ars.2013.5354
5. Sakaguchi M, Marutani E, Shin HS, Chen W, Hanaoka K, Xian M, et al. Sodium thiosulfate attenuates acute lung injury in mice. Anesthesiology. (2014) 121:1248–57. doi: 10.1097/ALN.0000000000000456
6. Acero G, Nava Catorce M, González-Mendoza R, Meraz-Rodríguez MA, Hernández-Zimbron LF, González-Salinas R, et al. Sodium thiosulphate attenuates brain inflammation induced by systemic lipopolysaccharide administration in C57BL/6J mice. Inflammopharmacology. (2017) 25:585–596. doi: 10.1007/s10787-017-0355-y
7. Renieris G, Droggiti DE, Katrini K, Koufargyris P, Gkavogianni T, Karakike E, et al. Host cystathionine-γ lyase derived hydrogen sulfide protects against Pseudomonas aeruginosa sepsis. PLoS Pathog. (2021) 17:e1009473. doi: 10.1371/journal.ppat.1009473
8. Datzmann T, Hoffmann A, McCook O, Merz T, Wachter U, Preuss J, et al. Effects of sodium thiosulfate (Na2S2O3) during resuscitation from hemorrhagic shock in swine with preexisting atherosclerosis. Pharmacol Res. (2020) 151:104536. doi: 10.1016/j.phrs.2019.104536
9. Merz T, Stenzel T, Nußbaum B, Wepler M, Szabo C, Wang R, et al. Cardiovascular disease and resuscitated septic shock lead to the downregulation of the H2S-producing enzyme cystathionine-γ-lyase in the porcine coronary artery. Intensive Care Med Exp. (2017) 1:17. doi: 10.1186/s40635-017-0131-8
10. Gröger M, Hogg M, Abdelsalam E, Kress S, Hoffmann A, Stahl B, et al. Effects of sodium thiosulfate during resuscitation from trauma-and-hemorrhage in cystathionine gamma lyase (CSE) knockout mice. Shock. (2022) 57:131–9. doi: 10.1097/SHK.0000000000001828
11. Yang G, Wu L, Jiang B, Yang W, Qi J, Cao K, et al. H2S as a physiologic vasorelaxant: hypertension in mice with deletion of cystathionine gamma-lyase. Science. (2008) 322:587–90. doi: 10.1126/science.1162667
12. Wu L, Yang W, Jia X, Yang G, Duridanova D, Cao K, et al. Pancreatic islet overproduction of H2S and suppressed insulin release in zucker diabetic rats. Lab Invest. (2009) 89:59–67. doi: 10.1038/labinvest.2008.109
13. Zhang H, Huang Y, Chen S, Tang C, Wang G, Du J, et al. Hydrogen sulfide regulates insulin secretion and insulin resistance in diabetes mellitus, a new promising target for diabetes mellitus treatment? A review. J Adv Res. (2020) 27:19–30. doi: 10.1016/j.jare.2020.02.013
14. Feliers D, Lee HJ, Kasinath BS. Hydrogen sulfide in renal physiology and disease. Antioxid Redox Signal. (2016) 25:720–31. doi: 10.1089/ars.2015.6596
15. Sen U, Pushpakumar S. Mini-review: diabetic renal complications, a potential stinky remedy. Am J Physiol Renal Physiol. (2016) 310:F119–22. doi: 10.1152/ajprenal.00299.2015
16. Yamamoto J, Sato W, Kosugi T, Yamamoto T, Kimura T, Taniguchi S. Distribution of hydrogen sulfide (H2S)-producing enzymes and the roles of the H2S donor sodium hydrosulfide in diabetic nephropathy. Clin Exp Nephrol. (2013) 1:32–40. doi: 10.1007/s10157-012-0670-y
17. Xue R, Hao DD, Sun JP, Li WW, Zhao MM, Li XH, et al. Hydrogen sulfide treatment promotes glucose uptake by increasing insulin receptor sensitivity and ameliorates kidney lesions in type 2 diabetes. Antioxid Redox Signal. (2013) 19:5–23. doi: 10.1089/ars.2012.5024
18. Xue H, Yuan P, Ni J, Li C, Shao D, Liu J, et al. HS inhibits hyperglycemia-induced intrarenal renin-angiotensin system activation via attenuation of reactive oxygen species generation. PLoS One. (2013) 8:e74366. doi: 10.1371/journal.pone.0074366
19. Ahmad FU, Sattar MA, Rathore HA, Abdullah MH, Tan S, Abdullah NA, et al. Exogenous hydrogen sulfide (H2S) reduces blood pressure and prevents the progression of diabetic nephropathy in spontaneously hypertensive rats. Ren Fail. (2012) 34:203–10. doi: 10.3109/0886022X.2011.643365
20. Ng HH, Yildiz GS, Ku JM, Miller AA, Woodman OL, Hart JL. Chronic NaHS treatment decreases oxidative stress and improves endothelial function in diabetic mice. Diab Vasc Dis Res. (2017) 14:246–53. doi: 10.1177/1479164117692766
21. Liu F, Chen DD, Sun X, Xie HH, Yuan H, Jia W, et al. Hydrogen sulfide improves wound healing via restoration of endothelial progenitor cell functions and activation of angiopoietin-1 in type 2 diabetes. Diabetes. (2014) 63:1763–78. doi: 10.2337/db13-0483
22. Wang G, Li W, Chen Q, Jiang Y, Lu X, Zhao X. Hydrogen sulfide accelerates wound healing in diabetic rats. Int J Clin Exp Pathol. (2015) 8:5097–104.
23. Yang G, Tang G, Zhang L, Wu L, Wang R. The pathogenic role of cystathionine γ-lyase/hydrogen sulfide in streptozotocin-induced diabetes in mice. Am J Pathol. (2011) 179:869–79. doi: 10.1016/j.ajpath.2011.04.028
24. Zhou X, Feng Y, Zhan Z, Chen J. Hydrogen sulfide alleviates diabetic nephropathy in a streptozotocin-induced diabetic rat model. J Biol Chem. (2014) 289:28827–34. doi: 10.1074/jbc.M114.596593
25. Si YF, Wang J, Guan J, Zhou L, Sheng Y, Zhao J. Treatment with hydrogen sulfide alleviates streptozotocin-induced diabetic retinopathy in rats. Br J Pharmacol. (2013) 169:619–31. doi: 10.1111/bph.12163
26. Esper AM, Martin GS. The impact of comorbid [corrected] conditions on critical illness. Crit Care Med. (2011) 12:2728–35. doi: 10.1097/CCM.0b013e318236f27e
27. Chang MW, Huang CY, Liu HT, Chen YC, Hsieh CH. Stress-induced and diabetic hyperglycemia associated with higher mortality among intensive care unit trauma patients: cross-sectional analysis of the propensity score-matched population. Int J Environ Res Public Health. (2018) 15:992. doi: 10.3390/ijerph15050992
28. Leiter EH. Multiple low-dose streptozotocin-induced hyperglycemia and insulitis in C57BL mice: influence of inbred background, sex, and thymus. Proc Natl Acad Sci USA. (1982) 79:630–4. doi: 10.1073/pnas.79.2.630
29. King AJ. The use of animal models in diabetes research. Br J Pharmacol. (2012) 166:877–94. doi: 10.1111/j.1476-5381.2012.01911.x
30. Deeds MC, Anderson JM, Armstrong AS, Gastineau DA, Hiddinga HJ, Jahangir A, et al. Single dose streptozotocin-induced diabetes: considerations for study design in islet transplantation models. Lab Anim. (2011) 45:131–40. doi: 10.1258/la.2010.010090
31. Hayashi K, Kojima R, Ito M. Strain differences in the diabetogenic activity of streptozotocin in mice. Biol Pharm Bull. (2006) 29:1110–9. doi: 10.1248/bpb.29.1110
32. Langgartner D, Wachter U, Hartmann C, Gröger M, Vogt J, Merz T, et al. Effects of psychosocial stress on subsequent hemorrhagic shock and Resuscitation in male mice. Shock. (2019) 51:725–30. doi: 10.1097/SHK.0000000000001204
33. Untereiner AA, Wang R, Ju Y, Wu L. Decreased gluconeogenesis in the absence of cystathionine gamma-lyase and the underlying mechanisms. Antioxid Redox Signal. (2016) 24:129–40. doi: 10.1089/ars.2015.6369
34. Morrison ML, Blackwood JE, Lockett SL, Iwata A, Winn RK, Roth MB. Surviving blood loss using hydrogen sulfide. J Trauma. (2008) 65:183–8. doi: 10.1097/TA.0b013e3181507579
35. Dyson A, Dal-Pizzol F, Sabbatini G, Lach AB, Galfo F, Dos Santos Cardoso J, et al. Ammonium tetrathiomolybdate following ischemia/reperfusion injury: chemistry, pharmacology, and impact of a new class of sulfide donor in preclinical injury models. PLoS Med. (2017) 14:e1002310. doi: 10.1371/journal.pmed.1002310
36. Whiteman M, Li L, Rose P, Tan CH, Parkinson DB, Moore PK. The effect of hydrogen sulfide donors on lipopolysaccharide-induced formation of inflammatory mediators in macrophages. Antioxid Redox Signal. (2010) 12:1147–54. doi: 10.1089/ars.2009.2899
37. Wepler M, Merz T, Wachter U, Vogt J, Calzia E, Scheuerle A, et al. The mitochondria-targeted H2S-donor AP39 in a murine model of combined hemorrhagic shock and blunt chest Trauma. Shock. (2019) 52:230–9. doi: 10.1097/SHK.0000000000001210
38. Schulz J, Kramer S, Kanatli Y, Kuebart A, Bauer I, Picker O, et al. Sodium thiosulfate improves intestinal and hepatic microcirculation without affecting mitochondrial function in experimental sepsis. Front Immunol. (2021) 12:671935. doi: 10.3389/fimmu.2021.671935
39. Snijder PM, Frenay AR, de Boer RA, Pasch A, Hillebrands JL, Leuvenink HG, et al. Exogenous administration of thiosulfate, a donor of hydrogen sulfide, attenuates angiotensin II-induced hypertensive heart disease in rats. Br J Pharmacol. (2015) 172:1494–504. doi: 10.1111/bph.12825
40. Nguyen ITN, Wiggenhauser LM, Bulthuis M, Hillebrands JL, Feelisch M, Verhaar MC, et al. Cardiac protection by oral sodium thiosulfate in a rat model of L-NNA-induced heart disease. Front Pharmacol. (2021) 12:650968. doi: 10.3389/fphar.2021.650968
41. Snijder PM, Frenay AR, Koning AM, Bachtler M, Pasch A, Kwakernaak AJ, et al. Sodium thiosulfate attenuates angiotensin II-induced hypertension, proteinuria and renal damage. Nitric Oxide. (2014) 42:87–98. doi: 10.1016/j.niox.2014.10.002
42. Nguyen ITN, Klooster A, Minnion M, Feelisch M, Verhaar MC, van Goor H, et al. Sodium thiosulfate improves renal function and oxygenation in L-NNA-induced hypertension in rats. Kidney Int. (2020) 98:366–77. doi: 10.1016/j.kint.2020.02.020
43. Nørgaard SA, Søndergaard H, Sørensen DB, Galsgaard ED, Hess C, Sand FW. Optimising streptozotocin dosing to minimise renal toxicity and impairment of stomach emptying in male 129/Sv mice. Lab Anim. (2020) 54:341–52. doi: 10.1177/0023677219872224
44. Maitra SR, Homan CS, Pan W, Geller ER, Henry MC, Thode HC Jr. Renal gluconeogenesis and blood flow during endotoxic shock. Acad Emerg Med. (1996) 3:1006–10. doi: 10.1111/j.1553-2712.1996.tb03343.x
45. Albuszies G, Radermacher P, Vogt J, Wachter U, Weber S, Schoaff M, et al. Effect of increased cardiac output on hepatic and intestinal microcirculatory blood flow, oxygenation, and metabolism in hyperdynamic murine septic shock. Crit Care Med. (2005) 33:2332–8. doi: 10.1097/01.ccm.0000182817.20977.e9
46. Albuszies G, Vogt J, Wachter U, Thiemermann C, Leverve XM, Weber S, et al. The effect of iNOS deletion on hepatic gluconeogenesis in hyperdynamic murine septic shock. Intensive Care Med. (2007) 33:1094–101. doi: 10.1007/s00134-007-0638-7
47. Yang S, Koo DJ, Zhou M, Chaudry IH, Wang P. Gut-derived norepinephrine plays a critical role in producing hepatocellular dysfunction during early sepsis. Am J Physiol Gastrointest Liver Physiol. (2000) 279:G1274–81. doi: 10.1152/ajpgi.2000.279.6.G1274
48. Merz T, Lukaschewski B, Wigger D, Rupprecht A, Wepler M, Gröger M, et al. Interaction of the hydrogen sulfide system with the oxytocin system in the injured mouse heart. Intensive Care Med Exp. (2018) 6:41. doi: 10.1186/s40635-018-0207-0
49. Merz T, Vogt JA, Wachter U, Calzia E, Szabo C, Wang R, et al. Impact of hyperglycemia on cystathionine-γ-lyase expression during resuscitated murine septic shock. Intensive Care Med Exp. (2017) 5:30. doi: 10.1186/s40635-017-0140-7
50. Vogt JA, Wachter U, Wagner K, Calzia E, Gröger M, Weber S, et al. Effects of glycemic control on glucose utilization and mitochondrial respiration during resuscitated murine septic shock. Intensive Care Med Exp. (2014) 2:19. doi: 10.1186/2197-425X-2-19
51. Hartmann C, Hafner S, Scheuerle A, Möller P, Huber-Lang M, Jung B, et al. The role of cystathionine-γ-lyase in blunt chest trauma in cigarette smoke exposed Mice. Shock. (2017) 47:491–9. doi: 10.1097/SHK.0000000000000746
52. Manna P, Gungor N, McVie R, Jain SK. Decreased cystathionine-γ-lyase (CSE) activity in livers of type 1 diabetic rats and peripheral blood mononuclear cells (PBMC) of type 1 diabetic patients. J Biol Chem. (2014) 289:11767–78. doi: 10.1074/jbc.M113.524645
53. Gheibi S, Samsonov AP, Gheibi S, Vazquez AB, Kashfi K. Regulation of carbohydrate metabolism by nitric oxide and hydrogen sulfide: implications in diabetes. Biochem Pharmacol. (2020) 176:113819. doi: 10.1016/j.bcp.2020.113819
54. Lefer DJ. Potential importance of alterations in hydrogen sulphide (H2S) bioavailability in diabetes. Br J Pharmacol. (2008) 155:617–9. doi: 10.1038/bjp.2008.359
55. Brancaleone V, Roviezzo F, Vellecco V, De Gruttola L, Bucci M, Cirino G. Biosynthesis of H2S is impaired in non-obese diabetic (n.d.) mice. Br J Pharmacol. (2008) 155:673–80. doi: 10.1038/bjp.2008.296
56. Sun HJ, Wu ZY, Nie XW, Bian JS. Role of endothelial dysfunction in cardiovascular diseases: the link between inflammation and hydrogen sulfide. Front Pharmacol. (2020) 10:1568. doi: 10.3389/fphar.2019.01568
57. Sun HJ, Wu ZY, Nie XW, Wang XY, Bian JS. An updated insight into molecular mechanism of hydrogen sulfide in cardiomyopathy and myocardial ischemia/reperfusion injury under diabetes. Front Pharmacol. (2021) 12:651884. doi: 10.3389/fphar.2021.651884
58. Kaneko Y, Kimura Y, Kimura H, Niki I. L-cysteine inhibits insulin release from the pancreatic beta-cell: possible involvement of metabolic production of hydrogen sulfide, a novel gasotransmitter. Diabetes. (2006) 55:1391–7. doi: 10.2337/db05-1082
59. Yang W, Yang G, Jia X, Wu L, Wang R. Activation of KATP channels by H2S in rat insulin-secreting cells and the underlying mechanisms. J Physiol. (2005) 569:519–31. doi: 10.1113/jphysiol.2005.097642
60. Xiong XQ, Wang WT, Wang LR, Jin LD, Lin LN. Diabetes increases inflammation and lung injury associated with protective ventilation strategy in mice. Int Immunopharmacol. (2012) 13:280–3. doi: 10.1016/j.intimp.2012.04.020
61. Dupuy V, Mayeur N, Buléon M, Jaafar A, Al Saati T, Schaak S, et al. Type 2 diabetes mellitus in mice aggravates the renal impact of hemorrhagic shock. Shock. (2012) 38:351–5. doi: 10.1097/SHK.0b013e318268810f
62. Marrie RA, Sellers EAC, Chen H, Fransoo R, Bernstein CN, Hitchon CA, et al. Markedly increased incidence of critical illness in adults with Type 1 diabetes. Diabet Med. (2017) 34: doi: 10.1111/dme.13404
63. Liou DZ, Singer MB, Barmparas G, Harada MY, Mirocha J, Bukur M, et al. Insulin-dependent diabetes and serious trauma. Eur J Trauma Emerg Surg. (2016) 42:491–6. doi: 10.1007/s00068-015-0561-5
64. Barrett CE, Park J, Kompaniyets L, Baggs J, Cheng YJ, Zhang P, et al. Intensive care unit admission, mechanical ventilation, and mortality among patients with type 1 diabetes hospitalized for COVID-19 in the U.S. Diabetes Care. (2021) 44:1788–96. doi: 10.2337/dc21-0604
65. Kompaniyets L, Agathis NT, Nelson JM, Preston LE, Ko JY, Belay B, et al. Underlying medical conditions associated with severe COVID-19 illness among children. JAMA Netw Open (2021) 4:e2111182. doi: 10.1001/jamanetworkopen.2021.11182
66. Renieris G, Katrini K, Damoulari C, Akinosoglou K, Psarrakis C, Kyriakopoulou M, et al. Serum hydrogen sulfide and outcome association in pneumonia by the SARS-CoV-2 coronavirus. Shock. (2020) 54:633–7. doi: 10.1097/SHK.0000000000001562
67. Dominic P, Ahmad J, Bhandari R, Pardue S, Solorzano J, Jaisingh K, et al. Decreased availability of nitric oxide and hydrogen sulfide is a hallmark of COVID-19. Redox Biol. (2021) 43:101982. doi: 10.1016/j.redox.2021.101982
68. Gorini F, Del Turco S, Sabatino L, Gaggini M, Vassalle C. H2S as a bridge linking inflammation, oxidative stress and endothelial biology: a possible defense in the fight against SARS-CoV-2 infection? Biomedicines. (2021) 9:1107. doi: 10.3390/biomedicines9091107
69. Radermacher P, Calzia E, McCook O, Wachter U, Szabo C. To the editor. Shock. (2021) 55:138–9. doi: 10.1097/SHK.0000000000001602
70. Datzmann T, Merz T, McCook O, Szabo C, Radermacher P. H2S as a therapeutic adjuvant against covid-19: why and how? Shock. (2021) 56:865–7. doi: 10.1097/SHK.0000000000001723
Keywords: gluconeogenesis, ureagenesis, hydrogen sulfide, cystathionine-β-synthase, heme oxygenase-1, IκBα, glucocorticoid receptor, lipolysis
Citation: Gröger M, Hogg M, Abdelsalam E, Kress S, Hoffmann A, Stahl B, Calzia E, Wachter U, Vogt JA, Wang R, Merz T, Radermacher P and McCook O (2022) Effects of Sodium Thiosulfate During Resuscitation From Trauma-and-Hemorrhage in Cystathionine-γ-Lyase Knockout Mice With Diabetes Type 1. Front. Med. 9:878823. doi: 10.3389/fmed.2022.878823
Received: 18 February 2022; Accepted: 06 April 2022;
Published: 29 April 2022.
Edited by:
Wolfgang Weihs, Medical University of Vienna, AustriaReviewed by:
Guangdong Yang, Laurentian University, CanadaRisheng Xu, The Johns Hopkins Hospital, Johns Hopkins Medicine, United States
Copyright © 2022 Gröger, Hogg, Abdelsalam, Kress, Hoffmann, Stahl, Calzia, Wachter, Vogt, Wang, Merz, Radermacher and McCook. This is an open-access article distributed under the terms of the Creative Commons Attribution License (CC BY). The use, distribution or reproduction in other forums is permitted, provided the original author(s) and the copyright owner(s) are credited and that the original publication in this journal is cited, in accordance with accepted academic practice. No use, distribution or reproduction is permitted which does not comply with these terms.
*Correspondence: Oscar McCook, T3NjYXIuTWNDb29rQHVuaS11bG0uZGU=
†These authors have contributed equally to this work and share first authorship