- 1Department of Periodontology and Implantology, Stomatological Hospital, Southern Medical University, Guangzhou, China
- 2Department of Prosthodontics, Stomatological Hospital, Southern Medical University, Guangzhou, China
Reaching areas at altitudes over 2,500–3,000 m above sea level has become increasingly common due to commerce, military deployment, tourism, and entertainment. The high-altitude environment exerts systemic effects on humans that represent a series of compensatory reactions and affects the activity of bone cells. Cellular structures closely related to oxygen-sensing produce corresponding functional changes, resulting in decreased tissue vascularization, declined repair ability of bone defects, and longer healing time. This review focuses on the impact of high-altitude hypoxia on bone defect repair and discusses the possible mechanisms related to ion channels, reactive oxygen species production, mitochondrial function, autophagy, and epigenetics. Based on the key pathogenic mechanisms, potential therapeutic strategies have also been suggested. This review contributes novel insights into the mechanisms of abnormal bone defect repair in hypoxic environments, along with therapeutic applications. We aim to provide a foundation for future targeted, personalized, and precise bone regeneration therapies according to the adaptation of patients to high altitudes.
Introduction
Due to commercial activities, military deployment, tourism, and entertainment, high-altitude areas (altitude ≥2,500–3,000 m) are among the most important residential and business spaces for modern humans. There are three major plateaus in the world, including the Tibetan Plateau, the Andes Mountains, and the Ethiopian Plateau. The total plateau area is estimated at more than 11,000,000 km2, with a population of ~107 million. The main characteristics of the high-altitude environment include (1) low pressure, oxygen deficiency, and thin air; (2) cold, dry, and strong winds; and (3) long sunshine time and intense ultraviolet radiation. Specifically, the main factors affecting the body are thin air, low atmospheric pressure, and reduced oxygen partial pressure in a high-altitude environment.
There is a relationship between altitude and the decreased partial pressure of oxygen (PO2; i.e., the tension produced by oxygen dissolved in the blood). At an altitude of ~3,000 m, although arterial PO2 (PaO2) is reduced, oxygen saturation can be well maintained (1). The high-altitude environment affects humans mostly because reduced PaO2 in the blood leads to hypoxia (2, 3). At sea level plains (henceforth referred to simply as “plains”), the PaO2 is ~21.15 kPa. However, as the altitude increases by 100 m, atmospheric pressure decreases by 5.9 mmHg, and PaO2 decreases by 1.2 mmHg (4). When the pressure in the air inhaled by humans is lower than 16 kPa (2,500–3,000 m), the symptoms of hypoxia start to appear, including increased breathing and heart rate, headache, loss of appetite, poor sleep, decreased exercise ability, and even mountain sickness (5).
The major metabolic phases of bone defect healing overlap with biological stages, including several stages: haematoma, unmineralized cartilage (soft callus), fibrous tissue, sencodary bone (hard callus), remodeled bone (6). Bone defect repair is affected in high-altitude environments. Indeed, the high-altitude environment has a systemic effect on humans, negatively impacting bone mass, microstructure, and biomechanics of normal bone (7), resulting in declined repair ability of bone defects as follows. First, the healing time of bone defects is significantly prolonged. The incidence of non-union at plateaus is 20–30%, which is significantly higher than that of plains (0.4%) (8). Bone repair efficiency is also significantly reduced (9), and fracture healing time is significantly longer, especially at extreme altitudes (5,400–6,700 m), than that found in coastal areas (10). Furthermore, X-ray findings reveal no periosteal hyperplasia and bony callus generation at the fracture end, while osseointegration is also poor after implantation (9, 11). Second, the different physiological components of bone defect healing are compromised. The capacity to regenerate bone is weaker based on the structural, geometrical, and material properties in a high-altitude environment (12). In addition, callus reconstruction is difficult, and the bone defect is filled with less callus tissue, with mostly new bone and cartilage (13). Moreover, the number of osteoblasts is reduced, and bone defect healing mainly involves endochondral bone (14). Third, different challenges are faced as part of bone defect treatment. For instance, in high-altitude areas, due to the special natural geographical environment of plateaus, the treatment of bone defects caused by war trauma differs from therapies applied in low altitude areas (15). After autogenous bone transplantation, hyperbaric oxygen therapy (HBOT) is often needed to achieve a relatively high success rate (16).
Herein, we review the physiological factors influencing bone repair, the cellular mechanisms of abnormal bone repair, and therapeutic research progress made in addressing the impact of a high-altitude environment on bone defect repair.
Factors Influencing Bone Defect Repair in High-Altitude Environments
The high-altitude environment can exert systemic effects on humans that presents as a series of compensatory reactions. Although these compensations are conducive to adaptation to low pressure and oxygen at high altitudes, they may affect bone defect repair (Figure 1).
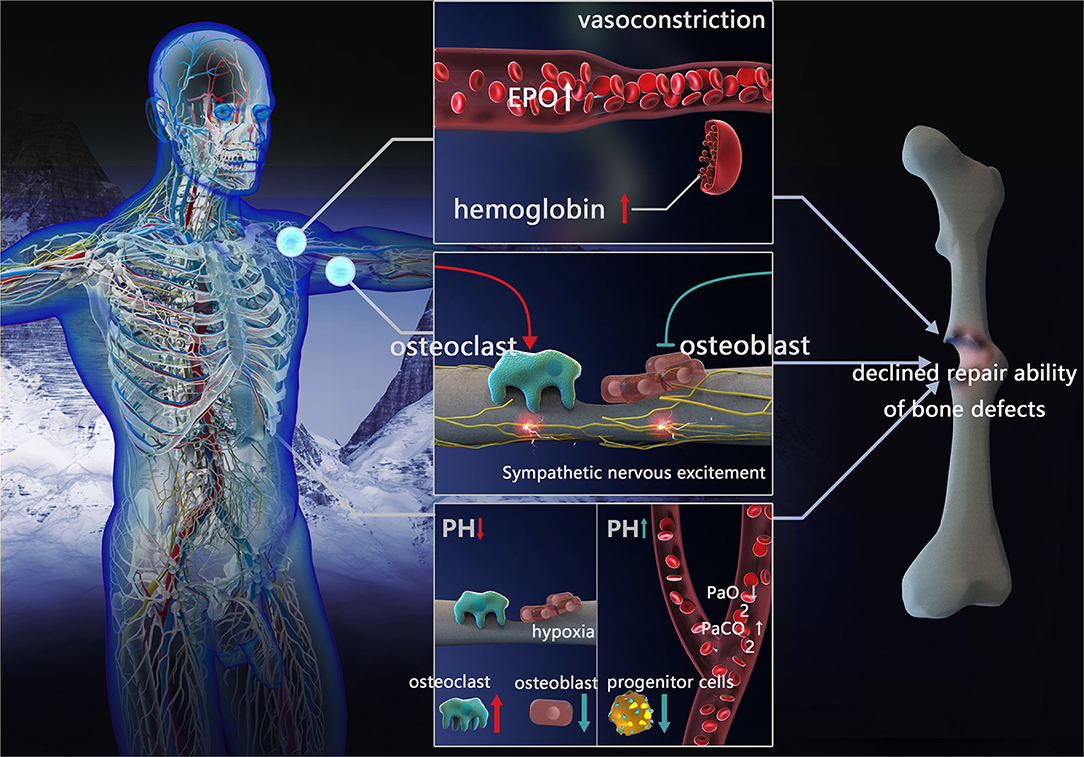
Figure 1. Factors influencing repair of bone defects in high-altitude environment. Blood compensation: vessels constrict, EPO concentration and hemoglobin increased. Sympathetic nervous excitement: inhibit osteoblast activity and promote osteoclast formation. Acid-base compensation: In local acid-base compensation, decreased pH impeding osteoblasts differentiation and enhanced osteoclast activation. In systemic acid-base compensation, with the decreasing of PaO2 and increasing of PaCO2, increased pH inhibits bone marrow progenitor cell proliferation (EPO, erythropoietin; PaCO2, partial pressure of arterial CO2; PaO2, partial pressure of oxygen).
Blood Compensation
The blood system is among the first responses to the high-altitude environment, leading to a series of compensatory reactions. When staying at a high altitude for a short time, the pulmonary arterioles contract, pulmonary blood flow resistance increases, pulmonary arterial pressure increases, and volume vessels contract (peripheral veins), resulting in a release of reserved blood, thus ensuring blood supply to important organs (heart and brain). After living on a plateau for a longer time, acclimatization to the environment occurs by increasing oxygen transport, erythropoietin (EPO) concentration, the number of red blood cells, and hemoglobin concentration (17). However, when the physiological capacity to adapt is exceeded, maladaptation occurs via increased blood viscosity, reduced oxygen transport and oxygen release to vital tissues, and further aggravation of tissue hypoxia. Due to genetic differences, the Andes and Han populations living on the elevated altitudes of Tibet are more susceptible to this type of maladaptation, while the Tibetan residents have higher resting and hypoxic ventilation responses (HVRs), lower arterial oxygen saturation, and reduced hemoglobin concentration at the same altitude (18).
Blood compensation may affect the healing of bone defects through increased EPO production in response to the low pressure and oxygen environment at high altitudes. This in turn affects mobilization and differentiation (osteoblasts, osteoclasts, and mature blood cells) in mesenchymal stem cells (MSCs) and hematopoietic stem and progenitor cells (HSPCs). It is known that low EPO concentration is conducive to bone formation and bone defect healing (19, 20), while high EPO levels lead to the stimulation of osteoclast precursors and induces bone loss, preventing healing (21).
Blood compensation may lead to insufficient blood supply through increased blood viscosity, thus affecting the healing of bone defects. During bone defect healing, the blood vessels and interstitial tissue around the bone need to continuously grow into the center of the defect; hence, the abundance of blood supply affects the healing process. At high altitudes, blood compensation leads to an increase in blood cells. When blood viscosity exceeds a certain limit, it leads to local microcirculation disturbance (22). Platelets in stagnant blood promote the release of thrombin and damage endothelial cells, resulting in increased 5-hydroxytryptamine and histamine content (23), basement membrane exposure, the release of coagulation factors, and even thrombosis (24–26). Therefore, poor blood supply induced by blood viscosity may be one of the factors influencing poor bone defect healing.
Sympathetic Nervous Excitement
In the high-altitude environment, low oxygen stimulates the sympathetic nervous system, prompts catecholamines secretion, accelerates the heartbeat, enhances myocardial contractility, increases cardiac output, and elevates arterial blood pressure to a certain extent. On the skeleton, the sympathetic nerve fibers run along the major artery and nourish the bones through nutrient pores. Both the periosteum and bone marrow receive nutrients from noradrenergic fibers (often associated with the vascular system), vesicular acetylcholine transporter (VAChT), and vasoactive intestinal polypeptide immunoreactive fibers (often associated with the parenchyma). Sympathetic fibers on the periosteum branch overlaying the bone marrow and dense mineralized bone region receive the greatest mechanical stress and load, while also having the highest metabolic rate. Furthermore, the periosteum is the site with the most abundant blood vessels and has the highest density of sympathetic and sensory fibers.
Sympathetic nervous excitement may affect bone defect repair through vasoconstriction, leading to an inadequate blood supply. In high-altitude environments, sympathetic nerves are excited, form a large number of sympathetic active substances, and act on the vascular smooth muscle α receptor, leading to vasoconstriction, enhanced resistance of surrounding blood vessels, and reduced perfusion flow of the tissue (27). Furthermore, it results in decreased blood supply and hypoxia in the soft tissue at and around the bone defects, affecting hematoma organization and callus formation, and interrupting the healing of the bone fracture. The effect of vasoconstriction on the reduction of local blood flow in bone defects is more pronounced with increasing altitude (28).
Sympathetic nervous excitement regulates bone homeostasis and promotes bone resorption. Sympathetic nervous excitement at high altitude may result in noradrenaline nerve endings releasing noradrenaline and stimulating β2-adrenergic receptor (β2AR) near osteoblasts and osteocytes, bone formation inhibition, increased receptor activator of nuclear factor-κB ligand (RANKL) expression, promotion of osteoclast formation, and increased bone resorption. Altogether, these effects impede bone formation. Lastly, the secretion of adrenergic agonists (catecholamines) also stimulates bone resorption, inducing bone loss (29).
Acid-Base Compensation
High-altitude hypoxia induces hyperventilation and increases pH, due to the decreased oxygen availability and subsequent lower PaO2. The HVR occurs when the body attempts to maintain PaO2 to adapt to the high altitude. Although the PaO2 level during HVR is corrected to a certain degree, partial pressure of arterial CO2 (PaCO2) is simultaneously reduced, further leading to decreased plasma H2CO3 concentration and increased pH (30). Minor changes in pH have negligible physiological effects, however, when the pH keeps increasing, a compensatory acid retention mechanism and increased excretion of HCO3 in urine is triggered, causing diuresis of sodium bicarbonate and potassium bicarbonate (31), in turn leading to decreased pH in arterial blood (pHa) compared to the normal level (pHa ≈ 7.4) (32, 33). Insufficient renal compensation may cause a continuous increase of blood pH, weaken HVR, and reduce oxygen saturation leading to the onset of acute mountain disease.
Nevertheless, local pH change in bone defects differs from that of systemic responses. In a high-altitude environment, due to decreased vascular perfusion, tissue hypoxia generates an acidic environment in the bone defect area. Moreover, when a bone defect occurs, disruption of the blood supply can have negative consequences for the bone via the direct actions of hypoxia and acidosis on bone cells (34). In severe hypoxia, glycometabolism at the injury site is incomplete, anaerobic glycolysis is enhanced, and local acidic products are increased (35).
Healing of the bone defect is affected by systemic and local acid-base compensation. A continuous increase of physiological pH caused by the high-altitude environment affects oxygen-carrying capacity, as well as the transportation and release of hemoglobin and blood. In addition, it increases the affinity of hemoglobin to oxygen (Bohr effect) and allows tissues to absorb more oxygen (33). Therefore, in terms of oxygen delivery to the bone tissue, the acid-base compensatory response under the high-altitude environment is advantageous for increasing the local oxygen content in bone defects. However, the pH increase resulting from this compensation does not promote bone marrow progenitor cell proliferation and may affect bone defect healing. Studies have reported that promyelocytic KG-1a cells (hematopoietic stem cells) cultured under high pH have significantly decreased proliferation and enhanced apoptosis (35). The bone contains a large number of alkaline minerals (hydroxyapatite), and bone cells are extremely sensitive to the direct effects of pH. When the acid-base balance cannot be maintained within a narrow range, these alkaline minerals can eventually be used to neutralize pH, in turn reducing alkaline mineral deposition by osteoblasts in the bone (36), and impeding osteoblasts differentiation (34, 37). In contrast, the osteoclastic differentiation and activation are enhanced (37, 38) resulting in detrimental bone defect repair.
Mechanism of Abnormal Bone Defect Repair in High-Altitude
High-altitude areas have thin air and reduced PaO2. Therefore, cellular structures closely related to oxygen sensing, including the mitochondria and cell membrane ion channels, produce corresponding functional changes causing oxidative stress response, ion permeability changes, signaling pathway activity changes, autophagy, apoptosis, etc. In addition, the high-altitude environment alters the epigenetic modification-related effects on genes, thus affecting protein function that may have consequences for bone defect repair (Figure 2).
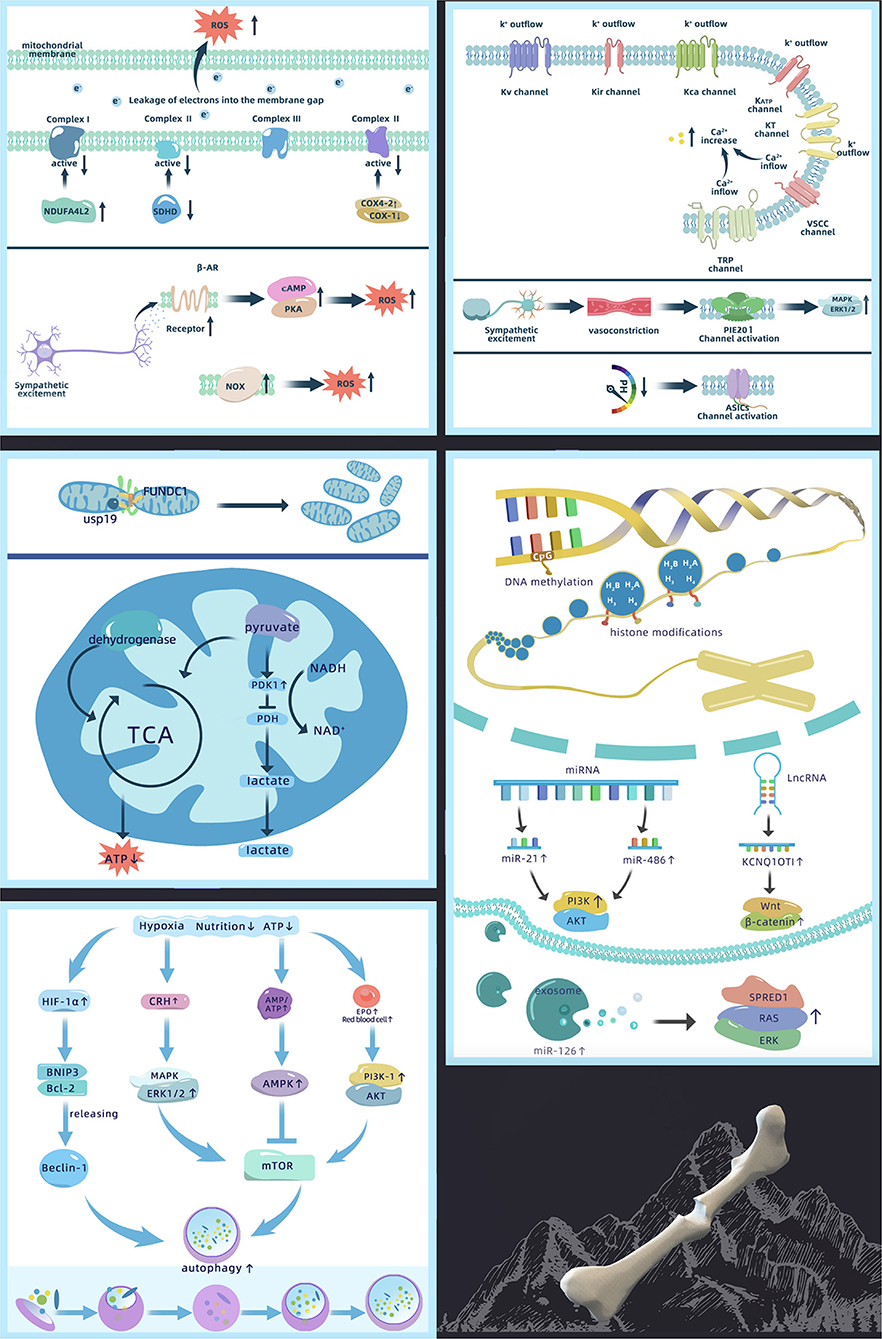
Figure 2. Mechanism of abnormal bone defect repair in high-altitude, including changes in ion permeability of the cell membrane, ROS production, changes in mitochondrial function, activation of autophagy, and epigenetics regulation [Akt, protein kinase B; AMPK, adenosine 5′-monophosphate-activated protein kinase; ASIC, acid sensitive ion channel; ATP, adenosine triphosphate; Bcl-2, B-cell lymphoma 2; BNIP3, Bcl-2 19 kDa interacting protein 3; COX, cytochrome C; CRH, corticotropin releasing hormone; EPO, erythropoietin; ERK1/2, extracellular regulated protein kinases 1/2; FUNDC1, FUN14 domain-containing 1; HIF-1α, hypoxia inducible factor-1α; KATP, ATP-sensitive K+ channels; KCa, Ca2+ activated K+ channel; KCNQ1OT1, potassium voltage-gated channel subfamily Q member 1 opposite strand 1; Kir, inward rectifying K+ channel; KT, double-pore K+ channels; Kv, voltage-gated potassium channel; lncRNA, long non-coding RNA; MAPK, mitogen-activated protein kinase; miRNA, micro-RNA; mTOR, mammalian target of rapamycin; NADH, nicotinamide adenine dinucleotide; NDUFA4L2, NADH dehydrogenase (ubiquinone) 1 alpha subcomplex 4-like 2; NOX, NADPH oxidase; PDH, pyruvate dehydrogenase; PDK1, pyruvate dehydrogenase kinase 1; PI3K, phosphoinositide 3-kinase; PKA, protein kinase A; ROS, reactive oxygen species; SDHD, succinate dehydrogenase complex subunit D; SPRED1, sprouty-related EVH1 domain containing 1; TCA, tricarboxylic acid cycle; TRP, transient receptor potential channel; USP19, ubiquitin specific protease 19; VSCC, voltage sensitive Ca2+ channel].
Changes in Ion Permeability of the Cell Membrane
Ion channels participate in a variety of high-altitude adaptive compensations such as HVR and vascular tension changes. It is generally accepted that high-altitude environments inhibit K+ channels and activate Ca2+ channels (39, 40). Altered K+ permeability affects osteoblast differentiation and proliferation (41). Meanwhile, altered Ca2+ permeability affects the mineralization of extracellular inorganic Ca2+, which acts as a second messenger, impacting the expression of downstream osteogenesis-related signaling pathways (42), thereby influencing bone defect repair.
High-altitude-related low oxygen inhibits the activity of K+ channels, leading to membrane depolarization. Acute hypoxia depolarizes the membrane potential by 15–20 mV (43). It has been confirmed that K+ channels affected by oxygen in bone cells include voltage-gated potassium channels (Kv), inward rectifying K+ channels (Kir), Ca2+-activated K+ channels (KCa), ATP-sensitive K+ channels (KATP), and two-pore K+ channels (KT) (44). However, the mechanism by which these K+ channels sense oxygen changes is not fully elucidated. Studies have found that K+ channels retain their hypoxic reactivity after recombination (45, 46), indicating that oxygen not only directly regulates K+ channels but also affects ion permeability through pore-forming subunits or regulatory β-subunits. Six transmembrane subunits and one pore-forming subunit (Kv1.2, Kv1.5, Kv2.1, Kv3.1, Kv3.3, Kv4.2, and Kv9.3) of the Kv channels are reversibly blocked by hypoxia (47). Furthermore, four transmembrane and two pore-forming subunits of the double-pore potassium ion channel KT are also involved in oxygen sensing peripheral chemoreceptors (48). However, whether other subunits play a role in the low-oxygen environment at high altitudes remains unclear.
High-altitude hypoxia increases intracellular Ca2+ levels and causes calcium overload, which results from Ca2+ channel activation. The main Ca2+ channels affected by oxygen in bone cells include voltage-sensitive Ca2+ channels (VSCCs) and transient receptor potential channels (TRPs). In these Ca2+ channels, TRPs are the most predominant oxygen sensors (49). TRP expression differs on the surface of different bone cells; e.g., TRP vanilloid-5 (TRPV5) is missing in osteoblasts (50). However, only the hypoxic responses of TRPV1 and TRPV4 have been examined. Nevertheless, TRPV5 and TRPV6 are also closely associated with bone defect healing (51). Different from the other TRPV channels, these are highly selective for Ca2+ and their changes under hypoxia need to be confirmed. In addition, although VSCC expression is greatly reduced on osteoblasts compared with excitable cells, reduced PaO2 can lead to rapid activation of L-type VSCCs (52). However, the change in T-type VSCC under hypoxic conditions has not been reported. This may be a result of the T-type VSCC only being involved at the early stage of cell differentiation, after which its activity significantly reduces (53).
In addition to oxygen-sensitive ion channels, only a few studies have examined changes in other ion channels that may be associated with high-altitude environments. For example, compensatory responses to high-altitude have been reported whereby sympathetic excitation can lead to local vasoconstriction with increased expression of the mechanically-gated channel Piezo1 during vasoconstriction (54), activating the downstream MAPK/ERK1/2 signaling pathways. In addition, the local extracellular environment of bone defects is acidified under hypoxia, which can activate acid-sensitive ion channels (34).
It may be speculated from existing studies that oxygen-sensitive ion channels play the main role in high-altitude bone defects. Most literature assessing the effects of high altitude on ion channels focused on pulmonary edema. However, whether other subtypes of TRPs (such as TRPC, TRPA, TRPM) also present similar changes in bone cells as pulmonary vascular cells have not been reported and should be considered for future research. In addition, there are few studies on Na+ channels. The latest study showed that Na+ acts as a second messenger to regulate the permeability of the inner mitochondrial membrane in an acute hypoxic environment (55). Therefore, attention should be paid to the changes of Na+ channels in high-altitude environments as well as the related effects on bone defect repair.
ROS Production
Both acute and long-term exposures to high-altitude environments induce the production of reactive oxygen species (ROS), which results in oxidative stress response (56, 57). The ROS level increase in the bone can affect bone defect healing by decreasing osteoblast activity and accelerating bone resorption by osteoclasts (58). In addition, ROS can also induce osteoblast and osteocyte apoptosis (59), affecting the quality of bone defect healing.
Hypoxia is the main factor increasing ROS in high-altitude environments. At a plateau, PaO2 gradually decreases with altitude elevation. The mitochondria, a main source of ROS, are affected by the changes in intracellular and extracellular PaO2. Multiple studies have suggested that mitochondrial complex III (ubiquinol-cytochrome c oxidoreductase) and complex I (NADH-ubiquinone oxidoreductase) are major sites of ROS production (60–62). Hypoxia reduces complex I activity by upregulating NDUFA4L2 (63). However, studies have found that complex IV (cytochrome C oxidase) and complex II (succinate dehydrogenase) in the mitochondria are also sensitive to hypoxia. This is because complex IV has a binding site for oxygen, and hypoxia can reduce its activity by upregulating COX4-2, an isoform of cytochrome C oxidase (COX), and downregulating COX-1 (64). It also affects the activity of complex II by decreasing SDHD expression (62). In addition, insufficient oxygen supply can also obstruct mitochondrial electron transport by partially leaking single electrons from complexes I–III directly to oxygen leading to the production of large amounts of ROS (56).
In addition to the effects of hypoxia, sympathetic nervous system excitement at high altitude may be one of the factors that contribute to increased ROS production. It was found that adrenaline receptors mediate the production of cellular ROS induced by sympathetic system over-excitation. β-AR promotes the mitochondrial tricarboxylic acid cycle, enhances oxidative respiration, and increases oxygen consumption through the classical cAMP/PKA pathway, which leads to enhanced electron leakage into the mitochondrial inner and outer membrane spaces, thereby increasing ROS production (65). In addition to the mitochondria, NOX (NADPH oxidase), distributed on the plasma membrane and multiple organelle membranes, also produces ROS upon sympathetic system excitation (66) and promotes vasoconstriction through a RhoA kinase-dependent pathway (67). However, studies examining sympathetic nervous excitement with ROS are currently limited to cardiomyocytes and vascular smooth muscle cells. Thus, whether a similar mechanism also exists in bone cells needs confirmation.
The results of in vitro mechanistic studies evaluating high-altitude-related ROS production as well as the actual in vivo situation in high-altitude populations may differ due to the genetic polymorphisms of ROS production in high-altitude environments. Studies have found that the mtDNA 10609T in Han people living on the plateau promotes the increase of intracellular ROS in hypoxia, while the mtDNA T8414 does not (68). In addition, the high-altitude environment increases ROS, which has a negative effect on bone defects. On the other hand, increased ROS can stabilize the activity of hypoxia-inducible factor 1-alpha (HIF-1α), which is helpful for the body to cope with the high-altitude environment. Therefore, balancing the advantages and disadvantages ROS still needs quantitative investigation.
Changes in Mitochondrial Function
Mitochondrial dysfunction can affect osteoblast, osteocyte, and vascular endothelial cell functions (69, 70) as well as inflammatory reactions in chondrocytes. In turn, this leads to metabolic disorders in chondrocytes, affecting endochondral osteogenesis (71). Moreover, osteogenic differentiation of human marrow MSCs can be impeded by mitochondrial dysfunction (72), which is not advantageous for bone defect repair.
Mitochondria are the main consumers of oxygen in cells and need to adapt collectively to the decrease in available oxygen at high altitudes. Low oxygen environments can lead to an increased number of mitochondria and imbalanced proteostasis. A possible mechanism is that hypoxia induces mitochondrial fission via mitochondrial outer-membrane protein FUNDC1 signaling. Under hypoxia, the deubiquitinase USP19 accumulates at the ER-mitochondria contact sites with FUNDC1. USP19 then interacts with and removes ubiquitin chains from FUNDC1 at the ER-mitochondria contact sites. After USP19 stabilizes FUNDC1 and subsequently promotes Drp1 oligomerization (73, 74), hypoxia-induced mitochondrial division occurs thus increasing the number of mitochondria. In addition, recent studies have found that hypoxia suppresses mTORC1 signaling and mediates homeostasis remodeling of mitochondrial proteins by regulating substrate-related mitochondrial metabolism through the mTORC1-LIPIN1-YME1L signaling axis (75).
In addition, high-altitude environments may also cause changes in mitochondrial respiration and aerobic capacity. However, how this dysfunction relates to the degree of hypoxia is unknown. Mitochondrial respiratory function is not affected in mild or early hypoxia. It was found that the effect of 15 days of mild normobaric hypoxia on mitochondrial function is negligible as mitochondria adapt to the environment by increasing LON protease content, optimizing respiratory chain function (76). However, severe hypoxia leads to mitochondrial dysfunction. When the PaO2 in the mitochondria drops to the critical point of 0.1 kPa (<1 mmHg), dehydrogenase activity decreases, reducing the respiratory function of the mitochondria and decreasing ATP production (77). Moreover, mitochondrial metabolic pathways switch from aerobic to anaerobic metabolism under hypoxic conditions. The possible explanation is that hypoxia upregulates pyruvate dehydrogenase kinase 1 (PDK1), inactivates pyruvate dehydrogenase (PDH), transforms pyruvate to acetyl-CoA, and reduces the availability of substrates for oxidative metabolism, thereby promoting the conversion of pyruvate to lactate (78).
However, changes in mitochondrial function under high-altitude environments are correlated with mitochondrial genes. Studies have found that mitochondrial haplogroups B and M7 may be related to inadaptability to hypoxia, while haplogroups G and M9a1a1c1b are related to hypoxia adaptation. Specifically the T3394C and G7697A mutations in haplogroup M9a1a1c1b may be the main factor improving the ability to adapt to the environment in Tibetans living on the plateau for generations (79). In addition, the mitochondrial genes MT-ND1 and MT-ND2, encoding two subunits of mitochondrial NADH dehydrogenase, play an important role in the oxidative phosphorylation electron transport chain and contribute significantly to high-altitude hypoxia adaptation of the mitochondria (80).
In addition to hypoxia, high-altitude factors such as cold (81) and strong ultraviolet radiation (82, 83) may also impact mitochondrial function. Nonetheless, no relevant studies have assessed their role on mitochondria in bone tissue, which may be an important future direction.
Autophagy
Both acute and chronic high-altitude exposures activate autophagy and increase cell death (84). Overall, autophagy exerts a protective effect under short-term moderate stimulation induced by the high-altitude environment (85, 86), which can increase the expression of vascular endothelial growth factor (VEGF) by stabilizing HIF-1α, thereby benefiting angiogenesis (87). Meanwhile, as a carrier of osteoblasts to secrete hydroxyapatite crystals, autophagosomes participate in bone formation (86, 88). However, a sustained high level of the oxidative stress response can overstimulate autophagy, leading to premature cell death (89).
Studies have found that autophagy acts in a HIF-1α-dependent manner under hypoxia (90). HIF-1α activates downstream BNIF3, then competitively binds Bcl-2 with Beclin-1 for subsequent activation of autophagy. However, the regulatory mechanism involved in autophagy has not been studied in bone cells. Findings in other cell types reveal that hypoxia affects autophagy via the HIF-1α/Beclin-1 pathway in SH-SY5Y cells (neuroblastoma cells), dendritic cells (DCs) (91), and vascular endothelial cells (87). However, this autophagy activating pathway is not present in all cell lines. For example, autophagy of NP cells (nucleus pulposus cells) in the hypoxic environment is independent of the HIF-1α pathway. Therefore, further investigation is needed to determine whether the same mechanism exists in skeletal cells.
In addition, a high-altitude environment can also regulate autophagy through the mTOR-related signaling pathway as mTOR kinase can be inhibited under conditions such as malnutrition, decreased ATP levels, and hypoxia. Thus, reduced metabolic activity induces autophagy (90). Cellular energy deficiency resulting from high altitude leads to increased AMP/ATP ratio (92), whereas hypoxia induces the phosphorylation of AMPK (93), all of which regulate the AMPK/mTOR signaling pathway. Moreover, the increased number of red blood cells and EPO caused by high-altitude compensation can activate the PI3K-1/Akt/mTOR signaling pathway (94). The low-oxygen environment at high altitude also upregulates corticotropin-releasing hormone (CRH) and regulates mTOR in autophagy through the MAPK/ERK1/2 pathway (95, 96).
However, the basal level of autophagy is not the same in different populations; hence, the effects of autophagy on bone cells may also differ between individuals in high-altitude environments. Studies have shown significant differences in the levels of autophagy markers, including LC3 and BNIP3, between Tibetans living at an altitude of 3,000 m and Han nationals living below 500 m (97). Long-term living in high-altitude environments could increase the level of basal autophagy—possibly by resisting the negative effects of oxidative stress through a higher level of autophagy.
Besides, bone marrow is in a hypoxic environment under physiological conditions. Thus, whether in vitro effects of hypoxia on autophagy signaling pathways could be translated in vivo need to be confirmed. However, through literature review, we found that altitude affects autophagy by various mechanisms, emphasizing the complexity of the autophagy signaling pathways. Future research should investigate whether autophagy is affected by the degree and duration of bone defects at high altitudes.
Epigenetics
Populations living at high altitudes for generations all have a genetic basis for adaptation to high-altitude environments. However, genetics alone cannot fully explain the mechanism of impaired bone defect repair at high altitudes. An increasing number of studies have confirmed that epigenetic alterations integrate genetic and environmental stimuli to participate in hypoxia adaptation of tissues and cells, thereby affecting bone defect repair by regulating related downstream genes. Some of the most prominent epigenetic mechanisms include DNA methylation, histone modifications, and non-coding RNAs.
The most intensively studied epigenetic mechanism to date is DNA methylation. DNA sequencing in high-altitude and plain populations reveals that genes with differential methylation are mainly involved in HIF-related signaling pathways (98, 99). The methylation levels of HIF-1α and HIF-2α promoter regions are significantly lower in plateau animals (99, 100). HIF-dependent hypoxia response element (HRE)-related genes are sensitive to methylation, and a large number of CpG dinucleotides are present at the binding site of the HRE sequence of HIF (101). When methylation changes occur at the above sites, binding to various transcription factors is inhibited. Studies have shown that chronic hypoxia induces CpG for VHL promoter methylation, reduces VHL expression, and increases EPO production by elevating HIF-2α expression in the bone marrow, leading to erythrocytosis (102). Meanwhile, HIF-1α cannot be degraded by ubiquitination and further accumulates in cells (103). Evidence suggests that DNA hypomethylation in high-altitude environments favors the expression of HIF genes, in turn triggering the hypoxia adaptation response of the tissue and cells by regulating the activity of downstream genes.
It is known that high-altitude hypoxia also leads to changes in histone modifications. The modification sites are generally located on four common histones, including H2A, H2B, H3, and H4—with H3 and H4 being the most common. Studies have shown that high-altitude environments increase the levels of H3K14ac, H4R3me2, H3K4me2, H3K4me3, H3K79me2, H3K9/27me2, H3K9me2, H3K27me3, and H3K4mel in mice (104). In addition, the Jumonji domain (JMJD) protein family comprises enzymes catalyzing the demethylation of arginine and lysine residues in histones. Both JMJD1A and JMJD2B retain their activities, bind to specific recognition sites of HIF-1α, and induce its expression under high-altitude hypoxia (105). The chemical modification of these histones ultimately alters the expression of the genes by changing the affinities of the promoter regions of genes associated with hypoxia response.
Non-coding RNAs are expressed differently in plateau and plain environments. Examining the expression profiles of miRNAs between these environments revealed a total of 26 differentially expressed miRNAs (106). The hypoxic environment upregulates the expression of miRNA-21 (107) and miR-486 (108), while increasing osteogenic differentiation of bone MSCs (BMSCs) through the PI3K/Akt pathway. Studies have shown that lncRNAs play an important role in the direct or indirect regulation of HIF-1α expression and related pathways, and interrupt angiogenesis and bone formation in hypoxia by negatively regulating HIF-1α at the mRNA level. The lncRNA KCNQ1OT1 exerts the primary effects in delayed fracture healing, inducing cell proliferation and inhibiting cell apoptosis by activating the Wnt/β-catenin signaling pathway (109). In addition, hypoxia promotes the production of miR-126 production in exosomes to enhance bone defect healing via the SPRED1/Ras/ERK signaling pathway (110).
It remains controversial whether epigenetic factors are beneficial to bone defect repair at high altitudes. Most studies investigating the impact of epigenetic factors started from the aspect of hypoxia in the high-altitude environment. However, it is important to identify other key factors involved in the healing of bone defects to determine how they are regulated under a high-altitude environment. Moreover, the histone modification process is extremely complex and has not been comprehensively studied. For example, the histone protease LSD1 is a key factor regulating endochondral ossification during bone regeneration (111). Yet, how it changes under high-altitude environments has not been elucidated, which deserves further attention.
Implications For Treatment
Oxygen Therapy
Oxygen therapy is the primary treatment for acute and chronic altitude sickness. It primarily serves to improve PaO2 and arterial oxygen saturation, increase the content of arterial oxygen, and correct various hypoxia levels caused by the high altitude. Furthermore, oxygen administration can be divided into systemic and local types.
The most common systemically administered oxygen therapy is HBOT (112). Hyperbaric oxygen can reduce the cardiac workload, improve cardiac function, block the vicious cycle of hypoxia leading to excessive erythrocyte proliferation, reduce the respiratory rate, and correct the acid-base balance. Hyperbaric oxygen can play a role in promoting the repair of both fresh and old bone defects. It helps reduce tissue edema, restore venous return, improve microcirculation (113, 114), and stimulate angiogenesis (115), Furthermore, it also increases PaO2 in the fractured area (especially in the callus and medullary cavity), enhances the activities of osteoclasts and osteoblasts, and accelerates bone callus formation (116). In addition, hyperbaric oxygen also enhances the anti-infective ability of local tissues, especially against anaerobic bacteria (117). Clinically, hyperbaric oxygen chambers have been widely used in patients with bone defects to shorten the recovery time. In response to peri-implant tissue stimulation by titanium (Ti) particle exposure, ROS production (118), pro-inflammatory cytokines, infiltration of inflammatory response cells, and activation of the osteoclast activity (119), HBOT can be used in combination with bone grafts (120). Lastly, HBOT also improves the implantation of osseointegration (121).
Topical oxygen therapy (TOT) has been used in diabetic skin ulcers, post-operative infections, and gangrenous lesions. The most common method uses the micro-oxygen wound therapy instrument, which delivers pure oxygen to the wound for 24 h via an oxygen administration tube yet is not readily applied in bone defect repair. Studies have also found that local oxygen administration improves post-operative local PaO2 and oxygen saturation at sternal defects and reduces the risk of infection (122). In addition, reports have adopted innovative local oxygen delivery methods. For instance, perfluoro-octane-loaded hollow particles can be used as a local oxygen source, increasing cell viability, and maintaining the osteogenic differentiation potential of human periosteum-derived cells under hypoxic conditions (123, 124). However, only local oxygen-releasing dressings, including OxygeneSys, Oxyzyme, and Oxyband, have been marketed to date, and oxygen released from these products can only act on the wound surface and cannot improve PaO2 to address deep bone defects.
In general, HBOT has been widely used in clinical practice with a definite curative effect, but measures should be taken according to local conditions. Specifically, the treatment pressure should not be too high, and the speeds of pressure increase and decrease rates should be reduced, with the times of pressure increase and decrease extended appropriately. In addition, the high-pressure oxygen chamber has various shortcomings, including inconvenient mobility, which cannot meet the criteria of emergency treatment to combat altitude sickness. Thus, scientists have also developed a vehicle-mounted mobile hyperbaric oxygen chamber. However, the effect of local oxygen therapy in the treatment of bone defects remains unclear and deserves further investigation.
Systemic Administration
Some drugs can promote the healing of bone defects by improving the physiological ability to adapt to hypoxic environments, reducing ROS, and improving ion permeability of the cell membrane.
As far as traditional Chinese medicine (TCM) is concerned, the anti-altitude sickness drug rhodiola has the obvious effect of inhibiting bone resorption. Studies have found that rhodiola reduces ROS production, significantly decreases the expression and activity of MMPs, and upregulates the TIMP protein (125). Salidroside, the major bioactive compound of rhodiola, has various pharmacological effects and acts through HIF-1α-VEGF (126) and BMP (127) pathways, simultaneously promoting angiogenesis and osteogenesis, thereby accelerating bone defect healing. Rosavin, a rhodiola component, can block the NF-κB and MAPK pathways, inhibit RANKL-induced osteoclast formation in vitro and in vivo, decrease the expression of genes related to osteoclast differentiation, and promote osteogenesis in BMSCs (128). In addition, Jiuerjiegusan and Jieguling capsules are used in the treatment of high-altitude traumatic fractures, also significantly accelerating fracture healing.
Supplementation of antioxidants can reduce the negative effects of oxidative stress on bone defect repair by reducing ROS amounts (129). Common oral exogenous antioxidants include vitamin C, vitamin E, and trace elements. Polyphenols in fruits and vegetables also act as natural antioxidants. The effects of oral antioxidants are currently controversial. Studies have found that antioxidant cocktail therapy has no effects on bone resorption or formation (130). Epidemiologic studies showed that although systemic administration of vitamin C improves the soft tissue healing of tooth extraction wounds (131, 132), there is no significant difference in the percentage of X-ray density of new bone formation (133). The main polyphenolic catechins in green tea increase the survival, proliferation, increasesdifferentiation, and mineralization of osteoblasts by promoting osteogenic differentiation of MSCs (134). Conjugated linoleic acid (CLA) is an important component of the Tibetan diet, with a strong antioxidant effect. CLA the quality and mechanical strength of bone callus fracture healing in rats (135), and significantly reduces alveolar bone loss in rats with periodontitis and diabetes (136).
Calcium ion antagonists, which dilate blood vessels and relax bronchial smooth muscles, are commonly used to treat high-altitude reactions, improving the anti-hypoxia ability to various degrees. Ronacaleret, a novel calcium-sensing receptor antagonist, stimulates the release of parathyroid hormone (PTH) and increases the expression of bone formation markers. A phase I and II clinical study found that it acts as a potent oral anabolic agent to promote bone fracture healing (137). However, it has not been reported whether other calcium antagonists can be used for the treatment of high-altitude-related bone defects by systemic administration.
Most of the above reports are basic research, and large-scale, placebo-controlled, long-term randomized trials with optimal timing of protocol interventions are still needed to determine the efficacy of drugs on bone defect repair. In addition, non-targeted mitochondrial antioxidants cannot accumulate in large quantities in key steps of mitochondrial ROS production, and may eventually interfere with subsequent physiological signal transduction (138). Therefore, the safety of these proof–of–concept drugs remains to be confirmed in further experiments. Under the guidance of the TCM theory, TCM has its advantages in fundamentally preventing and treating altitude sickness. Its combined application with Western medicine is expected to become the future therapeutic standard for treating plateau bone defects.
Local Drug Delivery
Drugs directly acting on bone defects are administered by local drug delivery. With the development of biomaterials and bone tissue engineering technology, local drugs are sometimes combined with biomaterials to enhance angiogenesis and osteogenesis abilities, which effectively repair bone defects and promote bone regeneration to a certain extent.
Bioactive materials have been widely reported in the treatment of bone defects, but whether they have the same efficacy in high-altitude environments remains to be confirmed. In view of the potential mechanism of poor bone defect repair at high altitudes, existing studies can serve as a certain reference. Amorphous silicon nitride (Si(On)x) can be used as nano-coating for titanium plates, which have a strong attachment to the surface of scaffolds and induces the sustainable release of Si (+4-valent) for a prolonged time (139), enhancing the expression of superoxide dismutase. Thus, its therapeutic use is also expected to promote bone tissue repair and angiogenesis by reducing the influence of ROS in the high-altitude environment (140). Bioactive borosilicate glass (BG) scaffolds and tricalcium phosphate (TCP) are commonly used materials in bone repair. DMOG, a small molecule angiogenic drug that can adjust the stability of HIF-1α (141), can be added to BG and TCP to promote new bone formation and neovascularization in bone defects (142).
HIF is a key factor in response to hypoxic stress in high-altitude environments; direct application of HIF or the use of HIF-related drugs may promote bone defect repair at high altitudes. For instance, DBBM-C (deproteinized bovine bone +10% collagen) combined with HIF-1α promotes the formation of new bone (143). HIF-1α mediates DNA delivery via the protein transduction domain (PTD), and local administration of HIF-1α via PTD promotes bone growth (142). Under severe hypoxia, mechanical growth factor E inhibits the expression of HIF-1α and its transfer to the nucleus, thus regulating the proliferation and osteogenic differentiation of BMSCs (144). In addition, local application of HIF-related drugs may also be helpful for cartilage repair. Studies have shown that HIF-1α combined with collagen scaffold can repair osteochondral defects of the condyle of the temporomandibular joint in rabbits (145). Local injection of icariin inhibits the NF-κB/HIF-2α signaling pathway, thereby enhancing chondrocyte viability (146).
As discussed earlier, autophagy plays an important role in poor bone defect repair at high altitudes. Therefore, applying drugs regulating autophagy may be a potential therapeutic approach. Metformin, which is commonly used to combat hyperglycemia, has also been shown to affect bone regeneration. Metformin increases autophagy in BMSCs under hypoxic conditions and upregulates the osteogenic markers Runx2, osteocalcin, and alkaline phosphatase to significantly accelerate the formation of new bone (147, 148). Simvastatin, a serum cholesterol-lowering drug, has been shown to promote bone regeneration. Topical administration of simvastatin enhances autophagy and reduces the activity of osteoclasts (149), as well as induces homing of endothelial progenitor cells and promotes angiogenesis (150). It is as effective in repairing long tubular and flat bone defects as autografts (151). Other studies have found that under hypoxic conditions, resveratrol and angiopoietin 2 improve the survival and differentiation of BMSCs through autophagy (152, 153). Furthermore, intraperitoneal administration of the autophagy inducer rapamycin in fractured rats improves the autophagy level, increases bone callus formation, and accelerates fracture healing (154). Nevertheless, rapamycin has many adverse reactions. Therefore, identifying autophagy modulators with reduced toxicity and good efficacy is a direction of future research.
Local drug therapy can act directly on the bone defect site to accelerate fracture healing and enhance bone graft stability. However, the condition of bone defects faced by clinicians in high-altitude environments is complex and often occurs in combination with hypothermia, hypoxemia, and infection. Therefore, it is possible to combine drugs that promote osteogenesis with those conferring anti-infection and rehydration to establish a suitable rescue treatment approach for bone defects in high-altitude areas.
Stem Cells
The regeneration ability of bone tissue cells is compromised in high-altitude environments. Fortunately, stem cell therapy by itself or in combination with drugs, surgery, biomaterials, etc., can play a role in accelerating wound repair by improving tissue differentiation ability.
In high altitude environment, EPO was increased. Erythropoietin receptor (EPOR) was expressed in bone marrow stromal cells (BMSC), and it was verified that increased EPO results in reduced bone by regulating BMSCs (155). Although the local transplantation of BMSCs accelerated wound repair of high-altitude femoral defects, the healing rate of the high-altitude group remained lower than that of the plain group (156). Therefore, improving the survival rate and osteogenic ability of transplanted BMSCs in hypoxic regions is crucial. Studies have shown that BMSC transplantation combined with FG4592 administration can further accelerate fracture healing by increasing the proliferation and migration of BMSCs (157). BMSCs are reprogrammed into induced pluripotent stem cells (iPSCs), called iPSC-MSCs, whose morphology, immunophenotype, in vitro differentiation potential, and DNA methylation pattern are similar to those of BMSCs. Nevertheless, iPSC-MSCs have a higher proliferative capacity and promote bone repair and angiogenesis more pronouncedly (158). A 3D cell oxygen permeation culture device “Oxy Chip” was developed to generate and supply oxygen to cell spheroids to prevent hypoxia (159). It could promote osteoblastic differentiation of MSCs.
Adipose-derived stem cells (ADSCs) could differentiate into chondroblasts after 2 days of in vitro culture without the addition of growth factors at low oxygen partial pressure (<1% PO2) (160). However, for osteogenic differentiation to be achieved, different growth factors or biomaterials should be combined to provide an osteogenic induction environment for ADSCs. The combination of ADSCs and autologous platelet-rich plasma (PRP) was not statistically significant compared with autologous bone grafts (161). This may be because the growth factor mixture in PRP has a short half-life in vivo, which cannot guarantee osteogenic induction of ADSCs during the whole process of bone repair. Nonetheless, tissue engineering scaffolds have advantages in providing a continuous environment for osteogenic induction. Some scholars have developed epigallocatechin gallate (EGCG)-coated synthetic fibers encapsulating ADSCs to fabricate stem cell spheroids for bone tissue regeneration. These EGCG fibers effectively delivered osteo-inductive and ROS scavenging signals to ADSCs in spheroids, upregulating the osteogenic markers RUNX2 and OPN (162). In addition, a novel 3D BG scaffold (BG-XLS/GelMA-DFO) combined with ADSCs could also promote bone regeneration under simulated hypoxia conditions (163).
The cell viability and survival time of ordinary 2D-cultured MSCs after transplantation is not ideal. It is the current trend to aggregate cells into 3D spheres or to bind, extend, and grow them on porous 3D scaffolds. Since blood supply plays a crucial role in bone defect repair, whether the 3D culture of stem cells can be vascularized has become an important hotspot for research. Organoids are stem cell-derived 3D culture systems. It is now possible to re-create the architecture and physiology of human organs in remarkable detail. A preliminary breakthrough has shown that organoids can form a vascular network through the Organ Bud technology (164, 165), which is expected to become a future research direction for developing treatment tools for bone defects at high altitudes by stem cell transplantation.
Gene Modifications
The rapid development of modern molecular biology theory and technology has given rise to innovative solutions to address bone defect repair. For instance, using gene therapy, a target gene combined with a carrier can be injected directly into the target tissue. Alternatively, gene-modified stem cells can be used to promote new bone formation and repair bone defects. The most common methods of gene modifications are viral and non-viral vectors, including retroviruses, lentiviruses, adenoviruses, liposomes, and cationic polymers.
Osteogenesis-related genes can be introduced to target cells by vectors. This enables achieve long-term stable expression of the genes of interest within the bone defect, which could promote bone defect repair. Studies have shown that adenovirus transduction of human BMP2 promotes osteogenic differentiation of adipose tissue fragments (166). Introducing the BMP2 gene into BMSCs can induce bone differentiation and accelerate the healing process (167–169). Furthermore, using gene modification, the OPG gene was introduced into BMSCs seeded on a hydroxyapatite (HA) scaffold to form a novel OPG-BMSC-HA complex, which could promote the osteogenic effect of BMSCs and facilitate bone defect reconstruction therapy (170). Runx2 gene-modified MSC-derived 3D spheroids have also been used to effectively promote bone regeneration (171). VEGF transfected with recombinant adenovirus vector preserves the oxygen sensitivity of HIF-1/HRE and promotes vascularization (172).
As small non-coding RNAs that regulate gene expression, miRNAs have become new targets for poor bone healing. Studies have shown that ADSCs transfected with miR-26a (173), as well as BMSCs transfected with miR-218 (174) and miR-29b-3p (175), can improve bone regeneration capacity. In addition, miR-378 can stimulate both osteogenesis and angiogenesis (176). Therefore, it can be used as a reference target for the treatment of bone defects at high altitudes (177). Regarding the mechanism underlying the effect of high altitude on bone repair, the hypoxic environment can upregulate miR-21 (107) and miR-486 (108). Therefore, these two miRNAs are also potential therapeutic targets. It was found that miR-21 activates the PI3K/Akt signaling pathway (178, 179) and significantly increases the volume of new bone formation and mineralization at the bone defect site. miR-486-3p targeting catenin β-interacting protein 1 can activate the Wnt/β-catenin signaling pathway and promote the bone formation of BMSCs (180).
Exosomes exhibit biological characteristics similar to those of the parent cells and can be used as carriers to deliver genes to cells (181). In addition, direct application of exosomes can also reduce the risk of immunogenicity, avoiding the ethical and technical issues linked to cell therapy (182). BMSC-derived exosomes carrying miR-335 promote fracture recovery through activation of the Wnt/β-catenin signaling pathway (183) and enhance bone regeneration by inhibiting hypoxia-induced osteocyte apoptosis (184)—thus, significantly preventing bone loss and increasing the blood vessel volume of the femoral head (185). Ther exosomal release is induced upon hypoxia (186) and this approach may be used in the treatment of bone defects in high-altitude-related hypoxic environments. In addition, umbilical cord MSC-derived exosomes increase the expression of VEGF and HIF-1α, which may accelerate fracture healing by promoting angiogenesis (181). The combination of exosomes with biomaterials is also one of the potential therapeutic strategies for repairing cartilage (187) and bone defects (188). Lastly, a novel “NANOBIOME” approach based on the biobanking of exosomes secreted by MSCs has shown promise as an innovative “cell-free” regenerative medicine (182).
Genetic regulation in organisms is a highly sophisticated dynamic process. The safety of gene-modified therapies in humans still needs consideration as their improper use may lead to cell dysfunction and other side effects. For example, miRNAs can be involved in multi-target regulation and may cause adverse reactions in tissues other than the bone when applied in vivo. In addition, human manipulation of epigenetic regulation requires higher legal requirements. Therefore, convenient, rapid, efficient, and safe epigenetic modification methods are required to achieve accurate treatment of bone defects at high altitudes.
Conclusions and Recommendations For Future Research
In most in vitro experiments, researchers applied oxygen concentrations of 1–5% as hypoxic conditions and used oxygen concentrations of ~21% at standard atmospheric pressure for comparison. However, the latter is indeed much higher than the oxygen concentration in the physiological state of the tissue microenvironment, whereas the former is close to normal physiological conditions in humans [e.g., the oxygen concentration in the bone marrow is 4–7% (189)]. Furthermore, studies examining high-altitude environments are often limited to reduced oxygen content which can be misleading. First, actual oxygen concentration at the plateau is not low as all oxygen levels are ~21% between sea level to an altitude of 100,000 m. Altitude sickness is actually caused by a drop in PaO2. At this elevation, hypoxia occurs when PaO2 in the air inhaled by the human body falls below 16 kPa (2,500–3,000 m). Since the key molecule in oxygen sensing and adaptation is HIF-1, it may be a more accurate method to simulate the effect of high altitude on cells by regulating the expression of intracellular HIF-1. For example, prolyl hydroxylase inhibitors can be used to effectively increase HIF-1α protein stability (190).
At present, there is no clear optimal treatment plan for bone defects at high altitudes. The existing clinical work in high-altitude areas is mainly based on systemic oxygen therapy and bone transplantation. This is because many treatment methods involving signaling pathways and gene modifications are still in the basic research stage. Therefore, drugs targeting mitochondrial dysfunction and novel bone graft materials with oxygen-carrying capacity are urgently needed. Elabel (ELA), a peptide hormone, has been shown to promote the growth, survival, and pluripotency of human embryonic stem cells (191, 192). However, to date, ELA has only been studied for the treatment of cardiovascular diseases (193, 194), and its therapeutic effect on bone defects at high altitudes requires investigation.
As biomaterial and bone tissue engineering technology is advancing, developing biomaterials for plateau environments could be a future research hotspot. A new type of bone graft with an oxygen-carrying function can provide both local oxygen supply and bone substitute. Wang et al. (195) developed a novel oxygen sustained-release biomaterial composed of CaO2/gelatin microspheres and a 3D printed polycaprolactone/nano-HA composite porous scaffold. Their results showed that CaO2/gelatin microspheres continuously released oxygen for 19 days, improving the survival rate of transplanted BMSCs in the rabbit model by reducing local apoptosis. However, further experiments evaluating high-altitude animal models are needed to confirm the repair effect of this material on bone defects.
With the discovery of mitochondrial dysfunction, autophagy, signaling pathways, and epigenetic mechanisms of high-altitude bone defects, the development of better, targeted, personalized, and precise bone defect repair methods adjustable according to the high-altitude adaptation of patients should be expected.
Author Contributions
MR and ZL conceptualized this manuscript. YW and PC retrieved and interpreted the information. PC and YL wrote the manuscript. WL revised the manuscript. All authors contributed to the article and approved the submitted version.
Funding
This study was funded by the Natural Science Foundation of Tibet Autonomous Region (Grant No. XZ2017ZR-ZY37); Guangdong Province Science and Technology Innovation Strategy Special Fund Project (Grant No. 2018KJY2014); Scientific Research Project of Southern Medical University Stomatological Hospital (Grant No. PY2018014), and the Medical Scientific Research Foundation of Guangdong Province of China (Grant No. A2020435).
Conflict of Interest
The authors declare that the research was conducted in the absence of any commercial or financial relationships that could be construed as a potential conflict of interest.
Publisher's Note
All claims expressed in this article are solely those of the authors and do not necessarily represent those of their affiliated organizations, or those of the publisher, the editors and the reviewers. Any product that may be evaluated in this article, or claim that may be made by its manufacturer, is not guaranteed or endorsed by the publisher.
References
1. Chen H, Xu W. Changes and comparison of atmospheric pressure and oxygen pressure at different altitudes. Tibet Sci Technol. (2018) 03:59–61. doi: 10.3969/j.issn.1004-3403.2018.03.021
2. Grocott MP, Martin DS, Levett DZ, McMorrow R, Windsor J, Montgomery HE, et al. Arterial blood gases and oxygen content in climbers on Mount Everest. N Engl J Med. (2009) 360:140–9. doi: 10.1056/NEJMoa0801581
3. West JB. Early history of high-altitude physiology. Ann N Y Acad Sci. (2016) 1365:33–42. doi: 10.1111/nyas.12719
4. Thomas ED, Peter H. High-Altitude Medicine. Travel Medicine. 4h ed. Elesvier (2019). p. 387–400. doi: 10.1016/B978-0-323-54696-6.00042-2
5. Bärtsch P, Saltin B. General introduction to altitude adaptation and mountain sickness. Scand J Med Sci Sports. (2008) 18:1–10. doi: 10.1111/j.1600-0838.2008.00827.x
6. Einhorn TA, Gerstenfeld LC. Fracture healing: mechanisms and interventions. Nat Rev Rheumatol. (2015) 11:45–54. doi: 10.1038/nrrheum.2014.164
7. Camacho-Cardenosa M, Camacho-Cardenosa A, Timon R, Olcina G, Tomas-Carus P, Brazo-Sayavera J. Can hypoxic conditioning improve bone metabolism? A systematic review. Int J Environ Res Public Health. (2019) 16:1799. doi: 10.3390/ijerph16101799
8. Du G, Qin J, Duo A, Li Q, Meng G. The influence factors of fracture healing in high altitude area. Guangdong Med J. (2016). 37:1823–6.
9. Lu C, Saless N, Wang X, Sinha A, Decker S, Kazakia G, et al. The role of oxygen during fracture healing. Bone. (2013) 52:220–9. doi: 10.1016/j.bone.2012.09.037
10. Basu M, Malhotra AS, Pal K, Chatterjee T, Ghosh D, Haldar K, et al. Determination of bone mass using multisite quantitative ultrasound and biochemical markers of bone turnover during residency at extreme altitude: a longitudinal study. High Alt Med Biol. (2013) 14:150–4. doi: 10.1089/ham.2012.1042
11. Xiao J, Li Y. The influence of high-altitude and hypoxia on implant osseointegration. J Pract Stomatol. (2012) 28:10–3. doi: 10.3969/j.issn.1001-3733.2012.01.02
12. Bozzini C, Champin GM, Alippi RM, Bozzini CE. Static biomechanics in bone from growing rats exposed chronically to simulated high altitudes. High Alt Med Biol. (2013) 14:367–74. doi: 10.1089/ham.2013.1038
13. Wang W, Yun Z, Peng H-Z, Yan S-J, Zhang H-T, Qiu X-C, et al. The hypobaric hypoxia environment impairs bone strength and quality in rats. Int J Clin Exp Med. (2017) 10:9397–406. Available online at: https://e-century.us/files/ijcem/10/6/ijcem0050265.pdf
14. Zhao H, Ren X-j, Zhang X, Mei R-f. The effects of high altitude on the histological outcome of experimental bone fracture. Orthopedic J China. (1999) 6:679–81.
15. Pandolf KB, Francesconi R, Sawka MN, Cymerman A, Hoyt RW, Young AJ, et al. United States Army Research Institute of Environmental Medicine: Warfighter research focusing on the past 25 years. Adv Physiol Educ. (2011) 35:353–60. doi: 10.1152/advan.00049.2011
16. Li Q, Li X, Gao J, Ma G, Wang Y. Treatment of tibial nonunion with interlocking intramedullary nail and bone grafting in high-altitude: a report of 45 cases China J Orthopaed Traumatol. (2011) 24:468–9. doi: 10.3969/j.issn.1003-0034.2011.06.008
17. Porcelli S, Marzorati M, Healey B, Terraneo L, Vezzoli A, Bella SD, et al. Lack of acclimatization to chronic hypoxia in humans in the Antarctica. Sci Rep. (2017) 7:18090. doi: 10.1038/s41598-017-18212-1
18. Haase VH. Regulation of erythropoiesis by hypoxia-inducible factors. Blood Rev. (2013) 27:41–53. doi: 10.1016/j.blre.2012.12.003
19. Garcia P, Speidel V, Scheuer C, Laschke MW, Holstein JH, Histing T, et al. Low dose erythropoietin stimulates bone healing in mice. J Orthop Res. (2011) 29:165–72. doi: 10.1002/jor.21219
20. Holstein JH, Orth M, Scheuer C, Tami A, Becker SC, Garcia P, et al. Erythropoietin stimulates bone formation, cell proliferation, and angiogenesis in a femoral segmental defect model in mice. Bone. (2011) 49:1037–45. doi: 10.1016/j.bone.2011.08.004
21. Hiram-Bab S, Liron T, Deshet-Unger N, Mittelman M, Gassmann M, Rauner M, et al. Erythropoietin directly stimulates osteoclast precursors and induces bone loss. FASEB J. (2015) 29:1890–900. doi: 10.1096/fj.14-259085
22. Brundrett G. Sickness at high altitude: a literature review. J R Soc Health. (2002) 122:14–20. doi: 10.1177/146642400212200109
23. Liu JQ, Zelko IN, Erbynn EM, Sham JS, Folz RJ. Hypoxic pulmonary hypertension: role of superoxide and NADPH oxidase (gp91phox). Am J Physiol Lung Cell Mol Physiol. (2006) 290:L2–L10. doi: 10.1152/ajplung.00135.2005
24. Yi Y-y, Liu B, Guan L. Effects of hypoxia exposure for different time on structure and function of red blood cells in rats. Chinese J Pathophysiol. (2018) 34:130–5. doi: 10.3969/j.issn.1000-4718.2018.01.022
25. Trunk AD, Rondina MT, Kaplan DA. Venous thromboembolism at high altitude: our approach to patients at risk. High Alt Med Biol. (2019) 20:331–6. doi: 10.1089/ham.2019.0049
26. Segler CP. Prophylaxis of climbers for prevention of embolic accidents. Med Hypotheses. (2001) 57:472–5. doi: 10.1054/mehy.2001.1367
27. Tymko MM, Tremblay JC, Bailey DM, Green DJ, Ainslie PN. The impact of hypoxaemia on vascular function in lowlanders and high altitude indigenous populations. J Physiol. (2019) 597:5759–76. doi: 10.1113/JP277191
28. Li S, Ba J, Zhu P, Hou W, Ma C, Li Y, et al. The effects of different altitude on revascularization in repair of long bone defect. J Altit Med. (2004) 14:12–7. doi: 10.3969/j.issn.1007-3809.2004.04.003
29. Elefteriou F. Impact of the autonomic nervous system on the skeleton. Physiol Rev. (2018) 98:1083–112. doi: 10.1152/physrev.00014.2017
30. Zouboules SM, Lafave HC, O'Halloran KD, Brutsaert TD, Nysten HE, Nysten CE, et al. Renal reactivity: acid-base compensation during incremental ascent to high altitude. J Physiol. (2018) 596:6191–203. doi: 10.1113/JP276973
31. Swenson ER, Duncan TB, Goldberg SV, Ahmad S, Ramirez G, Schoene RB. The effect of hypoxia in humans and its relationship to the hypoxic ventilatory response. J Appl Physiol. (1995) 78:377–83. doi: 10.1152/jappl.1995.78.2.377
32. Swenson ER. Carbonic anhydrase inhibitors and ventilation: a complex interplay of stimulation and suppression. Eur Respir J. (1998) 12:1242–7. doi: 10.1183/09031936.98.12061242
33. Swenson ER. Hypoxia and its acid-base consequences: from mountains to malignancy. Adv Exp Med Biol. (2016) 903:301–23. doi: 10.1007/978-1-4899-7678-9_21
34. Arnett TR. Acidosis, hypoxia and bone. Arch Biochem Biophys. (2010) 503:103–9. doi: 10.1016/j.abb.2010.07.021
35. Hamad M, Irhimeh MR, Abbas A. Hypercapnia slows down proliferation and apoptosis of human bone marrow promyeloblasts. Bioprocess Biosyst Eng. (2016) 39:1465–75. doi: 10.1007/s00449-016-1624-7
36. Bushinsky DA, Ori Y. Effects of metabolic and respiratory acidosis on bone. Curr Opin Nephrol Hypertens. (1993) 2:588–96. doi: 10.1097/00041552-199307000-00009
37. Kato K, Morita I. Promotion of osteoclast differentiation and activation in spite of impeded osteoblast-lineage differentiation under acidosis: effects of acidosis on bone metabolism. Biosci Trends. (2013) 7:33–41. doi: 10.5582/bst.2013.v7.1.33
38. Arnett TR, Gibbons DC, Utting JC, Orriss IR, Hoebertz A, Rosendaal M, et al. Hypoxia is a major stimulator of osteoclast formation and bone resorption. J Cell Physiol. (2003) 196:2–8. doi: 10.1002/jcp.10321
39. Murray F, Insel PA, Yuan JX. Role of O(2)-sensitive K(+) and Ca(2+) channels in the regulation of the pulmonary circulation: potential role of caveolae and implications for high altitude pulmonary edema. Respir Physiol Neurobiol. (2006) 151:192–208. doi: 10.1016/j.resp.2005.10.003
40. Mori Y, Takahashi N, Ogawa N, Gudermann T. Oxygen physiology: sensors and ion channels. Pflugers Arch. (2016) 468:1–2. doi: 10.1007/s00424-015-1762-9
41. Pangalos M, Bintig W, Schlingmann B, Feyerabend F, Witte F, Begandt D, et al. Action potentials in primary osteoblasts and in the MG-63 osteoblast-like cell line. J Bioenerg Biomembr. (2011) 43:311–22. doi: 10.1007/s10863-011-9354-7
42. Bergh JJ, Shao Y, Akanbi K, Farach-Carson MC. Rodent osteoblastic cells express voltage-sensitive calcium channels lacking a gamma subunit. Calcif Tissue Int. (2003) 73:502–10. doi: 10.1007/s00223-002-0016-y
43. Kolar F, Nekar J, Ostadal B, Maslov LN, Stakheev DL, Tayurskaya AS, et al. Role of ATP-sensitive K(+)-channels in antiarrhythmic and cardioprotective action of adaptation to intermittent hypobaric hypoxia. Bull Exp Biol Med. (2008) 145:418–21. doi: 10.1007/s10517-008-0106-6
44. Shimoda LA, Polak J. Hypoxia. 4 Hypoxia and ion channel function. Am J Physiol Cell Physiol. (2011) 300:C951–967. doi: 10.1152/ajpcell.00512.2010
45. Jiang C, Haddad GG. A direct mechanism for sensing low oxygen levels by central neurons. Proc Natl Acad Sci USA. (1994) 91:7198–201. doi: 10.1073/pnas.91.15.7198
46. Osipenko ON, Tate RJ, Gurney AM. Potential role for kv3. 1b channels as oxygen sensors. Circ Res. (2000) 86:534–40. doi: 10.1161/01.RES.86.5.534
47. Patel AJ, Honoré E. Molecular physiology of oxygen-sensitive potassium channels. Eur Respir J. (2001) 18:221–7. doi: 10.1183/09031936.01.00204001
48. Gurney AM, Osipenko ON, MacMillan D, McFarlane KM, Tate RJ, Kempsill FE. Two-pore domain K channel, TASK-1, in pulmonary artery smooth muscle cells. Circ Res. (2003) 93:957–64. doi: 10.1161/01.RES.0000099883.68414.61
49. Takahashi N, Kuwaki T, Kiyonaka S, Numata T, Kozai D, Mizuno Y, et al. TRPA1 underlies a sensing mechanism for O2. Nat Chem Biol. (2011) 7:701–11. doi: 10.1038/nchembio.640
50. van der Eerden BC, Hoenderop JG, de Vries TJ, Schoenmaker T, Buurman CJ, Uitterlinden AG, et al. The epithelial Ca2+ channel TRPV5 is essential for proper osteoclastic bone resorption. Proc Natl Acad Sci USA. (2005) 102:17507–12. doi: 10.1073/pnas.0505789102
51. Lieben L, Carmeliet G. The involvement of TRP channels in bone homeostasis. Front Endocrinology. (2012) 3:99. doi: 10.3389/fendo.2012.00099
52. Franco-Obregón A, López-Barneo J. Low PO2 inhibits calcium channel activity in arterial smooth muscle cells. Am J Physiol. (1996) 271:H2290–9. doi: 10.1152/ajpheart.1996.271.6.H2290
53. Gu Y, Preston MR, el Haj AJ, Hamid J, Zamponi GW, Howl J, et al. Osteoblasts derived from load-bearing bones of the rat express both L- and T-like voltage-operated calcium channels and mRNA for alpha 1C, alpha 1D and alpha 1G subunits. Pflugers Arch. (1999) 438:553–60. doi: 10.1007/s004240051075
54. Friedrich EE, Hong Z, Xiong S, Zhong M, Di A, Rehman J, et al. Endothelial cell Piezo1 mediates pressure-induced lung vascular hyperpermeability via disruption of adherens junctions. Proc Natl Acad Sci USA. (2019) 116:12980–5. doi: 10.1073/pnas.1902165116
55. Hernansanz-Agustín P, Choya-Foces C, Carregal-Romero S, Ramos E, Oliva T, Villa-Piña T, et al. Na(+) controls hypoxic signalling by the mitochondrial respiratory chain. Nature. (2020) 586:287–91. doi: 10.1038/s41586-020-2551-y
56. Gaur P, Prasad S, Kumar B, Sharma SK, Vats P. High-altitude hypoxia induced reactive oxygen species generation, signaling, and mitigation approaches. Int J Biometeorol. (2021) 65:601–15. doi: 10.1007/s00484-020-02037-1
57. Mrakic-Sposta S, Gussoni M, Dellanoce C, Marzorati M, Montorsi M, Rasica L, et al. Effects of acute and sub-acute hypobaric hypoxia on oxidative stress: a field study in the Alps. Eur J Appl Physiol. (2021) 121:297–306. doi: 10.1007/s00421-020-04527-x
58. Tao H, Ge G, Liang X, Zhang W, Sun H, Li M, et al. ROS signaling cascades: dual regulations for osteoclast and osteoblast. Acta Biochim Biophys Sin. (2020) 52:1055–62. doi: 10.1093/abbs/gmaa098
59. Pinna A, Torki Baghbaderani M, Vigil Hernandez V, Naruphontjirakul P, Li S, McFarlane T, et al. Nanoceria provides antioxidant and osteogenic properties to mesoporous silica nanoparticles for osteoporosis treatment. Acta Biomater. (2021) 122:365–76. doi: 10.1016/j.actbio.2020.12.029
60. Liu Y, Fiskum G, Schubert D. Generation of reactive oxygen species by the mitochondrial electron transport chain. J Neurochem. (2002) 80:780–7. doi: 10.1046/j.0022-3042.2002.00744.x
61. Quinlan CL, Perevoschikova IV, Goncalves RL, Hey-Mogensen M, Brand MD. The determination and analysis of site-specific rates of mitochondrial reactive oxygen species production. Methods Enzymol. (2013) 526:189–217. doi: 10.1016/B978-0-12-405883-5.00012-0
62. Fuhrmann DC, Brune B. Mitochondrial composition and function under the control of hypoxia. Redox Biol. (2017) 12:208–15. doi: 10.1016/j.redox.2017.02.012
63. Tello D, Balsa E, Acosta-Iborra B, Fuertes-Yebra E, Elorza A, Ordóñez Á, et al. Induction of the mitochondrial NDUFA4L2 protein by HIF-1alpha decreases oxygen consumption by inhibiting Complex I activity. Cell Metab. (2011) 14:768–79. doi: 10.1016/j.cmet.2011.10.008
64. Fukuda R, Zhang H, Kim JW, Shimoda L, Dang CV, Semenza GL. HIF-1 regulates cytochrome oxidase subunits to optimize efficiency of respiration in hypoxic cells. Cell. (2007) 129:111–22. doi: 10.1016/j.cell.2007.01.047
65. Bovo E, Mazurek SR, de Tombe PP, Zima AV. Increased energy demand during adrenergic receptor stimulation contributes to Ca(2+) wave generation. Biophys J. (2015) 109:1583–91. doi: 10.1016/j.bpj.2015.09.002
66. Saleem N, Goswami SK. Activation of adrenergic receptor in H9c2 cardiac myoblasts co-stimulates Nox2 and the derived ROS mediate the downstream responses. Mol Cell Biochem. (2017) 436:167–78. doi: 10.1007/s11010-017-3088-8
67. Tsai MH, Jiang MJ. Reactive oxygen species are involved in regulating alpha1-adrenoceptor-activated vascular smooth muscle contraction. J Biomed Sci. (2010) 17:67. doi: 10.1186/1423-0127-17-67
68. Jiang C, Cui J, Liu F, Gao L, Luo Y, Li P, et al. Mitochondrial DNA 10609T promotes hypoxia-induced increase of intracellular ROS and is a risk factor of high altitude polycythemia. PLoS ONE. (2014) 9:e87775. doi: 10.1371/journal.pone.0087775
69. Shimasaki M, Ueda S, Ichiseki T, Hirata H, Kawahara N, Ueda Y. Resistance of bone marrow mesenchymal stem cells in a stressed environment - Comparison with osteocyte cells. Int J Med Sci. (2021) 18:1375–81. doi: 10.7150/ijms.52104
70. Yang YD Li MM, Xu G, Zhang EL, Chen J, Sun B, et al. Targeting mitochondria-associated membranes as a potential therapy against endothelial injury induced by hypoxia. J Cell Biochem. (2019) 120:18967–78. doi: 10.1002/jcb.29220
71. Cillero-Pastor B, Martin MA, Arenas J, López-Armada MJ, Blanco FJ. Effect of nitric oxide on mitochondrial activity of human synovial cells. BMC Musculoskelet Disord. (2011) 12:42–4. doi: 10.1186/1471-2474-12-42
72. Hsu SH, Chen CT, Wei YH. Inhibitory effects of hypoxia on metabolic switch and osteogenic differentiation of human mesenchymal stem cells. Stem Cells. (2013) 31:2779–88. doi: 10.1002/stem.1441
73. Chai P, Cheng Y, Hou C, Yin L, Zhang D, Hu Y, et al. USP19 promotes hypoxia-induced mitochondrial division via FUNDC1 at ER-mitochondria contact sites. J Cell Biol. (2021) 220:e202010006. doi: 10.1083/jcb.202010006
74. Wu W, Li W, Chen H, Jiang L, Zhu R, Feng D. FUNDC1 is a novel mitochondrial-associated-membrane (MAM) protein required for hypoxia-induced mitochondrial fission and mitophagy. Autophagy. (2016) 12:1675–6. doi: 10.1080/15548627.2016.1193656
75. MacVicar T, Ohba Y, Nolte H, Mayer FC, Tatsuta T, Sprenger HG, et al. Lipid signalling drives proteolytic rewiring of mitochondria by YME1L. Nature. (2019) 575:361–5. doi: 10.1038/s41586-019-1738-6
76. Ferri A, Yan X, Kuang J, Granata C, Oliveira RSF, Hedges CP, et al. Fifteen days of moderate normobaric hypoxia does not affect mitochondrial function, and related genes and proteins, in healthy men. Eur J Appl Physiol. (2021) 121:2323–36. doi: 10.1007/s00421-021-04706-4
78. Kim JW, Tchernyshyov I, Semenza GL, Dang CV. HIF-1-mediated expression of pyruvate dehydrogenase kinase: a metabolic switch required for cellular adaptation to hypoxia. Cell Metab. (2006) 3:177–85. doi: 10.1016/j.cmet.2006.02.002
79. Li Q, Lin K, Sun H, Liu S, Huang K, Huang X, et al. Mitochondrial haplogroup M9a1a1c1b is associated with hypoxic adaptation in the Tibetans. J Hum Genet. (2016) 61:1021–6. doi: 10.1038/jhg.2016.95
80. Shi Y, Hu Y, Wang J, Elzo MA, Yang X, Lai S. Genetic diversities of MT-ND1 MT-ND2 genes are associated with high-altitude adaptation in yak. Mitochond DNA Part A DNA Mapp Sequenc Anal. (2018) 29:485–94. doi: 10.1080/24701394.2017.1307976
81. White CR, Alton LA, Frappell PB. Metabolic cold adaptation in fishes occurs at the level of whole animal, mitochondria and enzyme. Proc Biol Sci. (2012) 279:1740–7. doi: 10.1098/rspb.2011.2060
82. Gniadecki R, Thorn T, Vicanova J, Petersen A, Wulf HC. Role of mitochondria in ultraviolet-induced oxidative stress. J Cell Biochem. (2000) 80:216–22. doi: 10.1002/1097-4644(20010201)80:2<216::AID-JCB100>3.0.CO;2-H
83. Valencia A, Rajadurai A, Carle AB, Kochevar IE. 7-Dehydrocholesterol enhances ultraviolet A-induced oxidative stress in keratinocytes: roles of NADPH oxidase, mitochondria, and lipid rafts. Free Radic Biol Med. (2006) 41:1704–18. doi: 10.1016/j.freeradbiomed.2006.09.006
84. Luo B, Li J, Yang T, Li W, Zhang J, Wang C, et al. Evaluation of renal excretion and pharmacokinetics of furosemide in rats after acute exposure to high altitude at 4300 m. Biopharm Drug Dispos. (2018) 39:378–87. doi: 10.1002/bdd.2154
85. Zhang Y, Chen N. Autophagy is a promoter for aerobic exercise performance during high altitude training. Oxid Med Cell Longev. (2018) 2018:3617508. doi: 10.1155/2018/3617508
86. Xiao L, Xiao Y. The autophagy in osteoimmonology: self-eating, maintenance, and beyond. Front Endocrinol. (2019) 10:490. doi: 10.3389/fendo.2019.00490
87. Li Y, Sun R, Zou J, Ying Y, Luo Z. Dual roles of the AMP-activated protein kinase pathway in angiogenesis. Cells. (2019) 8:752. doi: 10.3390/cells8070752
88. Nollet M, Santucci-Darmanin S, Breuil V, Al-Sahlanee R, Cros C, Topi M, et al. Autophagy in osteoblasts is involved in mineralization and bone homeostasis. Autophagy. (2014) 10:1965–77. doi: 10.4161/auto.36182
89. Zahm AM, Bohensky J, Adams CS, Shapiro IM, Srinivas V. Bone cell autophagy is regulated by environmental factors. Cells Tissues Organs. (2011) 194:274–8. doi: 10.1159/000324647
90. Glick D, Barth S, Macleod KF. Autophagy: cellular and molecular mechanisms. J Pathol. (2010) 221:3–12. doi: 10.1002/path.2697
91. Naldini A, Morena E, Pucci A, Miglietta D, Riboldi E, Sozzani S, et al. Hypoxia affects dendritic cell survival: role of the hypoxia-inducible factor-1alpha and lipopolysaccharide. J Cell Physiol. (2012) 227:587–95. doi: 10.1002/jcp.22761
92. Alers S, Löffler AS, Wesselborg S, Stork B. Role of AMPK-mTOR-Ulk1/2 in the regulation of autophagy: cross talk, shortcuts, and feedbacks. Mol Cell Biol. (2012) 32:2–11. doi: 10.1128/MCB.06159-11
93. Mohammed Abdul KS, Jovanović S, Jovanović A. Exposure to 15% oxygen in vivo up-regulates cardioprotective SUR2A without affecting ERK1/2 and AKT: a crucial role for AMPK. J Cell Mol Med. (2017) 21:1342–50. doi: 10.1111/jcmm.13064
94. Nie X, Wang W, Wang Q, Zhu D, Song H. Intranasal erythropoietin ameliorates neurological function impairments and neural pathology in mice with chronic alcoholism by regulating autophagyrelated Nrf2 degradation. Mol Med Rep. (2019) 19:1139–49. doi: 10.3892/mmr.2018.9706
95. Wu J, Niu J, Li X, Li Y, Wang X, Lin J, et al. Hypoxia induces autophagy of bone marrow-derived mesenchymal stem cells via activation of ERK1/2. Cell Physiol Biochem. (2014) 33:1467–74. doi: 10.1159/000358711
96. Yang Q, Lu Z, Ramchandran R, Longo LD, Raj JU. Pulmonary artery smooth muscle cell proliferation and migration in fetal lambs acclimatized to high-altitude long-term hypoxia: role of histone acetylation. Am J Physiol Lung Cell Mol Physiol. (2012) 303:L1001–10. doi: 10.1152/ajplung.00092.2012
97. Hu Y, Sun Q, Li Z, Chen J, Shen C, Song Y, et al. High basal level of autophagy in high-altitude residents attenuates myocardial ischemia-reperfusion injury. J Thorac Cardiovasc Surg. (2014) 148:1674–80. doi: 10.1016/j.jtcvs.2014.03.038
98. Zhang B, Ban D, Gou X, Zhang Y, Yang L, Chamba Y, et al. Genome-wide DNA methylation profiles in Tibetan and Yorkshire pigs under high-altitude hypoxia. J Anim Sci Biotechnol. (2019) 10:25. doi: 10.1186/s40104-019-0316-y
99. Childebayeva A, Harman T, Weinstein J, Goodrich JM, Dolinoy DC, Day TA, et al. DNA methylation changes are associated with an incremental ascent to high altitude. Front Genet. (2019) 10:1062. doi: 10.3389/fgene.2019.01062
100. Nanduri J, Semenza GL, Prabhakar NR. Epigenetic changes by DNA methylation in chronic and intermittent hypoxia. Am J Physiol Lung Cell Mol Physiol. (2017) 313:L1096–100. doi: 10.1152/ajplung.00325.2017
101. Wenger RH, Kvietikova I, Rolfs A, Camenisch G, Gassmann M. Oxygen-regulated erythropoietin gene expression is dependent on a CpG methylation-free hypoxia-inducible factor-1 DNA-binding site. Eur J Biochem. (1998) 253:771–7. doi: 10.1046/j.1432-1327.1998.2530771.x
102. Yang M, Zhu M, Song K, Wuren T, Yan J, Ge RL, et al. VHL gene methylation contributes to excessive erythrocytosis in chronic mountain sickness rat model by upregulating the HIF-2alpha/EPO pathway. Life Sci. (2021) 266:118873. doi: 10.1016/j.lfs.2020.118873
103. Hatzimichael E, Dranitsaris G, Dasoula A, Benetatos L, Stebbing J, Crook T, et al. Von Hippel-Lindau methylation status in patients with multiple myeloma: a potential predictive factor for the development of bone disease. Clin Lymphoma Myeloma. (2009) 9:239–42. doi: 10.3816/CLM.2009.n.047
104. Wellmann S, Bettkober M, Zelmer A, Seeger K, Faigle M, Eltzschig HK, et al. Hypoxia upregulates the histone demethylase JMJD1A via HIF-1. Biochem Biophys Res Commun. (2008) 372:892–7. doi: 10.1016/j.bbrc.2008.05.150
105. Beyer S, Kristensen MM, Jensen KS, Johansen JV, Staller P. The histone demethylases JMJD1A and JMJD2B are transcriptional targets of hypoxia-inducible factor HIF. J Biol Chem. (2008) 283:36542–52. doi: 10.1074/jbc.M804578200
106. Chen F, Wang RJ, Li GZ, Zhang Y, Yu S, Liu YF, et al. miRNA array analysis of plasma miRNA alterations in rats exposed to a high altitude hypoxic environment. Mol Med Rep. (2018) 18:5502–10. doi: 10.3892/mmr.2018.9570
107. Yang C, Liu X, Zhao K, Zhu Y, Hu B, Zhou Y, et al. miRNA-21 promotes osteogenesis via the PTEN/PI3K/Akt/HIF-1alpha pathway and enhances bone regeneration in critical size defects. Stem Cell Res Ther. (2019) 10:65. doi: 10.1186/s13287-019-1168-2
108. Shi XF, Wang H, Xiao FJ, Yin Y, Xu QQ, Ge RL, et al. MiRNA-486 regulates angiogenic activity and survival of mesenchymal stem cells under hypoxia through modulating Akt signal. Biochem Biophys Res Commun. (2016) 470:670–7. doi: 10.1016/j.bbrc.2016.01.084
109. Gu H, Li Z, Lv XF, Zhao AB, Zhu MY, Zhang Y. LncRNA KCNQ1OT1 delayed fracture healing through the Wnt/beta-catenin pathway. Eur Rev Med Pharmacol Sci. (2019) 23:4575–83. doi: 10.26355/eurrev_201906_18034
110. Liu W, Li L, Rong Y, Qian D, Chen J, Zhou Z, et al. Hypoxic mesenchymal stem cell-derived exosomes promote bone fracture healing by the transfer of miR-126. Acta Biomater. (2020) 103:196–212. doi: 10.1016/j.actbio.2019.12.020
111. Sun J, Feng H, Xing W, Han Y, Suo J, Yallowitz AR, et al. Histone demethylase LSD1 is critical for endochondral ossification during bone fracture healing. Sci Adv. (2020) 6:eaaz1410. doi: 10.1126/sciadv.aaz1410
113. Kürklü M, Yurttaş Y, Köse O, Demiralp B, Yüksel HY, Kömürcü M. Adjunctive hyperbaric oxygen therapy in the treatment of atrophic tibial nonunion with Ilizarov external fixator: a radiographic and scintigraphic study in rabbits. Acta Orthop Traumatol Turc. (2012) 46:126–31. doi: 10.3944/AOTT.2012.2586
114. Pedersen TO, Xing Z, Finne-Wistrand A, Hellem S, Mustafa K. Hyperbaric oxygen stimulates vascularization and bone formation in rat calvarial defects. Int J Oral Maxillofac Surg. (2013) 42:907–14. doi: 10.1016/j.ijom.2013.01.003
115. Izumino J, Kaku M, Yamamoto T, Yashima Y, Kagawa H, Ikeda K, et al. Effects of hyperbaric oxygen treatment on calvarial bone regeneration in young and adult mice. Arch Oral Biol. (2020) 117:104828. doi: 10.1016/j.archoralbio.2020.104828
116. Grassmann JP, Schneppendahl J, Hakimi AR, Herten M, Betsch M, Logters TT, et al. Hyperbaric oxygen therapy improves angiogenesis and bone formation in critical sized diaphyseal defects. J Orthop Res. (2015) 33:513–20. doi: 10.1002/jor.22805
117. Sanford NE, Wilkinson JE, Nguyen H, Diaz G, Wolcott R. Efficacy of hyperbaric oxygen therapy in bacterial biofilm eradication. J Wound Care. (2018) 27(Suppl. 1):S20–8. doi: 10.12968/jowc.2018.27.Sup1.S20
118. Bressan E, Ferroni L, Gardin C, Bellin G, Sbricoli L, Sivolella S, et al. Metal nanoparticles released from dental implant surfaces: potential contribution to chronic inflammation and peri-implant bone loss. Materials. (2019) 12:2036. doi: 10.3390/ma12122036
119. Noronha Oliveira M, Schunemann WVH, Mathew MT, Henriques B, Magini RS, Teughels W, et al. Can degradation products released from dental implants affect peri-implant tissues? J Periodontal Res. (2018) 53:1–11. doi: 10.1111/jre.12479
120. Oh SE, Hu KS, Kim S. Eight-week healing of grafted calvarial bone defects with hyperbaric oxygen therapy in rats. J Periodontal Implant Sci. (2019) 49:228–36. doi: 10.5051/jpis.2019.49.4.228
121. Juan L, Peng L, Mengjun W, Yandong M. Impact of hyperbaric oxygen on the healing of bone tissues around implants. Implant Dent. (2018) 27:653–9. doi: 10.1097/ID.0000000000000825
122. Sun IF, Lee SS, Chiu CC, Lin SD, Lai CS. Hyperbaric oxygen therapy with topical negative pressure: an alternative treatment for the refractory sternal wound infection. J Card Surg. (2008) 23:677–80. doi: 10.1111/j.1540-8191.2008.00689.x
123. Hwang SC, Hwang DS, Kim HY, Kim MJ, Kang YH, Byun SH, et al. Development of bone regeneration strategies using human periosteum-derived osteoblasts and oxygen-releasing microparticles in mandibular osteomyelitis model of miniature pig. J Biomed Mater Res A. (2019) 107:2183–94. doi: 10.1002/jbm.a.36728
124. Kim HY, Kim SY, Lee HY, Lee JH, Rho GJ, Lee HJ, et al. Oxygen-releasing microparticles for cell survival and differentiation ability under hypoxia for effective bone regeneration. Biomacromolecules. (2019) 20:1087–97. doi: 10.1021/acs.biomac.8b01760
125. Huang LY, Yen IC, Tsai WC, Lee SY. Rhodiola crenulata suppresses high glucose-induced matrix metalloproteinase expression and inflammatory responses by inhibiting ROS-related HMGB1-TLR4 signaling in endothelial cells. Am J Chin Med. (2020) 48:91–105. doi: 10.1142/S0192415X20500056
126. Guo Q, Yang J, Chen Y, Jin X, Li Z, Wen X, et al. Salidroside improves angiogenesis-osteogenesis coupling by regulating the HIF-1alpha/VEGF signalling pathway in the bone environment. Eur J Pharmacol. (2020) 884:173394. doi: 10.1016/j.ejphar.2020.173394
127. Chen JJ, Zhang NF, Mao GX, He XB, Zhan YC, Deng HB, et al. Salidroside stimulates osteoblast differentiation through BMP signaling pathway. Food Chem Toxicol. (2013) 62:499–505. doi: 10.1016/j.fct.2013.09.019
128. Zhang W, Zhang W, Huo L, Chai Y, Liu Z, Ren Z, et al. Rosavin suppresses osteoclastogenesis in vivo and in vitro by blocking the nuclear factor kappa-light-chain-enhancer of activated B cells (NF-kappaB) and mitogen-activated protein kinase (MAPK) signaling pathways. Ann Transl Med. (2021) 9:383. doi: 10.21037/atm-20-4255
129. Steinbrenner H, Sies H. Protection against reactive oxygen species by selenoproteins. Biochim Biophys Acta. (2009) 1790:1478–85. doi: 10.1016/j.bbagen.2009.02.014
130. Austermann K, Baecker N, Zwart SR, Fimmers R, Frippiat JP, Stehle P, et al. Antioxidant supplementation does not affect bone turnover markers during 60 days of 6 degrees head-down tilt bed rest: results from an exploratory randomized controlled trial. J Nutr. (2021) 151:1527–38. doi: 10.1093/jn/nxab036
131. Aghajanian P, Hall S, Wongworawat MD, Mohan S. The roles and mechanisms of actions of vitamin C in bone: new developments. J Bone Miner Res. (2015) 30:1945–55. doi: 10.1002/jbmr.2709
132. Yingcharoenthana S, Ampornaramveth R, Subbalekha K, Sinpitaksakul P, Kamolratanakul P. A split-mouth randomized clinical trial to evaluate the effect of local and systemic administration of vitamin C on extraction wound healing. J Oral Sci. (2021) 63:198–200. doi: 10.2334/josnusd.20-0515
133. Huang HT, Cheng TL, Lin SY, Ho CJ, Chyu JY, Yang RS, et al. Osteoprotective roles of green tea catechins. Antioxidants. (2020) 9:1136. doi: 10.3390/antiox9111136
134. Shan Z, Luo ZP, Shen X, Chen L. Promotion of fracture healing by conjugated linoleic acid in rats. J Orthop Surg. (2017) 25:2309499017718910. doi: 10.1177/2309499017718910
135. Balci Yuce H, Akbulut N, Ocakli S, Kayir O, Elmastas M. The effect of commercial conjugated linoleic acid products on experimental periodontitis and diabetes mellitus in Wistar rats. Acta Odontol Scand. (2017) 75:21–9. doi: 10.1080/00016357.2016.1244355
136. Fitzpatrick LA, Smith PL, McBride TA, Fries MA, Hossain M, Dabrowski CE, et al. Ronacaleret, a calcium-sensing receptor antagonist, has no significant effect on radial fracture healing time: results of a randomized, double-blinded, placebo-controlled Phase II clinical trial. Bone. (2011) 49:845–52. doi: 10.1016/j.bone.2011.06.017
137. Fiorani M, Guidarelli A, Cantoni O. Mitochondrial reactive oxygen species: the effects of mitochondrial ascorbic acid vs. untargeted and mitochondria-targeted antioxidants. Int J Radiat Biol. (2021) 97:1055–62. doi: 10.1080/09553002.2020.1721604
138. Ilyas A, Odatsu T, Shah A, Monte F, Kim HK, Kramer P, et al. Amorphous silica: a new antioxidant role for rapid critical-sized bone defect healing. Adv Healthc Mater. (2016) 5:2199–213. doi: 10.1002/adhm.201600203
139. do Monte FA, Ahuja N, Awad KR, Pan Z, Young S, Kim HK, et al. ROS silicon oxynitrophosphide nanoscale coating enhances antioxidant marker-induced angiogenesis during in vivo cranial bone-defect healing. JBMR Plus. (2021) 5:e10425. doi: 10.1002/jbm4.10425
140. Jahangir S, Hosseini S, Mostafaei F, Sayahpour FA, Baghaban Eslaminejad M. 3D-porous beta-tricalcium phosphate-alginate-gelatin scaffold with DMOG delivery promotes angiogenesis and bone formation in rat calvarial defects. J Mater Sci Mater Med. (2018) 30:1. doi: 10.1007/s10856-018-6202-x
141. Jin X, Han D, Tao J, Huang Y, Zhou Z, Zhang Z, et al. Dimethyloxallyl glycine-incorporated borosilicate bioactive glass scaffolds for improving angiogenesis and osteogenesis in critical-sized calvarial defects. Curr Drug Deliv. (2019) 16:565–76. doi: 10.2174/1567201816666190611105205
142. Lim HC, Thoma DS, Jeon M, Song JS, Lee SK, Jung UW. Effect of hypoxia-inducible factor 1alpha on early healing in extraction sockets. Biomed Res Int. (2018) 18:8210637. doi: 10.1155/2018/8210637
143. Oh SM, Shin JS, Kim IK, Kim JH, Moon JS, Lee SK, et al. Therapeutic effects of HIF-1alpha on bone formation around implants in diabetic mice using cell-penetrating DNA-binding protein. Molecules. (2019) 24:760. doi: 10.3390/molecules24040760
144. Sha Y, Lv Y, Xu Z, Yang L, Hao X, Afandi R. MGF E peptide pretreatment improves the proliferation and osteogenic differentiation of BMSCs via MEK-ERK1/2 and PI3K-Akt pathway under severe hypoxia. Life Sci. (2017) 189:52–62. doi: 10.1016/j.lfs.2017.09.017
145. Cheng MS, Yi X, Zhou Q. Overexpression of HIF-1alpha in bone marrow mesenchymal stem cells promote the repair of mandibular condylar osteochondral defect in a rabbit model. J Oral Maxillofac Surg. (2021) 79:345.e1–e15. doi: 10.1016/j.joms.2020.10.013
146. Wang P, Meng Q, Wang W, Zhang S, Xiong X, Qin S, et al. Icariin inhibits the inflammation through down-regulating NF-kappaB/HIF-2alpha signal pathways in chondrocytes. Biosci Rep. (2020) 40:BSR20203107. doi: 10.1042/BSR20203107
147. Lin J, Xu R, Shen X, Jiang H, Du S. Metformin promotes the osseointegration of titanium implants under osteoporotic conditions by regulating BMSCs autophagy, and osteogenic differentiation. Biochem Biophys Res Commun. (2020) 531:228–35. doi: 10.1016/j.bbrc.2020.06.146
148. Akram Z, Vohra F, Javed F. Locally delivered metformin as adjunct to scaling and root planing in the treatment of periodontal defects: a systematic review and meta-analysis. J Periodontal Res. (2018) 53:941–9. doi: 10.1111/jre.12573
149. Xu R, Shi G, Xu L, Gu Q, Fu Y, Zhang P, et al. Simvastatin improves oral implant osseointegration via enhanced autophagy and osteogenesis of BMSCs and inhibited osteoclast activity. J Tissue Eng Regen Med. (2018) 12:1209–19. doi: 10.1002/term.2652
150. Song QS, Wang L, Zhu J, Han X, Li X, Yang Y, et al. Effect of simvastatin on inducing endothelial progenitor cells homing and promoting bone defect repair. Chin J Repar Reconstr Surg. (2010) 24:1103–6. Available online at: http://www.rrsurg.com/indexAction!downloadPdfByOss.action?id=MjAyNTI=
151. Dang L, Zhu J, Song C. The effect of topical administration of simvastatin on entochondrostosis and intramembranous ossification: An animal experiment. J Orthop Translat. (2021) 28:1–9. doi: 10.1016/j.jot.2020.11.009
152. Fan D, Liu H, Zhang Z, Su M, Yuan Z, Lin Y, et al. Resveratrol and angiogenin-2 combined with PEGDA/TCS hydrogel for the targeted therapy of hypoxic bone defects via activation of the autophagy pathway. Front Pharmacol. (2021) 12:618724. doi: 10.3389/fphar.2021.618724
153. Yin J, Gong G, Sun C, Yin Z, Zhu C, Wang B, et al. Angiopoietin 2 promotes angiogenesis in tissue-engineered bone and improves repair of bone defects by inducing autophagy. Biomed Pharmacother. (2018) 105:932–9. doi: 10.1016/j.biopha.2018.06.078
154. Yin ZY, Yin J, Huo YF, Yu J, Sheng LX, Dong YF. Rapamycin facilitates fracture healing through inducing cell autophagy and suppressing cell apoptosis in bone tissues. Eur Rev Med Pharmacol Sci. (2017) 21:4989–98. Available online at: http://www.europeanreview.org/wp/wp-content/uploads/4989-4998-Rapamycin-facilitates-the-fracture-healing.pdf
155. Suresh S, Rajvanshi PK, Noguchi CT. The many facets of erythropoietin physiologic and metabolic response. Front Physiol. (2020) 10:1534. doi: 10.3389/fphys.2019.01534
156. Wang K, Fu XP, Yang ZH, Zheng Y, Gao Z, Gao CY, et al. Bone marrow mesenchymal stem cell transplantation for repair of rat femoral defects at high altitude. Chin J Tissue Eng Res. (2014) 18:2185–90. doi: 10.3969/j.issn.2095-4344.2014.14.009
157. Chen C, Yan S, Qiu S, Geng Z, Wang Z. HIF/Ca(2+)/NO/ROS is critical in roxadustat treating bone fracture by stimulating the proliferation and migration of BMSCs. Life Sci. (2021) 264:118684. doi: 10.1016/j.lfs.2020.118684
158. Zhou M, Xi J, Cheng Y, Sun D, Shu P, Chi S, et al. Reprogrammed mesenchymal stem cells derived from iPSCs promote bone repair in steroid-associated osteonecrosis of the femoral head. Stem Cell Res Ther. (2021) 12:175. doi: 10.1186/s13287-021-02249-1
159. Sato T, Anada T, Hamai R, Shiwaku Y, Tsuchiya K, Sakai S, et al. Culture of hybrid spheroids composed of calcium phosphate materials and mesenchymal stem cells on an oxygen-permeable culture device to predict in vivo bone forming capability. Acta Biomater. (2019) 88:477–90. doi: 10.1016/j.actbio.2019.03.001
160. Govoni M, Muscari C, Bonafe F, Morselli PG, Cortesi M, Dallari D, et al. A brief very-low oxygen tension regimen is sufficient for the early chondrogenic commitment of human adipose-derived mesenchymal stem cells. Adv Med Sci. (2021) 66:98–104. doi: 10.1016/j.advms.2020.12.005
161. Silva DDD, Paz A, Portinho CP, Lima EOC, Kliemann LM, Collares MVM. Reconstruction of parietal bone defects with adiposederived mesenchymal stem cells. Experimental study Acta Cir Bras. (2021) 35:e351201. doi: 10.1590/acb351201
162. Byun HY, Jang GN, Lee J, Hong MH, Shin H, Shin H. Stem cell spheroid engineering with osteoinductive and ROS scavenging nanofibers for bone regeneration. Biofabrication. (2020) 10:1088. doi: 10.1088/1758-5090/abd56c
163. Zheng X, Zhang X, Wang Y, Liu Y, Pan Y, Li Y, et al. Hypoxia-mimicking 3D bioglass-nanoclay scaffolds promote endogenous bone regeneration. Bioact Mater. (2021) 6:3485–95. doi: 10.1016/j.bioactmat.2021.03.011
164. Takebe T, Zhang RR, Koike H, Kimura M, Yoshizawa E, Enomura M, et al. Generation of a vascularized and functional human liver from an iPSC-derived organ bud transplant. Nat Protoc. (2014) 9:396–409. doi: 10.1038/nprot.2014.020
165. Takebe T, Enomura M, Yoshizawa E, Kimura M, Koike H, Ueno Y, et al. Vascularized and complex organ buds from diverse tissues via mesenchymal cell-driven condensation. Cell Stem Cell. (2015) 16:556–65. doi: 10.1016/j.stem.2015.03.004
166. Ren B, Betz VM, Thirion C, Salomon M, Klar RM, Jansson V, et al. Gene activated adipose tissue fragments as advanced autologous biomaterials for bone regeneration: osteogenic differentiation within the tissue and implications for clinical translation. Sci Rep. (2019) 9:224. doi: 10.1038/s41598-018-36283-6
167. Bougioukli S, Sugiyama O, Alluri RK, Yoho R, Oakes DA, Lieberman JR. In vitro evaluation of a lentiviral two-step transcriptional amplification system using GAL4FF transactivator for gene therapy applications in bone repair. Gene Ther. (2018) 25:260–8. doi: 10.1038/s41434-018-0024-9
168. He W, Chen L, Huang Y, Xu Z, Xu W, Ding N, et al. Synergistic effects of recombinant Lentiviral-mediated BMP2 and TGF-beta3 on the osteogenic differentiation of rat bone marrow mesenchymal stem cells in vitro. Cytokine. (2019) 120:1–8. doi: 10.1016/j.cyto.2019.03.020
169. He J, Han X, Wang S, Zhang Y, Dai X, Liu B, et al. Cell sheets of co-cultured BMP-2-modified bone marrow stromal cells and endothelial progenitor cells accelerate bone regeneration in vitro. Exp Ther Med. (2019) 18:3333–40. doi: 10.3892/etm.2019.7982
170. Liu X, Bao C, Xu HHK, Pan J, Hu J, Wang P, et al. Osteoprotegerin gene-modified BMSCs with hydroxyapatite scaffold for treating critical-sized mandibular defects in ovariectomized osteoporotic rats. Acta Biomater. (2016) 42:378–88. doi: 10.1016/j.actbio.2016.06.019
171. Yanagihara K, Uchida S, Ohba S, Kataoka K, Itaka K. Treatment of bone defects by transplantation of genetically modified mesenchymal stem cell spheroids. Mol Ther Methods Clin Dev. (2018) 9:358–66. doi: 10.1016/j.omtm.2018.04.006
172. Song X, Shi L, Chen L, Liu X, Qu X, Wang K, et al. Endothelial cells modified by adenovirus vector containing nine copies hypoxia response elements and human vascular endothelial growth factor as the novel seed cells for bone tissue engineering. Acta Biochim Biophys Sin. (2017) 49:973–8. doi: 10.1093/abbs/gmx101
173. Wang Z, Zhang D, Hu Z, Cheng J, Zhuo C, Fang X, et al. MicroRNA-26a-modified adipose-derived stem cells incorporated with a porous hydroxyapatite scaffold improve the repair of bone defects. Mol Med Rep. (2015) 12:3345–50. doi: 10.3892/mmr.2015.3795
174. Shi L, Feng L, Liu Y, Duan JQ, Lin WP, Zhang JF, et al. MicroRNA-218 promotes osteogenic differentiation of mesenchymal stem cells and accelerates bone fracture healing. Calcif Tissue Int. (2018) 103:227–36. doi: 10.1007/s00223-018-0410-8
175. Lee WY, Li N, Lin S, Wang B, Lan HY, Li G. miRNA-29b improves bone healing in mouse fracture model. Mol Cell Endocrinol. (2016) 430:97–107. doi: 10.1016/j.mce.2016.04.014
176. Hupkes M, Sotoca AM, Hendriks JM, van Zoelen EJ, Dechering KJ. MicroRNA miR-378 promotes BMP2-induced osteogenic differentiation of mesenchymal progenitor cells. BMC Mol Biol. (2014) 15:1. doi: 10.1186/1471-2199-15-1
177. Xing Y, Hou J, Guo T, Zheng S, Zhou C, Huang H, et al. microRNA-378 promotes mesenchymal stem cell survival and vascularization under hypoxic-ischemic conditions in vitro. Stem Cell Res Ther. (2014) 5:130. doi: 10.1186/scrt520
178. Lv C, Yang S, Chen X, Zhu X, Lin W, Wang L, et al. MicroRNA-21 promotes bone mesenchymal stem cells migration in vitro by activating PI3K/Akt/MMPs pathway. J Clin Neurosci. (2017) 46:156–62. doi: 10.1016/j.jocn.2017.07.040
179. Liu Y, Liu J, Xia T, Mi BB, Xiong Y, Hu LC, et al. MiR-21 promotes fracture healing by activating the PI3K/Akt signaling pathway. Eur Rev Med Pharmacol Sci. (2019) 23:2727–33. doi: 10.26355/eurrev_201904_17544
180. Zhang Z, Jiang W, Hu M, Gao R, Zhou X. MiR-486-3p promotes osteogenic differentiation of BMSC by targeting CTNNBIP1 and activating the Wnt/beta-catenin pathway. Biochem Biophys Res Commun. (2021) 566:59–66. doi: 10.1016/j.bbrc.2021.05.098
181. Zhang Y, Hao Z, Wang P, Xia Y, Wu J, Xia D, et al. Exosomes from human umbilical cord mesenchymal stem cells enhance fracture healing through HIF-1alpha-mediated promotion of angiogenesis in a rat model of stabilized fracture. Cell Prolif. (2019) 52:e12570. doi: 10.1111/cpr.12570
182. Codispoti B, Marrelli M, Paduano F, Tatullo M. NANOmetric BIO-banked MSC-derived exosome (NANOBIOME) as a novel approach to regenerative medicine. J Clin Med. (2018) 7:357. doi: 10.3390/jcm7100357
183. Hu H, Wang D, Li L, Yin H, He G, Zhang Y. Role of microRNA-335 carried by bone marrow mesenchymal stem cells-derived extracellular vesicles in bone fracture recovery. Cell Death Dis. (2021) 12:156. doi: 10.1038/s41419-021-03430-3
184. Shao YZ, Chen S, Zhou YS. Applications of stem cell-derived extracellular vesicles in bone regenerative therapy. Chin J Stomatol. (2020) 55:206–11. doi: 10.3760/cma.j.issn.1002-0098.2020.03.013
185. Bister N, Pistono C, Huremagic B, Jolkkonen J, Giugno R, Malm T. Hypoxia and extracellular vesicles: A review on methods, vesicular cargo and functions. J Extracell Vesicles. (2020) 10:e12002. doi: 10.1002/jev2.12002
186. Jiang S, Tian G, Yang Z, Gao X, Wang F, Li J, et al. Enhancement of acellular cartilage matrix scaffold by Wharton's jelly mesenchymal stem cell-derived exosomes to promote osteochondral regeneration. Bioact Mater. (2021) 6:2711–28. doi: 10.1016/j.bioactmat.2021.01.031
187. Liu A, Lin D, Zhao H, Chen L, Cai B, Lin K, et al. Optimized BMSC-derived osteoinductive exosomes immobilized in hierarchical scaffold via lyophilization for bone repair through Bmpr2/Acvr2b competitive receptor-activated Smad pathway. Biomaterials. (2021) 272:120718. doi: 10.1016/j.biomaterials.2021.120718
188. Wan Safwani WK, Wong CW, Yong KW, Choi JR, Mat Adenan NA, Omar SZ, et al. The effects of hypoxia and serum-free conditions on the stemness properties of human adipose-derived stem cells. Cytotechnology. (2016) 68:1859–72. doi: 10.1007/s10616-015-9939-9
189. Ko SH, Nauta AC, Morrison SD, Hu MS, Zimmermann AS, Chung MT, et al. PHD-2 suppression in mesenchymal stromal cells enhances wound healing. Plast Reconstr Surg. (2018) 141:55e−67e. doi: 10.1097/PRS.0000000000003959
190. Fu J, Chen X, Liu X, Xu D, Yang H, Zeng C, et al. ELABELA ameliorates hypoxic/ischemic-induced bone mesenchymal stem cell apoptosis via alleviation of mitochondrial dysfunction and activation of PI3K/AKT and ERK1/2 pathways. Stem Cell Res Ther. (2020) 11:541. doi: 10.1186/s13287-020-02063-1
191. Ma J, Hu H, Lin M, Chen L, Liu M, Li H, et al. ELABELA alleviates syncytiotrophoblast hypoxia/reoxygenation injury and preeclampsia-like symptoms in mice by reducing apoptosis. Placenta. (2021) 106:30–9. doi: 10.1016/j.placenta.2021.02.002
192. Liu W, Yan J, Pan W, Tang M. Apelin/Elabela-APJ: a novel therapeutic target in the cardiovascular system. Ann Transl Med. (2020) 8:243. doi: 10.21037/atm.2020.02.07
193. Yang P, Read C, Kuc RE, Buonincontri G, Southwood M, Torella R, et al. Elabela/Toddler is an endogenous agonist of the apelin apj receptor in the adult cardiovascular system, and exogenous administration of the peptide compensates for the downregulation of its expression in pulmonary arterial hypertension. Circulation. (2017) 135:1160–73. doi: 10.1161/CIRCULATIONAHA.116.023218
194. Wang C, Xu H, Liu C, Peng Z, Min R, Zhang Z, et al. CaO2/gelatin oxygen slow-releasing microspheres facilitate tissue engineering efficiency for the osteonecrosis of femoral head by enhancing the angiogenesis and survival of grafted bone marrow mesenchymal stem cells. Biomater Sci. (2021) 9:3005–18. doi: 10.1039/D0BM02071K
Keywords: hypoxia, plateau, bone defect, bone regeneration, high altitude
Citation: Chen P, Liu Y, Liu W, Wang Y, Liu Z and Rong M (2022) Impact of High-Altitude Hypoxia on Bone Defect Repair: A Review of Molecular Mechanisms and Therapeutic Implications. Front. Med. 9:842800. doi: 10.3389/fmed.2022.842800
Received: 24 December 2021; Accepted: 15 April 2022;
Published: 10 May 2022.
Edited by:
Xu Yan, Victoria University, AustraliaReviewed by:
Marco Tatullo, University of Bari Medical School, ItalyRui Hua, The University of Texas Health Science Center at San Antonio, United States
Copyright © 2022 Chen, Liu, Liu, Wang, Liu and Rong. This is an open-access article distributed under the terms of the Creative Commons Attribution License (CC BY). The use, distribution or reproduction in other forums is permitted, provided the original author(s) and the copyright owner(s) are credited and that the original publication in this journal is cited, in accordance with accepted academic practice. No use, distribution or reproduction is permitted which does not comply with these terms.
*Correspondence: Mingdeng Rong, cm1kZW5nQHNtdS5lZHUuY24=; Ziyi Liu, ODk0NDIyMzQ1QHFxLmNvbQ==
†These authors share first authorship