- 1Division of Hematology Oncology, Department of Pediatrics, Children's Hospital of Michigan, Central Michigan University School of Medicine, Detroit, MI, United States
- 2Division of Critical Care Medicine, Department of Pediatrics, Texas Children's Hospital, Baylor University School of Medicine, Houston, TX, United States
- 3Division of Critical Care Medicine, Department of Pediatrics, Duke Children's Hospital, Duke University School of Medicine, Durham, NC, United States
Pediatric mechanical circulatory support can be lifesaving. However, managing anticoagulation is one of the most challenging aspects of care in patients requiring mechanical circulatory support. Effective anticoagulation is even more difficult in pediatric patients due to the smaller size of their blood vessels, increased turbulent flow, and developmental hemostasis. Recently, viscoelastic testing (VET) has been used as a qualitative measure of anticoagulation efficacy in patients receiving extracorporeal membrane oxygenation (ECMO) and ventricular assist devices (VAD). Thromboelastography (TEG®) and thromboelastometry (ROTEM®) provide a global qualitative assessment of hemostatic function from initiation of clot formation with the platelet-fibrin interaction, platelet aggregation, clot strength, and clot lysis. This review focuses on the TEG®/ROTEM® and important laboratory and patient considerations for interpretation in the ECMO and VAD population. We summarize the adult and pediatric ECMO/VAD literature regarding VET values, VET-platelet mapping, utility over standard laboratory monitoring, and association with outcome measures such as blood product utilization, bleeding, and thrombosis.
Introduction
Mechanical circulatory support in children is provided utilizing extracorporeal membrane oxygenation (ECMO) or ventricular assist devices (VADs). These therapies are used for respiratory, cardiac, or extracorporeal cardiopulmonary resuscitation (ECPR) support. While ECMO and VADs can be lifesaving, patients are at increased risk of bleeding (due to critical illness and systemic anticoagulation) and thrombosis. According to the Extracorporeal Life Support Organization (ELSO) registry, neonatal and pediatric patients make up about one-half of overall ECMO runs (1). In the bleeding and thrombosis during ECMO (BATE) study performed prospectively at eight centers affiliated with the Collaborative Pediatric Critical Care Research Network (CPCCRN), bleeding events were observed in 70% of children and thrombotic events in 38%. The presence of either bleeding or thrombosis was associated with adverse cognitive and functional outcomes, and bleeding events were associated with increased mortality (2). The Berlin Heart EXCOR Pediatric VAD Investigational Device Exemption (IDE) study, which included children ≤ 16 years of age across 17 pediatric cardiac centers, reported major bleeding occurred in 43% of patients, neurologic events in 28%, and pump changes for suspected/confirmed thrombosis in 56% of patients (3). There were five patient deaths in this cohort and four of these were related to thrombotic events (3). While these studies are informative, the true incidence of bleeding and thrombosis in pediatric ECMO/VAD is unknown due to a limited number of prospective outcome studies, variable definitions of bleeding and thrombosis used, and a lack of validated severity of bleeding and thrombosis scoring tools. We know that bleeding and thrombosis contribute to morbidity and mortality. Attempts to mitigate these risks have focused on circuit modifications, anticoagulant medications, and changes in hemostatic monitoring including viscoelastic testing (VET).
In this review, we provide an overview of standard anticoagulant and antiplatelet medications, traditional laboratory measures of anticoagulation, a summary of thromboelastometry and thromboelastography technique, current evidence to support VET in pediatric ECMO/VAD, and limitations of VET in this population.
Overview of Anticoagulant Medications and Platelet Inhibitors In Ecmo/Vad
The ultimate goal of anticoagulation during extracorporeal support is to prevent thrombosis that occurs with exposure of blood to the nonendothelial surface of the ECMO or VAD circuit. Activation of the alternative pathway through the complement system and inflammatory cytokines stimulates both the intrinsic and extrinsic coagulation pathways with subsequent shift in balance to a procoagulant state in the circuit (4). With this in mind, the ideal anticoagulant would target inflammatory markers, platelet activation in addition to coagulant proteins without increasing bleeding risk. Unfortunately, today no such therapeutic option exists.
Anticoagulant Medications
Unfractionated heparin (UFH) remains the most widely used anticoagulant for neonatal and pediatric mechanical circulatory devices, specifically ECMO (5). UFH is a sulfated polysaccharide that produces its anticoagulant effect by inactivating free thrombin and activated factor X (FXa) by binding to antithrombin (AT), which induce a confirmational change in AT structure and enhance its serine protease inhibiting activity by 500–1000 folds. Heparin binds to AT by a high-affinity pentasaccharide, which is present on about a third of heparin molecules. Thrombin inhibition is dependent upon heparin binding to both the coagulation enzyme and AT; whereas inhibition of FXa is dependent only upon binding to AT (6). Thrombin bound to fibrin or subendothelium is not inhibited by the AT-UFH complex. UFH also has the ability to bind to plasma proteins, platelet factor 4, high molecular weight von Willebrand factor (vWF) and endothelial cells independently of AT which results in a variable response to heparin and even heparin resistance (6). In addition, these proteins are released during increased inflammation and activation of the coagulation system, leading to inefficient anticoagulant activity. The possibility of heparin induced thrombocytopenia (HIT) as well as wide interpatient variability are major shortcomings of UFH. Despite this, the use of UFH is well-known to treating clinicians with a short half-life and easy reversibility. Therapeutic efficacy of UFH can be measured by the activated clotting time (ACT), activated partial thromboplastin time (aPTT), anti-factor Xa activity (anti-FXa) and VET.
AT is a serine protease inhibitor necessary for heparin to exert its anticoagulant effect. Some centers include routine monitoring of AT and replacement with either fresh frozen plasma or AT concentrates. The rationale for the replacement of AT is to improve the effectiveness of heparin and reduce thrombotic complications. However, target AT levels remain unclear and guidelines for replacement with fresh frozen plasma (FFP) or AT concentrates vary significantly across centers. AT use remains controversial, as there is the potential risk of bleeding and added cost. A Cochrane review of 20 trials using AT replacement in critically ill patients showed no significant effect on mortality in all subgroup populations, including children, but had a 1.5-fold increased risk of bleeding (7). Until larger clinical trials can establish the effectiveness of AT replacement in pediatric ECMO/VAD, AT concentrates should be used with caution.
The use of direct thrombin inhibitors (DTIs) is becoming increasingly more common in the ECMO/VAD population, even in non-HIT scenarios. DTIs inhibit thrombin irrespective of whether the thrombin is free, or fibrin bound. Bivalirudin is the most commonly utilized direct thrombin inhibitor in ECMO and VADs. Bivalirudin is eliminated primarily by a non-organ mechanism (proteolysis) with only a minor (20%) component of renal clearance. Bivalirudin has the shortest half-life (about 25 min) and also has an intermediate affinity to thrombin in comparison to lepirudin (highest affinity) and argatroban (lowest affinity) (8).
Though studies are limited, bivalirudin thus far has been used effectively in both pediatric ECMO and VAD patients. In a single-center retrospective cohort, Seelhammer et al. evaluated a total of 422 ECMO patients (89 pediatric) and did not find an increase in adverse outcomes in the pediatric population. In the adult subgroup, bivalirudin was associated with improved overall survival (9). There is no consensus on bolus or continuous infusion dosing of bivalirudin. Loading dose has been reported as 0.4–0.5 mg/kg and continuous infusion rates from 0.05 to 0.5 mg/kg/h (10–12) in ECMO patients, with higher dosing reported in VADs (13). Pediatric studies are limited with mostly retrospective single-center data or small case series in the ECMO literature. Some pediatric programs have reported using bivalirudin as a first line agent with good outcomes; however, high-quality studies are lacking (11, 13–15). In the Berlin Heart EXCOR and Advanced Cardiac Therapies Improving Outcome Network (ACTION) post-approval surveillance study report, 92% of patients in the post surveillance group (post-market approval of EXCOR VAD) were transitioned to bivalirudin and also had decreased bleeding events, improved survival, lower stroke, and fewer thrombotic events (16).
Antiplatelet Agents
Antiplatelet agents are infrequently used during pediatric ECMO. The non-biologic surface of the ECMO circuit induces ongoing platelet activation and adhesion, which leads to platelet dysfunction (17, 18). Initiation of ECMO is associated with a decline in platelet count in neonates and pediatric patients. In a large multicenter study, the unadjusted association of average daily platelet count with mortality had a roughly linear association with the log odds of mortality up to a platelet count of approximately 115 × 109/L. On multivariable analyses, average daily platelet count was not independently associated with mortality however platelet transfusion volume was associated with mortality. This suggests that the platelet count was not the reason for increased mortality but factors associated with platelet transfusion (19). There is a paucity of data about adjunct antiplatelet therapy in pediatric ECMO patients with hypercoagulable states. Recently, two hypercoagulable COVID-19 adults were supported with venovenous (VV) ECMO and thromboelastography-guided antiplatelet therapy was initiated with good outcomes (20). In a case series of three pediatric patients with the multisystem inflammatory syndrome in children (MIS-C) supported with venoarterial (VA) ECMO, aspirin was used pre-ECMO cannulation due to coronary dilation. Interestingly, the only patient not treated with aspirin pre-cannulation had early circuit thrombosis requiring circuit change despite similar elevation in inflammatory markers in all patients (21). However, the Extracorporeal Life Support Organization (ELSO) registry data suggests similar frequency of thrombotic events in adult COVID-19 ECMO patients compared to the overall ELSO registry (22). Thus, there is no evidence to support routine use of antiplatelet medications in ECMO, however they may be beneficial in select cases.
Antiplatelet agents have been routinely used in the VAD population. The Edmonton anticoagulation and platelet inhibition protocol was the first widely utilized detailed guideline for pediatric VADs and included treatment with aspirin and clopidogrel (23). Antiplatelet therapy was guided by TEG®-platelet mapping. A more aggressive triple weight-based antiplatelet strategy was employed by Rosenthal et al. and this group reported 84% lower rate of stroke then the Edmonton guideline (24). With the more widespread use of DTIs for pediatric VAD patients, aspirin and dipyridamole are the most commonly used anti-platelet agents (3, 25, 26). A recent report using data from 10 centers affiliated with ACTION, showed lower stroke and major late bleeding in patients anticoagulated with DTIs. In this study, 88% of patients were also treated with antiplatelet agents, but dual antiplatelet therapy was used in less than a third of patients (27).
Traditional Laboratory Anticoagulant Monitoring In Ecmo/Vad
The assays used and monitoring frequency varies significantly between institutions and range from once daily to multiple time points in 24 h. In addition to the assays discussed below, hemoglobin/hematocrit, platelet count and fibrinogen levels are routinely screened. Transfusion thresholds also vary by institution and by the patient's clinical status. In general, hemostatic monitoring practice has moved from ACT only to multi-assay based monitoring (28).
Activated Clotting Time
The ACT is a whole blood, point-of-care test that measures the time it takes for clot formation using an intrinsic pathway activator. Advantageous to pediatrics, this point-of-care test is easy to perform with fast results and requires only a small amount of blood. Initially, this test was used during cardiopulmonary bypass where heparin dosing is much higher than what is used during ECMO. During ECMO, the dosing of heparin is lower and there has been poor correlation to the ACT (29). In conjunction, it is important to remember that the duration of ECMO is much longer (days to weeks) than that of cardiopulmonary bypass (hours) leading to a sustained hyperinflammatory state which may further interfere with anticoagulant monitoring. The ACT is also impacted by several other factors including hemodilution, hypothermia, coagulation factor deficiencies and platelet dysfunction, all of which may occur during ECMO therapy (30). Previous adult and pediatric ECMO studies have reported that the ACT had no correlation to UFH dose and was relatively insensitive to changes in UFH dose (31, 32). Despite these limitations, the ACT remains in broad use in up to 68 to 97% of institutions (5, 33). The most commonly reported and recommended goal ACT for ECMO is 180–220 s (28, 34, 35), but it is unclear if this reflects adequate anticoagulation for pediatric patients (36–38).
Activated Partial Thromboplastin Time
The aPTT is a plasma-based assay that evaluates the intrinsic and common factor pathways (factors XII, XI, IX, X, V, II and fibrinogen). This test is used to monitor anticoagulation effects of both UFH and direct thrombin inhibitors. aPTT testing is inexpensive and has the ability to detect underlying factor deficiencies (inherited or acquired), vitamin K deficiency or the presence of disseminated intravascular coagulation. However, the sensitivity of aPTT to heparin effect is both analyzer and method dependent and thus each laboratory must have their own determination of normal range. These reference ranges may change frequently due to variations in aPTT reagents between lots (39). Baseline aPTT vary significantly with age due to developmental hemostasis, and thus neonates and young children have different reference ranges than adult patients. In addition, there is an age-dependent effect of UFH on the prolongation of the aPTT (40). Generally, programs target an aPTT goal of 1.5–2.5 times baseline, which is based upon the normal range of the anti-factor Xa assay at most centers. Interestingly, there is a wide variation of reported aPTT values (in pediatric ECMO and VAD) that fall in the therapeutic window of anti-Xa (28, 40, 41). Considering developmental hemostasis in this age group and wide variation of aPTT ranges, using the aPTT for monitoring alone may be insufficient.
Anti-factor Xa Activity
Anti-FXa is another plasma-based assay that determines the anticoagulant activity of UFH by measuring the ability of heparin-bound AT to inhibit FXa. This assay is a photometric test; thus, it is important to note that hyperbilirubinemia, elevated triglyceride and heme levels interference may cause falsely low results. In addition, AT levels will influence the assay and results may also be inaccurate if the laboratory adds exogenous AT to the specimen (28). This is particularly important in neonates and young children, as these differences are likely to be more prominent (42). The main advantage of anti-FXa is that it directly measures the heparin effect rather than heparin concentration. Therapeutic levels are considered to be 0.3–0.7 IU/ml. Specifically, in mechanical circulatory devices, anti-FXa has been shown to correlate better with heparin dosing than the ACT (31), and in one study, an increase in heparin by 10 units resulted in a reproducible increase in anti-FXa level by 0.07 IU/ml (28). Thus, anti-FXa was better correlated with heparin effect than ACT which is poorly correlated with heparin dosing (32). Unfortunately, discordance between aPTT values and anti-FXa is common in pediatric ECMO and makes interpretation of these labs challenging (31).
Antithrombin
As previously discussed, some centers routinely monitor AT levels. AT has been reported to increase by about 1% for each ECMO day and is not related to daily volume of FFP transfusions. (28). Bembea et al. found that the median baseline AT activity for which patients received treatment was 43%, range 38–64%. AT levels were inversely correlated with the ACT, even after adjustment for heparin dose, but had a strong positive correlation to anti-FXa level (28).
Dilute Thrombin Time
The dilute thrombin time is a plasma-based assay that is used to determine the anticoagulant activity of DTIs, like bivalirudin. Here patient plasma is diluted with human fibrinogen or normal human plasma and then clotting is initiated by the addition of thrombin; the clotting time is then measured and compared to a laboratory generated standard reference curve (43). A recent study demonstrated that the aPTT and DTT have poor correlation in samples from pediatric VAD patients (44). Because the DTT is not affected by heparin, lupus anticoagulants or vitamin-K dependent factors it may be superior for monitoring anticoagulant activity; however, studies are limited and it remains unclear if the DTT is preferred over the aPTT for monitoring (43).
Viscoelastic Tests
Limitations in traditional laboratory testing have led some centers to adopt monitoring programs focused on multiple measures of hemostasis. Thromboelastography (TEG®) (Haemonetics Corp, Braintree, MA, USA) and thromboelastometry (ROTEM®) (Tem International GmbH, Munich, Germany) are two VETs used to assess the underlying hemostatic potential from initial clot formation through fibrinolysis in whole blood. The technology was first developed in the late 1940's and has shown benefit in monitoring coagulopathy in cardiac surgery and liver transplant (45–47). In the pediatric cardiac surgical population, VET-based transfusion algorithms for bleeding patients have led to decreased blood loss and transfusion requirements. VET is now recommended as an alternative to standard assays for managing intraoperative bleeding (48). Recently this testing has been applied to even more clinical states, including inherited coagulation disorders and in ECMO and VAD. VET can provide a more global view of hemostasis in pediatric ECMO/VAD by displaying all phases of coagulation. Figure 1 depicts the graphical tracing produced by TEG® and ROTEM®.
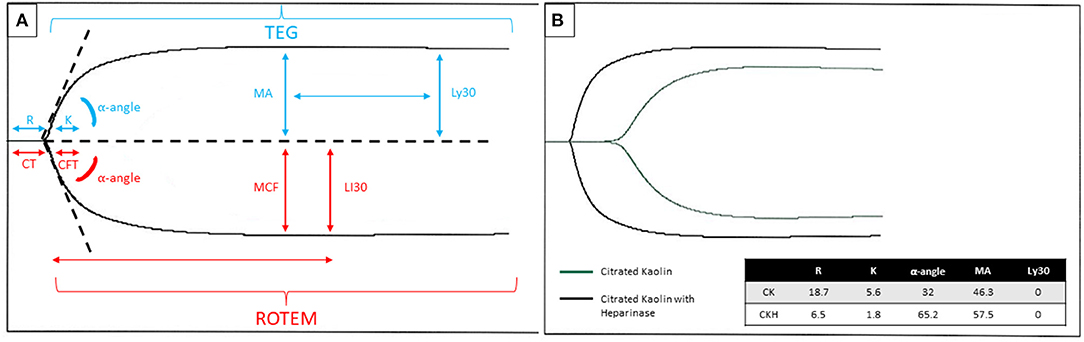
Figure 1. (A) Graphical tracing produced by the TEG® (blue font color)/ROTEM® (red font color) with parameters labeled for each respective test. The R/CT and K/CFT may be affected by heparin or other anticoagulants and coagulation factor deficiencies. The MA/MCF may be affected by fibrinogen level, absolute platelet count, or platelet dysfunction. The Ly30/LI30 may be affected by hyperfibrinolytic states or inherited/acquired factor XIII deficiency. (B) An example of a TEG® tracing in a patient on ECMO with citrated kaolin +/- heparinase. Legend: Blue arrow depicts the timepoint to measure Ly30, measured at 30 min after the MA. The red arrow shows the time point to measure LI30, measured at 30 min after the CT. R, reaction time, CT, clotting time; K, kinetic time; CFT, clot formation time; MA, maximum amplitude; MCF, maximum clot firmness; Ly30, lysis time 30 min after maximum amplitude; LI30, lysis time 30 min after clotting time; CK, citrated kaolin; CKH, citrated kaolin with heparinase.
VET Techniques
TEG®
Today, there are two different technologies used to run TEG®, the TEG® 5000 and the TEG® 6S (Figure 2). The TEG® 5000 can analyze two samples simultaneously, using a fixed pin on a torsion wire suspended into an oscillating cylindrical cup containing a small amount of whole blood. The most commonly used activators include citrated kaolin, kaolin and tissue factor. As the first fibrin strands form and clot strength increases, more rotation is transmitted on the torsion wire, which is detected by an electromagnetic transducer (49, 50). The technique requires fine-tuned expertise for reproducible results but may also be adversely affected by the need for multiple blood sample transfers and vibration or movement of the machine.
The TEG® 6S was developed to overcome barriers seen with the TEG® 5000. TEG® 6S is an automated cartridge-based technology that uses a 4-channel microfluidic system containing the required reagents (citrated kaolin, citrated kaolin + heparinase, kaolin and kaolin + heparinase) and automatically aspirates citrated blood into the different test chambers (50). The system is compact with easy portability and requires only one blood sample transfer from the operator into the cartridge. In this test, as the clot formation and propagation occur, the clot is exposed to the high-frequency ultrasound pulses; with clot strength being measured by a resonance method (50).
Both systems have the ability to assess platelet function through the TEG® Platelet Mapping® (TEG®PM) (Haemonetics Corp, Braintree, MA, USA) assay. The assay assesses degree of platelet inhibition by comparison of the patient's full hemostatic potential and the difference when looking at the contribution of platelets through activation of specific receptors [e.g., adenosine diphosphate (ADP) and arachidonic acid (AA)].
Automated TEG® variables are described in Table 1. In addition to these parameters, several TEG® derived parameters can assist with overall thrombotic potential, including the clotting index (CI) and the shear modulus strength (G). The CI is a global assessment of clot formation that uses a linear combination of the R-time, K-time, MA and alpha angle. Using the amplitude at the MA variable, G is calculated in Kdynes/cm2 and represents maximum clot strength.
ROTEM®
The thromboelastometry (ROTEM®) system utilizes a fixed cylindrical cup containing a small amount of whole blood while a pin oscillates through application of a constant force (Figure 2). As clot strength increases, the rotating pin is obstructed and is detected optically using a charge-coupled device image sensor system. A standard ROTEM® device can analyze four samples simultaneously and utilizes automated pipetting (49). The ROTEM® provides the same information as the TEG® but uses different terminology (Table 1). ROTEM® may also be reported based on the activator used (Table 2). It is important to note that although the same information is achieved, the results are not interchangeable as the mechanisms of each assay are different.
Interpretation in Clinical Practice
Most commonly, the samples obtained for VET are collected in sodium citrate tubes which necessitates timely processing. Standard values have been reported in healthy children and in children with congenital heart disease (51, 52). However, it has been reported that the use of samples collected in this way may result in significant artifacts on TEG® analysis and could lead to heparin overdosing in these patients (53). In addition, discrepant results have been reported using TEG® 5000, TEG® 6S and ROTEM® on the same whole blood sample in orthotopic liver transplant and trauma patients; although all three tests could identify abnormalities in clot formation (50, 54). If TEG® 6S provides an accurate assessment of coagulopathy in the ECMO and VAD population, its use at the bedside would allow for point of care results and decreased need for skilled laboratory personnel.
Another benefit of VET testing is the value in patients anticoagulated with DTIs. Dilute thrombin time is not readily available in many institutions and standard laboratory monitoring may not accurately reflect anticoagulant effect of DTIs. TEG® ecarin clotting time has shown excellent correlation with chromogenic bivalirudin levels, r2 = 0.746 (55). In a pediatric study including 106 blood samples of children supported with ECMO on bivalirudin, prolongation of clotting time on ROTEM intrinsic coagulation pathway (INTEM) and INTEM with heparinase (HEPTEM) showed moderate to strong correlation with aPTT and hepzyme aPTT suggesting that ROTEM may provide a good alternative to those standard assays. In this report, the target range of INTEM CT was 260–300 s for children without evidence of heparin-like activity anticoagulated with bivalirudin during ECMO (56).
Management of anticoagulation is predominantly assessed by using the R-time (TEG®) or CT (ROTEM®) and comparing the values with and without the presence of heparin. Reference ranges are based upon individual lab standardization. In an adult randomized controlled trial, anticoagulation using aPTT based protocol compared to TEG® based protocol targeting TEG®-Kaolin R-time 16–24 min (normal value: 4–8 min) found that TEG based titration was safe, feasible, and resulted in lower heparin dose compared to aPTT based protocol (57). A pediatric retrospective study found that anti-FXa activity > 0.25 IU/ml (sensitivity 81%, specificity 67%, PPV 81%, and NPV 58%) and a TEG® R-time > 17.85 min (sensitivity 84%, specificity 68%, PPV 82%, and NPV 59%) may minimize the risk of thrombosis in pediatric and neonatal ECMO patients but could not find an optimal target to minimize bleeding (58). In general, programs target somewhere between 2 and 4 times the baseline TEG® R-time. In the event of incomplete correction of the R/CT time with the addition of heparinase, it may suggest coagulation factors deficiency and consideration may be given to replacement with FFP based on presence or absence of clinical bleeding. In addition, some patients develop acquired von Willebrand deficiency or other clotting factor deficiencies due to consumption in the circuit and abnormalities can prompt additional laboratory testing to identify deficiencies. Clot strength as assessed by the MA/MCF is influenced by fibrinogen, absolute platelet count and function. Increased lysis time may be indicative of hyperfibrinolysis (circuit disseminated intravascular coagulopathy or acquired factor XIII deficiency). Importantly, viscoelastic tests may help diagnose covert coagulopathy that may not be identified on standard laboratory assays and could contribute to bleeding risk (59).
Evidence to Support VET Use in ECMO and VAD
Although limited, studies evaluating the use of VET in adult ECMO and VAD have increased over the last decade. Pediatric ECMO/VAD studies have reported VET-based anticoagulation algorithms and associations with clinical outcomes including bleeding and thrombosis. Figures 3, 4 describe the clinical features and TEG®/ROTEM® parameters that have been associated with increased bleeding and thrombotic complications. In a recent report using data from 265 children in the Pediatric ECMO Outcomes Registry (PEDECOR) VET was utilized in of centers contributing data, although bleeding and thrombotic complications were not different between centers utilizing VET compared to centers that did not (60). We review these pediatric studies below and in Table 3.
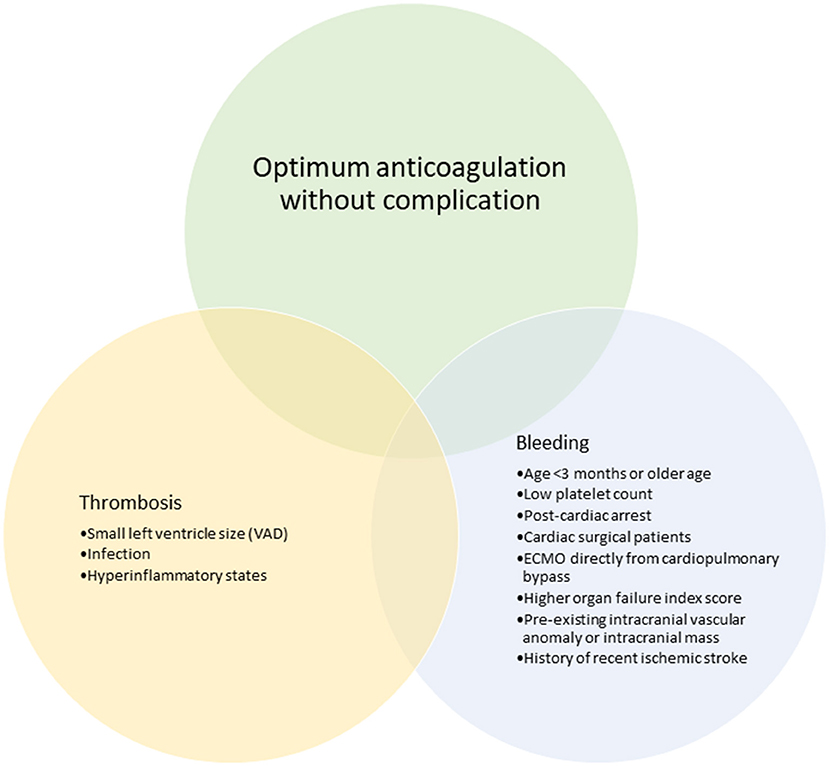
Figure 3. Clinical features that have been associated with bleeding and thrombotic complications in pediatric ECMO and VAD.
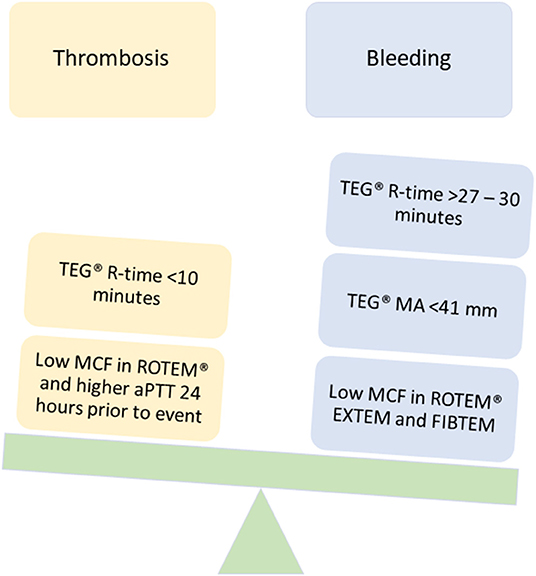
Figure 4. TEG®/ROTEM® parameters that have been associated with bleeding and thrombotic complications in pediatric ECMO and VAD.
VET Impact on Bleeding
Bleeding is the most commonly encountered hemostatic complication during ECMO/VAD. Adult studies of VET to predict and prevent bleeding have reported mixed results. Panigada et al. randomized patients to a TEG®-based protocol vs. a standard of care aPTT-based protocol and found more anticoagulation dosing adjustments were made in the TEG® group, lower dose of heparin was administered, and target parameters were less frequently in therapeutic range in the TEG® group. Despite these findings, there were no significant differences in hemostatic complications or transfusion support (57). Conversely, a recent retrospective cohort study in adult ECMO patients compared pre-protocol (aPTT alone, goal 60–80 s) to post-protocol (aPTT, goal 60–80 s + TEG®, goal R-time 2–4 times baseline) and reported that overall bleeding complications were similar, but that there was a significant decrease in retroperitoneal bleeding events and that overall mortality during ECMO support was significantly lower in the post-protocol group (75).
In a meta-analysis on the impact of point-of-care testing in adult and pediatric ECMO patients, the use of VET was associated with lower in-hospital mortality and decreased bleeding events, but this was not statistically significant. There was a significant reduction in the frequency of surgical revision for bleeding (76). Hypocoagulability and bleeding complications have been correlated with ROTEM® in both ECMO/VAD. A prospective study including 24 ECMO and 23 VAD adult patients found that a decreased maximum clot firmness (MCF) in EXTEM and FIBTEM was associated with severe bleeding, and decreased MCF in FIBTEM was associated with increased 30-day mortality risk (65).
Clinical data supporting VET specific to the neonatal and pediatric population are limited to a few prospective studies. In pediatric ECMO, most reports are retrospective chart reviews utilizing TEG® and TEG®PM. In CDH neonates, the addition of TEG® for monitoring showed a significant reduction in hemothoraces and improved blood product utilization (66). However, correlation of the TEG® parameters to other monitoring tests (ACT and aPTT) are challenging due to the variability of heparin effect. Alexander et al. found a weak correlation of the TEG® heparinase variables and the aPTT/ACT, but a stronger correlation between the TEG® MA and platelet count (62). In the PEDECOR study, VET identified platelet dysfunction most commonly and bleeding patients who had VET performed received more cryoprecipitate transfusions than those who did not have VET (60). A chart review of children <18 years on ECMO looked at severe platelet dysfunction (<50% aggregation on TEG®PM) and bleeding. Severe platelet dysfunction was more common for ADP (92%) vs. arachidonic acid (75%) but no difference in the TEG® parameters between the bleeding and non-bleeding group. However, the absolute platelet count and TEG®PM had increased predictive value for severe bleeding and mortality compared to monitoring with ACT (69). Although TEG®PM is used less frequently in ECMO, this study suggests it may aid in prevention of severe bleeding. Importantly, TEG® has been reported to aid in detection and management of hyperfibrinolysis (77). Similar findings have been reported with the use of ROTEM® in pediatrics. A retrospective observational cohort study, including 73 pediatric patients found no association between bleeding complications and results of the ROTEM®, aPTT, and anti-FXa. However, the multivariate analysis showed that surgical intervention and age <29 days significantly increased the incidence of major bleeding events (68). Conversely, as mentioned above, Teruya et al. found that the ROTEM® CT on INTEM and HEPTEM had a moderate to strong correlation with the aPTT and HaPTT for children receiving bivalirudin for anticoagulation which may be a major application of VET for pediatric ECMO patients (56).
VET Impact on Thrombosis
Thrombotic complications can range from mild to severe and have detrimental effects on patient outcomes. Conventional monitoring tests are associated with significant variability and have not been shown to reliably correlate with thrombosis. Unfortunately, to date the correlation of VET with thrombosis has also been limited (65, 76). A recent retrospective pediatric ECMO cohort study reported reduced MCF (in all ROTEM® components) and higher minimum aPTT 24 h was associated with increased thrombotic risk (68). While limited, this study suggests that ROTEM® could be more predictive of thrombotic risk than aPTT and in this scenario could aid clinicians in assessment of thrombotic risk and reevaluation of targeted management/prevention.
VET has been applied more frequently in the pediatric VAD population. Dosing of dipyridamole and aspirin were found to increase across all ages with increasing duration of support, which may indicate progressive inflammation, vasculopathy or adverse thrombotic events (3). TEG® or ROTEM® with platelet mapping has been used to identify inadequate platelet inhibition and its routine use may allow prevention of thombotic complications (78). Unfortunately, the clinical utility of TEG®PM has been questioned due to technical challenges resulting in variable test results and validity must be confirmed by visual interpretation of the generated curve within certain criteria (79). This makes the application of TEG®PM limited in universal application and more reliant on experienced specialist interpretation that may not be available at all centers. Despite these limitations, in the ACTION DTI harmonization protocol, aspirin effect is monitored either by TEG®PM or the functional assay VerifyNow which evaluates platelet aggregation by a turbidimetric-based optical detection (80).
VET Impact During COVID-19
Severe COVID-19 respiratory illness has a significant impact on hemostatic balance and the systemic inflammation seen in these patients contributes to a hypercoagulable state. Yuriditsky et al. reported that in severe COVID-19 illness, the TEG® was able to reliably identify this hypercoagulable state. The alpha angle and MA were elevated in 70 and 60%, respectively. The clotting index was hypercoagulable (>3) in 50% of patients with severe COVID-19 and 31% of these patients suffered from thromboembolic events. The correlations between TEG® and traditional inflammatory/coagulation laboratory values was weak to moderate suggesting that TEG® was better able to predict thromboembolic event then routine laboratory tests (81). Subtle changes in TEG® or ROTEM® variables may precede changes in routine laboratory values (i.e., fibrinogen) and clinical picture (i.e., hyperfibrinolysis) before a major hemostatic event occurs (82). Specifically, the use of VET during COVID-19 adult ECMO has found associations with d-dimer, fibrinogen and TEG® MA values and arrest of fibrinolysis as demonstrated by the TEG® Ly30 (20, 83, 84).
In pediatric patients with acute COVID-19 infection, one small case series reported ROTEM® parameters associated with hypercoagulable state but no clinically significant thrombotic events (85). In pediatric MIS-C, a single center report (n = 30) showed patients had evidence of hypercoagulability on TEG® but similarly did not demonstrate any symptomatic thrombotic event or major bleeding (86). In a single center cohort (n = 22) of pediatric patients with acute COVID-19 and MIS-C, TEG® uncovered a pattern of accelerated clot formation and increased clot strength in a subset of children and unlike the previous reports this cohort included two patients with significant thrombotic complications (87).
Limitations of VET in Pediatric ECMO/VAD
While the use of VET in pediatric ECMO/VAD has increased, limitations exist. Importantly, technical considerations, including skills to properly maintain the device and expert proficiency in handling blood samples, are needed for reliable results. After sample procurement, activation of the sample is performed. In TEG® and ROTEM, contact activation (intrinsic coagulation pathway) using ellagic acid or kaolin can provide information like activated partial thromboplastin time. While tissue factor activation (extrinsic coagulation pathway) provides information like prothrombin time. Lyophilized heparinase neutralization of heparin, blocking of platelet contribution to clot formation with platelet inhibitors, or inhibiting fibrinolysis with antifibrinolytics can be used. Thus, activators for both the intrinsic and extrinsic systems are available. When celite and kaolin are used as activators for TEG® in the presence of aprotinin, there is a prolongation of R time, a decrease in α angle, and a decrease in MA, suggesting that these are not ideal activators for a TEG®-guided transfusion algorithm (88). TEG® has a poor correlation with frequently used laboratory testing and has not been shown to predict bleeding or thrombosis during ECMO. Currently, the cut-off values based on TEG® and ROTEM-based parameters are not well established for pediatric patients. In addition, results should be interpreted by an experienced clinician who understands the intricacies of the test and variables affecting the hemostatic system. Today, the amount of blood required to run the VET is small. Still, it may be problematic in the neonatal population, especially those awaiting cardiac transplantation, where it is essential to limit blood product exposure. Another limitation is that von Willebrand deficiency cannot reliably be detected using VETs. In addition to all of these factors, the child's clinical status is the most critical variable, and decisions based on VET/laboratory tests may not reflect the dynamic changes in the critically ill child.
Future Considerations
With clot development, the state of blood changes from a viscous fluid to a semisolid gel, and these changes can be detected by sonic estimation of elasticity via resonance (SEER) sonorheometry (Quantra™). This newer ultrasound-based VET uses high frequency ultrasound pulses to characterize the dynamic changes in viscoelastic properties of a blood sample during coagulation. Clot stiffness as measured by SEER have shown strong positive correlations in TEG® and ROTEM® looking at G and FIBTEM, respectively; as well as a strong correlation to fibrinogen and platelet count (89, 90).
Another area under investigation is the use of a shear gradient activated microfluidic approach. The microfluidic device mimics stenosed arteriolar vessels, allowing for the assessment of thrombotic potential within small sample volumes under pathophysiologic flow. This technology has shown when integrated into the extracorporeal circuit in pig endotoxemia or heparin therapy models, it reports real time data of alterations in coagulation ex vivo that are more reliable than standard coagulation assays (e.g., ACT and aPTT) (91). The introduction of this technology into human mechanical circuits could kickstart the possibility of personalized diagnostics and minute-to-minute real-time surveillance of the hemostatic balance and reduce need for blood draws. Unfortunately, this technology is currently available only for research studies or in limited academic institutions.
Conclusions
The use of VET for guiding anticoagulation management in pediatric ECMO and VAD is increasing. Studies are limited in this population and no high quality data supports VET algorithms over standard coagulation testing. However, VETs may provide benefit in detecting subtle changes in this high-risk population. Incorporation of these assays into general monitoring practice may aid in precision anticoagulant management. VET use in COVID-19 is promising. Additional studies are needed in the pediatric ECMO and VAD population to determine optimal role of VET.
Author Contributions
KR wrote the manuscript draft and finalized changes after revision and approval. AS and KC revised, read and approved final manuscript.
Conflict of Interest
The authors declare that the research was conducted in the absence of any commercial or financial relationships that could be construed as a potential conflict of interest.
The reviewer HJD declared a past co-authorship with the author KC to the handling editor.
Publisher's Note
All claims expressed in this article are solely those of the authors and do not necessarily represent those of their affiliated organizations, or those of the publisher, the editors and the reviewers. Any product that may be evaluated in this article, or claim that may be made by its manufacturer, is not guaranteed or endorsed by the publisher.
Abbreviations
AA, Arachidonic acid; ACT, Activated clotting time; ACTION, Advanced cardiac therapies improving outcome network; ADP, Adenosine diphosphate; Anti-FXa, Anti-factor Xa; aPTT, Activated partial thromboplastin time; AT, Antithrombin; CT, Clotting time; DTI, Direct thrombin inhibitor; DTT, Dilute thrombin time; ECMO, Extracorporeal membrane oxygenation; ECPR, Extracorporeal cardiopulmonary resuscitation; EXTEM, Extrinsic thromboelastometry; FFP, Fresh frozen plasma; FIBTEM, Functional fibrinogen thromboelastometry; FXa, Factor Xa; HaPTT, Heparinase activated partial thromboplastin time; HEPTEM, Heparinase thromboelastometry; HIT, Heparin induced thrombocytopenia; INTEM, Intrinsic thromboelastometry; K-time, Kinetic time; Ly30, Clot lysis time; MA, Maximum amplitude; MCF, Maximum clot firmness; MIS-C, Multisystem inflammatory syndrome in children; R-time, Reaction time; ROTEM®, Thromboelastometry; TEG®, Thromboelastography; TEG®PM, Thromboelastography with platelet mapping; UFH, Unfractionated heparin; VA, Venoarterial; VAD, Ventricular assist device; VET, Viscoelastic testing; VV, Venovenous; vWF, von Willebrand factor.
References
1. ECLS Registry Report International Summary. Available onilen at: https://www.elso.org/Registry/Statistics/InternationalSummary.aspx (accessed October 18, 2021).
2. Dalton HJ, Reeder R, Garcia-Filion P, Holubkov R, Ber RA, Zuppa A, et al. Factors associated with bleeding and thrombosis in children receiving extracorporeal membrane oxygenation (ECMO). Am J Respir Crit Care Med. (2017) 196:762–71. doi: 10.1164/rccm.201609-1945OC
3. Steiner ME, Bomgaars LR, Massicotte MP. Antithrombotic therapy in a prospective trial of a pediatric ventricular assist device. ASAIO J. (2016) 62:719–27. doi: 10.1097/MAT.0000000000000434
4. Saini A, Spinella PC. Management of anticoagulation and hemostasis for pediatric extracorporeal membrane oxygenation. Clin Lab Med. (2014) 34:655–73. doi: 10.1016/j.cll.2014.06.014
5. Bembea MM, Annich G, Rycus P, Oldenburg G, Berkowitz I, Pronovost P. Variability in anticoagulation management of patients on extracorporeal membrane oxygenation: an international survey. Pediatr Crit Care Med. (2013) 14:1–15. doi: 10.1097/PCC.0b013e31827127e4
6. Hirsh J, Anand SS, Halperin JL, Fuster V. Mechanism of action and pharmacology of unfractionated heparin. Arterioscler Thromb Vasc Biol. (2001) 21:1094–6. doi: 10.1161/hq0701.093686
7. Afshari A, Wetterslev J, Brok J, Møller A. Antithrombin III in critically ill patients: systematic review with meta-analysis and trial sequential analysis. Br Med J. (2007) 335:1248–51. doi: 10.1136/bmj.39398.682500.25
8. Warkentin TE, Greinacher A, Koster A. Bivalirudin. Thromb Haemost. (2008) 99:830–9. doi: 10.1160/TH07-10-0644
9. Seelhammer TG, Bohman JK, Schulte PJ, Hanson AC, Aganga DO. Comparison of bivalirudin versus heparin for maintenance systemic anticoagulation during adult and pediatric extracorporeal membrane oxygenation. Crit Care Med. (2021) 49:1481–92. doi: 10.1097/CCM.0000000000005033
10. Sanfilippo F, Asmussen S, Maybauer DM, Santonocito C, Fraser JF, Erdoes G, et al. Bivalirudin for alternative anticoagulation in extracorporeal membrane oxygenation: a systematic review. J Intensive Care Med. (2017) 32:312–9. doi: 10.1177/0885066616656333
11. Kaushik S, Derespina KR, Chandhoke S, Shah DD, Cohen T, Shlomovich M, et al. Use of bivalirudin for anticoagulation in pediatric extracorporeal membrane oxygenation (ECMO). Perfus. (2021) 2676591211034314. doi: 10.1177/02676591211034314
12. Nagle EL, Dager WE, Duby JJ, Roberts AJ, Kenny LE, Murthy MS, et al. Bivalirudin in pediatric patients maintained on extracorporeal life support. Pediatr Crit Care Med. (2013) 14:e182–8. doi: 10.1097/PCC.0b013e31827200b6
13. Campbell CT, Diaz L, Kelly B. Description of bivalirudin use for anticoagulation in pediatric patients on mechanical circulatory support. Ann Pharmacother. (2021) 55:59–64. doi: 10.1177/1060028020937819
14. Hamzah M, Jarden AM, Ezetendu C, Steward R. Evaluation of bivalirudin as an alternative to heparin for systemic anticoagulation in pediatric extracorporeal membrane oxygenation. Pediatr Crit Care Med. (2020) 21:827–34. doi: 10.1097/PCC.0000000000002384
15. Schill MR, Douds MT, Burns EL, Lahart MA, Said AS, Abarbanell AM. Is anticoagulation with bivalirudin comparable to heparin for pediatric extracorporeal life support? Results from a high-volume center. Artif Organs. (2021) 45:15–21. doi: 10.1111/aor.13758
16. Zafar F, Conway J, Bleiweis MS, Al-Aklabi M, Ameduri R, Barnes A, et al. Berlin Heart EXCOR and ACTION post-approval surveillance study report. J Hear Lung Transplant. (2021) 40:251–9. doi: 10.1016/j.healun.2021.01.010
17. Vroman L, Adams A, Fischer G, Munoz P. Interaction of high molecular weight kininogen, factor XII, and fibrinogen in plasma at interfaces. Blood. (1980) 55:156–9. doi: 10.1182/blood.V55.1.156.156
18. Wendel HP, Ziemer G. Coating-techniques to improve the hemocompatibility of artificial devices used for extracorporeal circulation. Eur J Cardio-thoracic Surg. (1999) 16:342–50. doi: 10.1016/S1010-7940(99)00210-9
19. Cashen K, Dalton H, Reeder RW, Saini A, Zuppa AF, Shanley TP, et al. Platelet transfusion practice and related outcomes in pediatric extracorporeal membrane oxygenation. Pediatr Crit Care Med. (2020) 21:178–85. doi: 10.1097/PCC.0000000000002102
20. Smolarz A, McCarthy P, Shmookler A, Badhwar V, Hayanga AJ, Sakhuja A. Utilization of thromboelastogram and inflammatory markers in the management of hypercoagulable state in patients with COVID-19 requiring ECMO support. Case Reports Crit Care. (2021) 2021:3–6. doi: 10.1155/2021/8824531
21. Schneider J, Tilford B, Safa R, Dentel J, Veenstra M, Ang J, et al. Extracorporeal membrane oxygenation for multisystem inflammatory syndrome in children. Perfus. (2021) 26:2676591211020904. doi: 10.1177/02676591211020904
22. Barbaro RP, MacLaren G, Boonstra PS, Jwashyna TJ, Slutsky AS, Fan E, et al. Extracorporeal membrane oxygenation support in COVID-19: an international cohort study of the extracorporeal life support organization registry. Lancet. (2020) 396:1071–8. doi: 10.1016/S0140-6736(20)32008-0
23. Morales DLS, Almond CSD, Jaquiss RDB, Rosenthal DN, Naftel DC, Massicotte MP, et al. Bridging children of all sizes to cardiac transplantation: the initial multicenter North American experience with the Berlin Heart EXCOR ventricular assist device. J Hear Lung Transplant. (2011) 30:1–8. doi: 10.1016/j.healun.2010.08.033
24. Rosenthal DN, Lancaster CA, McElhinney DB, Chen S, Stein ML, Lin A, et al. Impact of a modified anti-thrombotic guideline on stroke in children supported with a pediatric ventricular assist device. J Hear Lung Transplant. (2017) 36:1250–7. doi: 10.1016/j.healun.2017.05.020
25. Emani S, Trainor B, Zurakowski D, Baird CW, Fynn-Thompson FE, Pigula FA, et al. Aspirin unresponsiveness predicts thrombosis in high-risk pediatric patients after cardiac surgery. J Thorac Cardiovasc Surg. (2014) 148:810–6. doi: 10.1016/j.jtcvs.2014.06.016
26. Huang JY, Monagle P, Massicotte MP, VanderPluym CJ. Antithrombotic therapies in children on durable ventricular assist devices: a literature review. Thromb Res. (2018) 172:194–203. doi: 10.1016/j.thromres.2018.02.145
27. Vanderpluym CJ, Cantor RS, MacHado D, Boyle G, May L, Griffiths E, et al. Utilization and outcomes of children treated with direct thrombin inhibitors on paracorporeal ventricular assist device support. ASAIO J. (2020) 66:939–45. doi: 10.1097/MAT.0000000000001093
28. Bembea MM, Schwartz J, Shah N, Colantuoni E, Lehmann C, Kickler T, et al. Anticoagulation monitoring during pediatric extracorporeal membrane oxygenation. ASAIO J. (2013) 59:63–8. doi: 10.1097/MAT.0b013e318279854a
29. Owings JT, Pollock ME, Gosselin RC, Ireland K, Jahr JS, Larkin EC. Anticoagulation of children undergoing cardiopulmonary bypass is overestimated by current monitoring techniques. Arch Surg. (2000) 135:1042–7. doi: 10.1001/archsurg.135.9.1042
30. Horton S, Augustin S. Activated clotting time (ACT). In: Monagle P, editor. Haemostasis Methods in Molecular Biology (Methods and Protocols), Vol 992. Totawa, NJ: Humana Press (2013). doi: 10.1007/978-1-62703-339-8_12
31. Liveris A, Bello RA, Friedmann P, Duffy MA, Manwani D, Killinger JS, et al. Anti-factor Xa assay is a superior correlate of heparin dose than activated partial thromboplastin time or activated clotting time in pediatric extracorporeal membrane oxygenation. Pediatr Crit Care Med. (2014) 15:72–9. doi: 10.1097/PCC.0000000000000028
32. Delmas C, Jacquemin A, Vardon-Bounes F, Georges B, Guerrero F, Hernandez N, et al. Anticoagulation Monitoring Under ECMO Support: A Comparative Study Between the Activated Coagulation Time and the Anti-Xa Activity Assay. J Intensive Care Med. (2020) 35:679–86. doi: 10.1177/0885066618776937
33. Ozment CP, Scott BL, Bembea MM, Spinella PC, Pediatric Pediatric ECMO (PediECMO) subgroup of the Pediatric Acute Lung Injury and Sepsis Investigators (PALISI) Network and the Extracorporeal Life Support Organization (ELSO). Anticoagulation and transfusion management during neonatal and pediatric extracorporeal membrane oxygenation: a survey of medical directors in the United States. Pediatr Crit Care Med. (2021) 22:530–41. doi: 10.1097/PCC.0000000000002696
34. Drop JGF, Wildschut ED, Gunput STG, de Hoog M, van Ommen CH. Challenges in maintaining the hemostatic balance in children undergoing extracorporeal membrane oxygenation: a systematic literature review. Front Pediatr. (2020) 8:1–11. doi: 10.3389/fped.2020.612467
35. Giorni C, Rizza A, Favia I, Amodeo A, Chiusolo F, Picardo SG, et al. Pediatric mechanical circulatory support: pathophysiology of pediatric hemostasis and available options. Front Cardiovasc Med. (2021) 8:1–14. doi: 10.3389/fcvm.2021.671241
36. Baird CW, Zurakowski D, Robinson B, Gandhi S, Burdis-Koch L, Tamblyn J, et al. Anticoagulation and pediatric extracorporeal membrane oxygenation: impact of activated clotting time and heparin dose on survival. Ann Thorac Surg. (2007) 83:912–20. doi: 10.1016/j.athoracsur.2006.09.054
37. Keogh J, Davidson SJ, Machin M, Hall J, Kelleher AA. Heparin concentrations in neonates during cardiopulmonary bypass as measured by the activated clotting time and anti-Xa assay. Anaesthesia. (2009) 64:1388–9. doi: 10.1111/j.1365-2044.2009.06169_3.x
38. Stocker CF, Horton SB. Anticoagulation strategies and difficulties in neonatal and paediatric extracorporeal membrane oxygenation (ECMO). Perfus. (2016) 31:95–102. doi: 10.1177/0267659115590626
39. Marlar RA, Clement B, Gausman J. Activated partial thromboplastin time monitoring of unfractionated heparin therapy: issues and recommendations. Semin Thromb Hemost. (2017) 43:253–60. doi: 10.1055/s-0036-1581128
40. Kamdar A, Rintoul N, Raffini L. Anticoagulation in neonatal ECMO. Semin Perinatol. (2018) 42:122–8. doi: 10.1053/j.semperi.2017.12.008
41. Murphy DA, Hockings LE, Andrews RK, Aubron C, Gardiner EE, Pellegrino VA, et al. Extracorporeal membrane oxygenation-hemostatic complications. Transfus Med Rev. (2015) 29:90–101. doi: 10.1016/j.tmrv.2014.12.001
42. Ignjatovic V, Summerhayes R, Gan A, Than J, Chan A, Cochrane A, et al. Monitoring unfractionated heparin (UFH) therapy: which anti factor Xa assay is appropriate? Thromb Res. (2007) 120:347–51. doi: 10.1016/j.thromres.2006.10.006
43. Love JE, Ferrell C, Chandler WL. Monitoring direct thrombin inhibitors with a plasma diluted thrombin time. Thromb Haemost. (2007) 98:234–42. doi: 10.1160/TH06-10-0607
44. Engel E, Losos M, Martin J, Palumbo JS, Lorts A, Geer R, et al. Use of bivalirudin-specific monitoring assays in ventricular assist device patients. Blood. (2021) 138:3236. doi: 10.1182/blood-2021-148028
45. De Pietri L, Ragusa F, Deleuterio A, Begliomini B, Serra V. Reduced transfusion during OLT by POC coagulation management and TEG functional fibrinogen. Transplant Direct. (2016) 2:e49. doi: 10.1097/TXD.0000000000000559
46. Kane LC, Woodward CS, Husain SA, Frei-Jones MJ. Thromboelastography-does it impact blood component transfusion in pediatric heart surgery? J Surg Res. (2015) 200:21–7. doi: 10.1016/j.jss.2015.07.011
47. Romlin BS, Wåhlander H, Berggren H, Synnergren M, Baghaei F, Nilsson K, et al. Intraoperative thromboelastometry is associated with reduced transfusion prevalence in pediatric cardiac surgery. Anesth Analg. (2011) 112:30–6. doi: 10.1213/ANE.0b013e3181fe4674
48. Faraoni D, Meier J, New H V, Van der Linden PJ, Hunt BJ. Patient blood management for neonates and children undergoing cardiac surgery: 2019 NATA guidelines. J Cardiothorac Vasc Anesth. (2019) 33:3249–63. doi: 10.1053/j.jvca.2019.03.036
49. Whiting D, Dinardo JA. TEG and ROTEM: technology and clinical applications. Am J Hematol. (2014) 89:228–32. doi: 10.1002/ajh.23599
50. Robson JL, Watts ADJ, McCulloch TJ, Paleologos MS, Mortimer RA, Kam PCA. Correlation and agreement between the teg® 5000 and the teg® 6s during liver transplant surgery. Anaesth Intensive Care. (2019) 47:32–9. doi: 10.1177/0310057X18811731
51. Miller BE, Bailey JM, Mancuso TJ, Weinstein MS, Holbrook GW, Silvey EM, et al. Functional maturity of the coagulation system in children: an evaluation using thrombelastography. Anesth Analg. (1997) 84:745–8. doi: 10.1213/00000539-199704000-00008
52. Rizza A, Ricci Z, Pezzella C, Favia I, Di Felice G, Ranucci M, et al. Kaolin-activated thromboelastography and standard coagulation assays in cyanotic and acyanotic infants undergoing complex cardiac surgery: a prospective cohort study. Paediatr Anaesth. (2017) 27:170–80. doi: 10.1111/pan.13038
53. Gilman EA, Koch CD, Santrach PJ, Schears GJ, Karon BS. Fresh and citrated whole-blood specimens can produce different thromboelastography results in patients on extracorporeal membrane oxygenation. Am J Clin Pathol. (2013) 140:165–9. doi: 10.1309/AJCPYIQ9JNNSEN4Q
54. Neal MD, Moore EE, Walsh M, Thomas S, Callcut RA, Kornblith LZ, et al. A comparison between the TEG 6s and TEG 5000 analyzers to assess coagulation in trauma patients. J Trauma Acute Care Surg. (2020) 88:279–85. doi: 10.1097/TA.0000000000002545
55. Carroll RC, Chavez JJ, Simmons JW, Snider CC, Wortham DC, Bresee SJ, et al. Measurement of patients' bivalirudin plasma levels by a Thrombelastograph® ecarin clotting time assay: a comparison to a standard activated clotting time. Anesth Analg. (2006) 102:1316–9. doi: 10.1213/01.ane.0000205746.50440.98
56. Teruya J, Hensch L, Bruzdoski K, Adachi I, Hui SKR, Kostousov V. Monitoring bivalirudin therapy in children on extracorporeal circulatory support devices: thromboelastometry versus routine coagulation testing. Thromb Res. (2020) 186:54–7. doi: 10.1016/j.thromres.2019.12.007
57. Panigada ME, Iapichino G, Brioni M, Panarello G, Protti A, Grasselli G, et al. Thromboelastography-based anticoagulation management during extracorporeal membrane oxygenation: a safety and feasibility pilot study. Ann Intensive Care. (2018) 8:7s. doi: 10.1186/s13613-017-0352-8
58. Henderson N, Sullivan JE, Myers J, Wells T, Calhoun A, Berkenbosch J, et al. Use of thromboelastography to predict thrombotic complications in pediatric and neonatal extracorporeal membranous oxygenation. J Extra Corpor Technol. (2018) 50:149–54.
59. Yabrodi M, Ciccotello C, Bhatia AK, Davis J, Maher KO, Deshpande SR. Measures of anticoagulation and coagulopathy in pediatric cardiac extracorporeal membrane oxygenation patients. Int J Artif Organs. (2020) 1–8. doi: 10.1177/0391398820985525
60. Burton C, Furlong-Dillard JM, Jawad K, Feygin Y, Berkenbosch JW, Tzanetos DT. Analysis of viscoelastic testing in pediatric patients using the pediatric extracorporeal membrane oxygenation outcomes registry. ASAIO J. (2021) 67:1251–6. doi: 10.1097/MAT.0000000000001388
61. Stammers AH, Willett L, Fristoe L, Merrill J, Stover T, Hunt A, et al. Coagulation monitoring during extracorporeal membrane oxygenation: the role of thromboelastography. J Extra Corpor Technol. (1995) 27:137–45.
62. Alexander DC, Butt WW, Best JD, Donath SM, Monagle PT, Shekerdemian LS. Correlation of thromboelastography with standard tests of anticoagulation in paediatric patients receiving extracorporeal life support. Thromb Res. (2010) 125:387–92. doi: 10.1016/j.thromres.2009.07.001
63. Davis MC, Andersen NEO, Johansson P, Andersen LW. Use of thromboelastograph and factor VII for the treatment of postoperative bleeding in a pediatric patient on ECMO after cardiac surgery. J Extra Corpor Technol. (2006) 38:165–7.
64. Northrop MS, Sidonio RF, Phillips SE, Smith AH, Daphne HC, Pietsch JB, et al. The use of an extracorporeal membrane oxygenation anticoagulation laboratory protocol is associated with decreased blood product use, decreased hemorrhagic complications, and increased circuit life. Pediatr Crit Care Med. (2015) 16:66–74. doi: 10.1097/PCC.0000000000000278
65. Laine A, Niemi T, Suojaranta-Ylinen R, Raivio P, Soininen L, Lemström K, et al. Decreased maximum clot firmness in rotational thromboelastometry (ROTEM®) is associated with bleeding during extracorporeal mechanical circulatory support. Perfus. (2016) 31:625–33. doi: 10.1177/0267659116647473
66. Phillips RC, Shahi N, Leopold D, Levek C, Shirek G, Hilton S, et al. Thromboelastography-guided management of coagulopathy in neonates with congenital diaphragmatic hernia supported by extracorporeal membrane oxygenation. Pediatr Surg Int. (2020) 36:1027–33. doi: 10.1007/s00383-020-04694-0
67. Ranucci M, Baryshnikova E, Cota M, Carboni G, Isgrò G, Carlucci C, et al. Coagulation monitoring in postcardiotomy ECMO: conventional tests, point-of-care, or both? Minerva Anestesiol. (2016) 82:858–66.
68. Drop JG, Erdem Ö, Wildschut ED, van Rosmalen J, de Maat MPM, Kuiper JW, et al. Use of rotational thromboelastometry to predict hemostatic complications in pediatric patients undergoing extracorporeal membrane oxygenation: a retrospective cohort study. Res Pract Thromb Haemost. (2021) 5:1–11. doi: 10.1002/rth2.12553
69. Saini A, Hartman ME, Gage BF, Said A, Gazit AZ, Eghtesady P, et al. Incidence of platelet dysfunction by thromboelastography-platelet mapping in children supported with ECMO: a pilot retrospective study. Front Pediatr. (2016) 3:1–8. doi: 10.3389/fped.2015.00116
70. Bhatia AK, Yabrodi M, Carroll M, Bunting S, Kanter K, Maher KO, et al. Utility and correlation of known anticoagulation parameters in the management of pediatric ventricular assist devices. World J Cardiol. (2017) 9:749–56. doi: 10.4330/wjc.v9.i9.749
71. Bingham KR, Riley JB, Schears GJ. Anticoagulation management during first five days of infant-pediatric extracorporeal life support. J Extra Corpor Technol. (2018) 50:30–7.
72. Ferguson LP, Duong P, Pearce KF, Murphy P, Biss TT. Monitoring of antiplatelet therapy in children on ventricular assist device support: comparison of multiplate and thromboelastography platelet mapping. ASAIO J. (2019) 65:84–93. doi: 10.1097/MAT.0000000000000768
73. Moynihan K, Johnson K, Straney L, Stocker C, Anderson B, Venugopal P, et al. Coagulation monitoring correlation with heparin dose in pediatric extracorporeal life support. Perfus. (2017) 32:675–85. doi: 10.1177/0267659117720494
74. Rabinowitz EJ, Ouyang A, Armstrong DR, Wallendorf M, Said AS. Poor reliability of common measures of anticoagulation in pediatric extracorporeal membrane oxygenation. ASAIO J. (2021). doi: 10.1097/MAT.0000000000001582. [Epub ahead of print].
75. Colman E, Yin EB, Laine G, Chatterjee S, Saatee S, Herlihy JP, et al. Evaluation of a heparin monitoring protocol for extracorporeal membrane oxygenation and review of the literature. J Thorac Dis. (2019) 11:3325–35. doi: 10.21037/jtd.2019.08.44
76. Jiritano F, Fina D, Lorusso R, Kowalewski M, Matteucci M, Serra R, et al. Systematic review and meta-analysis of the clinical effectiveness of point-of-care testing for anticoagulation management during ECMO. J Clin Anesth. (2021) 73:110330. doi: 10.1016/j.jclinane.2021.110330
77. Seelhammer TG, Mangla J, Demirci O. The use of thromboelastography to titrate tranexamic acid therapy for abatement of lysis-induced hemorrhagic complications during venoarterial extracorporeal membrane oxygenation. J Cardiothorac Vasc Anesth. (2019) 33:1059–62. doi: 10.1053/j.jvca.2018.07.024
78. Almond CS, Buchholz H, Massicotte P, Ichord R, Rosenthal DN, Uzark K, et al. Berlin Heart EXCOR Pediatric ventricular assist device investigational device exemption study: study design and rationale. Am Heart J. (2011) 162:425–35.e6. doi: 10.1016/j.ahj.2011.05.026
79. Nelles NJ, Chandler WL. Platelet mapping assay interference due to platelet activation in heparinized samples. Am J Clin Pathol. (2014) 142:331–8. doi: 10.1309/AJCPQ9BYJ0OEENGA
80. Smith JW, Steinhubl SR, Lincoff AM, Coleman JC, Lee TT, Hillman RS, et al. Rapid platelet-function assay an automated and quantitative cartridge- based method. Circulation. (1999) 99:620–5. doi: 10.1161/01.CIR.99.5.620
81. Yuriditsky E, Horowitz JM, Merchan C, Ahuja T, Brosnahan SB, McVoy L, et al. Thromboelastography Profiles of Critically Ill Patients with Coronavirus Disease 2019. Crit Care Med. (2020) 48:1319–26. doi: 10.1097/CCM.0000000000004471
82. Seelhammer TG, Plack D, Lal A, Nabzdyk CGS. COVID-19 and ECMO: an unhappy marriage of endothelial dysfunction and hemostatic derangements. J Cardiothorac Vasc Anesth. (2020) 34:3193–6. doi: 10.1053/j.jvca.2020.09.132
83. Chandel A, Patolia S, Looby M, Bade N, Khangoora V, King CS. Association of D-dimer and fibrinogen with hypercoagulability in COVID-19 requiring extracorporeal membrane oxygenation. J Intensive Care Med. (2021) 36:689–95. doi: 10.1177/0885066621997039
84. Nakashima R, Nishihara M, Iyonaga T, Iwasaka S, Yamamoto Y, Shono Y, et al. Efficacy of thromboelastography in the management of anticoagulation for veno-venous extracorporeal membrane oxygenation in a coronavirus disease 2019 patient. Medicine. (2021) 100:e26313. doi: 10.1097/MD.0000000000026313
85. Al-Ghafry M, Aygun B, Appiah-Kubi A, Vlachos A, Ostovar G, Capone C, et al. Are children with SARS-CoV-2 infection at high risk for thrombosis? Viscoelastic testing and coagulation profiles in a case series of pediatric patients. Pediatr Blood Cancer. (2020) 67:e28737. doi: 10.1002/pbc.28737
86. Ankola AA, Bradford VR, Newburger JW, Emani S, Dionne A, Friedman K, et al. Coagulation profiles and viscoelastic testing in multisystem inflammatory syndrome in children. Pediatr Blood Cancer. (2021) 68:1–9. doi: 10.1002/pbc.29355
87. Morparia K, McQueen D, Kalyanaraman M, Bergel M, Narang S, Saini A. Thromboelastography variables in critically ill children with COVID-19. Pediatr Blood Cancer. (2021) 69:e29426. doi: 10.1002/pbc.29426
88. Avidan MS, Da Fonseca J, Parmar K, Alcock E, Ponte J, Hunt BJ. Hunt. The effects of aprotinin on thromboelastography with three different activators. Anesth. (2001) 95:1169–74. doi: 10.1097/00000542-200111000-00021
89. Reynolds PS, Middleton P, Mccarthy H, Spiess BD. A comparison of a new ultrasound-based whole blood viscoelastic test (SEER Sonorheometry) versus thromboelastography in cardiac surgery. Anesth Analg. (2016) 123:1400–7. doi: 10.1213/ANE.0000000000001362
90. Baryshnikova E, Di Dedda U, Ranucci M. A comparative study of SEER sonorheometry versus standard coagulation tests, rotational thromboelastometry, and multiple electrode aggregometry in cardiac surgery. J Cardiothorac Vasc Anesth. (2019) 33:1590–8. doi: 10.1053/j.jvca.2019.01.011
Keywords: extracorporeal membrane oxygenation, thromboelastography, thromboelastometry (ROTEM®), ventricular assist device (VAD), pediatric, child-age
Citation: Regling K, Saini A and Cashen K (2022) Viscoelastic Testing in Pediatric Mechanical Circulatory Support. Front. Med. 9:854258. doi: 10.3389/fmed.2022.854258
Received: 13 January 2022; Accepted: 14 April 2022;
Published: 06 May 2022.
Edited by:
Barbara Zieger, University Hospital Freiburg, GermanyReviewed by:
J Peter Ray Pelletier, UF Health Shands Hospital, United StatesHeidi J. Dalton, Inova Health System, United States
Copyright © 2022 Regling, Saini and Cashen. This is an open-access article distributed under the terms of the Creative Commons Attribution License (CC BY). The use, distribution or reproduction in other forums is permitted, provided the original author(s) and the copyright owner(s) are credited and that the original publication in this journal is cited, in accordance with accepted academic practice. No use, distribution or reproduction is permitted which does not comply with these terms.
*Correspondence: Katherine Regling, a3JlZ2xpbmcmI3gwMDA0MDtkbWMub3Jn; Arun Saini, YXNhaW5pJiN4MDAwNDA7YmNtLmVkdQ==; Katherine Cashen, a2F0aGVyaW5lLmNhc2hlbiYjeDAwMDQwO2R1a2UuZWR1