- 1Comprehensive Cancer Center Eugène Marquis, Rennes, France
- 2Univ Rennes, Inrae, Inserm, Institut NUMECAN (Nutrition, Métabolismes et Cancer), UMR_A 1341, UMR_S 1241, Rennes, France
Over the last couple of decades, gallium-68 (68Ga) has gained a formidable interest for PET molecular imaging of various conditions, from cancer to infection, through cardiac pathologies or neuropathies. It has gained routine use, with successful radiopharmaceuticals such as somatostatin analogs ([68Ga]Ga-DOTATOC and [68Ga]GaDOTATATE) for neuroendocrine tumors, and PSMA ligands for prostate cancer. It represents a major clinical impact, particularly in the context of theranostics, coupled with their 177Lu-labeled counterparts. Beside those, a bunch of new 68Ga-labeled molecules are in the preclinical and clinical pipelines, with some of them showing great promise for patient care. Increasing clinical demand and regulatory issues have led to the development of automated procedures for the production of 68Ga radiopharmaceuticals. However, the widespread use of these radiopharmaceuticals may rely on simple and efficient radiolabeling methods, undemanding in terms of equipment and infrastructure. To make them technically and economically accessible to the medical community and its patients, it appears mandatory to develop a procedure similar to the well-established kit-based 99mTc chemistry. Already available commercial kits for the production of 68Ga radiopharmaceuticals have demonstrated the feasibility of using such an approach, thus paving the way for more kit-based 68Ga radiopharmaceuticals to be developed. This article discusses the development of 68Ga cold kit radiopharmacy, including technical issues, and regulatory aspects.
Introduction
Today, medicine makes an extensive use of various imaging modalities based on different physical properties, including CT, ultrasonography, MRI, optical imaging or radionuclide-based single-photon emission computed tomography (SPECT), and PET, alone or in combination, to get anatomical and/or functional information in a non-invasive way. In particular, molecular imaging, always making use of more precise contrast agents, enables the visualization, characterization, and measurement of biological processes at molecular and cellular levels in humans and other living systems (1). Among available modalities, PET imaging has the advantage of unlimited depth penetration, very high sensitivity, capability to detect early changes at cellular or even molecular level, and superior resolution and quantification compared to SPECT (2–5). This type of examination allows for functional exploration of biological processes in order to obtain a diagnosis, prognosis, follow-up, or preselection for targeted therapy depending on the drug used (6–8). PET imaging and its hybrid derivatives PET/CT and the more recent PET/MR have had a tremendous impact on patient management, and have now become the new standard for functional imaging, both in oncology and non-oncological setting (9–14).
Positron emission tomography (PET) imaging is currently dominated by 18F-fluorinated radiotracers, and particularly the glucose analog 18F-fluorodeoxyglucose ([18F]-FDG), covering about 90% of PET scans in oncology, neurology, and cardiology. This tracer has found wide applications, taking advantage of increased glucose metabolic rates under several conditions, especially most cancers (2, 15). However, because of its mechanism of action, [18F]-FDG lacks specificity and cannot differentiate between a tumor-associated high metabolic rate and one due to infection or inflammation. Moreover, some tumors are non-FDG-avid and, thus, can hardly be imaged by it (16). This is the case, for instance, of early-stage prostate cancer and some types of gastric cancers (17, 18). This and a better understanding of biological mechanisms underlying metabolic and pathogenic pathways coupled with progress in radiochemistry have stimulated the development of novel PET radiopharmaceuticals (19–25). Initially, PET radiotracers were small endogenous molecules in which one atom was replaced with an equivalent or similar positron-emitting atom (11C, 13N, 18F, and 124I), thus minimally altering their in vivo behavior. With the expansion of medical applications, the quest for new PET nuclides has extended to radiometals, such as 64Cu, 68Ga, and 89Zr, to cite the most prominent ones (25–27).
Gallium-68 belongs to the family of post-transition metals including, among others, indium-111 and thallium-201. It decays to 89% by positron emission and to 11% via electron capture, with average positron energy per disintegration of 740 keV (Eβ + max = 1.899 keV). Its 67.8-min physical half-life is compatible with the pharmacokinetics of most radiopharmaceuticals of low molecular weight, such as small organic molecules, peptides, or even antibody fragments, and oligonucleotides. It allows comfortable use from radiolabeling of PET tracers to acquisition of PET images. Another advantage of its short half-life is limited irradiation of a patient for an injected activity compatible with good PET image quality. In addition, gallium-68 can be conveniently obtained from a germanium-68/gallium-68 (68Ge/68Ga) generator, which can be used within a nuclear medicine department for 1 year thanks to the long half-life of the parent element (t1/2 Ge = 270.8 days). Besides, possible combination with 90Y, 177Lu, or, more recently, 225Ac to form a theranostic pair is another valuable feature (28). All these advantages make gallium-68 a powerful alternative to fluorine-18 (29), and have stirred an ever-increasing interest in 68Ga-based radiopharmacy and the number of patents pertaining to gallium-68 issued over the last decade (30). Spurred by the clinical and commercial success of 68Ga-labeled somatostatin analogs and PSMA ligands, numerous 68Ga-labeled agents have been reported, with some encouraging preclinical and clinical outcomes (31–36). 68Ga has even been described as a potential PET surrogate for 99mTc, the workhorse for SPECT imaging (31, 37). Like the latter, it is expected that the development of kit-based 68Ga radiopharmacy would further expand its clinical usefulness by simplifying and reducing investment costs necessary for automated procedures while still respecting good radiopharmacy practice.
68Ga Production
68Ge/68Ga Generators
One of the main advantages of 68Ga is its cyclotron-independent mode of production. It is conveniently produced “on-demand” with a 68Ge/68Ga generator, in a similar way to the well-known 99Mo/99mTc generator (38). 68Ga radiotracers can, thus, be available worldwide in a flexible way, even in centers far away from cyclotrons or production sites. With the secular equilibrium between the parent radionuclide 68Ge and its daughter 68Ga, the maximum theoretical activity generated is reached 14.1 h after last elution. However, after a time equal to three half-lives of 68Ga, or 3.4 h, nearly 91% of the maximum theoretical activity has already been generated. This allows, if necessary in clinical use, for an elution approximately every 4 h (up to 3 elutions/day), depending on the initial activity of the generator and its age (39).
The production of 68Ga from 68Ge has been described since the 1950s, and the first generator dates from 1960 and relied on a liquid/liquid extraction process (40). This extraction process was quickly supplanted by solid/liquid extraction via an ion exchange resin (41). The stationary phase (matrix) selectively retains Ge4+ ions while facilitating the elution of Ga3+ ions, which have chemical properties sufficiently different to allow several various methods for efficient separation (38). Currently, several generators are commercially available. They all consist of a solid matrix and are eluted with an HCl solution to obtain 68Ga3+. These generators differ from each other by the composition of their matrix, which is either inorganic or organic, and the concentration of HCl used for elution (ranging from 0.01 M for nano-zirconia matrix to 1 M for tin dioxide matrix) (39). As a result, they each have specific characteristics, which represent a major difference with 99Mo/99mTc generators and one of the foremost challenges to develop cold kit formulations. Up to recently developed GMP-generators, obtained 68Ga eluate was not directly amenable to direct radiolabeling because of high volume, thus low 68Ga concentration, high [H+] concentration, 68Ge breakthrough, and presence of other potentially competing metallic cation impurities. Different elution procedures and Post-processing methods include: eluate fractionation, anion exchange, cation exchange, and a combination thereof (39, 42–44).
At this time, two TiO2-based 68Ge/68Ga generators are available for human clinical use, with marketing authorizations from the American Food and Drug Administration (FDA) and the European Medicines Agency (EMA): GalliaPharm® (Eckert & Ziegler AG, Berlin, Germany) and GalliAd® (IRE Elit, Fleurus, Belgium). The 68Ge/68Ga generator (GeGrant®) of ITG (Garching, Germany), with a dodecylgallate-modified SiO2 resin, has recently been granted a Type II Drug Master File from the FDA (Figure 1). Eluates of these generators comply with Pharmacopeia and can be used as is, without the need for post-processing, notably for reconstitution of cold kits.
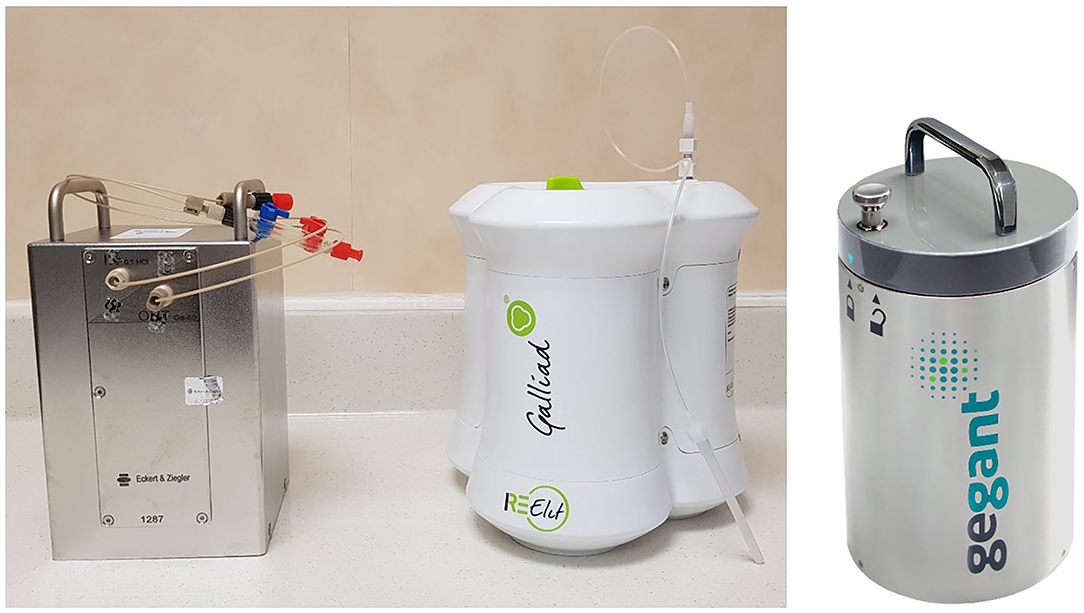
Figure 1. Radiopharmaceutical grade germanium-68 (68Ge)/gallium-68 (68Ga) generators from left to right: Galliapharm®, Galli Ad®, and GeGant®.
Cyclotron Production
Although 68Ge/68Ga-generators represent a convenient option for PET molecular imaging, there remains several limitations. First, the cost of generators can be high, depending on the supplier and/or the grade, and is only amortized if a sufficient amount of exams is performed. Second, because of constant increase in demand in recent years, there was shortage in generators from 2017 to 2018, which posed a threat on the development of clinical use of 68Ga-based imaging agents (45). Other supply issues are anticipated in the future, with increasing demand for 68Ga-imaging, particularly when PSMA imaging agents are approved and become reimbursable (46). Production capacities have been increased and secured. They will, non-etheless, remain limited and may not fulfill the global demand, with a maximum of about 3 preparations of 3 patient doses per day. Alternative production routes have, thus, been investigated (47). In this context, production of 68Ga with existing medical cyclotrons looks particularly attractive. Indeed, the global worldwide network of medical cyclotrons has quickly expanded over the last years.
Production of 68Ga in cyclotrons is usually done via the 68Zn(p, n)68Ga reaction., This reaction has high cross-section in the energy range of 11–14 MeV; thus, it is easily accessible in small medical cyclotrons (< 20 MeV). Either solid or liquid targets can be used, both having their advantages and limitations. For instance, with solid targetry, it is possible to obtain high amounts of 68GaCl3, up to 194 GBq, but with complex and expensive infrastructures (target holder, cooling, target transfer, and target processing) (48, 49). Utilization of a liquid target (dissolution of 68Zn in nitric acid) leads to lower amounts of radioactive material but offers a cost-effective alternative to solid targets, needing less investments, notably for 18F-producing cyclotrons that would like to implement 68Ga production. This looks particularly suited for small on-site production within hospital cyclotron facilities (50, 51). Main disadvantages of cyclotron production are the co-production of other radionuclides (potentially non-removable, since they possess the same chemistry like 66Ga and 67Ga) and the presence of metal impurities that may perturb the radiolabeling reaction of 68GaCl3. This necessitates complex target design and purification procedures, and is particularly true for solid targets (52). Because of growing interest in accelerator-produced 68GaCl3, the latter now has a European Pharmacopeia monograph (monograph 3109) (53). Cyclotron-produced 68Ga, either with solid or liquid targets, has reached the clinic (51, 54). No differences were observed in the quality of studies whether 68Ga was produced from a cyclotron or from a generator.
Gallium Chemistry
Gallium is located in group 13 in the 4th period of the Periodic Table, with the electronic configuration [Ar]3d104s24p1. In aqueous solution, only the +III oxidation state is stable. The Ga3+ cation is a hard Lewis acid (pKa = 2,6), because of its high cation density and short ionic radius (62 pm), with close coordination chemistry to Fe(III), which has a similar ionic radius (65 pm) and same ionic charge (55). It is an electron acceptor. It has a d10 electronic configuration allowing it to accept different numbers of coordination, mostly 6, but 4 or 5 are also possible. It will preferentially coordinate with hard bases (electron donor species, with high electronegativity), that is to say ligands containing nitrogen, oxygen or, to a lesser extent, sulfur (such as carboxylate, phosphonate, hydroxamate, amines, thiolates, and phenolates groups), to form thermodynamically stable complexes (37, 56). However, the ionic form Ga3+ is only stable in a very acidic medium below pH 3. Indeed, at pH above 3, water acts as a weak ligand and gallium associates with hydroxide groups as described below:
Ga3+ + OH− ↔ [Ga(OH)]2+ + OH− ↔ [Ga(OH)2]+ + OH− ↔ [Ga(OH)3] + OH− ↔ [Ga(OH)4]−
The prevalent species formed at pH 3-7, [Ga(OH)3], is insoluble, and gallium is no longer available for a complexation reaction. At basic pH (> 7), gallium forms the gallate ion [Ga(OH)4]− which is soluble. However, gallium remains unavailable for any complexation reaction (57). Hydrolysis and formation of insoluble hydroxides in the preparation of 68Ga radiopharmaceuticals remain a problem that can be circumvented by using weak, stabilizing ligands such as citrate, acetate, oxalate, and HEPES (2-[4-(2-hydroxyethyl)piperazin-1-yl]ethanesulfonic acid) (58). The latter, acting as both a buffer and a weak chelating ligand, has demonstrated superior performance when radiolabeling with 68Ga (59). There are, however, several limitations regarding its use pertaining to its potential toxicity for human use (60). For this, reason, acetate buffers are usually preferred.
The design of most 68Ga-labeled radiotracers is based on the use of bifunctional chelating agents. These compounds are capable of both coordinating the radiometal on one side and covalently conjugating to carrier molecules (i.e., a peptide) by an appropriate functional group on the other side. Bifunctional chelators must meet several criteria for the development of 68Ga-based radiopharmaceuticals:
- Their binding to a vector molecule must not alter their complexation to the metal. Chelation must be rapid and effective.
- Conversely, their binding to the vector molecule must not disturb the chemical characteristics, and therefore, its pharmacodynamic parameters in vivo. The size and charge of chelates can change the affinity of the tracer for its receptor. Lipophilicity may also interfere with the elimination of radiopharmaceutical drugs.
- They must be stable, with respect to hydrolysis, to avoid formation of hydroxides.
- The obtained chelate must be kinetically stable against demetallation, at physiological pH, and in the presence of other cations present in the serum (Ca2+, Zn2+, Mg2+).
- 68Ga complexes must be more stable than 68Ga-transferrin complexes to avoid transchelation because transferrin has two binding sites of metal ions. As already mentioned, gallium has a strong similarity to iron, from a chemical point of view. In vivo, iron is transported by transferrin mainly at the hepatic level. This protein tends to remove gallium from its ligand if the complex is of low affinity, which explains the hepatic binding of gallium. Transferrin, therefore, constitutes a sort of “reference” during tests of gallium-68 chelating agents [mean pGa is 19.7, and log (β) is 20.3 and 19.3 on each of the two sites of binding carried by transferrin] (61).
A strong coordination of chelating agents is always necessary to obtain sufficient stability. Therefore, ligands that form highly stable complexes with Ga3+ ions are hexadentate. They sequester Ga3+ using its maximum coordination number (n = 6). The chemistry of six-coordinate Ga(III) complexes has been comprehensively reviewed (62). Chelation with gallium-68 has been extensively studied, allowing fine tuning of obtained 68Ga imaging agents in terms of charge and lipophilicity, and thus their pharmacokinetics and biodistribution profiles (27, 29, 31, 37, 63–67). There are two main classes of bifunctional chelating agents: macrocyclic and acyclic ligands. In general, acyclic chelating complexes are less inert than macrocycles of comparable stability, but they have higher complexation kinetics than cyclic chelating agents. Overall, a large number of labeling carried out with these acyclic molecules can be done at room temperature rather quickly and with good yield. Examples of chelating agents used with 68Ga are given in Figure 2.
Acyclic polyaminopolycarboxylate ligands, such as ethylenediaminetetraacetic acid (EDTA) and diethylenetriaminepentaacetic acid (DTPA), were among the first chelators to be used with 68Ga for blood cell and renal imaging (68, 69). They have also been used as bifunctional chelators. Unfortunately, most of these complexes showed low stability in vivo, since such anionic complexes tend to undergo acid- or cation-promoted demetallation. More stable complexes were subsequently synthesized. Among the most widely described ligands investigated, one can cite desferrioxamine (DFO) and its derivatives, which form a stable, neutral complex by coordinating Ga(III) through three hydroxamate groups (70). H2DEDPA (6,6'-(ethane-1,2diylbis(azanediyl))bis(methylene)dipicolinic acid) has good characteristics in terms of labeling parameters, quantitatively chelating 68Ga3+within 10 min at RT, with ligand concentrations as low as 10−7 M (71). Its in vivo clearance, however, appears slow (72). Tripodal compounds of the 3,4-hydroxypyridinone family, such as tris(hydroxypyridinone) (aka THP or CP256) and NTP(PrHP)3, are also promising chelators for 68Ga, with quick chelating ability and high in vivo stability (73, 74). They also allow for complexation of 68Ga3+ above pH 5 at ambient temperature and can be conjugated to peptides or other targeting molecules. THP shows remarkably high affinity for Ga3+ ion [log (β) of 35 with Ga3+, pGa of 30]. Maybe its main drawback is its sensitivity for metallic impurities commonly present in 68Ge/68Ga generators or with the material used, such as Al3+, Fe3+, and Ti4+, although it was shown to be less sensible than DOTA or NOTA to other metallic impurities possibly present (Zn2+, Cr3+, Ni2+, and Pb2+) (75). Several 68Ga hydroxypyridinone-based bioconjugates with biomolecules of interest (PSMA, TATE, RGD…) have been reported in the literature (76). The latter two examples ([68Ga]Ga-THP-TATE and [68Ga]Ga-THP-RGD3) led to inferior biodistribution results when compared with their DOTA-counterparts, while [68Ga]Ga-THP-PSMA led to positive outcomes, with tumor imaging characteristics comparable to the current “gold standard” [68Ga]Ga-PSMA-11 (77).
Finally, HBED (N,N'-bis(2-hydroxybenzyl)ethylenediamine-N,N'-diacetic acid) is currently considered the gold standard for acyclic chelating agents. HBED is a related derivative of EDTA, which was first described in the 1960s (78). It has a strong affinity for Ga3+ with a pGa of 28.6. It is characterized by rapid complexation reaction with Ga3+ with very good yields, and its stability in serum is comparable to that of NOTA, which sets it apart from the majority of acyclic chelators. The complexation reaction is rapid even at ambient temperature; it is, therefore, a particularly advantageous ligand for radiolabeling of thermolabile molecules. Among its functionalized derivatives, HBED-CC (N,N'-bis[2-hydroxy-5-(carboxyethyl)benzyl]ethylenediamine-N,N'-diacetic acid) has been particularly studied and used. It presents bifunctionalization with two carboxyl arms in the phenyl rings, which allows its conjugation to multiple vector molecules (79–82). HBED derivatives have a coordination of six and engage four oxygen atoms (the two hydroxyls of the phenyl rings and those of the two acetic functions) and two nitrogen atoms (those of the ethylenediamine function) in the complexation of Ga3+. This complexation causes a modification of the conformation of the ligand that can form three distinct diastereomers each having two enantiomers (67). Heating is necessary to increase the formation of the most thermodynamically favored isomer, since configuration might impact the biological behavior of the radiotracer.
Poly-aza-macrocycles derived from cyclen (1,4,7,10-tetraazacyclododecane) and TACN (1,4,7-triazacyclononane) are currently the most studied ligands. The metal is trapped within a cage-like structure formed by the ligand. It is possible to increase the number of interactions with the radionuclide by adding electron donor groups on the side chains of the chelating agents, which improves the stability of the complex and may eventually provide additional functionalization sites useful for conjugation to the vector molecule. Cyclic chelating agents exhibit good complexation inertia, with little demetallation phenomena seen in vivo; hence their significant use (37). DOTA, a cyclen substituted by four carboxylic arms (1,4,7,10-tetraazacyclododecane-1,4,7,10-tetraacetic acid), is the most common gallium chelator (83). In the case of 68Ga, the maximum coordination number is 6; therefore, only two carboxyl arms are used for complexation; thus, one of the carboxyl groups in DOTA is often transformed and used to allow for conjugation to the vector in the form of a very low complexing activated ester. This decreases the denticity of the chelator, which often causes decrease in stability, especially for metals with a coordination number of 8. Despite an average stability with Ga [log (β) of 21.33 with Ga3+ and pGa of 15.3], close to those of the Ga-transferrin complex, DOTA has great inertia kinetic. However, this involves a slow complexation reaction. Radiolabeling protocols necessitate heating at more than 90°C for several minutes to obtain a yield >90%. This limits its use on heat-resistant molecules. Many DOTA derivatives have, thus, been developed to address these issues (increase the denticity of DOTA and/or allow labeling at room temperature): p-SCN-Bn-DOTA, C-DOTA, DOTAGA, DOTASA, CB-DO2A, TCMC… (37, 84).
Derived from TACN, NOTA (1,4,7-triazacyclononane-1,4,7-triacetic acid) is a hexadentate chelating agent whose N3O3 core provides high thermodynamic stability [log (β) of 30.98 and pGa of 26.4]. This is due to the size of its cycle, which is more adapted to the ionic radius of Ga3+ compared to DOTA. NOTA engages its three carboxylate arms in the complexation of Ga3+ and presents a neutral charge at physiological pH. In this configuration, none of the arms are available for functionalization. If one of its carboxylate arms is mobilized to create a direct bond to a vector, the thermodynamic stability of the complex is compromised, because the optimal coordination of 6 of Ga3+ is no longer possible. In addition, the complex becomes positively charged, which can alter the biological properties of the vector (85). Different functionalized derivatives have been proposed to be conjugated to peptides while preserving the maximum denticity of the NOTA, with, in particular, functionalization on a carbon between a carboxyl and a nitrogen of the ring (i.e., NODAGA). Phosphinic and phosphonic acids functionalized polyazacycloalkanes, such as NOTP, NOPO, and TRAP derivatives, and showed themselves as powerful bifunctional chelators for the preparation of 68Ga-based targeted imaging probes, forming very stable complexes (85–87). They demonstrate significantly improved selectivity for Ga3+ ions, and, hence, are less sensitive to potential metal ion contaminants (88, 89). These triazacyclononane-triphosphinates ligands also have the advantage of chelating 68Ga at very low concentrations and in strongly acidic media. They, thus, would permit labeling directly from an acidic generator eluate, which could ease automation or kit formulation (90).
Recently, hybrid chelators, combining fast complexation kinetics under mild conditions of acyclic ligands with the prolonged complex stability of the macrocyclic ones, have been introduced. H4AAZTA (N,N',N”,N”(6-amino-6-methylperhydro-1,4-diazepine)-tetraacetic acid) demonstrates good qualities, especially regarding its simple conditions of use, and high kinetic inertness and thermodynamic stability of the resulting complex, with a pGa of 22.4 (91, 92). It is, however, not totally stable against serum and competitors. Other more stable chelators based on the 6-amino-diazepine scaffold, have been developed, DATAX chelators (93). They allow for quantitative labeling with 68Ga with favorable kinetics at ambient temperature, and within a large range of pH (4-7). Besides, formed 68Ga-chelates display excellent stability toward transchelation (94). A further advantage of this type of ligands is their suitability to also chelate therapeutic 177Lu radionuclide (95).
Tsoniou et al. have performed an extensive side-by-side comparison with some of the most prominent chelators for 68Ga labeling (67). They demonstrated that NOTA, NOTP, TRAP, HBED, DFO, and THP were all efficiently and quickly labeled at near neutral pH, room temperature, and that with low chelator concentration, THP and DFO are the most effective under these conditions. They are, thus, ideal candidates for instant kit preparation. Among TACN ligands, NOTP appeared as probably the most promising one for labeling under mild conditions (ambient temperature, near-neutral pH). The Ga(NOTP)3− complex, however, appears to be more prone to hydrolysis under basic conditions (86). So far, studies investigating its potential usefulness as a bifunctional chelating agent are scarce. Unfortunately, AAZTA and DATA derivatives were not included in the comparative study by Tsoniou et al. However, a direct comparison between DATA chelators and NOTA demonstrated the potential superiority of the DATA family over NOTA (94). DATA chelators represent, thus, another potential ideal candidate for instant kit preparation. Care should be taken when choosing the chelator for it can have a non-negligible influence on final radiotracer behavior (96). Choice of optimal bifunctional chelator candidate for the preparation of 68Ga imaging agents has to be made based on systematic comparison among different chelators coupled with the same targeting moiety. The best candidate is the one with the best compromise between radiochemistry considerations and pharmacologic parameters, determined by in vitro and subsequently in vivo studies. For instance, Varasteh et al. investigated the influence of four different macrocyclic chelators on a bombesin analog targeting properties (97), while Renard et al. evaluated seven different chelators coupled to a neurotensin receptor 1 antagonist (98). Other examples of such a strategy also include comparison of [68Ga]Ga-THP-TATE with [68Ga]Ga-DOTATATE and [68Ga]Ga-AAZTA5-PSMA-617 with [68Ga]Ga-DOTA-PSMA-617 (99, 100).
Automation
Radiolabeling developed in preclinical research often starts with a manual optimization phase of reaction conditions. However, in order to improve the radiation protection of personnel and facilitate transfer for routine clinical use, automated modules have been developed. They are piloted by software that allows to remotely control the sequence of operations required for labeling. They allow higher reproducibility and robustness, especially in critical stages such as elution of generator and purification. Parameters are checked continuously, and summary data are plotted and stored. It, thus, enables to face the increasing regulatory issues required for hospital-based preparation of PET radiopharmaceuticals (101, 102). Transfer to other institutions is also facilitated with the use of standardized and validated technologies and procedures.
Several semi-automated and automated systems have been developed, either in-house built or commercially available products, combining generator elution and post-processing, 68Ga-radiolabeling, and purification of the final 68Ga radiotracer. It has to be noted that all post-processing approaches (fractionation, anionic and cationic purification) have been adopted in commercially available automated systems. These apparatuses can be classified into two types, depending on the technology used: fixed tubing or single-use disposable cassette (103). Both approaches have their respective pros and cons. Fixed tubing systems were the first to be developed and are still extensively used in preclinical research (104). They are indeed extremely flexible. Modification at will of sequence parameters to optimize labeling is possible. They are also cost-effective. On the other hand, they require stringent cleaning and disinfection procedures to maintain sterility, and cross-contamination cannot be excluded if several radiotracers are prepared using the same module. The cassette system approach presents with the advantage of using sterile single-use cassettes. It, therefore, offers enhanced microbiological safety and eliminates risks of cross-contamination, accordingly better complying with cGMP requirements. Multiple syntheses can easily be achieved, even for different tracers and/or radionuclides, by simply changing the cassette (105). Cassette-based synthesizers, nonetheless, have some downsides. They allow less flexibility than fixed tubing ones. Moreover, using disposable cassettes has a non-negligible cost and generates reliance on cassette manufacturers. New, validated cassette development strongly depends on marketing considerations. Presently, there is a number of sterile GMP cassettes to produce 68Ga-radiotracers for targeted imaging of somatostatin and chemokine receptors or prostate-specific membrane antigen, commercially available from all automated synthesizer manufacturers. Figure 3 presents a typical automated procedure for 68Ga labeling of a DOTA-peptide.
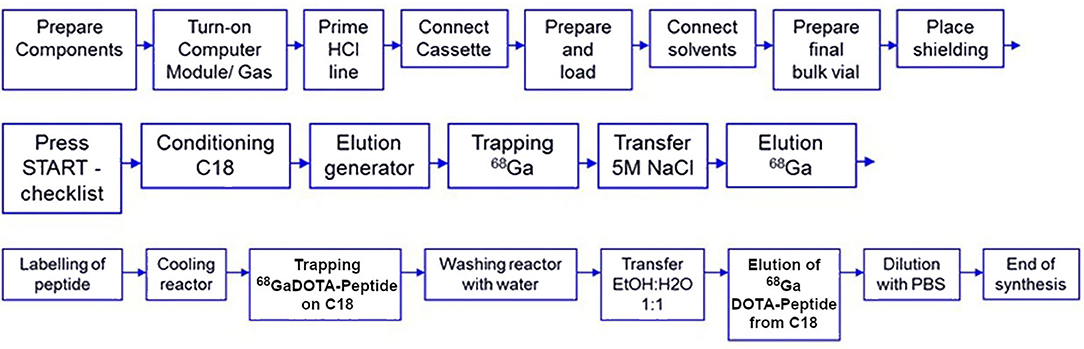
Figure 3. Typical automated procedure for 68Ga labeling of a DOTA peptide [adapted from (106)].
Future developments in automation might be using microfluidic, in a dose-on-demand strategy. This approach would enable reducing the size of modules as well as cost to produce a dose (107, 108). Care should be taken, though, of possible surface effects and radiolysis owing to high radionuclide concentration. Research on that domain is still very preliminary and has not reached clinical use.
Cold Kits
As mentioned above, automated preparation of 68Ga imaging agents generates substantial investments (in hot cells, synthesis modules, and quality control equipment), which may not be easily accessible to small-scale radiopharmacies. This could hamper the widespread use of 68Ga-PET imaging. As a consequence, interest in the development of cold kit formulations, as a simpler and cheaper approach, has increased steeply in recent years (94, 109–111).
Technical Challenges
Generator Eluate
The synthesis of 99mTc radiopharmaceuticals is easily carried out using commercial kits, containing a reducing agent (most often tin chloride), a ligand (intended to form the desired complex or an intermediate complex), and, possibly, other components (buffer, stabilizing agents, cryoprotectant, excipients…). Kits are lyophilized and sealed, and are, therefore, sterile, pyrogen-free, and can be stored for a long time. This approach offers convenience and ease of use, simply adding the required activity in a certain volume of eluate, with or without the need for heating (112). Technetium-99m issued from 99Mo/99mTc is eluted under the form of sodium pertechnetate, with a sterile and pyrogen-free solution of sodium chloride. This solution can be used directly for the preparation of radiotracers.
On the other hand, 68Ga eluate is a strongly acidic solution, and is obtained using HCl in various amounts and various molarities, depending on generator type. It can also come from a cyclotron, with still other characteristics. This is a challenge for the formulation of kits. Indeed, addition of highly varying 68Ga eluates into a fixed buffer amount inevitably leads to pH variations of the final solution. However, apart from use with few chelating agents, gallium chemistry is very pH-sensitive. It is, thus, essential to buffer the solution within an adequate pH range and stabilize 68Ga3+ ion (58). One proposed solution is to prepare 1-vial generator-specific kits, with a fixed amount of buffer in the freeze-dried vial to enable to reach optimal pH in function of HCl volume and molarity required to elute the generator. For instance, to develop a kit-based [68Ga]Ga-DOTATOC preparation, Asti et al. prepared kits with either 180 or 380 μl of 1.5 M sodium formate for use with, respectively, ITG (eluted with 0.05 M HCl) and Eckert & Ziegler (eluted with 0.1 M HCl) generators, to maintain the reaction pH around 3.3 (113). Vats et al. developed three different formulations of a [68Ga]Ga-RM2 kit to be used with ITG, Eckert & Ziegler, and iThemba generators (Faure, South Africa) (114). With this solution not being very practical, another proposed possibility has been to develop 2-vial kit formulations similar, for instance, to [99mTc]Tc-mercaptoacetyltriglycine ([99mTc]-Mertiatide). One vial contains the lyophilized ligand and excipients, and the other the buffer solution. This way, it is possible to adjust the necessary amount of buffer to reach suitable pH for the labeling to proceed. This can be exemplified by [68Ga]Ga-DOTATOC and [68Ga]Ga-DOTATATE kit formulations (106). The kit for the preparation of [68Ga]Ga-HBED-CC-PSMA-11, developed by ANMI (now part of Telix Pharmaceuticals, Melbourne, Australia), is also provided with a third sterile vacuumed vial for initial elution before addition of the buffer-dissolved precursor (Figure 4).
Most of the kits developed up to now have been validated with one generator model, usually dependent upon geographic and/or financial availability. There is currently only one example of a cold kit used with an accelerator-produced 68GaCl3 eluate (115). When using different generators, besides tweaking the formulation, it might also be required to adapt the radiolabeling protocol. For instance, [68Ga]Ga-DOTATOC (Somakit TOC® Novartis) was initially developed for use with a Galliapharm® (Eckert & Ziegler, Basel, Switzerland) generator, at a time when the latter was the only authorized generator for human use. It was, thus, designed to be reconstituted with 5 ml of 68Ga-eluate. To be used with the newly introduced GalliAd® (IRE Elit) generator and its 1.1-ml eluate, a different procedure had to be validated by, notably, dilution with sterile water (Figure 5). When preparing [68Ga]Ga-DOTAGA-TOC and [68Ga]Ga-DOTAGA-TATE using an Eckert & Ziegler generator, Satpati et al. passed the generator eluate through a Strata™ X-C cation exchange column, which was then eluted with a 500-μl acetone/HCl mixture (97.6%/0.02 M) before the addition of the purified eluate to the kit vial (117). Using an ITG generator, the eluate was directly added to the kit vial. Tuning the molarity of the buffer is also a way to enhance the robustness of buffering toward HCl. In another study by Satpati et al., they reported that increasing sodium acetate buffer from 0.5 to 1.5 M allowed to yield suitable pH conditions either with an Eckert & Ziegler generator or with an ITG generator when preparing [68Ga]Ga-DKFZ-PSMA-11 (118). Using even a very small amount (250 μL) of 2-M acetate buffer, on the other hand, led to very high pH of 5.5, even for the most acidic E&Z generator.
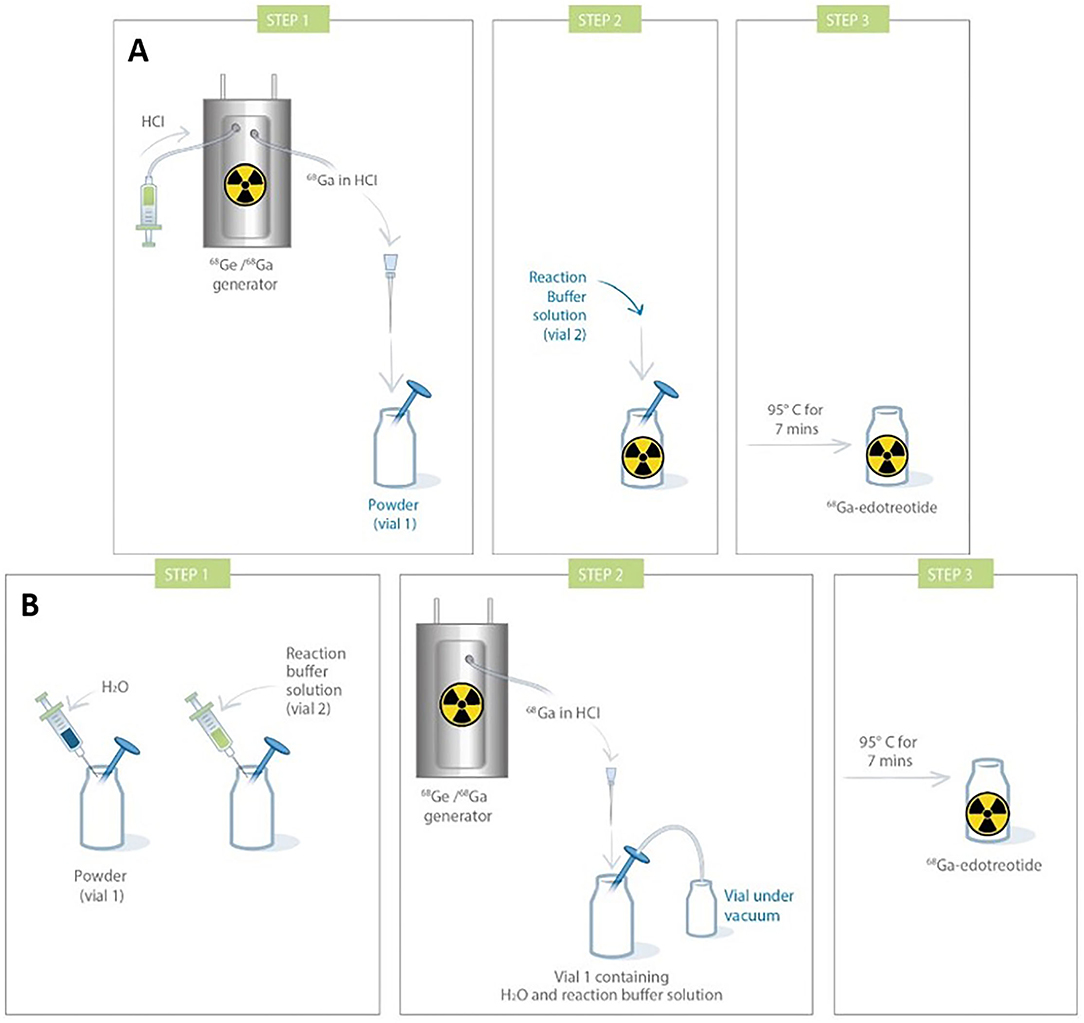
Figure 5. Comparison of procedures for Somakit TOC® labeling with 2 different commercial generators [(A). Galliapharm (Eckert & Ziegler) GalliAd (IRE Elit); (B). GalliAd® and IRE Elit) (116).
Chelator
Use of robust, gallium-specific, bifunctional chelators is another step toward kit-based 68Ga-radiopharmacy. Promising ligands, like macrocyclic NOTA phosphinic derivatives, acyclic HBED-CC and THP, and hybrid DATA chelators, allow for fast and easy complexation with 68Ga under mild conditions, which is amenable to instant kit-type procedure. Several kits using these chelators have been reported in the literature, the most notable being HBED-CC-PSMA-11 used in two commercially available cold kits for prostate cancer imaging (Illumet™/Illuccix®, Telix Pharmaceuticals, and IsoPROTrace-11®, Isotopia, Petah Tikva, Israel) (119). The main difference between both kits is that the first kit consists of three vials, while the latter contains only one. PSMA binding motif lysine-urea-glutamate (KuE) has been attached to a THP chelator in yet another investigational kit, which was recently the object of a phase 2 trial (120). The same motif was also successfully grafted on DATA5M and AAZTA5 chelators, but no kit formulation has been reported to date, although it is amenable to do (121).
Even though these chelators revealed themselves as successful in complexation of 68Ga in a fast and stable manner, they, with the possible exception of AAZTA/DATA chelators, unfortunately do not allow for complexation of other radiometals with higher coordination number, such as 177Lu, 90Y, and 225Ac. Thus, from a theranostics perspective, DOTA and its derivatives still remain the chelator of choice, even for 68Ga. Besides,68Ga-labeled DOTA-peptides generally demonstrate very good pharmacological properties. Kit formulation with DOTA/DOTAGA precursors is, therefore, still an active research area (113, 114, 117, 122).
Precursor
To be sure that the reaction is complete and no post-labeling purification step is required, kits are generally prepared with higher ligand amount. For instance, 40 μG of a DOTATOC precursor is present in lyophilized kit formulation, while only 20–25 μg is necessary when [68Ga]Ga-DOTATOC is prepared manually or with an automated synthesizer (123). Likewise, 10 μg of a PSMA-11 precursor for automated process is used vs. 25 μg for an Illumet™ kit. This has to be optimized finely to prevent saturation of targeted receptors with unlabeled biomolecule. On the other hand, this, plus shorter production time and higher radiolabeling yield with the kit than with automated module synthesis, might allow for multiple patient preparations, although kits are usually recommended as single-dose preparations (118, 124, 125). Regarding outcomes of both processes, in terms of product characteristics, quality control gave comparable results (Figure 6A), and both methods are reliable and comply with Good Manufacturing Practices and European Pharmacopeia specifications (113, 124, 126, 127). In terms of clinical applications, a direct comparison on patients with prostate cancer found no significant differences in PET/CT image quality (Figures 6B,C) (126).
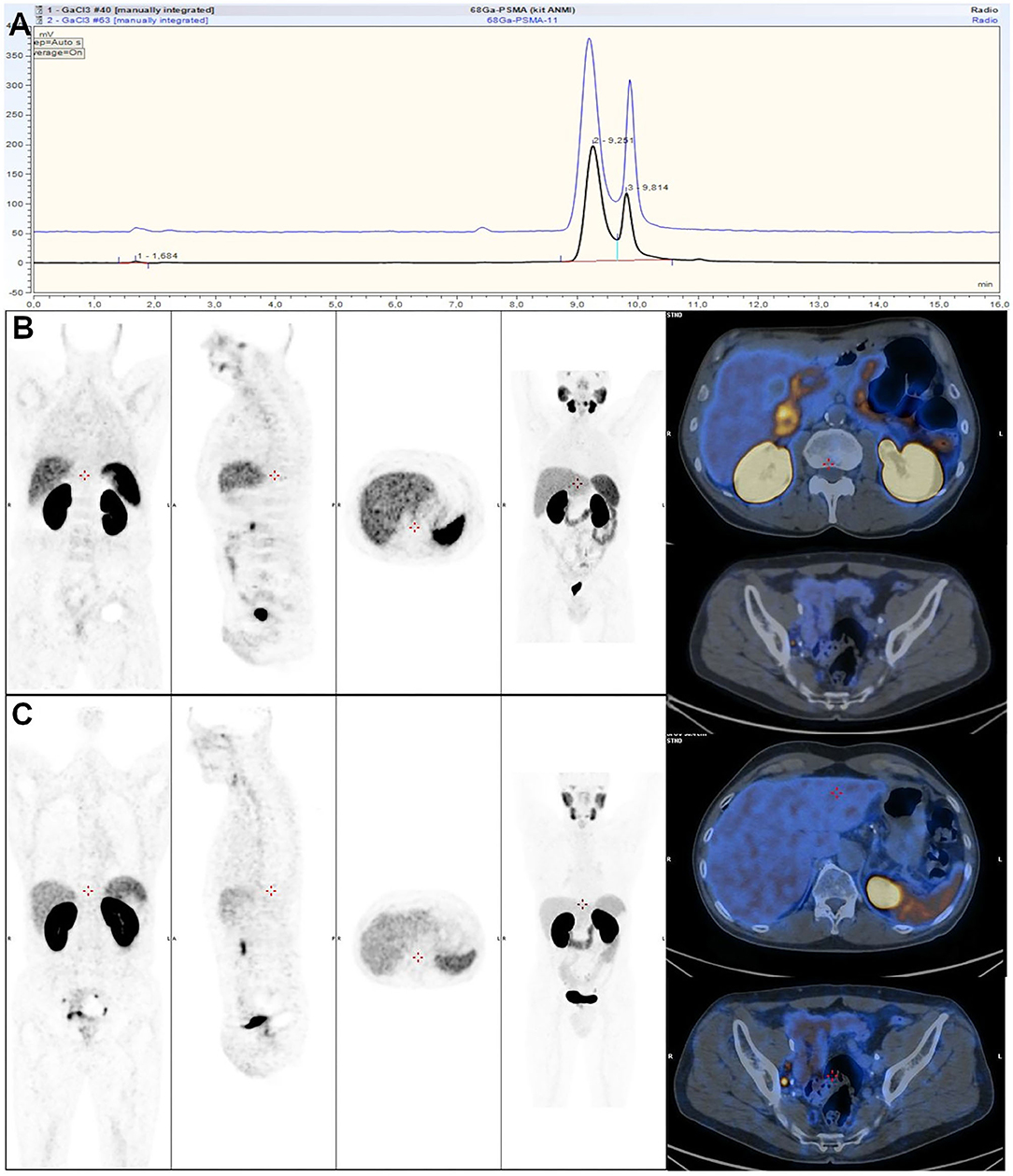
Figure 6. (A) Comparison of HPLC profiles of automated (blue) and cold kit-based (black) labeling of [68Ga]Ga-HBED-CC-PSMA-11. (B,C) Comparison of 2 68Ga-PSMA-11 PET/CT acquisitions rated as good quality using 68Ga-PSMA-11 synthetized with sterile cold kit (B) and 68Ga-PSMA-11 synthetized with automated module (C). (B,C) from (126).
Kit-Based vs. Module Based Radiolabeling
Several studies discussed the respective pros and cons of cold kit-based preparation and module-based automated synthesis (126–128). To summarize, main advantages of the kit-based process are cost, shorter production time, simplicity of use, no purification needed, no EtOH content, and lower final volume. Main drawbacks are radiation exposure, higher amount of precursor usually needed, strong dependence on generator quality since there is no purification step, and few kits currently established and even fewer commercially available. To detail more precisely the cost efficiency of 68Ga cold kit labeling, besides savings on expensive synthesizer and its maintenance, and, according to local regulation, even the need for a shielded hot cell, cost per synthesis is significantly decreased. For instance, Kleynhans et al. calculated that one [68Ga]Ga-HBED-CC-PSMA-11 synthesis with a module and commercially available cassette and precursor costed 274 €, and that the cold-kit synthesis amounted for only 10 € with an in-house made kit (127). It should be noted that a commercial kit would be more expensive. In a study on [68Ga]Ga-RM2 synthesis, consumable costs for a synthesis was reported to be 282.1 vs. 65.7 € for a module and a kit, respectively, whereas the shorter production time and higher radiolabeling yield with the kit would allow for the injection of at least two additional patients (125). This gain in production time may also improve patient workflow, improving, again, cost efficiency.
Concerning radiation protection, there are only few direct comparisons between kit-based and module-based preparations reported in the literature. Frindel et al. compared [68Ga]Ga-DOTATOC kit preparation with automated [68Ga]Ga-DOTANOC labeling (129). Based on detector probes fixed on the first phalanx of each middle finger, they extrapolated extremity dose measurements for a 1.85-GBq generator at calibration. They reported 70 and 132 μSv for the automated labeling (left and right hand) and 179 and 152 μSv for the kit-based preparation. In the study by Kleynhans et al. on [68Ga]Ga-HBED-CC-PSMA-11 synthesis, whole-body exposure was found to be significantly lower for the automated synthesis with 2.05 ± 0.99 μSv vs. 14.32 ± 5.3 μSv per synthesis (127). However, automated synthesis was performed on a hot cell with 50-mm lead shielding, while the kit preparation was realized in a 3-mm lead-shielded class II biosafety cabinet, with a 10-mm lead shielded tabletop shield. When performing kit radiolabeling on a 50-mm lead hot cell, whole-body exposure was brought down to 2 ± 0.5 μSv per synthesis. Extremity dose was calculated to be 1.5 ± 0.4 mSv per kit synthesis. A major advantage of automated synthesis on radiation exposure was lower variation. For the kit labeling, radiation exposure was highly varying, depending on operator experience. Moreover, this can lead to radiation protection concerns in centers with high patient workflow. To circumvent this problem, several teams have investigated the possibility of partly or fully automating kit preparation. For instance, some teams eluted their 68Ge/68Ga generator with an automated module before reconstituting the kit vial (130). Revy et al. investigated both an automated and a semi-automated method for the labeling of kit-based DOTATOC formulation with 68Ga (131). Automated method led to longer preparation time, and, above all, possible product loss. Indeed, since the synthesizer they used, like most commercial synthesizers, does not possess a cooling block, transfer to another vial after the heating step was necessary to avoid overheating. The semi-automated process was developed as an alternative to product transfer with manual removal of the vial from the heating block. Semi-automated vs. manual kit labeling reduced significantly radiation exposure with a whole-body dose of 0.35 ± 0.19 vs. 0.98 ± 0.96 μSv/GBq and an extremity dose below the limit of quantification vs. 0.567 and 0.467 mSv/GBq (right and left hands). These methods are easily transposable to other cold kit preparations, especially those not requiring heating. Kit-based preparation of 68Ga-MAA (macroaggregated albumin) was conveniently automated using small Modular Lab EAZY (Eckert & Ziegler) (132). In front of this interest in the automation of kit radiolabeling, the industry has developed dedicated modules, like KitLab (Eckert & Ziegler) and MorGaNA (Tema Sinergie, Faenza, Italy) (133). These have the advantage of small footprint but have less flexibility than common automated modules.
Regulatory Aspects
There are several articles that review the regulatory framework for radiopharmaceuticals in the US and Europe (101, 134–136). Radiopharmaceuticals are defined as medicinal products. In Europe, medicinal products are regulated in Directive 2001/83/EC (137), which also extends to cold kits, radionuclide generators, and radionuclide precursors (138). This implies that radiopharmaceuticals, and kits, need a marketing authorization, and a production process according to Good Manufacturing Practices (GMP), whose guidelines are specified in a separate Directive 2003/94/EC (139). Specific rules apply according to the status of the radiotracer. Investigational medicinal products and radiopharmaceuticals prepared “in-house” for local use (magistral/officinal preparation or compounding) are exempted from Directive 2001/83/EC (101, 140). Taking into account the particularities of radiopharmaceuticals, the EANM and its Radiopharmacy Committee have released specific guidelines, dubbed current Good Radiopharmacy Practice (cGRPP), for the production of radiopharmaceuticals in small-scale radiopharmacies, which do not cover commercial radiopharmaceuticals (141). For radiopharmaceuticals prepared from a licensed generator and a licensed kit, quality control should be realized according to the procedures described in the Summary of Product Characteristics (SmPC) for generator eluates and kit-based radiopharmaceuticals. When preparing from unlicensed components, procedures are more stringent to ensure maximum safety. When available, use of the appropriate European Pharmacopeia (Ph. Eur.) monograph or similar guidelines and documents published by the FDA is required. Implementation of a new radiopharmaceutical into pharmacopeias follows an extended procedure. For instance, [68Ga]Ga-PSMA-11 was granted a Ph. Eur. monograph in 2020 (monograph 3044) despite its extensive use for several years (53). Consequently, the majority of 68Ga-based imaging agents is not yet represented with their own monographs in the Pharmacopeias. In this case, general Ph. Eur. monograph “Radiopharmaceutical preparations” (monograph 0125) or closely related specific monographs (i.e., [68Ga]Ga-DOTATOC monograph, monograph 2482) can be used. Additionally, local authorities could demand more detailed quality control, even for licensed radiopharmaceuticals. The quality of the final radiopharmaceutical must fulfill all specifications given by the relevant legislation or Pharmacopeia no matter the synthesis route (manual, automated, or kit-based) (142).
A kit (or radiolabeling kit) is defined as “Any preparation to be reconstituted or combined with radionuclides in the final radiopharmaceutical, usually prior to its administration” (141). Cold kit-based radiolabeling is considered a closed procedure, consisting of preparation of a sterile radiopharmaceutical through the addition of a sterile eluate to a sterilized close vial containing a set of sterile, lyophilized ingredients via a system closed to the atmosphere. The final product is, thus, a sterile and pyrogen-free solution suitable for intravenous injection. It is of utmost importance to rigorously follow the instructions given by the manufacturer, especially regarding the maximum activity and volume of 68Ga eluate that is transferred to a kit vial (116). The European Pharmacopeia states that “the marketing authorization holder of a licensed kit is responsible to ensure compliance of the kit with the requirements of its marketing authorization, while the final user carries the responsibility for the quality of the preparation and the handling. If the given instructions are not strictly followed or if one or more components used for the reconstitution do not have marketing authorization, it is the responsibility of the final user to demonstrate that the quality of the final preparation is suitable for the intended use” (143). On its side, the FDA considers “cold kits to be finished drug products. Therefore, preparation of a radiopharmaceutical from the components of a cold kit according to FDA approved labeling is not compounding. However, if an ingredient is added, or if the cold kit is otherwise manipulated in a manner not considered a minor deviation, it would be considered compounding,” which could only be done by or under the supervision of an authorized radiopharmacist (144).
68Ga Cold Kits and Their Applications
An overview of the kits reported in the literature is given in Table 1.
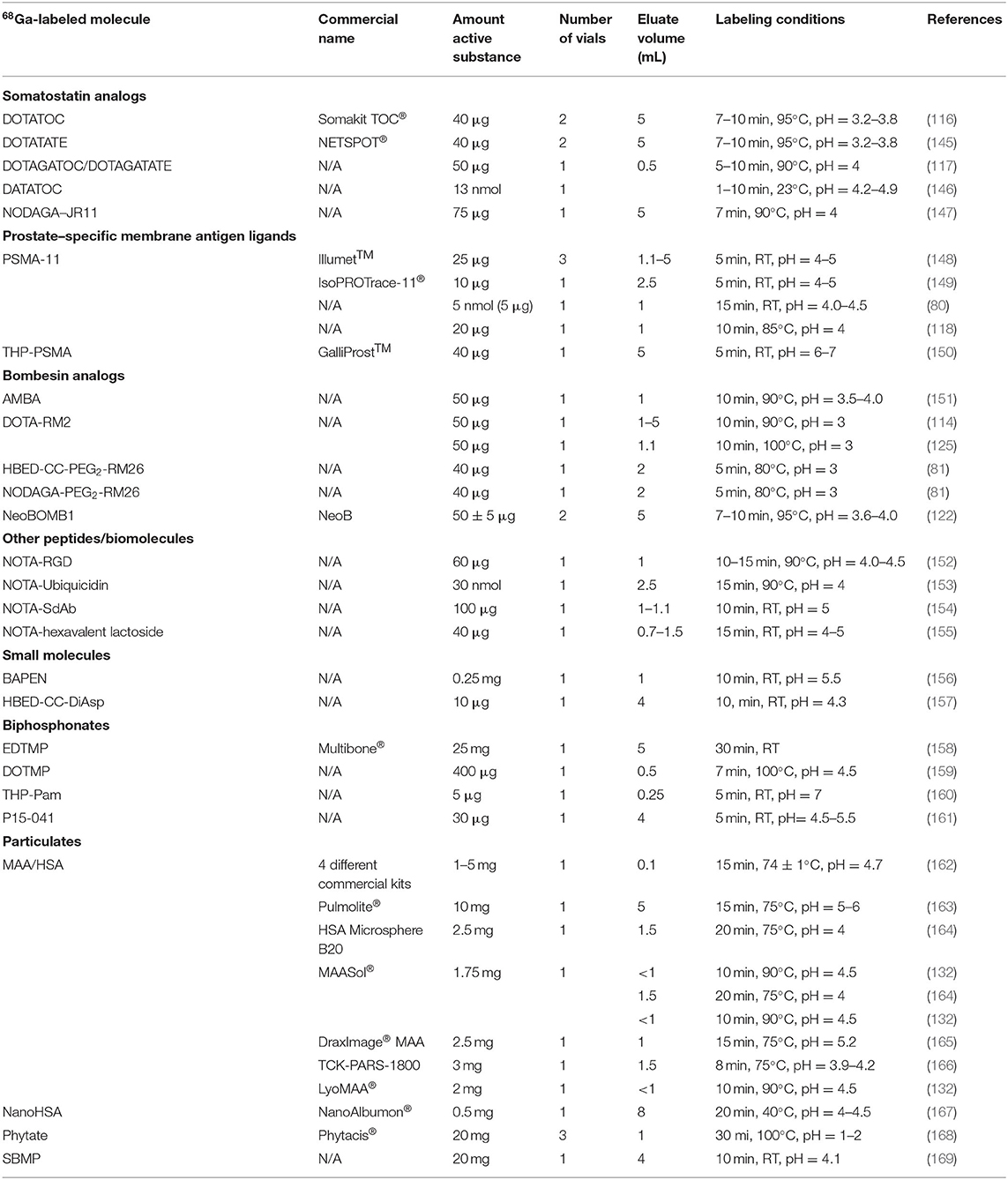
Table 1. Overviewof cold kits reported in the literature for the preparation of gallium-68 (68Ga) imaging agents.
Neuroendocrine Tumor Imaging
Somatostatin receptors (SSTRs) are widely expressed in the whole body, but their expression is significantly enhanced in many solid tumors, especially gastro-entero-pancreatic neuroendocrine tumors (GEP-NETs). Thus, radiolabeled somatostatin analogs have been developed to visualize the distribution of receptor overexpression in tumors and/or from a therapeutic perspective (84). Among them, [68Ga]Ga-DOTATOC ([68Ga-DOTA-Tyr3]octreotide), [68Ga]Ga-DOTATATE ([68Ga-DOTATyr3]octreotate), and [68Ga]Ga-DOTANOC ([68Ga-DOTA1NaI3]octreotide) have shown themselves particularly useful for neuroendocrine tumor imaging, leading to the approval of the first two for GEP-NET imaging (170). Evaluation of a cold kit-based preparation of 68Ga-labeled somatostatin analogs was first described by Mukherjee et al. (171). They consisted of single vial kits containing 50 μg of a lyophilized peptide plus sodium acetate in order to achieve a pH in the range of 3.5–4 upon addition of the 68Ga eluate (1 mL of 0.1 M HCl). A small study on 10 patients with a freeze-dried kit-prepared [68Ga]Ga-DOTATOC allowed to identify sites of primary and metastatic diseases with good accuracy and specificity (172). Other teams around the world also successfully developed kit formulations for DOTA-somatostatin analogs (113, 173). Positive clinical results with these radiotracers eventually led to the approval of [68Ga]Ga-DOTATATE (NETSPOT™) in the United States and [68Ga]Ga-DOTATOC (Somakit TOC®) in Europe (174). First clinical investigations with this [68Ga]Ga-DOTATOC kit successfully validated its safety and clinical utility (106).
Radiolabeling with DOTA peptides requires heating at over 90°C, and might not represent the best candidate for kit-based radiopharmacy. To achieve rapid labeling under mild conditions, somatostatin analogs based on other more “user-friendly” chelators have been proposed. THP-Tyr3-octreotate (THP-TATE) has been labeled with 68Ga in <2 min at room temperature, in ≥ 95% radiochemical yield (99). No freeze-dried kit formulation has, however, been reported to date. One of the reasons may be the higher retention in non-target organs and lower tumor-to-liver ratio than for [68Ga]Ga-DOTATATE, which limits its interest in clinic. [68Ga]Ga-DATATOC, based on a DATA chelator, has been radiolabeled with 68Ga in 1–10 min, depending on the post-processing method used, at ambient temperature using a single vial kit containing 13 nm of a peptide derivative (146). In a first-in-human study, it demonstrated higher tumor-to-liver contrast in a NET-patient compared to [68Ga]Ga-DOTATOC, while in a subsequent study with 50 patients, it displayed comparable results with [68Ga]Ga-DOTANOC (175, 176). It has been hypothesized that somatostatin, antagonists, and other peptides might be more useful than agonists. Actually, after binding to its receptor, an agonist analog is internalized into a cell as a ligand-receptor complex. This internalization allows it to accumulate into the cell. This does only slightly occur for antagonists, and they do not activate the receptor. Antagonists do, however, accumulate more on the target, as a consequence of a greater number of target binding sites for antagonists and a more slowly dissociation kinetics than for agonists, and probably due to a possible ligand rebinding mechanism (36, 84, 177). In that context, 68Ga-OPS202 ([68Ga]Ga-NODAGA-JR11) has attracted particular attention and demonstrated superiority over [68Ga]Ga-DOTATOC (178). Satpati et al. recently reported the development of a cold kit for 68Ga-OPS202, formulated with either HEPES or sodium acetate buffer (147). To our knowledge, 68Ga-OPS202 prepared this way has not yet been evaluated in humans.
Prostate Cancer Imaging
Prostate-specific membrane antigen (PSMA), a type II transmembrane protein on the surface of cancer cells, is considered to be the most interesting antigen in prostate cancer, since it is overexpressed in high-grade tumors, metastases, and hormone-resistant tumors with low concomitant expression (100 to 1,000 times less) in normal tissues (179). PSMA is, therefore, an ideal target for molecular imaging of prostate cancer, especially for the development of small radiolabeled molecules, having fast plasma clearance and generating little background noise (180). Identification of the binding site of PSMA substrates promoted the development of small molecule ligands or inhibitors of PSMA. PSMA inhibitors can be grouped into three families: phosphorus inhibitors, sulfur inhibitors, and urea-based inhibitors. The latter have great affinity and good specificity for PSMA as well as rapid internalization into tumor cells (181). The small molecule Lys-Urea-Glu (Lys-NH-CO-NH-Glu) composed of two amino acids, lysine and glutamate, united by a urea unit is one of them. This pharmacophore has been conjugated with several chelators for labeling with 68Ga (182). Among them, HBED-CC linked to the Lys-Urea-Glu motif through a 6-aminohexanoic (Ahx) spacer afforded particularly positive results (183). Eder et al. were particularly interested in the influence that the proportion of isomers could have on the characteristics of the binding of the radiotracer to its target (184). They showed that specific binding to PSMA and internalization of the radiotracer were comparable, whatever the proportion of isomers present. Successful clinical investigations eventually led to FDA approval for the UCLA Biomedical Cyclotron Facility (Los Angeles, CA, United States) and the UCSF Radiopharmaceutical Facility (San Francisco, CA, United States) (119). This imaging agent, indeed, has had a significant impact on prostate cancer management (185).
Preparation of a freeze-dried cold kit formulation for the preparation of [68Ga]Ga-HBED-CC-PSMA-11 was first reported by Ebenhan et al. in 2015 (80). The radiotracer was conveniently prepared in 15 min, at room temperature, from a single-vial kit containing 5 μg of HBED-CC-PSMA-11 precursor. It passed all quality control criteria and, in a 15-patient study, was able to detect primary prostate cancer as well as metastatic lesions. Other kit formulations containing HBED-CC-PSMA-11 have been subsequently set up (118, 148). Two commercial kits are now available for investigational studies, as mentioned above, allowing for fast labeling of 68Ga in 5 min at room temperature (148, 149). Both kit formulations demonstrated their clinical applicability and are compatible with all commercial 68Ge/68Ga generators as well as cyclotron production.
Another PSMA derivative, based on the promising THP chelator [tris(hydroxypyridinone)], has also attracted attention. A single-vial kit formulation has been developed by (Theragnostics, Bracknell, UK) (GalliProst™). It contains 40 μg of THP-Glu-urea-Lys(Ahx), which is a higher amount than in PSMA-11 kits, and complete labeling occurs in 5 min at ambient temperature (150). Positive clinical outcomes, i.e., good detection rates in patients with biochemical recurrence coupled with ease of use, led to an ongoing phase 2 clinical trial (120, 186–188). It has to be noted, however, that detection rates are lower than with other PSMA imaging agents.
Gastrin-releasing peptide receptors (GRPRs) are another potential target for prostate cancer imaging and therapy. Various 68Ga-labeled peptides targeting these receptors have been reported in the literature, among which are bombesin derivatives, either agonists (i.e., AMBA) or antagonists (i.e., RM2) (189–191). The GRPR antagonist [68Ga]Ga-RM2 (RM2=DOTA-4-amino-1-carboxymethylpiperidine-D-Phe-Gln-Trp-Ala-Val-Gly-His-Sta-Leu-NH2) has been particularly attractive for prostate cancer imaging. In small cohorts of patients, it emerged as a possible alternative to [68Ga]Ga-PSMA-11 imaging, thanks to its different biological processes (192). Its role is, non-etheless, still a matter of debate (193). In view of the potential interest in this radiotracer, several kit formulations have been reported in the literature. Vats et al. developed three single-vial formulations, each adapted to one single 68Ge/68Ga generator type (114). In vitro studies demonstrated that [68Ga]Ga-RM2 prepared this way was suitable for GRPR imaging. Soon after, Chastel et al. developed a similar kit formulation (50 μg of peptide precursor, same reaction conditions) usable with a GalliAd® generator (125). Besides demonstrating high uptake in GRP-R-expressing PC-3 cells, they established the importance of excipients (scavenger, bulking agent) in the formulation of freeze-dried kits, since composition without trehalose did not meet criteria for lyophilization.
Lung Perfusion Imaging
Albumin particles were the first reported example of cold kit labeling with 68Ga, as far as in the end of the 1980s, for imaging pulmonary perfusion with higher resolution and sensitivity than conventional 99mTc SPECT imaging. Experiments were conducted using commercial [99mTc]Tc-MAA kits (162). Since then, different types of albumin particles have been labeled, either macroaggregated albumin or human serum albumin (HSA), still with various commercial 99mTc-kits, and employing a similar procedure to prepare the kits for the labeling with 68Ga. The kits were suspended in 5-ml sterile saline, vigorously shaken, then centrifugated to separate particles from stannous chloride and other components, such as polysorbate or free albumin. After centrifugation, the supernatant was discarded and albumin particles were resuspended in a small volume of sterile water or saline and used for labeling with 68Ga (164, 170). Some authors also relyophilized the kits before use (165). It was, however, demonstrated that removal of stannous chloride prior to labeling with 68Ga was not necessary. Indeed, absence or presence of SnCl2 had no impact on labeling efficiency (132). Unfortunately, despite initial promises and possible lower-dose delivery to critical organs, 68Ga-MAA or 68Ga-HSA has never broken through (166).
To avoid the use of human blood derivatives, starch-based microparticles (SBMPs) have been proposed as a surrogate to albumin particles for lung imaging and labeled with 99mTc (194). Verger et al. reported the labeling of these SBMPs with 68Ga, but only investigated their potential use as a pre-therapeutic tool for radioembolization of liver cancer (169). In a similar manner, phytate particles, using a commercial Phytacis® kit for 99mTc, have been proposed as a possible 68Ga perfusion imaging agent (168).
Other Applications
As mentioned above, GRPR imaging has found interest in prostate cancer diagnostic and staging. GRP receptors are, however, expressed in several other cancers, which could benefit from the use of GRPR-targeting 68Ga radiotracers (195). Gastrin-releasing peptide receptors are notably overexpressed in breast and lung cancers. Besides [68Ga]Ga-RM2, several other cold kit formulations have been reported with GRPR-addressed peptides. Pandey et al. described a kit formulation with GRPR agonist [68Ga]Ga-AMBA ([68Ga]Ga-DO3A-CH2CO-G-[4-aminobenzoyl]-QWAVGHLM-NH2), while Satpati et al. reported the labeling of the antagonist RM26 (D-Phe-Gln-Trp-Ala-Val-Gly-His-Sta-Leu-NH2), conjugated either with a NODAGA or an HBED-CC chelator (81, 151). Another promising bombesin derivative is the antagonist NeoBomb1. It is currently the object of a clinical study on patients with oligometastatic gastrointestinal stromal tumors, and is prepared using a kit procedure (196). This cold kit, designed for use with a Galliapharm® generator, enables fast radiolabeling of peptides in <10 min with a 2-vial formulation (122).
Several other kit-based 68Ga radiosyntheses have been reported with potentially interesting peptides. Integrin receptor targeting [68Ga]Ga-NOTA-RGD, for angiogenesis imaging, has been described by Ebenhan et al. using a single-vial kit formulation (152). Ubiquicidin (UBI 29-41 = TGRAKRRMQYNRR) is an antimicrobial peptide designed as an infection-specific imaging agent when radiolabeled. It was first labeled with 99mTc (197). A 68Ga-PET surrogate has subsequently been developed and investigated using a kit-based preparation (153). This small study on 10 patients gave mixed results, as 4 patients gave false negative results possibly because of antibiotic treatment (2 patients) or because of very low microbial population. Studies with a larger number of patients are necessary to assess its usefulness.
Biphosphonates are another important class of imaging agents. They are extensively used for bone imaging, in particular for visualization of skeletal metastases. Several bisphosphonate derivatives have been labeled with 68Ga, for which kit formulations have been reported. EDTMP (ethylenediamine tetramethylene phosphonic acid) has been radiolabeled with 68Ga using a commercial Multibone® kit, initially designed for use with 99mTc, but its utility remains uncertain (158). 68Ga-labeled BPAMD (4-{[bis-(phosphonomethyl))carbamoyl]methyl}-7,10-bis(carboxy methyl)-1,4,7,10-tetraazacyclododec-1-yl)acetic acid) has shown favorable preclinical and clinical properties. The radiotracer, prepared with a kit-based procedure, gave satisfactory results in terms of ease of use and clinical outcome in a small cohort of patients (198). [68Ga]Ga-THP-Pam, based on pamidronic acid conjugated to a THP chelator, was easily obtained under mild conditions (5 min, RT, and pH 7) and demonstrated high in vivo affinity for bone tissue, comparable to [18F]NaF (160). Latest example to date is [68Ga]Ga-P15-041, an HBED-CC-derived bisphosphonate (161).
Future Developments
Several other 68Ga imaging agents demonstrated promising preclinical and clinical outcomes. They are currently prepared manually or through automated procedures, which limits their dissemination. The set-up of cold kit formulations for these radiotracers would, thus, be of great benefit for the medical community. Among the radiotracers of interest, one can cite the peptides neurotensin and exendin-4, for which receptors are overexpressed in several tumor types (199–202). These two peptide derivatives could be of interest particularly in pancreatic cancers. Preliminary clinical results demonstrated the safety and tolerance of a 68Ga-labeled agonist neurotensin peptide ([68Ga]Ga-NT-20.3) conjugated to a DOTA chelator (203). Antagonist derivatives have also been investigated with various common 68Ga chelators (98). It was shown that the DOTAGA chelator gave the best results in vitro and in vivo, and that the THP chelator, a priori the best suited chelator among the ones investigated for kit formulation thanks to its radiolabeling characteristics, led to poor tumor uptake. Several chelators have been conjugated with exendin-4 (DOTA, NODAGA, DFO, and HBED-CC), and some of them are suitable for cold kit preparation (130, 204, 205).
Nevertheless, radiotracers with highest promises are probably the CXCR4 and FAP inhibitor ligands, targeting a tumor microenvironment. Chemokine receptor 4 (CXCR4) is overexpressed in numerous tumor types, and plays a critical role in tumor growth and invasiveness, as well as metastasis (206). The 68Ga-labeled CXCR4 antagonist ([68Ga]-Pentixafor), prepared automatically through a commercial single-use cassette (207), has revealed itself as a useful clinical tool in hemotologic diseases and in solid tumors, as well as under benign conditions (208–210). Fibrobast activation proteins (FAPs), overexpressed in a variety of tumors, are involved in several tumor-promoting activities and represent a prospective theranostic target. A family of inhibitors of this protein based on a quinolone scaffold has been developed and labeled with several diagnostic and therapeutic radionuclides, such as 68Ga (211, 212). Among the FAPI derivatives developed, [68Ga]Ga-FAPI-4 and [68Ga]Ga-FAPI-46, with a DOTA chelator, led to impressive results (213–215). New derivatives suitable for cold kit radiolabeling were recently described, one with a NOTA chelator (FAPI-74) (216), and another with the DATA5M chelator (217).
Conclusion
Radiolabeling of clinically useful 68Ga-PET imaging agents with ready-to-use lyophilized cold kits may contribute to their worldwide dissemination, in particular in small or low-budget centers not equipped with an elaborate and expensive set-up, enabling for earlier and better diagnostics and treatment response assessment in the context of personalized medicine. Kit-based synthesis has proven to be “user-friendly,” trustworthy, robust, and cost-effective. New chelators such as THP, DATA, and NOTA derivatives, enabling “instant kit” procedures, are particularly appealing in that regard. The repertoire of applications is steadily increasing, following clinical demand. Cold kit formulations of most promising new radiotracers such as CXCR4 and FAPI ligands are eagerly awaited.
In large centers with high patient throughput, however, automated modules may most probably remain the favored production mode for 68Ga-labeled imaging agents, maybe in conjunction with kits, based on better GMP-compliance, and for radioprotection reasons. Another reason might be early access to newly designed radiotracers, since kit formulations will only be developed for already established tracers, having demonstrated their clinical effectiveness.
Eventually, effective development of 68Ga cold kits will rely on easy access to 68Ga, either through a generator or a cyclotron, simplicity, and robustness of 68Ga incorporation into novel radiopharmaceuticals, successful clinical applications, and strong medical industry support.
Author Contributions
The author confirms being the sole contributor of this work and has approved it for publication.
Funding
This study was partly supported by Labex IRON (Grant No: ANR-11-LABX-0018) and Rennes Métropole (Grant No: 09 EML 2014).
Conflict of Interest
The author declares that the research was conducted in the absence of any commercial or financial relationships that could be construed as a potential conflict of interest.
Publisher's Note
All claims expressed in this article are solely those of the authors and do not necessarily represent those of their affiliated organizations, or those of the publisher, the editors and the reviewers. Any product that may be evaluated in this article, or claim that may be made by its manufacturer, is not guaranteed or endorsed by the publisher.
References
2. Griffeth LK. Use of Pet/Ct scanning in cancer patients: technical and practical considerations. Baylor Univ Med Cent Proc. (2005) 18:321–30. doi: 10.1080/08998280.2005.11928089
3. Torres Martin de Rosales R, Arstad E, Blower PJ. Nuclear imaging of molecular processes in cancer. Target Oncol. (2009) 4:183–97. doi: 10.1007/s11523-009-0120-2
4. Pysz MA, Gambhir SS, Willmann JK. Molecular imaging: current status and emerging strategies. Clin Radiol. (2010) 65:500–16. doi: 10.1016/j.crad.2010.03.011
5. Anderson CJ, Lewis JS. Current status and future challenges for molecular imaging. Phil Trans R Soc A. (2017) 375:20170023. doi: 10.1098/rsta.2017.0023
6. Jain SK. The promise of molecular imaging in the study and treatment of infectious diseases. Mol Imaging Biol. (2017) 19:341–7. doi: 10.1007/s11307-017-1055-0
7. Duclos V, Iep A, Gomez L, Goldfarb L, Besson FL. PET molecular imaging: a holistic review of current practice and emerging perspectives for diagnosis, therapeutic evaluation and prognosis in clinical oncology. Int J Mol Sci. (2021) 22:4159. doi: 10.3390/ijms22084159
8. Marcus C, Sheikhbahaei S, Shivamurthy VKN, Avey G, Subramaniam RM. PET imaging for head and neck cancers. Radiol Clin North Am. (2021) 59:773–88. doi: 10.1016/j.rcl.2021.05.005
9. Basu S. Personalized versus evidence-based medicine with PET-based imaging. Nat Rev Clin Oncol. (2010) 7:665–8. doi: 10.1038/nrclinonc.2010.121
10. Hicks R, Hofman M. Is there still a role for SPECT–CT in oncology in the PET–CT era? Nat Rev Clin Oncol. (2012) 9:712–20. doi: 10.1038/nrclinonc.2012.188
11. Zhang XY, Yang ZL, Lu GM, Yang GF, Zhang LJ. PET/MR imaging: new frontier in Alzheimer's disease and other dementias. Front Mol Neurosci. (2017) 10:343. doi: 10.3389/fnmol.2017.00343
12. Arnon-Sheleg E, Israel O, Keidar Z. PET/CT imaging in soft tissue infection and inflammation - an update. Semin Nucl Med. (2019) 50:35–49. doi: 10.1053/j.semnuclmed.2019.07.005
13. Elsinga P.H. Nuclear medicine imaging tracers for neurology. In: Dierckx RAJO, Otte A, de Vries EFJ, van Waarde A, Leenders KL, editors. PET and SPECT in Neurology. Cham: Springer (2021), 3–35. doi: 10.1007/978-3-030-53168-3_1
14. Balogh V, MacAskill MG, Hadoke PWF, Gray GA, Tavares AAS. Positron emission tomography techniques to measure active inflammation, fibrosis and angiogenesis: potential for non-invasive imaging of hypertensive heart failure. Front Cardiovasc Med. (2021) 8:719031. doi: 10.3389/fcvm.2021.719031
15. Kayani I, Groves AM. 18F-fluorodeoxyglucose PET/CT in cancer imaging. Clin Med. (2006) 6:240–4. doi: 10.7861/clinmedicine.6-3-240
16. Hofman MS, Hicks RJ. How we read oncologic FDG PET/CT. Cancer Imaging. (2016) 16:35. doi: 10.1186/s40644-016-0091-3
17. Kim SJ, Cho YS, Moon SH, Bae JM, Kim S, Choe YS, et al. Primary tumor 8F-FDG avidity affects the performance of 8F-FDG PET/CT for detecting gastric cancer recurrence. J Nucl Med. (2016) 57:544–50. doi: 10.2967/jnumed.115.163295
18. Shen K, Liu B, Zhou X, Ji Y, Chen L, Wang Q, et al. The evolving role of 18F-FDG PET/CT in diagnosis and prognosis prediction in progressive prostate cancer. Front Oncol. (2021) 11:683793. doi: 10.3389/fonc.2021.683793
19. Groves AM, Win T, Haim SB, Ell PJ. Non-[18F]FDG PET in clinical oncology. Lancet Oncol. (2007) 8:822–30. doi: 10.1016/S1470-2045(07)70274-7
20. Rice SL, Roney CA, Daumar P, Lewis JS. The next generation of positron emission tomography radiopharmaceuticals in oncology. Semin Nucl Med. (2011) 41:265–82. doi: 10.1053/j.semnuclmed.2011.02.002
21. Jiang L, Tu Y, Shi H, Cheng Z. PET probes beyond 18F-FDG. J Biomed Res. (2014) 28:435–46. doi: 10.7555/JBR.28.20130196
22. Kircher M, Lapa C. Infection and inflammation imaging: beyond FDG. PET Clin. (2020) 15:215–29. doi: 10.1016/j.cpet.2019.11.004
23. Lau J, Rousseau E, Kwon D, Lin KS, Bénard F, Chen X. Insight into the development of PET radiopharmaceuticals for oncology. Cancers. (2020) 12:1312. doi: 10.3390/cancers12051312
24. Lopci E, Fanti S. Non-FDG PET/CT. Recent Results Cancer Res. (2020) 216:669–718. doi: 10.1007/978-3-030-42618-7_20
25. Coenen HH, Ermert J. Expanding PET-applications in life sciences with positron-emitters beyond fluorine-18. Nucl Med Biol. (2021) 92:241–69. doi: 10.1016/j.nucmedbio.2020.07.003
26. Southworth R, Torres Martin de Rosales R, Meszaros LK, Ma MT, Mullen GED, Fruhwirth G, et al. Opportunities and challenges for metal chemistry in molecular imaging: from gamma camera imaging to PET and multimodality imaging. Adv Inorg Chem. (2016) 68:1–41. doi: 10.1016/bs.adioch.2015.09.001
27. Brandt M, Cardinale J, Aulsebrook ML, Gasser G, Mindt TL. An overview of PET radiochemistry, Part 2: radiometals. J Nucl Med. (2018) 59:1500–6. doi: 10.2967/jnumed.117.190801
28. Werner RA, Bluemel C, Allen-Auerbach MS, Higuchi T, Herrmann K. 68Gallium- and 90Yttrium-/177Lutetium: “theranostic twins” for diagnosis and treatment of NETs. Ann Nucl Med. (2015) 29:1–7. doi: 10.1007/s12149-014-0898-6
29. Maecke HR, André JP. 68Ga-PET radiopharmacy: a generator-based alternative to 18F-radiopharmacy. Ernst Schering Res Found Workshop. (2007) 62:215–42. doi: 10.1007/978-3-540-49527-7_8
30. Tanzey SS, Thompson S, Scott PJ, Brooks AF. Gallium-68: methodology and novel radiotracers for positron emission tomography (2012-2017). Pharm Pat Anal. (2018) 7:193–227. doi: 10.4155/ppa-2018-0016
31. Velikyan I. Positron emitting [68Ga]Ga-based imaging agents: chemistry and diversity. Med Chem. (2011) 7:345–79. doi: 10.2174/157340611796799195
32. Smith DL, Breeman WA, Sims-Mourtada J. The untapped potential of Gallium 68-PET: the next wave of 68Ga-agents. Appl RadiatIsot. (2013) 76:14–23. doi: 10.1016/j.apradiso.2012.10.014
33. Velikyan I. Prospective of 68Ga-radiopharmaceutical development. Theranostics. (2014) 4:47–80. doi: 10.7150/thno.7447
34. Velikyan I. Continued rapid growth in 68Ga applications: update 2013 to June 2014. J Labelled Comp Radiopharm. (2015) 58:99–121. doi: 10.1002/jlcr.3250
35. Vorster M, Maes A, WieleCv, Sathekge M. Gallium-68 PET: a powerful generator-based alternative to infection and inflammation imaging. Semin Nucl Med. (2016) 46:436–47. doi: 10.1053/j.semnuclmed.2016.04.005
36. Zhang J, Singh A, Kulkarni HR, Schuchardt C, Müller D, Wester HJ, et al. From bench to bedside-the bad berka experience with first-in-human studies. Semin Nucl Med. (2019) 49:422–37. doi: 10.1053/j.semnuclmed.2019.06.002
37. Bartholomä MD, Louie AS, Valliant JF, Zubieta J. Technetium and gallium derived radiopharmaceuticals: comparing and contrasting the chemistry of two important radiometals for the molecular imaging era. Chem Rev. (2010) 110:2903–20. doi: 10.1021/cr1000755
38. Chakravarty R, Chakraborty S, Ram R, Vatsa R, Bhusari P, Shukla J, et al. Detailed evaluation of different 68Ge/68Ga generators: an attempt toward achieving efficient 68Ga radiopharmacy. J Labelled Comp Radiopharm. (2016) 59:87–94. doi: 10.1002/jlcr.3371
39. Velikyan I. 68Ga-based radiopharmaceuticals: production and application relationship. Molecules. (2015) 20:12913–43. doi: 10.3390/molecules200712913
40. Gleason GI. A positron cow. Int J Appl RadiatIsot. (1960) 8:90–4. doi: 10.1016/0020-708X(60)90052-1
42. Zhernosekov KP, Filosofov DV, Baum RP, Aschoff P, Bihl H, Razbash AA, et al. Processing of generator-produced 68Ga for medical application. J Nucl Med. (2007) 48:1741–8. doi: 10.2967/jnumed.107.040378
43. Roesch F. Maturation of a key resource - the germanium-68/gallium-68 generator: development and new insights. CurrRadiopharm. (2012) 5:202–11. doi: 10.2174/1874471011205030202
44. Rösch F. Past, present and future of 68Ge/68Ga generators. ApplRadiatIsot. (2013) 76:24–30. doi: 10.1016/j.apradiso.2012.10.012
45. Mueller D, Fuchs A, Leshch Y, Proehl M. The shortage of approved 68Ge/68Ga generators - incoming material inspection and GMP compliant use of non-approved generators. J Nucl Med. (2019) 60(Suppl. 1):1059.
47. Kumar K. The current status of the production and supply of Gallium-68. Cancer BiotherRadiopharm. (2020) 35:163–6. doi: 10.1089/cbr.2019.3301
48. Becker KV, Chernysheva M, Barnhart TE, Gagnon K, Engle JW. A review of accelerator-produced Ga-68 with solid targets. CurrRadiopharm. (2021) 1:315–324. doi: 10.2174/1874471013666201224113651
49. Thisgaard H, Kumlin J, Langkjær N, Chua J, Hook B, Jensen M, et al. Multi-curie production of gallium-68 on a biomedical cyclotron and automated radiolabelling of PSMA-11 and DOTATATE. EJNMMI Radiopharm Chem. (2021) 6:1. doi: 10.1186/s41181-020-00114-9
50. do Carmo SJC, Scott PJH, Alves F. Production of radiometals in liquid targets. EJNMMI Radiopharm Chem. (2020) 5:2. doi: 10.1186/s41181-019-0088-x
51. Rodnick ME, Sollert C, Stark D, Clark M, Katsifis A, Hockley BG, et al. Cyclotron-based production of 68Ga, [68Ga]GaCl3, and [68Ga]Ga-PSMA-11 from a liquid target. EJNMMI Radiopharm Chem. (2020) 5:25. doi: 10.1186/s41181-020-00106-9
52. Talip Z, Favaretto C, Geistlich S, Meulen NPV. A step-by-step guide for the novel radiometal production for medical applications: case studies with 68Ga, 44Sc, 177Lu and 161Tb. Molecules. (2020) 25:966. doi: 10.3390/molecules25040966
53. Council of Europe, European Pharmacopoeia Commission, European Directorate for the Quality of Medicines & Healthcare. European Pharmacopoeia. 10th ed. Strasbourg: DeutscherApotheker Verlag (2020).
54. Jussing E, Milton S, Samén E, Moein MM, Bylund L, Axelsson R, et al. Clinically applicable cyclotron-produced Gallium-68 gives high-yield radiolabeling of DOTA-based tracers. Biomolecules. (2021) 11:1118. doi: 10.3390/biom11081118
55. McInnes LE, Rudd SE, Donnelly PS. Copper, gallium and zirconium positron emission tomography imaging agents: the importance of metal ion speciation. Coord Chem Rev. (2017) 352:499–516. doi: 10.1016/j.ccr.2017.05.011
56. Wadas TJ, Wong EH, Weisman GR, Anderson CJ. Coordinating radiometals of copper, gallium, indium, yttrium and zirconium for PET and SPECT imaging of disease. Chem Rev. (2010) 110:2858–902. doi: 10.1021/cr900325h
57. Kulprathipanja S, Hnatowich DJ. A method for determining the pH stability range of gallium radiopharmaceuticals. Int J Appl RadiatIsot. (1977) 28:229–33. doi: 10.1016/B978-0-08-021344-6.50028-8
58. Bauwens M, Chekol R, Vanbilloen H, Bormans G, Verbruggen A. Optimal buffer choice of the radiosynthesis of 68Ga-Dotatoc for clinical application. Nucl Med Commun. (2010) 31:753–8. doi: 10.1097/MNM.0b013e32833acb99
59. Martins AF, Prata MI, Rodrigues SP, Geraldes CF, Riss PJ, Amor-Coarasa A, et al. Spectroscopic, radiochemical, and theoretical studies of the Ga3+-N-2-hydroxyethyl piperazine-N'-2-ethanesulfonic acid (HEPES buffer) system: evidence for the formation of Ga3+ - HEPES complexes in 68Ga labeling reactions. Contrast Media Mol Imaging. (2013) 8:265–73. doi: 10.1002/cmmi.1517
60. le Roux J, Kleynhans J, Rubow S. The use of HEPES-buffer in the production of gallium-68 radiopharmaceuticals - time to reconsider strict pharmacopoeial limits? EJNMMI Radiopharm Chem. (2021) 6:15. doi: 10.1186/s41181-021-00129-w
61. Harris WR, Pecoraro VL. Thermodynamic binding constants for gallium transferrin. Biochemistry. (1983) 22:292–9. doi: 10.1021/bi00271a010
62. Bandoli G, Dolmella A, Tisato F, Porchia M, Refosco F. Mononuclear sixcoordinatedGa(III) complexes: a comprehensive survey. Coord Chem Rev. (2009) 253:56–77. doi: 10.1016/j.ccr.2007.12.001
63. Notni J, Pohle K, Wester HJ. Comparative gallium-68 labeling of TRAP-, NOTA-, and DOTA-peptides: practical consequences for the future of gallium-68-PET. EJNMMI Res. (2012) 2:28. doi: 10.1186/2191-219X-2-28
64. Burke BP, Clemente GS, Archibald SJ. Recent advances in chelator design and labelling methodology for 68Ga radiopharmaceuticals. J Labelled Comp Radiopharm. (2014) 57:239–43. doi: 10.1002/jlcr.3146
65. Price TW, Greenman J, Stasiuk GJ. Current advances in ligand design for inorganic positron emission tomography tracers 68Ga, 64Cu, 89Zr and 44Sc. Dalton Trans. (2016) 45:15702–24. doi: 10.1039/C5DT04706D
66. Spang P, Herrmann C, Roesch F. Bifunctional Gallium-68 chelators: past, present, and future. Semin Nucl Med. (2016) 46:373–94. doi: 10.1053/j.semnuclmed.2016.04.003
67. Tsionou MI, Knapp CE, Foley CA, Munteanu CR, Cakebread A, Imberti C, et al. Comparison of macrocyclic and acyclic chelators for gallium-68 radiolabelling. RSC Adv. (2017) 7:49586–99. doi: 10.1039/C7RA09076E
68. Welch MJ, Thakur ML, Coleman RE, Patel M, Siegel BA, Ter-Pogossian M. Gallium-68 labeled red cells and platelets: new agents for positron tomography. J Nucl Med. (1977) 18:558–62.
69. Gündel D, Pohle U, Prell E, Odparlik A, Thews O. Assessing glomerular filtration in small animals using [68Ga]DTPA and [68Ga]EDTA with PET imaging. Mol Imaging Biol. (2018) 20:457–64. doi: 10.1007/s11307-017-1135-1
70. Petrik M, Umlaufova E, Raclavsky V, Palyzova A, Havlicek V, Pfister J, et al. 68Ga-labelled desferrioxamine-B for bacterial infection imaging. Eur J Nucl Med Mol Imaging. (2021) 48:372–82. doi: 10.1007/s00259-020-04948-y
71. Boros E, Ferreira CL, Cawthray JF, Price EW, Patrick BO, Wester DW, et al. Acyclic chelate with ideal properties for 68Ga PET imaging agent elaboration. J Am Chem Soc. (2010) 132:15726–33. doi: 10.1021/ja106399h
72. Boros E, Ferreira CL, Yapp DT, Gill RK, Price EW, Adam MJ, et al. RGD conjugates of the H2dedpa scaffold: synthesis, labeling and imaging with 68Ga. Nucl Med Biol. (2012) 39:785–94. doi: 10.1016/j.nucmedbio.2012.01.003
73. Chaves S, Mendonça AC, Marques SM, Prata MI, Santos AC, Martins AF, et al. A gallium complex with a new tripodal tris-hydroxypyridinone for potential nuclear diagnostic imaging: solution and in vivo studies of 67Ga-labeled species. J InorgBiochem. (2011) 105:31–8. doi: 10.1016/j.jinorgbio.2010.09.012
74. Cusnir R, Imberti C, Hider RC, Blower PJ, Ma MT. Hydroxypyridinone chelators: from iron scavenging to radiopharmaceuticals for PET Imaging with Gallium-68. Int J Mol Sci. (2017) 18:116. doi: 10.3390/ijms18010116
75. Cusnir R, Cakebread A, Cooper MS, Young JD, Blower PJ, Ma MT. The effects of trace metal impurities on Ga-68-radiolabelling with a tris(3-hydroxy-1,6-dimethylpyridin-4-one) (THP) chelator. RSC Adv. (2019) 9:37214–37221. doi: 10.1039/C9RA07723E
76. Zhou X, Dong L, Shen L. Hydroxypyridinones as a very promising platform for targeted diagnostic and therapeutic radiopharmaceuticals. Molecules. (2021) 26:6997. doi: 10.3390/molecules26226997
77. Imberti C, Chen YL, Foley CA, Ma MT, Paterson BM, Wang Y, et al. Tuning the properties of tris(hydroxypyridinone) ligands: efficient 68Ga chelators for PET imaging. Dalton Trans. (2019) 48:4299–313. doi: 10.1039/C8DT04454F
78. Eplattenier FL, Murase I, Martell AE. New multidentate ligands. VI. chelating tendencies of N,N′-Di(2-hydroxybenzyl)ethylenediamine-N,N′-diacetic Acid. J Am Chem Soc. (1967) 89:837–43. doi: 10.1021/ja00980a019
79. Eder M, Wängler B, Knackmuss S, LeGall F, Little M, Haberkorn U, et al. Tetrafluorophenolate of HBED-CC: a versatile conjugation agent for 68Ga-labeled small recombinant antibodies. Eur J Nucl Med Mol Imaging. (2008) 35:1878–86. doi: 10.1007/s00259-008-0816-z
80. Ebenhan T, Vorster M, Marjanovic-Painter B, Wagener J, Suthiram J, Modiselle M, et al. Development of a single vial kit solution for radiolabeling of 68Ga-DKFZ-PSMA-11 and its performance in prostate cancer patients. Molecules. (2015) 20:14860–78. doi: 10.3390/molecules200814860
81. Satpati D, Vats K, Sharma R, Kameswaran M, Sarma HD, Dash A. Synthesis, radiolabeling, and evaluation of gastrin releasing peptide receptor antagonist 68Ga-HBED-CC-RM26. J Labelled Comp Radiopharm. (2019) 62:843–9. doi: 10.1002/jlcr.3795
82. Klika KD, Da Pieve C, Kopka K, Smith G, Makarem A. Synthesis and application of a thiol-reactive HBED-type chelator for development of easy-to-produce Ga-radiopharmaceutical kits and imaging probes. Org Biomol Chem. (2021) 19:1722–6. doi: 10.1039/D0OB02513E
83. Stasiuk GJ, Long NJ. The ubiquitous DOTA and its derivatives: the impact of 1,4,7,10-tetraazacyclododecane-1,4,7,10-tetraacetic acid on biomedical imaging. Chem Commun. (2013) 49:2732–46. doi: 10.1039/c3cc38507h
84. Eychenne R, Bouvry C, Bourgeois M, Loyer P, Benoist E, Lepareur N. Overview of radiolabeled somatostatin analogs for cancer imaging and therapy. Molecules. (2020) 25:4012. doi: 10.3390/molecules25174012
85. Šimeček J, Schulz M, Notni J, Plutnar J, Kubíček V, Havlíčková J, et al. Complexation of metal ions with TRAP (1,4,7-triazacyclononane phosphinic acid) ligands and 1,4,7-triazacyclononane-1,4,7-triacetic acid: phosphinate-containing ligands as unique chelators for trivalent gallium. Inorg Chem. (2012) 51:577–90. doi: 10.1021/ic202103v
86. Prata MI, Santos AC, Geraldes CF, de Lima JJ. Structural and in vivo studies of metal chelates of Ga(III) relevant to biomedical imaging. J InorgBiochem. (2000) 79:359–63. doi: 10.1016/S0162-0134(99)00232-9
87. Notni J, Šimeček J, Wester HJ. Phosphinic acid functionalized polyazacycloalkane chelators for radiodiagnostics and radiotherapeutics: unique characteristics and applications. ChemMedChem. (2014) 9:1107–15. doi: 10.1002/cmdc.201400055
88. Šimeček J, Hermann P, Wester HJ, Notni J. How is 68Ga labeling of macrocyclic chelators influenced by metal ion contaminants in 68Ge/68Ga generator eluates? ChemMedChem. (2013) 8:95–103. doi: 10.1002/cmdc.201200471
89. Vágner A, Forgács A, Brücher E, Tóth I, Maiocchi A, Wurzer A, et al. Equilibrium thermodynamics, formation, and dissociation kinetics of trivalent iron and gallium complexes of Triazacyclononane-Triphosphinate (TRAP) chelators: unraveling the foundations of highly selective Ga-68 labeling. Front Chem. (2018) 6:170. doi: 10.3389/fchem.2018.00170
90. Notni J, Šimeček J, Hermann P, Wester HJ. TRAP, a powerful and versatile framework for gallium-68 radiopharmaceuticals. Chemistry. (2011) 17:14718–22. doi: 10.1002/chem.201103503
91. Waldron BP, Parker D, Burchardt C, Yufit DS, Zimny M, Roesch F. Structure and stability of hexadentate complexes of ligands based on AAZTA for efficient PET labelling with gallium-68. Chem Commun. (2013) 49:579–81. doi: 10.1039/C2CC37544C
92. Baranyai Z, Uggeri F, Maiocchi A, Giovenzana GB, Cavallotti C, Takács A, et al. Equilibrium, kinetic and structural studies of AAZTA complexes with Ga3+, In3+ and Cu2+. Eur J Inorg Chem. (2013) 2013:147–62. doi: 10.1002/ejic.201201108
93. Farkas E, Nagel J, Waldron BP, Parker D, Tóth I, Brücher E, et al. Equilibrium, kinetic and structural properties of Gallium(III) and some divalent metal complexes formed with the new DATAm and DATA5m ligands. Chemistry. (2017) 23:10358–71. doi: 10.1002/chem.201701508
94. Seemann J, Waldron BP, Roesch F, Parker D. Approaching 'Kit-Type' Labelling with 68Ga: the DATA chelators. ChemMedChem. (2015) 10:1019–26. doi: 10.1002/cmdc.201500092
95. Greifenstein L, Grus T, Nagel J, Sinnes JP, Rösch F. Synthesis and labeling of a squaric acid containing PSMA-inhibitor coupled to AAZTA5 for versatile labeling with 44Sc, 64Cu, 68Ga and 177Lu. Appl RadiatIsot. (2020) 156:108867. doi: 10.1016/j.apradiso.2019.108867
96. Fani M, Del Pozzo L, Abiraj K, Mansi R, Tamma ML, Cescato R, et al. PET of somatostatin receptor-positive tumors using 64Cu- and 68Ga-somatostatin antagonists: the chelate makes the difference. J Nucl Med. (2011) 52:1110–8. doi: 10.2967/jnumed.111.087999
97. Varasteh Z, Mitran B, Rosenström U, Velikyan I, Rosestedt M, Lindeberg G, et al. The effect of macrocyclic chelators on the targeting properties of the 68Ga-labeled gastrin releasing peptide receptor antagonist PEG2-RM26. Nucl Med Biol. (2015) 42:446–54. doi: 10.1016/j.nucmedbio.2014.12.009
98. Renard E, Moreau M, Bellaye PS, Guillemin M, Collin B, Prignon A, et al. Positron emission tomography imaging of neurotensin receptor-positive tumors with 68Ga-Labeled antagonists: the chelate makes the difference again. J Med Chem. (2021) 64:8564–78. doi: 10.1021/acs.jmedchem.1c00523
99. Ma MT, Cullinane C, Waldeck K, Roselt P, Hicks RJ, Blower PJ. Rapid kit-based 68Ga-labelling and PET imaging with THP-Tyr3-octreotate: a preliminary comparison with DOTA-Tyr3-octreotate. EJNMMI Res. (2015) 5:52. doi: 10.1186/s13550-015-0131-1
100. Sinnes JP, Bauder-Wüst U, Schäfer M, Moon ES, Kopka K, Rösch F. 68Ga, 44Sc and 177Lu-labeled AAZTA5-PSMA-617: synthesis, radiolabeling, stability and cell binding compared to DOTA-PSMA-617 analogues. EJNMMI Radiopharm Chem. (2020) 5:28. doi: 10.1186/s41181-020-00107-8
101. KolencPeitl P, Rangger C, Garnuszek P, Mikolajczak R, Hubalewska-Dydejczyk A, Maina T, et al. Clinical translation of theranostic radiopharmaceuticals: current regulatory status and recent examples. J Labelled Comp Radiopharm. (2019) 62:673–83. doi: 10.1002/jlcr.3712
102. Decristoforo C, Neels O, Patt M. Emerging radionuclides in a regulatory framework for medicinal products - how do they fit? Front Med. (2021) 8:678452. doi: 10.3389/fmed.2021.678452
103. Boschi S, Malizia C, Lodi F. Overview and perspectives on automation strategies in 68Ga radiopharmaceutical preparations. Recent Results Cancer Res. (2013) 194:17–31. doi: 10.1007/978-3-642-27994-2_2
104. Decristoforo C, Knopp R, von Guggenberg E, Rupprich M, Dreger T, Hess A, et al. A fully automated synthesis for the preparation of 68Ga-labelled peptides. Nucl Med Commun. (2007) 28:870–5. doi: 10.1097/MNM.0b013e3282f1753d
105. Petrik M, Knetsch PA, Knopp R, Imperato G, Ocak M, von Guggenberg E, et al. Radiolabelling of peptides for PET, SPECT and therapeutic applications using a fully automated disposable cassette system. Nucl Med Commun. (2011) 32:887–95. doi: 10.1097/MNM.0b013e3283497188
106. Manoharan P, Lamarca A, Navalkissoor S, Calero J, Chan PS, Julyan P, et al. Safety, tolerability and clinical implementation of 'ready-to-use' 68gallium-DOTA0-Tyr3-octreotide (68Ga-DOTATOC) (SomaKIT TOC) for injection in patients diagnosed with gastroenteropancreatic neuroendocrine tumours (GEP-NETs). ESMO Open. (2020) 5:e000650. doi: 10.1136/esmoopen-2019-000650
107. Pfaff S, Philippe C, Pichler V, Hacker M, Mitterhauser M, Wadsak W. Microfluidic 68Ga-labeling: a proof of principle study. Dalton Trans. (2018) 47:5997–6004. doi: 10.1039/C8DT00158H
108. Zhang X, Liu F, Payne AC, Nickels ML, Bellan LM, Manning HC. High-yielding radiosynthesis of [68Ga]Ga-PSMA-11 using a low-cost microfluidic device. Mol Imaging Biol. (2020) 22:1370–9. doi: 10.1007/s11307-020-01515-7
109. Satpati D. Recent breakthrough in 68Ga-Radiopharmaceuticals cold kits for convenient PET radiopharmacy. Bioconjug Chem. (2021) 32:430–47. doi: 10.1021/acs.bioconjchem.1c00010
110. Mukherjee A. An update on extemporaneous preparation of radiopharmaceuticals using freeze-dried cold kits. Mini Rev Med Chem. (2021) 21:1322–36. doi: 10.2174/1389557520999201214233634
111. Morgat C. Labelling cold kit with 68Ga - the future is bright. Eur J Nucl Med Mol Imaging. (2021) 48:S243. doi: 10.1007/s00259-021-05547-1
112. Nowotnik DP. Physico-chemical concepts in the preparation of technetium radiopharmaceuticals. In: Sampson CB, editor. Textbook of Radiopharmacy, Theory and Practice, 2nd ed. Amsterdam, NL: Gordon and Breach Science Publishers (1994). p. 29–50.
113. Asti M, Iori M, Capponi PC, Rubagotti S, Fraternali A, Versari A. Development of a simple kit-based method for preparation of pharmaceutical-grade 68Ga-DOTATOC. Nucl Med Commun. (2015) 36:502–10. doi: 10.1097/MNM.0000000000000275
114. Vats K, Sharma R, Kameswaran M, Satpati D, Dash A. Single vial cold kits optimized for preparation of gastrin releasing peptide receptor (GRPR)-radioantagonist68Ga-RM2 using three different 68Ge/68Ga generators. J Pharm Biomed Anal. (2019) 163:39–44. doi: 10.1016/j.jpba.2018.09.045
115. Lin M, Ta R, Day A, Moin C, Le D, Ravizzini G. Validating cyclotron-produced 68Ga as an alternative source for compounding radiopharmaceutical kits. J Nucl Med. (2020) 61:466.
116. Summary of Product Characteristics of SomaKit TOC, INN-edotreotide (europa,.eu). Available online at: https://www.ema.europa.eu/en/documents/product-information/somakit-toc-epar-product-information_en.pdf (accessed August 06, 2021)
117. Satpati D, Shinto A, Kamaleshwaran KK, Sarma HD, Dash A. Preliminary PET/CT imaging with somatostatin analogs [68Ga]DOTAGA-TATE and [68Ga]DOTAGA-TOC. Mol Imaging Biol. (2017) 19:878–84. doi: 10.1007/s11307-017-1072-z
118. Satpati D, Shinto A, Kamaleshwaran KK, Sane S, Banerjee S. Convenient preparation of [68Ga]DKFZ-PSMA-11 using a robust single-vial kit and demonstration of its clinical efficacy. Mol Imaging Biol. (2016) 18:420–7. doi: 10.1007/s11307-016-0943-z
119. Hennrich U, Eder M. [68Ga]Ga-PSMA-11: the first FDA-Approved 68Ga-Radiopharmaceutical for PET imaging of prostate cancer. Pharmaceuticals. (2021) 14:713. doi: 10.3390/ph14080713
120. Afaq A, Payne H, Davda R, Hines J, Cook GJR, Meagher M, et al. A Phase II, Open-label study to assess safety and management change using 68Ga-THP PSMA PET/CT in patients with high risk primary prostate cancer or biochemical recurrence after radical treatment: the PRONOUNCED study. J Nucl Med. (2021) 62:1727–34. doi: 10.2967/jnumed.120.257527
121. Lahnif H, Grus T, Pektor S, Greifenstein L, Schreckenberger M, Rösch F. Hybrid chelator-based PSMA radiopharmaceuticals: translational approach. Molecules. (2021) 26:6332. doi: 10.3390/molecules26216332
122. Pretze M, Reffert L, Diehl S, Schönberg SO, Wängler C, Hohenberger P, et al. GMP-compliant production of [68Ga]Ga-NeoB for positron emission tomography imaging of patients with gastrointestinal stromal tumor. EJNMMI Radiopharm Chem. (2021) 6:22. doi: 10.1186/s41181-021-00137-w
123. Amor-Coarasa A, Schoendorf M, Meckel M, Vallabhajosula S, Babich JW. Comprehensive quality control of the ITG 68Ge/68Ga generator and synthesis of 68Ga-DOTATOC and 68Ga-PSMA-HBED-CC for clinical imaging. J Nucl Med. (2016) 57:1402–5. doi: 10.2967/jnumed.115.171249
124. Golan H, Esa M, Moshkoviz K, Feldhaim A, Hoch B, Shalom E. Enhancing capacity and synthesis of [68Ga]68-Ga-PSMA-HBED-CC with the lyophilized ready-to-use kit for nuclear pharmacy applications. Nucl Med Commun. (2020) 41:986–90. doi: 10.1097/MNM.0000000000001232
125. Chastel A, Vimont D, Claverol S, Zerna M, Bodin S, Berndt M, et al. 68Ga-Radiolabeling and pharmacological characterization of a kit-based formulation of the Gastrin-Releasing Peptide Receptor (GRP-R) antagonist RM2 for convenient preparation of [68Ga]Ga-RM2. Pharmaceutics. (2021) 13:1160. doi: 10.3390/pharmaceutics13081160
126. Calderoni L, Farolfi A, Pianori D, Maietti E, Cabitza V, Lambertini A, et al. Evaluation of an automated module synthesis and a sterile cold kit-based preparation of 68Ga-PSMA-11 in patients with prostate cancer. J Nucl Med. (2020) 61:716–22. doi: 10.2967/jnumed.119.231605
127. Kleynhans J, Rubow S, le Roux J, Marjanovic-Painter B, Zeevaart JR, Ebenhan T. Production of [68Ga]Ga-PSMA: Comparing a manual kit-based method with a module-based automated synthesis approach. J Labelled Comp Radiopharm. (2020) 63:553–63. doi: 10.1002/jlcr.3879
128. Meisenheimer M, Kürpig S, Essler M, Eppard E. Manual vs automated 68Ga-radiolabelling-A comparison of optimized processes. J Labelled Comp Radiopharm. (2020) 63:162–73. doi: 10.1002/jlcr.3821
129. Frindel M, Rauscher A, Baumgartner P, Faivre-Chauvet A, Varmenot N, Kraeber-Bodéré F, et al. Comparison of automated and manual labeling methods for somatostatin analogues with the example of DOTANOC and SomaKit TOC®. EJNMMI Radiopharm Chem. (2018) 3:7.
130. Migliari S, Sammartano A, Scarlattei M, Baldari G, Janota B, Bonadonna RC, et al. Feasibility of a scale-down production of [68Ga]Ga-NODAGA-Exendin-4 in a hospital based radiopharmacy. CurrRadiopharm. (2021). doi: 10.2174/1874471014666210309151930 [Epub ahead of print].
131. Revy A, Hallouard F, Joyeux-Klamber S, Skanjeti A, Rioufol C, Fraysse M. Feasibility and evaluation of automated methods for radiolabeling of radiopharmaceutical kits with Gallium-68. CurrRadiopharm. (2019) 12:229–37. doi: 10.2174/1874471012666190110170623
132. Mueller D, Kulkarni H, Baum RP, Odparlik A. Rapid synthesis of 68Ga-labeled macroaggregated human serum albumin (MAA) for routine application in perfusion imaging using PET/CT. Appl RadiatIsot. (2017) 122:72–7. doi: 10.1016/j.apradiso.2017.01.003
133. Burke BP, Archibald SJ. Labeling with Gallium-68. In: Kilbourn MR, Scott PJH, editors. Handbook of Radiopharmaceuticals: Methodology and Applications, 2nd ed. Hoboken, NJ: Wiley-Blackwell (2021). p. 291–323. doi: 10.1002/9781119500575.ch9
134. Foens CS, Fairobent LA, Guiberteau MJ; Nuclear Regulatory Commission. 10 CFR Part 35: changes to the NRC rule governing the medical use of radioisotopes and implications for radiologic practice. J Am Coll Radiol. (2006) 3:96–101. doi: 10.1016/j.jacr.2005.09.012
135. Lange R, ter Heine R, Decristoforo C, Peñuelas I, Elsinga PH, van der Westerlaken MM, et al. Untangling the web of European regulations for the preparation of unlicensed radiopharmaceuticals: a concise overview and practical guidance for a risk-based approach. Nucl Med Commun. (2015) 36:414–22. doi: 10.1097/MNM.0000000000000276
136. Decristoforo C, Penuelas I, Patt M, Todde S. European regulations for the introduction of novel radiopharmaceuticals in the clinical setting. Q J Nucl Med Mol Imaging. (2017) 61:135–44. doi: 10.23736/S1824-4785.17.02965-X
137. Directive 2001/83/EC of the European Parliament and of the Council of 6 November 2001 on the Community Code Relating to Medicinal Products for Human Use. (2001). Available online at: https://eur-lex.europa.eu/LexUriServ/LexUriServ.do?uri=CELEX:32001L0083:EN:HTML (accessed December 06, 2021).
138. Neels O, Patt M, Decristoforo C. Radionuclides: medicinal products or rather starting materials? EJNMMI Radiopharm Chem. (2019) 4:22. doi: 10.1186/s41181-019-0074-3
139. Commission Directive 2003/94/EC of 8 October 2003 Laying Down the Principles and Guidelines of Good Manufacturing Practice in Respect of Medicinal Products for Human Use and Investigational Medicinal Products for Human Use (Text with EEA relevance). (2003). Available online at: eur-lex.europa.eu/legal-content/EN/TXT/HTML/?uri=CELEX:32003L0094&qid=1639047815389&from=EN (accessed December 06, 2021)
140. Decristoforo C, Patt M. Are we “preparing” radiopharmaceuticals? EJNMMI Radiopharm Chem. (2017) 1:12. doi: 10.1186/s41181-016-0011-7
141. Gillings N, Hjelstuen O, Ballinger J, Behe M, Decristoforo C, Elsinga P, et al. Guideline on current good radiopharmacy practice (cGRPP) for the small-scale preparation of radiopharmaceuticals. EJNMMI Radiopharm Chem. (2021) 6:8. doi: 10.1186/s41181-021-00123-2
142. Schmidt A, Schottelius M, Herz M, Wester H. Production of clinical radiopharmaceuticals: general pharmaceutical and radioanalytical aspects. J RadioanalNucl Chem. (2016) 311:1551–7. doi: 10.1007/s10967-016-5125-6
143. European Directorate of Quality of Medicines. Compounding of radiopharmaceuticals. Pharmeuropa. (2011) 23:643.
144. Compounding and Repackaging of Radiopharmaceuticals By Outsourcing Facilities Guidance for Industry. (2018). Available online at: https://www.fda.gov/regulatory-information/search-fda-guidance-documents/compounding-and-repackaging-radiopharmaceuticals-outsourcing-facilities-guidance-industry (accessed December13, 2021)
145. Summary of Product Characteristics of NETSPOT (fda.gov). Available online at: https://www.accessdata.fda.gov/drugsatfda_docs/label/2016/208547s000lbl.pdf (accessed August 06, 2021)
146. Seemann J, Waldron B, Parker D, Roesch F. DATATOC: a novel conjugate for kit-type 68Ga labelling of TOC at ambient temperature. EJNMMI Radiopharm Chem. (2017) 1:4. doi: 10.1186/s41181-016-0007-3
147. Satpati D, Vats K. Development of freeze-dried kits for one-step expeditious formulation of [68Ga]Ga-NODAGA-JR11. Nucl Med Biol. (2021) 96–97:S47. doi: 10.1016/S0969-8051(21)00340-1
148. Beheshti M, Paymani Z, Brilhante J, Geinitz H, Gehring D, Leopoldseder T, et al. Optimal time-point for 68Ga-PSMA-11 PET/CT imaging in assessment of prostate cancer: feasibility of sterile cold-kit tracer preparation? Eur J Nucl Med Mol Imaging. (2018) 45:1188–96. doi: 10.1007/s00259-018-3970-y
149. Kurash MM, Gill R, Khairulin M, Harbosh H, Keidar Z. 68Ga-labeled PSMA-11 (68Ga-isoPROtrace-11) synthesized with ready to use kit: normal biodistribution and uptake characteristics of tumour lesions. Sci Rep. (2020) 10:3109. doi: 10.1038/s41598-020-60099-y
150. Young JD, Abbate V, Imberti C, Meszaros LK, Ma MT, Terry SYA, et al. 68Ga-THP-PSMA: a PET imaging agent for prostate cancer offering rapid, room-temperature, 1-step kit-based radiolabeling. J Nucl Med. (2017) 58:1270–7. doi: 10.2967/jnumed.117.191882
151. Pandey U, Mukherjee A, Jindal A, Gamre N, Korde A, Ram R, et al. Preparation and evaluation of a single vial AMBA kit for 68Ga labeling with potential for imaging of GRP receptor-positive cancers. J RadioanalNucl Chem. (2016) 307:1115–24. doi: 10.1007/s10967-015-4290-3
152. Ebenhan T, Schoeman I, Rossouw DD, Grobler A, Marjanovic-Painter B, Wagener J, et al. Evaluation of a flexible NOTA-RGD kit solution using Gallium-68 from different 68Ge/68Ga-generators: pharmacokinetics and biodistribution in nonhuman primates and demonstration of solitary pulmonary nodule imaging in humans. Mol Imaging Biol. (2017) 19:469–82. doi: 10.1007/s11307-016-1014-1
153. Bhusari P, Bhatt J, Sood A, Kaur R, Vatsa R, Rastogi A, et al. Evaluating the potential of kit-based 68Ga-ubiquicidin formulation in diagnosis of infection: a pilot study68Ga. Nucl Med Commun. (2019) 40:228–34. doi: 10.1097/MNM.0000000000000943
154. Baudhuin H, Van Bockstal PJ, De Beer T, Vaneycken I, Bridoux J, Raes G, et al. Lyophilization of NOTA-sdAbs: First step towards a cold diagnostic kit for 68Ga-labeling. Eur J Pharm Biopharm. (2021) 166:194–204. doi: 10.1016/j.ejpb.2021.06.012
155. Yu HM, Chan CH, Chen JH, Chien CY, Wang PY, Juan WC, et al. Development of single vial kits for preparation of 68Ga-labelled hexavalent lactoside for PET imaging of asialoglycoprotein receptor. J Labelled Comp Radiopharm. (2018) 61:885–94. doi: 10.1002/jlcr.3673
156. Yang BY, Jeong JM, Kim YJ, Choi JY, Lee YS, Lee DS, et al. Formulation of 68Ga BAPEN kit for myocardial positron emission tomography imaging and biodistribution study. Nucl Med Biol. (2010) 37:149–55. doi: 10.1016/j.nucmedbio.2009.10.010
157. Shi S, Zhang L, Wu Z, Zhang A, Hong H, Choi SR, et al. [68Ga]Ga-HBED-CC-DiAsp: a new renal function imaging agent. Nucl Med Biol. (2020) 82-83:17–24. doi: 10.1016/j.nucmedbio.2019.12.005
158. Mitterhauser M, Toegel S, Wadsak W, Lanzenberger RR, Mien LK, Kuntner C, et al. Pre vivo, ex vivo and in vivo evaluations of [68Ga]-EDTMP. Nucl Med Biol. (2007) 34:391–7. doi: 10.1016/j.nucmedbio.2007.03.002
159. Lim JC, Choi SM, Cho EH, Lee SY, Dho SH, Kim SY. Development of freeze-dried DOTMP kits for labeling with 68Ga. J Radiat Indus. (2015) 9:63–8. doi: 10.23042/radin.2015.9.2.63
160. Keeling GP, Sherin B, Kim J, San Juan B, Grus T, Eykyn TR, et al. [68Ga]Ga-THP-Pam: a bisphosphonate PET tracer with facile radiolabeling and broad calcium mineral affinity. Bioconjug Chem. (2021) 32:1276–89. doi: 10.1021/acs.bioconjchem.0c00401
161. Hong H, Ploessl K, Zha Z, Wang H, Guo R, Xie Q, et al. Development and validation of a kit formulation of [68Ga]Ga-P15-041 as a bone imaging agent. Appl RadiatIsot. (2021) 169:109485. doi: 10.1016/j.apradiso.2020.109485
162. Even GA, Green MA. Gallium-68-labeled macroaggregated human serum albumin, 68Ga-MAA. Int J Rad Appl Instrum B. (1989) 16:319–21. doi: 10.1016/0883-2897(89)90014-7
163. Mathias CJ, Green MA. A convenient route to [68Ga]Ga-MAA for use as a particulate PET perfusion tracer. Appl RadiatIsot. (2008) 66:1910–2. doi: 10.1016/j.apradiso.2008.06.004
164. Maus S, Buchholz HG, Ament S, Brochhausen C, Bausbacher N, Schreckenberger M. Labelling of commercially available human serum albumin kits with 68Ga as surrogates for 99mTc-MAA microspheres. Appl RadiatIsot. (2011) 69:171–5. doi: 10.1016/j.apradiso.2010.09.008
165. Amor-Coarasa A, Milera A, Carvajal D, Gulec S, McGoron AJ. Lyophilized kit for the preparation of the PET perfusion agent [68Ga]-MAA. Int J Mol Imaging. (2014) 2014:269365. doi: 10.1155/2014/269365
166. Shanehsazzadeh S, Lahooti A, Yousefnia H, Geramifar P, Jalilian AR. Comparison of estimated human dose of 68Ga-MAA with 99mTc-MAA based on rat data. Ann Nucl Med. (2015) 29:745–53. doi: 10.1007/s12149-015-0997-z
167. Marenco M, Canziani L, De Matteis G, Cavenaghi G, Aprile C, Lodola L. Chemical and physical characterisation of human serum albumin nanocolloids: kinetics, strength and specificity of bonds with 99mTc and 68Ga. Nanomaterials. (2021) 11:1776. doi: 10.3390/nano11071776
168. Hsieh W, Ali M, Praehofer R, Tsopelas C. 68Ga-Ca-phytate particles: a potential lung perfusion agent of synthetic origin prepared in a cold kit format. J Labelled Comp Radiopharm. (2016) 59:506–16. doi: 10.1002/jlcr.3441
169. Verger E, Drion P, Meffre G, Bernard C, Duwez L, Lepareur N, et al. 68Ga and 188Re starch-based microparticles as theranostic tool for the hepatocellular carcinoma: radiolabeling and preliminary in vivo rat studies. PLoS One. (2016) 11:e0164626. doi: 10.1371/journal.pone.0164626
170. Virgolini I, Ambrosini V, Bomanji JB, Baum RP, Fanti S, Gabriel M, et al. Procedure guidelines for PET/CT tumour imaging with 68Ga-DOTA-conjugated peptides: 68Ga-DOTA-TOC, 68Ga-DOTA-NOC, 68Ga-DOTA-TATE. Eur J Nucl Med Mol Imaging. (2010) 37:2004–10. doi: 10.1007/s00259-010-1512-3
171. Mukherjee A, Pandey U, Chakravarty R, Sarma HD, Dash A. Development of single vial kits for preparation of 68Ga-labelled peptides for PET imaging of neuroendocrine tumours. Mol Imaging Biol. (2014) 16:550–7. doi: 10.1007/s11307-014-0719-2
172. Pandey U, Korde A, Mukherjee A, Shinto A, Kamaleswaran KK, Jose RP, et al. Clinical experience with indigenous kit-based preparation of 68Ga-DOTATOC using commercial 68Ge/68Ga generator. Appl RadiatIsot. (2018) 136:59–64. doi: 10.1016/j.apradiso.2018.02.002
173. Prince D, Rossouw D, Davids C, Rubow S. Development and evaluation of user-friendly single vial DOTA-Peptide kit formulations, specifically designed for radiolabelling with 68Ga from a tin Dioxide 68Ge/68Ga generator. Mol Imaging Biol. (2017) 19:817–24. doi: 10.1007/s11307-017-1077-7
174. Hennrich U, Benešová M. [68Ga]Ga-DOTA-TOC: the first FDA-Approved 68Ga-Radiopharmaceutical for PET imaging. Pharmaceuticals. (2020) 13:38. doi: 10.3390/ph13030038
175. Sinnes JP, Nagel J, Waldron BP, Maina T, Nock BA, Bergmann RK, et al. Instant kit preparation of 68Ga-radiopharmaceuticals via the hybrid chelator DATA: clinical translation of [68Ga]Ga-DATA-TOC. EJNMMI Res. (2019) 9:48. doi: 10.1186/s13550-019-0516-7
176. Yadav D, Ballal S, Yadav MP, Tripathi M, Roesch F, Bal C. Evaluation of [68Ga]Ga-DATA-TOC for imaging of neuroendocrine tumours: comparison with [68Ga]Ga-DOTA-NOC PET/CT. Eur J Nucl Med Mol Imaging. (2020) 47:860–9. doi: 10.1007/s00259-019-04611-1
177. Ginj M, Zhang H, Waser B, Cescato R, Wild D, Wang X, et al. Radiolabeled somatostatin receptor antagonists are preferable to agonists for in vivo peptide receptor targeting of tumors. Proc Natl Acad Sci USA. (2006) 103:16436–41. doi: 10.1073/pnas.0607761103
178. Nicolas GP, Schreiter N, Kaul F, Uiters J, Bouterfa H, Kaufmann J, et al. Sensitivity comparison of 68Ga-OPS202 and 68Ga-DOTATOC PET/CT in patients with gastroenteropancreatic neuroendocrine tumors: a prospective Phase II imaging study. J Nucl Med. (2018) 59:915–21. doi: 10.2967/jnumed.117.199760
179. Carter RE, Feldman AR, Coyle JT. Prostate-specific membrane antigen is a hydrolase with substrate and pharmacologic characteristics of a neuropeptidase. Proc Natl Acad Sci USA. (1996) 93:749–53. doi: 10.1073/pnas.93.2.749
180. Mease RC, Foss CA, Pomper MG. PET imaging in prostate cancer: focus on prostate-specific membrane antigen. Curr Top Med Chem. (2013) 13:951–62. doi: 10.2174/1568026611313080008
181. Maurer T, Eiber M, Schwaiger M, Gschwend JE. Current use of PSMA-PET in prostate cancer management. Nat Rev Urol. (2016) 13:226–35. doi: 10.1038/nrurol.2016.26
182. Ray Banerjee S, Chen Z, Pullambhatla M, Lisok A, Chen J, Mease RC, et al. Preclinical comparative study of 68Ga-Labeled DOTA, NOTA, and HBED-CC chelated radiotracers for targeting PSMA. Bioconjug Chem. (2016) 27:1447–55. doi: 10.1021/acs.bioconjchem.5b00679
183. Eder M, Schäfer M, Bauder-Wüst U, Hull WE, Wängler C, Mier W, et al. 68Ga-complex lipophilicity and the targeting property of a urea-based PSMA inhibitor for PET imaging. Bioconjug Chem. (2012) 23:688–97. doi: 10.1021/bc200279b
184. Eder M, Neels O, Müller M, Bauder-Wüst U, Remde Y, Schäfer M, et al. Novel preclinical and radiopharmaceutical aspects of [68Ga]Ga-PSMA-HBED-CC: a new PET tracer for imaging of prostate cancer. Pharmaceuticals. (2014) 7:779–96. doi: 10.3390/ph7070779
185. Sonni I, Eiber M, Fendler WP, Alano RM, Vangala SS, Kishan AU, et al. Impact of 68Ga-PSMA-11 PET/CT on staging and management of prostate cancer patients in various clinical settings: a prospective single-center study. J Nucl Med. (2020) 61:1153–60. doi: 10.2967/jnumed.119.237602
186. Derlin T, Schmuck S, Juhl C, Teichert S, Zörgiebel J, Wester HJ, et al. Imaging characteristics and first experience of [68Ga]THP-PSMA, a novel probe for rapid kit-based Ga-68 labeling and PET imaging: comparative analysis with [68Ga]PSMA I&T. Mol Imaging Biol. (2018) 20:650–8. doi: 10.1007/s11307-018-1160-8
187. Derlin T, Schmuck S, Juhl C, Zörgiebel J, Schneefeld SM, Walte ACA, et al. PSA-stratified detection rates for [68Ga]THP-PSMA, a novel probe for rapid kit-based 68Ga-labeling and PET imaging, in patients with biochemical recurrence after primary therapy for prostate cancer. Eur J Nucl Med Mol Imaging. (2018) 45:913–22. doi: 10.1007/s00259-017-3924-9
188. Kulkarni M, Hughes S, Mallia A, Gibson V, Young J, Aggarwal A, et al. The management impact of 68gallium-tris(hydroxypyridinone) prostate-specific membrane antigen (68Ga-THP-PSMA) PET-CT imaging for high-risk and biochemically recurrent prostate cancer. Eur J Nucl Med Mol Imaging. (2020) 47:674–86. doi: 10.1007/s00259-019-04643-7
189. Varshney R, Hazari PP, Fernandez P, Schulz J, Allard M, Mishra AK. 68Ga-labeled bombesin analogs for receptor-mediated imaging. Recent Results Cancer Res. (2013) 194:221–56. doi: 10.1007/978-3-642-27994-2_12
190. Sonni I, Baratto L, Iagaru A. Imaging of prostate cancer using Gallium-68-Labeled bombesin. PET Clin. (2017) 12:159–71. doi: 10.1016/j.cpet.2016.11.003
191. Baratto L, Jadvar H, Iagaru A. Prostate cancer theranostics targeting gastrin-releasing peptide receptors. Mol Imaging Biol. (2018) 20:501–9. doi: 10.1007/s11307-017-1151-1
192. Minamimoto R, Hancock S, Schneider B, Chin FT, Jamali M, Loening A, et al. Pilot comparison of 68Ga-RM2 PET and 68Ga-PSMA-11 PET in patients with biochemically recurrent prostate cancer. J Nucl Med. (2016) 57:557–62. doi: 10.2967/jnumed.115.168393
193. Hoberück S, Michler E, Wunderlich G, Löck S, Hölscher T, Froehner M, et al. 68Ga-RM2 PET in PSMA- positive and -negative prostate cancer patients. Nuklearmedizin. (2019) 58:352–62. doi: 10.1055/a-0990-8898
194. Lacoeuille F, Hindré F, Denizot B, Bouchet F, Legras P, Couturier O, et al. New starch-based radiotracer for lung perfusion scintigraphy. Eur J Nucl Med Mol Imaging. (2010) 37:146–55. doi: 10.1007/s00259-009-1226-6
195. Moreno P, Ramos-Alvarez I, Moody TW, Jensen RT. Bombesin related peptides/receptors and their promising therapeutic roles in cancer imaging, targeting and treatment. Expert Opin Ther Targets. (2016) 20:1055–73. doi: 10.1517/14728222.2016.1164694
196. Gruber L, Jiménez-Franco LD, Decristoforo C, Uprimny C, Glatting G, Hohenberger P, et al. MITIGATE-NeoBOMB1, a Phase I/IIa study to evaluate safety, pharmacokinetics, and preliminary imaging of 68Ga-NeoBOMB1, a gastrin-releasing peptide receptor antagonist, in GIST patients. J Nucl Med. (2020) 61:1749–55. doi: 10.2967/jnumed.119.238808
197. Akhtar MS, Qaisar A, Irfanullah J, Iqbal J, Khan B, Jehangir M, et al. Antimicrobial peptide 99mTc-ubiquicidin 29-41 as human infection-imaging agent: clinical trial. J Nucl Med. (2005) 46:567–73.
198. Guleria M, Das T, Amirdhanayagam J, Shinto AS, Kamaleshwaran KK, Pandian A, et al. Convenient formulation of 68Ga-BPAMD patient dose using lyophilized BPAMD kit and 68Ga sourced from different commercial generators for imaging of skeletal metastases. Cancer BiotherRadiopharm. (2019) 34:67–75. doi: 10.1089/cbr.2018.2605
199. Körner M, Christ E, Wild D, Reubi JC. Glucagon-like peptide-1 receptor overexpression in cancer and its impact on clinical applications. Front Endocrinol. (2012) 3:158. doi: 10.3389/fendo.2012.00158
200. Morgat C, Mishra AK, Varshney R, Allard M, Fernandez P, Hindié E. Targeting neuropeptide receptors for cancer imaging and therapy: perspectives with bombesin, neurotensin, and neuropeptide-Y receptors. J Nucl Med. (2014) 55:1650–7. doi: 10.2967/jnumed.114.142000
201. Maschauer S, Prante O. Radiopharmaceuticals for imaging and endoradiotherapy of neurotensin receptor-positive tumors. J Labelled Comp Radiopharm. (2018) 61:309–25. doi: 10.1002/jlcr.3581
202. Wild D, Antwi K, Fani M, Christ ER. Glucagon-like Peptide-1 receptor as emerging target: will it make it to the clinic? J Nucl Med. (2021) 62:44S−50S. doi: 10.2967/jnumed.120.246009
203. Hodolic M, Wu WY, Zhao Z, Yu F, Virgolini I, Wang F. Safety and tolerability of 68Ga-NT-20.3, a radiopharmaceutical for targeting neurotensin receptors, in patients with pancreatic ductal adenocarcinoma: the first in-human use. Eur J Nucl Med Mol Imaging. (2021) 48:1229–34. doi: 10.1007/s00259-020-05045-w
204. Kaeppeli SAM, Schibli R, Mindt TL, Behe M. Comparison of desferrioxamine and NODAGA for the gallium-68 labeling of exendin-4. EJNMMI Radiopharm Chem. (2019) 4:9. doi: 10.1186/s41181-019-0060-9
205. Li L, Zhao R, Hong H, Li G, Zhang Y, Luo Y, et al. 68Ga-labelled-exendin-4: new GLP1R targeting agents for imaging pancreatic β-cell and insulinoma. Nucl Med Biol. (2021) 102-103:87–96. doi: 10.1016/j.nucmedbio.2021.10.001
206. Walenkamp AME, Lapa C, Herrmann K, Wester HJ. CXCR4 ligands: the next big hit? J Nucl Med. (2017) 58:77S−82S. doi: 10.2967/jnumed.116.186874
207. Spreckelmeyer S, Schulze O, Brenner W. Fully-automated production of [68Ga]Ga-PentixaFor on the module Modular Lab-PharmTracer. EJNMMI Radiopharm Chem. (2020) 5:8. doi: 10.1186/s41181-020-0091-2
208. Werner RA, Kircher S, Higuchi T, Kircher M, Schirbel A, Wester HJ, et al. CXCR4-directed imaging in solid tumors. Front Oncol. (2019) 9:770. doi: 10.3389/fonc.2019.00770
209. Kircher M, Tran-Gia J, Kemmer L, Zhang X, Schirbel A, Werner RA, et al. Imaging inflammation in atherosclerosis with CXCR4-Directed 68Ga-Pentixafor PET/CT: correlation with 18F-FDG PET/CT. J Nucl Med. (2020) 61:751–6. doi: 10.2967/jnumed.119.234484
210. Herhaus P, Lipkova J, Lammer F, Yakushev I, Vag T, Slotta-Huspenina J, et al. CXCR4-Targeted PET imaging of central nervous system B-Cell lymphoma. J Nucl Med. (2020) 61:1765–71. doi: 10.2967/jnumed.120.241703
211. Lindner T, Loktev A, Altmann A, Giesel F, Kratochwil C, Debus J, et al. Development of quinoline-based theranostic ligands for the targeting of fibroblast activation protein. J Nucl Med. (2018) 59:1415–22. doi: 10.2967/jnumed.118.210443
212. Imlimthan S, Moon ES, Rathke H, Afshar-Oromieh A, Rösch F, Rominger A, et al. New frontiers in cancer imaging and therapy based on radiolabeled fibroblast activation protein inhibitors: a rational review and current progress. Pharmaceuticals. (2021) 14:1023. doi: 10.3390/ph14101023
213. Giesel FL, Kratochwil C, Lindner T, Marschalek MM, Loktev A, Lehnert W, et al. 68Ga-FAPI PET/CT: biodistribution and preliminary dosimetry estimate of 2 DOTA-Containing FAP-targeting agents in patients with various cancers. J Nucl Med. (2019) 60:386–92. doi: 10.2967/jnumed.118.215913
214. Meyer C, Dahlbom M, Lindner T, Vauclin S, Mona C, Slavik R, et al. Radiation dosimetry and biodistribution of 68Ga-FAPI-46 PET imaging in cancer patients. J Nucl Med. (2020) 61:1171–7. doi: 10.2967/jnumed.119.236786
215. Wang Q, Yang S, Tang W, Liu L, Chen Y. 68Ga-DOTA-FAPI-04 PET/CT as a promising tool for differentiating ovarian physiological uptake: preliminary experience of comparative analysis with 18F-FDG. Front Med. (2021) 8:748683. doi: 10.3389/fmed.2021.748683
216. Giesel FL, Adeberg S, Syed M, Lindner T, Jiménez-Franco LD, Mavriopoulou E, et al. FAPI-74 PET/CT using either 18F-AlF or Cold-Kit 68Ga labeling: biodistribution, radiation dosimetry, and tumor delineation in lung cancer patients. J Nucl Med. (2021) 62:201–7. doi: 10.2967/jnumed.120.245084
217. Moon ES, Elvas F, Vliegen G, De Lombaerde S, Vangestel C, De Bruycker S, et al. Targeting fibroblast activation protein (FAP): next generation PET radiotracers using squaramide coupled bifunctional DOTA and DATA5m chelators. EJNMMI Radiopharm Chem. (2020) 5:19. doi: 10.1186/s41181-020-00102-z
Keywords: cold kit, gallium-68, molecular imaging, positron emission tomography (PET), radiolabeling, radiopharmaceuticals
Citation: Lepareur N (2022) Cold Kit Labeling: The Future of 68Ga Radiopharmaceuticals? Front. Med. 9:812050. doi: 10.3389/fmed.2022.812050
Received: 09 November 2021; Accepted: 07 January 2022;
Published: 10 February 2022.
Edited by:
Francesco Cicone, University of Catanzaro, ItalyReviewed by:
Ismaheel Lawal, University of Pretoria, South AfricaLucia Baratto, Stanford University, United States
Renata Mikolajczak, Radioisotope Centre POLATOM, National Centre for Nuclear Research, Poland
Copyright © 2022 Lepareur. This is an open-access article distributed under the terms of the Creative Commons Attribution License (CC BY). The use, distribution or reproduction in other forums is permitted, provided the original author(s) and the copyright owner(s) are credited and that the original publication in this journal is cited, in accordance with accepted academic practice. No use, distribution or reproduction is permitted which does not comply with these terms.
*Correspondence: Nicolas Lepareur, bi5sZXBhcmV1ckByZW5uZXMudW5pY2FuY2VyLmZy