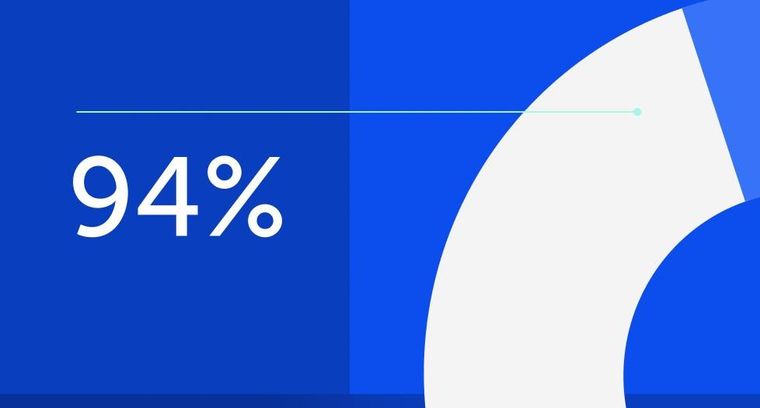
94% of researchers rate our articles as excellent or good
Learn more about the work of our research integrity team to safeguard the quality of each article we publish.
Find out more
MINI REVIEW article
Front. Med., 09 January 2023
Sec. Nuclear Medicine
Volume 9 - 2022 | https://doi.org/10.3389/fmed.2022.1071086
This article is part of the Research TopicTargeted Alpha Particle Therapy in OncologyView all 17 articles
Targeted alpha therapy (TAT) is a promising approach for addressing unmet needs in oncology. Inherent properties make α-emitting radionuclides well suited to cancer therapy, including high linear energy transfer (LET), penetration range of 2–10 cell layers, induction of complex double-stranded DNA breaks, and immune-stimulatory effects. Several alpha radionuclides, including radium-223 (223Ra), actinium-225 (225Ac), and thorium-227 (227Th), have been investigated. Conjugation of tumor targeting modalities, such as antibodies and small molecules, with a chelator moiety and subsequent radiolabeling with α-emitters enables specific delivery of cytotoxic payloads to different tumor types. 223Ra dichloride, approved for the treatment of patients with metastatic castration-resistant prostate cancer (mCRPC) with bone-metastatic disease and no visceral metastasis, is the only approved and commercialized alpha therapy. However, 223Ra dichloride cannot currently be complexed to targeting moieties. In contrast to 223Ra, 227Th may be readily chelated, which allows radiolabeling of tumor targeting moieties to produce targeted thorium conjugates (TTCs), facilitating delivery to a broad range of tumors. TTCs have shown promise in pre-clinical studies across a range of tumor-cell expressing antigens. A clinical study in hematological malignancy targeting CD22 has demonstrated early signs of activity. Furthermore, pre-clinical studies show additive or synergistic effects when TTCs are combined with established anti-cancer therapies, for example androgen receptor inhibitors (ARI), DNA damage response inhibitors such as poly (ADP)-ribose polymerase inhibitors or ataxia telangiectasia and Rad3-related kinase inhibitors, as well as immune checkpoint inhibitors.
Despite drug discovery advances, an unmet clinical need for novel oncology treatment modalities persists. Targeted alpha therapy (TAT) represents one such modality, as α-particles have several properties of potential value in cancer therapy. These include high linear energy transfer (LET), short penetration range, and induction of complex double-stranded DNA breaks (1). High LET means a low number of hits are needed to induce cell death (1), while the short-path length of α-particles (50–100 μm) is expected to minimize damage to surrounding healthy tissue (1). Furthermore, complex double-stranded DNA breaks induced by alpha-radiation are hard to repair, promoting cell cycle arrest and cell death (1, 2). TATs may also promote T-cell infiltration through induction of immunogenic cell death (3–6), or have increased potency against tumor cells with alterations in DNA damage repair genes (cytotoxic radiation-induced DNA damage increases their susceptibility to apoptosis) (7–9).
Selective tumor targeting by TATs can be achieved through two primary mechanisms: inherent radionuclide properties (1) and the ability to chelate the radionuclide to a tumor-targeting molecule (e.g., a monoclonal antibody, peptide or small molecule) (1). Over the last 20 years, several α-particle-emitting radionuclides have been investigated as TATs, including: actinium-225 (225Ac, half-life 9.9 days); astatine-211 (211At, half-life 7.2 h); bismuth-213 (213Bi, half-life 45.6 min); radium-223 (223Ra, half-life 11.4 days); and thorium-227 (227Th, half-life 18.7 days) (1). Lead-212 (212Pb, half-life 10.64 h) is a β-emitter; however, it generates the daughter nuclides bismuth-212 (212Bi) and polonium-212 (212Po), which are short-lived α-particle emitters (10). 223Ra dichloride was the first and is still the only approved TAT (11, 12), and is approved for use in metastatic castration-resistant prostate cancer (mCRPC) with bone metastases (13, 14). 223Ra dichloride acts as a calcium mimetic and is preferentially taken up in osteoblastic bone metastases (15, 16); it cannot currently be complexed to targeting moieties, although recent developments have shown promise (14, 17). Most other TATs, like targeted thorium conjugates (TTCs) or targeted actinium conjugates, use isotopes chelated to various targeting moieties. This enables delivery to a wide range of tumors (14), extending the clinical application of radionuclides.
227Th, the progenitor of 223Ra, can be used in TTCs, comprised of the 227Th α-emitting radionuclide, a chelator such as octadentate 3,2-hydroxypyridinone (3,2-HOPO), and a tumor-targeting moiety (13, 14). TTCs enable selective delivery of 227Th to tumors by targeting antigens expressed in cancer tissues but absent or at low levels in normal tissues (2). For a therapeutic window, TTC characteristics must allow for efficient delivery, accumulation and retention in tumors, while sparing nearby healthy tissue (14). Cytotoxicity results from the induction of clustered double-stranded DNA breaks, followed by subsequent G2/M phase cell cycle arrest and apoptosis (14). Immunogenic cell death has also been demonstrated, occurring via increased tumor infiltration by CD8+ T cells (5, 14). The activity of TTCs is not reliant on cellular internalization of 227Th, given the α-particle path length of 20–100 μM (2–10 cell diameters) in tissue, a property which may overcome heterogeneous antigen expression (14).
The relatively long half-life of 227Th (18.7 days) compared with other radionuclides in current use for TAT (1) highlights the need to identify appropriate targeting moieties that complement the properties of 227Th. For example, while typically longer than that of small molecules, the half-lives of antibodies used as therapeutic agents vary considerably (6–32 days) (17–19), suggesting that some may not be suitable for delivery of a radionuclide with a longer half-life. For TTCs, while it may be preferable to select antibodies with comparable half-lives to 227Th, data are not yet available as to whether this would be necessary for therapeutic efficacy.
When the 227Th component of a TTC decays, recoil energy releases the daughter radionuclide 223Ra from the chelator (14). Whilst data on the safety and biodistribution of 223Ra released from TTCs are not available, 223Ra is well tolerated when it is used as a treatment (20) and it is rapidly cleared from plasma into the small bowel and excreted (21). Furthermore, the amount of 223Ra released from a TTC will be much smaller than that of a therapeutic dose of 223Ra. Daughter radionuclides of 227Th that lie downstream of 223Ra in the decay cascade have very short half-lives (14) and have no clinical consequence, as indicated by the good tolerability of 223Ra as a cancer therapeutic (20).
Pre-clinical and clinical studies of TTCs have included several tumor types expressing a range of different cancer-related antigens (Figure 1).
Figure 1. Timeline of TTC development. ARI, androgen receptor inhibitor; CD, cluster of differentiation; FGFR, fibroblast growth factor receptor; HER2, human epidermal growth factor receptor-2; MSLN, mesothelin; PARP, poly (ADP)-ribose polymerase; PD-L1, programmed death-ligand 1; PDX, patient-derived xenograft; PSMA, prostate-specific membrane antigen; TTC, targeted thorium conjugate; AML, acute myeloid leukemia; ATR, ataxia telangiectasia and rad3-related protein; mCRPC, metastatic castration-resistant prostate cancer; ORR, overall response rate; TNBC, triple-negative breast cancer; TEAE, treatment-emergent adverse event.
Initial Pre-clinical studies focusing on hematological cancers, targeting CD22 or CD33 in lymphoma and acute myeloid leukemia (AML), respectively, demonstrated promising anti-tumor activity (14, 22). Furthermore, CD22-TTC (BAY 1862864) has been investigated in a Phase 1 study in patients with CD22-positive relapsed/refractory B-cell non-Hodgkin lymphoma (23). In this setting, CD22-TTC was safe, with the most common grade ≥3 adverse events being neutropenia, thrombocytopenia, and leukopenia (23). Maximum 227Th blood concentrations increased proportionally to the dose administered and stability of CD22-TTC in the blood was demonstrated (23). The overall objective response rate (ORR) was 24% (5/21 patients: 1 complete and 4 partial responses), with the highest ORR seen in patients with relapsed low-grade lymphomas [3/10 patients (30%)] (23).
CD27, part of the tumor necrosis factor receptor superfamily, plays a vital role in T- and B-cell co-stimulation (24). Physiological expression of CD70, the natural ligand of CD27, is transient and restricted to activated immune cells (24). However, CD70 dysregulation and overexpression has been observed in several cancers (25–28), where it may play a role in tumor progression and immunosuppression (29). Therefore, CD70-TTCs have the potential to both eliminate cancer cells and modulate immune responses. A CD70-TTC has been shown to reduce cell viability in renal cancer cell lines and significantly inhibit tumor growth in a renal cancer xenograft model (25).
Approximately 25–30% of breast cancers overexpress human epidermal growth factor receptor-2 (HER2), which is associated with more aggressive disease (30). Intrinsic and acquired resistance to HER2-targeting antibodies or antibody drug conjugates (ADC) necessitates development of novel therapies (31, 32). A HER2-TTC, utilizing the HER2 antibody trastuzumab (227Th-trastuzumab), showed significant dose-dependent anti-tumor effects in HER2-expressing breast cancer xenografts (33, 34). Moreover, when 227Th-trastuzumab was compared with lutetium-177 (177Lu; a β-particle emitter) complexed with trastuzumab, in a similar xenograft study, each radionuclide conjugate had significant anti-tumor effects and increased survival, although efficacy was higher with 227Th-trastuzumab than with 177Lu-trastuzumab. However, 177Lu-trastuzumab had a superior therapeutic index (34). Additionally, clinically relevant concentrations of 227Th-trastuzumab induced cytotoxic effects in HER2-expressing breast cancer cell lines (35).
Initial HER2-targeted agents were ineffective against HER2-low breast cancer (36). However, the ADC trastuzumab deruxtecan recently demonstrated efficacy in this setting (37). Notably, HER2-TTC has been shown to inhibit tumor growth in HER2-low colorectal cancer (CRC) xenografts (9), highlighting its potential as an alternative treatment option for HER2-low cancers. Furthermore, a Phase I trial of a HER2-TTC is ongoing in advanced HER2-expressing cancers: HER2-high and low expression in breast, gastric/gastroesophageal and other tumors (38).
Fibroblast growth factor receptor 2 (FGFR2) is also a promising target for TTCs, with amplifications in FGFR2 observed in a subset of triple-negative breast cancers (TNBCs) (39–41). Elevated FGFR2 is associated with an aggressive cancer phenotype and resistance to targeted therapy (39, 42), making FGFR2-TTCs an attractive therapeutic option. Indeed, in a human TNBC xenograft model, single-dose FGFR2-TTC reduced tumor growth and was well tolerated (43).
HER2 is overexpressed in over 20% of all gastric cancers and is a valid therapeutic target in this setting (44, 45). HER2-TTC was associated with potent target-mediated cytotoxicity in various cancer cell lines, including gastric cancer cell lines, expressing different levels of HER2 (46).
FGFR2 is also a potential target for TTCs, with some gastric cancers overexpressing the protein (47, 48). In gastric cancer xenograft models, tumor growth was inhibited after a single dose of FGFR2-TTC (48).
Next-generation sequencing identified FGFR2 aberrations in a subset (1.4%) of patients with CRC (49) and FGFR2 expression has been seen in 2.9% of patients with CRC (50), indicating some patients may benefit from therapeutic targeting of this protein. In support of this, single-dose FGFR2-TTC inhibited tumor growth in a xenograft model of CRC (48).
HER2-TTC has also been evaluated in CRC models in combination with a poly (ADP)-ribose polymerase (PARP) inhibitor, which is discussed later in this review (9).
Mesothelioma is a rare malignant growth of mesothelial cells, occurring in lining layers of the viscera, e.g., pleura, peritoneum and pericardium (51). Mesothelin (MSLN) mediates cellular adhesion and is normally only expressed in mesothelial cells; however, when dysregulated in cancer, MSLN promotes proliferation, migration and invasion, making it an attractive target for TTC-based therapy (52–55). MSLN-TTC has shown potent cytotoxic effects in MSLN-positive cancer cell lines (including mesothelioma) and, when used in single- or multiple-dose regimens in cell line- and patient-derived xenograft models, the conjugate had significant anti-tumor activity and was well tolerated (56). Furthermore, MSLN-TTC prolonged survival in a disseminated lung cancer model in mice (56).
A first-in-human Phase I study of MSLN-TTC in patients with advanced cancer (mesothelioma, as well as MSLN-positive recurrent serous ovarian cancer and pancreatic adenocarcinoma) was completed in the first half of 2022 (57); results are being analyzed for future publication.
Mesothelin-targeted thorium conjugate has been investigated in MSLN-positive ovarian cancer models, with significant anti-tumor activity seen when MSLN-TTC was used in single-dose regimens in cell line-derived xenografts and single- and multiple-dose regimens in patient-derived xenografts (56). Data from the aforementioned first-in-human study of MSLN-TTC in patients with advanced cancer, including ovarian cancer, are awaited with interest.
Pre-clinical studies have also explored the potential for HER2-TTCs in HER2-positive forms. 227Th-trastuzumab demonstrated cytotoxic effects in HER2-expressing ovarian cancer cell lines when used at clinically relevant concentrations (35). Furthermore, in HER2-positive ovarian cancer xenograft models, 227Th-trastuzumab delayed tumor growth and was associated with survival benefit vs. unlabeled trastuzumab (58, 59) or 177Lu-trastuzumab (at the same absorbed radiation dose to tumor) (59). Notably, fractionation of 227Th-trastuzumab dosing in xenograft models reduced toxicity while retaining efficacy, showing that administration schedule is an important consideration for TTCs (60).
A TTC targeting prostate-specific membrane antigen (PSMA) has been developed. In vitro, the antibody-based PSMA-TTC was rapidly internalized in a target-dependent manner, selectively reduced PSMA-expressing cell viability, and induced double-stranded DNA breaks, cell cycle arrest (G2/M phase), and apoptosis in prostate cancer cells (61). Consistent with this, induction of DNA damage markers and apoptosis was observed with PSMA-TTC in patient-derived xenografts in mice (61). Further in vivo data showed PSMA-TTC was associated with delayed tumor growth/tumor regression in PSMA-positive patient- and cell line-derived xenograft models mimicking different prostate cancer stages, including models resistant to standard-of-care anti-androgens (including enzalutamide) (61). This effect was seen with single as well as fractionated dosing (61). In a mouse model replicating prostate cancer bone metastases, PSMA-TTC significantly reduced the growth of tumors in the bone and was associated with changes in tumor-induced bone morphology vs. controls (61).
A Phase I clinical study of PSMA-TTC, either alone or in combination with the novel androgen receptor inhibitor (ARI) darolutamide, in patients with mCRPC is currently ongoing; the primary completion date was August 2022, the estimated completion date is November 2023 (62).
Due to the unique mode of action of TTCs, there is a strong rationale for combining these with other cancer therapies, and this has been investigated in several pre-clinical studies.
As TTCs induce complex double-stranded DNA breaks (1), it is of interest to combine their use with PARP inhibitors, as PARP-1 and PARP-2 are involved in DNA damage repair (63, 64). BRCA mutations have been shown to sensitize cells to PARP inhibition (65, 66), as BRCA proteins are crucial for the repair of double-stranded DNA breaks (63). Indeed, in a BRCA2-mutated prostate cancer xenograft model, PSMA-TTC plus the PARP inhibitor olaparib showed more notable anti-tumor activity than PSMA-TTC alone, while olaparib alone showed no activity (67).
Additionally, HER2-TTC has been investigated in parental and BRCA2 knockout HER2-expressing CRC cell lines and their corresponding xenograft models (9). In cell viability assays, the effect of HER2-TTC plus olaparib was synergistic in BRCA2 knockout cells vs. additive in parental cells (9). Similarly, when combined with olaparib in BRCA2-deficient xenografts, low-dose HER2-TTC resulted in similar tumor growth inhibition to high-dose HER2-TTC alone, with the combination concluded as being synergistic; by contrast, no synergistic effects were seen with the combination in the parental xenograft model (9). These findings support further evaluation of PARP inhibitors in combination with TTCs.
Another protein involved in double-stranded DNA break repair is DNA-dependent protein kinase (DNA-PK), which plays a key role in non-homologous end joining (NHEJ) (68). Loss of DNA-PK makes cells more susceptible to radiation, as NHEJ is important for the repair of DNA double-strand breaks that are induced by ionizing radiation (68). Combining PSMA-TTC with a DNA-PK inhibitor resulted in synergistic anti-proliferative effects in prostate cancer cells (69). The combination was also more effective than PSMA-TCC monotherapy in prostate tumor-bearing mice (69), indicating the clinical potential for this combination.
FGFR2-TTC has been investigated in combination with an inhibitor of the ataxia telangiectasia and rad3-related protein (ATR), an enzyme involved in DNA damage response (43, 70–72). In vitro, the combination of FGFR2-TTC plus ATR inhibitor reduced cell viability and increased levels of γH2A.X (an indicator of double-strand DNA breaks) vs. FGFR2-TTC alone, while also reducing FGFR2-TTC-mediated cell cycle arrest (43). In vivo, tumor growth was significantly inhibited when the two agents were used in combination at single-agent doses known to have no effect (43). Data from ovarian cancer models studying the MSLN-TTC plus ATR inhibitor combination support these findings (7).
Immunostimulatory effects have been shown with radiation, including external beam radiotherapy and α-particle emitters, with the former showing anti-tumor effects when combined with immune checkpoint inhibitors (4, 73–76). These data provide rationale for combining a TTC with an immune checkpoint inhibitor, such as programmed death ligand-1 (PD-L1). MSLN-TTC demonstrated a robust immunostimulatory effect in human cancer cell lines (5). Moreover, in immunocompetent mice bearing implanted murine tumors expressing human MSLN, tumor growth was inhibited by MSLN-TTC and anti-PD-L1 individually, with this benefit enhanced when these agents were used in combination (5). Dendritic cell migration out of tumors and CD8+ T-cell infiltration into tumors was observed when MSLN-TTC was administered as monotherapy, with more extensive T-cell infiltration seen when MSLN-TTC was combined with anti-PD-L1 (5).
Although ARIs are a common treatment option for patients with prostate cancer, treatment resistance eventually develops (77). This highlights the need for new therapeutic approaches, such as novel combination treatments or new agents with different mechanisms of action, to overcome this therapeutic barrier.
The ARI darolutamide is approved for non-metastatic CRPC in key markets (78, 79) and more recently for use in combination with docetaxel for metastatic hormone-sensitive prostate cancer in the United States (79). Darolutamide has been shown to induce PSMA expression in prostate cancer cell lines and xenografts (80, 81), providing a rationale for combining the drug with a PSMA-TTC. In prostate cancer xenograft models, darolutamide-mediated increase of PSMA expression facilitated tumor uptake of PSMA-TTC, and darolutamide also impaired PSMA-TTC-mediated induction of DNA damage repair genes (80). Furthermore, the combination of PSMA-TTC plus darolutamide demonstrated synergistic inhibition of tumor growth in xenograft models (80). The tumor inhibitory activity of the combination was also more notable than either agent alone in xenograft models that were either resistant to the ARI enzalutamide (80) or hormone independent (81). These results support clinical investigation of this combination.
227Th is one of a number of α-emitters suitable for chelation and conjugation to tumor-targeting moieties and thus has the potential to cover a broad tumor range. Indeed, pre-clinical studies have shown anti-tumor activity of TTCs as monotherapy across a broad range of tumor types, and TTCs targeting HER2, PSMA, MSLN, and CD22 are under investigation in clinical studies. Furthermore, there is a strong rationale and pre-clinical evidence for combining TTCs with other targeted therapies, supporting their clinical evaluation. However, no additional TTC clinical trials are currently planned.
In addition to 227Th, various other α-emitters are being explored as conjugates for the treatment of cancer. Those considered to be the most suitable include 225Ac, 211At, 213Bi, and 212Pb (the latter being a β-emitter that generates daughter α-emitters) (1, 82), with the most clinical experience being available for 225Ac and 213Bi (83–90).
The clinical potential of targeted radionuclide therapy is further highlighted by the recent US approval of 177Lu-PSMA-617 (a β-emitter conjugated to a small molecule PSMA ligand) for the treatment of mCRPC (91–93). Moreover, promising early clinical data has indicated that targeting PSMA with 225Ac via a small molecule (84, 94, 95) or an antibody (96) has substantial potential in advanced prostate cancer, including for patients who have received radiotherapeutics utilizing 177Lu (97), and suggests feasibility of using different targeted radionuclides sequentially.
In summary, TATs represent an important therapeutic development in oncology and offer promise for addressing unmet medical needs for patients, such as resistance to established therapies.
VW, HH, VJ, and UBH contributed to the conception and design. JK, CAS, AMW, SH, AS, AC, VW, HH, VJ, and UBH contributed to the drafting and revising of the work, and approval of the final version. All authors agreed to be accountable for all aspects of the respective work.
The authors thank Katrine Wickstroem Biseth, Olav Ryan, Roger M. Bjerke, Ingrid Moen, Veronique Cruciani, Baard Indrevoll, Steinar Uran, Roger Smeets, Thanushan Rajanayagam, Liv-Ingrid Oedegardstuen, Aasmund Larsen, Anne Mobergslien, and Alex Papple (all Bayer AS, Norway) as well as Thorsten Poethko, Sandra Johanssen, Mark Trautwein, Christoph Mahlert, Lars Linden, Sabine Zitzmann-Kolbe, Volker Stickel, Martin Kohs, Melanie Appel, Maria Spelling, Seren Nesan, Meike Fehder, Sarah Boettcher, Lisa Bartnitzky, Kathleen Stadelmann, Stefan Stargard, Jochen Hilbig, Monika Klotz, Claudia Kamfenkel, Stefanie Mai, Tobias Heinrich, and Vu Tung Le (all Bayer AG) for their contributions to data included in this review. Cancer Communications and Consultancy Ltd., Cheshire, United Kingdom, provided medical writing assistance (funded by Bayer). Dr. Lila Adnane (Bayer) provided editorial assistance.
JK, CAS, AMW, SH, AS, AC, VW, HH, VJ, and UBH were employed by Bayer.
All claims expressed in this article are solely those of the authors and do not necessarily represent those of their affiliated organizations, or those of the publisher, the editors and the reviewers. Any product that may be evaluated in this article, or claim that may be made by its manufacturer, is not guaranteed or endorsed by the publisher.
1. Parker C, Lewington V, Shore N, Kratochwil C, Levy M, Lindén O, et al. Targeted alpha therapy, an emerging class of cancer agents: a review. JAMA Oncol. (2018) 4:1765–72. doi: 10.1001/jamaoncol.2018.4044
2. Tafreshi N, Doligalski M, Tichacek C, Pandya D, Budzevich M, El-Haddad G, et al. Development of targeted alpha particle therapy for solid tumors. Molecules. (2019) 24:4314. doi: 10.3390/molecules24234314
3. Ménager J, Gorin J, Maurel C, Drujont L, Gouard S, Louvet C, et al. Combining α-radioimmunotherapy and adoptive T cell therapy to potentiate tumor destruction. PLoS One. (2015) 10:e0130249. doi: 10.1371/journal.pone.0130249
4. Malamas A, Gameiro S, Knudson K, Hodge J. Sublethal exposure to alpha radiation (223ra dichloride) enhances various carcinomas’ sensitivity to lysis by antigen-specific cytotoxic T lymphocytes through calreticulin-mediated immunogenic modulation. Oncotarget. (2016) 7:86937–47. doi: 10.18632/oncotarget.13520
5. Lejeune P, Cruciani V, Berg-Larsen A, Schlicker A, Mobergslien A, Bartnitzky L, et al. Immunostimulatory effects of targeted thorium-227 conjugates as single agent and in combination with anti-Pd-L1 therapy. J Immunother Cancer. (2021) 9:e002387. doi: 10.1136/jitc-2021-002387
6. Patel R, Hernandez R, Carlson P, Grudzinski J, Bates A, Jagodinsky J, et al. Low-dose targeted radionuclide therapy renders immunologically cold tumors responsive to immune checkpoint blockade. Sci Transl Med. (2021) 13:eabb3631. doi: 10.1126/scitranslmed.abb3631
7. Wickstroem K, Hagemann U, Cruciani V, Wengner A, Kristian A, Ellingsen C, et al. Synergistic effect of a mesothelin-targeted (227)Th conjugate in combination with DNA damage response inhibitors in ovarian cancer xenograft models. J Nucl Med. (2019) 60:1293–300. doi: 10.2967/jnumed.118.223701
8. van der Doelen M, Isaacsson Velho P, Slootbeek P, Pamidimarri Naga S, Bormann M, van Helvert S, et al. Impact of DNA damage repair defects on response to radium-223 and overall survival in metastatic castration-resistant prostate cancer. Eur J Cancer. (2020) 136:16–24. doi: 10.1016/j.ejca.2020.05.001
9. Wickstroem K, Karlsson J, Ellingsen C, Cruciani V, Kristian A, Hagemann U, et al. Synergistic effect of a HER2 targeted thorium-227 conjugate in combination with olaparib in a BRCA2 deficient xenograft model. Pharmaceuticals. (2019) 12:155. doi: 10.3390/ph12040155
10. Kokov K, Egorova B, German M, Klabukov I, Krasheninnikov M, Larkin-Kondrov A, et al. 212Pb: production approaches and targeted therapy applications. Pharmaceutics. (2022) 14:189. doi: 10.3390/pharmaceutics14010189
11. EMC. Xofigo Summary of Product Characteristics. (n.d.). Available online at: https://www.medicines.org.uk/emc/product/5204/smpc (Accessed September 20, 2022).
12. Xofigo. Xofigo Prescribing Information. (n.d.). Available online at: https://www.Xofigo-us.com/sites/G/files/vrxlpx3306/Files/2020-09/Xofigo_Pi.Pdf (Accessed September 20, 2022).
13. Ramdahl T, Bonge-Hansen H, Ryan O, Larsen S, Herstad G, Sandberg M, et al. An efficient chelator for complexation of thorium-227. Bioorg Med Chem Lett. (2016) 26:4318–21. doi: 10.1016/j.bmcl.2016.07.034
14. Hagemann U, Wickstroem K, Hammer S, Bjerke R, Zitzmann-Kolbe S, Ryan O, et al. Advances in precision oncology: targeted thorium-227 conjugates as a new modality in targeted alpha therapy. Cancer Biother Radiopharm. (2020) 35:497–510. doi: 10.1089/cbr.2020.3568
15. Suominen M, Wilson T, Käkönen S, Scholz A. The mode-of-action of targeted alpha therapy radium-223 as an enabler for novel combinations to treat patients with bone metastasis. Int J Mol Sci. (2019) 20:3899. doi: 10.3390/ijms20163899
16. Suominen M, Fagerlund K, Rissanen J, Konkol Y, Morko J, Peng Z, et al. Radium-223 inhibits osseous prostate cancer growth by dual targeting of cancer cells and bone microenvironment in mouse models. Clin Cancer Res. (2017) 23:4335–46. doi: 10.1158/1078-0432.CCR-16-2955
17. Grevys A, Frick R, Mester S, Flem-Karlsen K, Nilsen J, Foss S, et al. Antibody variable sequences have a pronounced effect on cellular transport and plasma half-life. iScience. (2022) 25:103746. doi: 10.1016/j.isci.2022.103746
18. Castelli M, McGonigle P, Hornby P. The pharmacology and therapeutic applications of monoclonal antibodies. Pharmacol Res Perspect. (2019) 7:e00535. doi: 10.1002/prp2.535
19. Ovacik M, Lin K. Tutorial on monoclonal antibody pharmacokinetics and its considerations in early development. Clin Transl Sci. (2018) 11:540–52. doi: 10.1111/cts.12567
20. Parker C, Coleman R, Sartor O, Vogelzang N, Bottomley D, Heinrich D, et al. Three-year safety of radium-223 dichloride in patients with castration-resistant prostate cancer and symptomatic bone metastases from phase 3 randomized alpharadin in symptomatic prostate cancer trial. Eur Urol. (2018) 73:427–35. doi: 10.1016/j.eururo.2017.06.021
21. Carrasquillo J, O’Donoghue J, Pandit-Taskar N, Humm J, Rathkopf D, Slovin S, et al. Phase I pharmacokinetic and biodistribution study with escalating doses of 223Ra-dichloride in men with castration-resistant metastatic prostate cancer. Eur J Nucl Med Mol Imaging. (2013) 40:1384–93. doi: 10.1007/s00259-013-2427-6
22. Hagemann U, Wickstroem K, Wang E, Shea A, Sponheim K, Karlsson J, et al. In vitro and in vivo efficacy of a novel CD33-targeted thorium-227 conjugate for the treatment of acute myeloid leukemia. Mol Cancer Ther. (2016) 15:2422–31. doi: 10.1158/1535-7163.MCT-16-0251
23. Linden O, Bates A, Cunningham D, Hindorf C, Larsson E, Cleton A, et al. 227Th-labeled anti-CD22 antibody (BAY 1862864) in relapsed/refractory CD22-positive non-hodgkin lymphoma: a first-in-human, phase I study. Cancer Biother Radiopharm. (2021) 36:672–81. doi: 10.1089/cbr.2020.4653
24. Starzer A, Berghoff A. New emerging targets in cancer immunotherapy: CD27 (TNFRSF7). ESMO Open. (2020) 4(Suppl. 3):e000629. doi: 10.1136/esmoopen-2019-000629
25. Hagemann U, Mihaylova D, Uran S, Borrebaek J, Grant D, Bjerke R, et al. Targeted alpha therapy using a novel CD70 targeted thorium-227 conjugate in in vitro and in vivo models of renal cell carcinoma. Oncotarget. (2017) 8:56311–26. doi: 10.18632/oncotarget.16910
26. Lens S, Drillenburg P, den Drijver B, van Schijndel G, Pals S, van Lier R, et al. Aberrant expression and reverse signalling of CD70 on malignant B cells. Br J Haematol. (1999) 106:491–503. doi: 10.1046/j.1365-2141.1999.01573.x
27. Diegmann J, Junker K, Gerstmayer B, Bosio A, Hindermann W, Rosenhahn J, et al. Identification of CD70 as a diagnostic biomarker for clear cell renal cell carcinoma by gene expression profiling, real-time RT-PCR and immunohistochemistry. Eur J Cancer. (2005) 41:1794–801. doi: 10.1016/j.ejca.2005.05.005
28. Wischhusen J, Jung G, Radovanovic I, Beier C, Steinbach J, Rimner A, et al. Identification of CD70-mediated apoptosis of immune effector cells as a novel immune escape pathway of human glioblastoma. Cancer Res. (2002) 62:2592–9.
29. Flieswasser T, Van den Eynde A, Van Audenaerde J, De Waele J, Lardon F, Riether C, et al. The CD70-CD27 axis in oncology: the new kids on the block. J Exp Clin Cancer Res. (2022) 41:12. doi: 10.1186/s13046-021-02215-y
30. Slamon D, Leyland-Jones B, Shak S, Fuchs H, Paton V, Bajamonde A, et al. Use of chemotherapy plus a monoclonal antibody against HER2 for metastatic breast cancer that overexpresses HER2. N Engl J Med. (2001) 344:783–92. doi: 10.1056/NEJM200103153441101
31. Pohlmann P, Mayer I, Mernaugh R. Resistance to trastuzumab in breast cancer. Clin Cancer Res. (2009) 15:7479–91. doi: 10.1158/1078-0432.Ccr-09-0636
32. Hunter F, Barker H, Lipert B, Rothé F, Gebhart G, Piccart-Gebhart M, et al. Mechanisms of resistance to trastuzumab emtansine (T-DM1) in HER2-positive breast cancer. Br J Cancer. (2020) 122:603–12. doi: 10.1038/s41416-019-0635-y
33. Abbas N, Heyerdahl H, Bruland O, Borrebæk J, Nesland J, Dahle J. Experimental α-Particle radioimmunotherapy of breast cancer using 227Th-labeled p-benzyl-DOTA-trastuzumab. EJNMMI Res. (2011) 1:18. doi: 10.1186/2191-219x-1-18
34. Abbas N, Heyerdahl H, Bruland ØS, Brevik E, Dahle J. Comparing high LET 227Th- and low LET 177Lu-trastuzumab in mice with HER-2 positive SKBR-3 xenografts. Curr Radiopharm. (2013) 6:78–86. doi: 10.2174/18744710113069990017
35. Heyerdahl H, Krogh C, Borrebæk J, Larsen Å, Dahle J. Treatment of HER2-expressing breast cancer and ovarian cancer cells with alpha particle-emitting 227Th-trastuzumab. Int J Radiat Oncol Biol Phys. (2011) 79:563–70. doi: 10.1016/j.ijrobp.2010.08.038
36. Lai H, Han J, Fu X, Ren Y, Li Z, You F. Targeted approaches to HER2-low breast cancer: current practice and future directions. Cancers. (2022) 14:3774. doi: 10.3390/cancers14153774
37. Modi S, Jacot W, Yamashita T, Sohn J, Vidal M, Tokunaga E, et al. Trastuzumab deruxtecan in previously treated HER2-low advanced breast cancer. N Engl J Med. (2022) 387:9–20. doi: 10.1056/NEJMoa2203690
38. Clinicaltrials.Gov. A first in human study of BAY2701439 to look at safety, how the body absorbs, distributes and excretes the drug, and how well the drug works in participants with advanced cancer expressing the HER2 Protein. ClinicalTrials.gov identifier NCT04147819. (2019). Available online at: https://clinicaltrials.gov/ct2/show/NCT04147819 (Accessed September 2, 2022).
39. Lei J, Lee M, Miao K, Huang Z, Yao Z, Zhang A, et al. Activation of FGFR2 signaling suppresses BRCA1 and drives triple-negative mammary tumorigenesis that is sensitive to immunotherapy. Adv Sci. (2021) 8:e2100974. doi: 10.1002/advs.202100974
40. Du J, Zhao Q, Liu K, Li Z, Fu F, Zhang K, et al. FGFR2/STAT3 signaling pathway involves in the development of MMTV-related spontaneous breast cancer in TA2 mice. Front Oncol. (2020) 10:652. doi: 10.3389/fonc.2020.00652
41. Turner N, Lambros M, Horlings H, Pearson A, Sharpe R, Natrajan R, et al. Integrative molecular profiling of triple negative breast cancers identifies amplicon drivers and potential therapeutic targets. Oncogene. (2010) 29:2013–23. doi: 10.1038/onc.2009.489
42. Fernández-Nogueira P, Mancino M, Fuster G, López-Plana A, Jauregui P, Almendro V, et al. Tumor-associated fibroblasts promote HER2-targeted therapy resistance through FGFR2 activation. Clin Cancer Res. (2020) 26:1432–48. doi: 10.1158/1078-0432.Ccr-19-0353
43. Wickstroem K, Hagemann U, Kristian A, Ellingsen C, Sommer A, Ellinger-Ziegelbauer H, et al. Preclinical combination studies of an FGFR2 targeted thorium-227 conjugate and the ATR inhibitor BAY 1895344. Int J Radiat Oncol Biol Phys. (2019) 105:410–22. doi: 10.1016/j.ijrobp.2019.06.2508
44. Fong C, Chau I. HER2 inhibition in gastric cancer-novel therapeutic approaches for an established target. Cancers. (2022) 14:3824. doi: 10.3390/cancers14153824
45. Van Cutsem E, Bang Y, Feng-Yi F, Xu J, Lee K, Jiao S, et al. HER2 screening data from ToGA: targeting HER2 in gastric and gastroesophageal junction cancer. Gastric Cancer. (2015) 18:476–84. doi: 10.1007/s10120-014-0402-y
46. Karlsson J, Hagemann U, Schatz C, Grant D, Kristian A, Ellingsen C, et al. Abstract 5859: HER2-targeted thorium-227 conjugate (HER2-TTC): efficacy in preclinical models of trastuzumab and T-DM1 resistance. Cancer Res. (2017) 77(Suppl. 13):5859. doi: 10.1158/1538-7445.AM2017-5859
47. Carter J, Cottrell C, McNulty S, Vigh-Conrad K, Lamp S, Heusel J, et al. FGFR2 amplification in colorectal adenocarcinoma. Cold Spring Harb Mol Case Stud. (2017) 3:a001495. doi: 10.1101/mcs.a001495
48. Hagemann U, Sommer A, Kristian A, Wang E, Larsen Å, Wirnitzer U, et al. Abstract 5199: preclinical activity of the FGFR2-targeted thorium-227 conjugate in preclinical models of colorectal, gastric and triple-negative breast cancer. Cancer Res. (2017) 77(Suppl. 13):5199. doi: 10.1158/1538-7445.AM2017-5199
49. Helsten T, Elkin S, Arthur E, Tomson B, Carter J, Kurzrock R. The FGFR landscape in cancer: analysis of 4,853 tumors by next-generation sequencing. Clin Cancer Res. (2016) 22:259–67. doi: 10.1158/1078-0432.CCR-14-3212
50. Yasui H, Takeno A, Hara H, Imamura H, Akamatsu H, Fujitani K, et al. Prospective analysis of the expression status of FGFR2 and HER2 in colorectal and gastric cancer populations: DS-screen study. Int J Colorectal Dis. (2022) 37:1393–402. doi: 10.1007/s00384-022-04162-2
52. Servais E, Colovos C, Rodriguez L, Bograd A, Nitadori J, Sima C, et al. Mesothelin overexpression promotes mesothelioma cell invasion and MMP-9 secretion in an orthotopic mouse model and in epithelioid pleural mesothelioma patients. Clin Cancer Res. (2012) 18:2478–89. doi: 10.1158/1078-0432.CCR-11-2614
53. Melaiu O, Stebbing J, Lombardo Y, Bracci E, Uehara N, Bonotti A, et al. MSLN gene silencing has an anti-malignant effect on cell lines overexpressing mesothelin deriving from malignant pleural mesothelioma. PLoS One. (2014) 9:e85935. doi: 10.1371/journal.pone.0085935
54. Castelletti L, Yeo D, van Zandwijk N, Rasko J. Anti-mesothelin car T cell therapy for malignant mesothelioma. Biomark Res. (2021) 9:11. doi: 10.1186/s40364-021-00264-1
55. Lv J, Li P. Mesothelin as a biomarker for targeted therapy. Biomark Res. (2019) 7:18. doi: 10.1186/s40364-019-0169-8
56. Hagemann U, Ellingsen C, Schuhmacher J, Kristian A, Mobergslien A, Cruciani V, et al. Mesothelin-targeted thorium-227 conjugate (MSLN-TTC): preclinical evaluation of a new targeted alpha therapy for mesothelin-positive cancers. Clin Cancer Res. (2019) 25:4723–34. doi: 10.1158/1078-0432.CCR-18-347656
57. Clinicaltrials.Gov. First-in-human study of BAY2287411 injection, a thorium-227 labeled antibody-chelator conjugate, in patients with tumors known to express mesothelin. ClinicalTrials.gov identifier: NCT03507452. (2018). Available online at: https://clinicaltrials.gov/ct2/show/NCT03507452 (Accessed September 2, 2022).
58. Heyerdahl H, Abbas N, Sponheim K, Mollatt C, Bruland Ø, Dahle J. Targeted alpha therapy with 227Th-trastuzumab of intraperitoneal ovarian cancer in nude mice. Curr Radiopharm. (2013) 6:106–16. doi: 10.2174/18744710113069990018
59. Abbas N, Bruland ØS, Brevik E, Dahle J. Preclinical evaluation of 227Th-labeled and 177Lu-labeled trastuzumab in mice with HER-2-positive ovarian cancer xenografts. Nucl Med Commun. (2012) 33:838–47. doi: 10.1097/MNM.0b013e328354df7c
60. Heyerdahl H, Abbas N, Brevik E, Mollatt C, Dahle J. Fractionated therapy of HER2-expressing breast and ovarian cancer xenografts in mice with targeted alpha emitting 227Th-DOTA-p-benzyl-trastuzumab. PLoS One. (2012) 7:e42345. doi: 10.1371/journal.pone.0042345
61. Hammer S, Hagemann U, Zitzmann-Kolbe S, Larsen A, Ellingsen C, Geraudie S, et al. Preclinical efficacy of a PSMA-targeted thorium-227 conjugate (PSMA-TTC), a targeted alpha therapy for prostate cancer. Clin Cancer Res. (2020) 26:1985–96. doi: 10.1158/1078-0432.Ccr-19-2268
62. Clinicaltrials.Gov. Study to evaluate the safety, tolerability, pharmacokinetics, and antitumor activity of a thorium-227 labeled antibody-chelator conjugate alone and in combination with darolutamide, in patients with metastatic castration resistant prostate cancer. ClinicalTrials.gov identifier: NCT03724747. (2018). Available online at: https://clinicaltrials.gov/ct2/show/NCT03724747 (Accessed September 2, 2022).
63. Stok C, Kok Y, van den Tempel N, van Vugt M. Shaping the BRCAness mutational landscape by alternative double-strand break repair, replication stress and mitotic aberrancies. Nucleic Acids Res. (2021) 49:4239–57. doi: 10.1093/nar/gkab151
64. Morales J, Li L, Fattah F, Dong Y, Bey E, Patel M, et al. Review of poly (ADP-ribose) polymerase (PARP) mechanisms of action and rationale for targeting in cancer and other diseases. Crit Rev Eukaryot Gene Expr. (2014) 24:15–28. doi: 10.1615/critreveukaryotgeneexpr.2013006875
65. Rottenberg S, Jaspers J, Kersbergen A, van der Burg E, Nygren A, Zander S, et al. High sensitivity of BRCA1-deficient mammary tumors to the PARP inhibitor AZD2281 alone and in combination with platinum drugs. Proc Natl Acad Sci USA. (2008) 105:17079–84. doi: 10.1073/pnas.0806092105
66. Bryant H, Schultz N, Thomas H, Parker K, Flower D, Lopez E, et al. Specific killing of BRCA2-deficient tumours with inhibitors of poly(ADP-ribose) polymerase. Nature. (2005) 434:913–7. doi: 10.1038/nature03443
67. Schatz C, Hammer S, Wengner A, Hagemann U, Mumberg D, Scholz A. Abstract 1393: PSMA-targeted thorium conjugate (BAY 2315497) and olaparib combination show synergistic anti-tumor activity in prostate cancer models. Cancer Res. (2021) 81(Suppl. 13):1393. doi: 10.1158/1538-7445.Am2021-1393
68. Chen S, Lees-Miller J, He Y, Lees-Miller S. Structural insights into the role of DNA-PK as a master regulator in NHEJ. Genome Instab Dis. (2021) 2:195–210. doi: 10.1007/s42764-021-00047-w
69. Berger M, Wortmann L, Buchgraber P, Lucking U, Zitzmann-Kolbe S, Wengner A, et al. BAY-8400: a novel potent and selective DNA-PK inhibitor which shows synergistic efficacy in combination with targeted alpha therapies. J Med Chem. (2021) 64:12723–37. doi: 10.1021/acs.jmedchem.1c00762
70. Wengner A, Siemeister G, Lücking U, Lefranc J, Wortmann L, Lienau P, et al. The novel ATR inhibitor BAY 1895344 is efficacious as monotherapy and combined with DNA damage-inducing or repair-compromising therapies in preclinical cancer models. Mol Cancer Ther. (2020) 19:26–38. doi: 10.1158/1535-7163.Mct-19-0019
71. Yap T, Tan D, Terbuch A, Caldwell R, Guo C, Goh B, et al. First-in-human trial of the oral ataxia telangiectasia and RAD3-Related (ATR) inhibitor BAY 1895344 in patients with advanced solid tumors. Cancer Discov. (2021) 11:80–91. doi: 10.1158/2159-8290.Cd-20-0868
72. Marechal A, Zou L. DNA damage sensing by the ATM and ATR kinases. Cold Spring Harb Perspect Biol. (2013) 5:a012716. doi: 10.1101/cshperspect.a012716
73. Gameiro S, Jammeh M, Wattenberg M, Tsang K, Ferrone S, Hodge J. Radiation-induced immunogenic modulation of tumor enhances antigen processing and calreticulin exposure, resulting in enhanced T-cell killing. Oncotarget. (2014) 5:403–16. doi: 10.18632/oncotarget.1719
74. Vanpouille-Box C, Alard A, Aryankalayil M, Sarfraz Y, Diamond J, Schneider R, et al. DNA exonuclease Trex1 regulates radiotherapy-induced tumour immunogenicity. Nat Commun. (2017) 8:15618. doi: 10.1038/ncomms15618
75. Ko E, Formenti S. Radiation therapy to enhance tumor immunotherapy: a novel application for an established modality. Int J Radiat Biol. (2019) 95:936–9. doi: 10.1080/09553002.2019.1623429
76. Gorin J, Ménager J, Gouard S, Maurel C, Guilloux Y, Faivre-Chauvet A, et al. Antitumor immunity induced after α irradiation. Neoplasia. (2014) 16:319–28. doi: 10.1016/j.neo.2014.04.002
77. Simon I, Perales S, Casado-Medina L, Rodríguez-Martínez A, Garrido-Navas M, Puche-Sanz I, et al. Cross-resistance to abiraterone and enzalutamide in castration resistance prostate cancer cellular models is mediated by AR transcriptional reactivation. Cancers. (2021) 13:1483. doi: 10.3390/cancers13061483
78. European Medicines Agency. Nubeqa Summary of Product Characteristics. (n.d.). Available online at: https://www.ema.europa.eu/en/medicines/human/epar/nubeqa (Accessed October 3, 2022).
79. Food and Drug Administration. Nubeqa Prescribing Information. (n.d.). Available online at: https://www.accessdata.fda.gov/drugsatfda_docs/label/2022/212099s002lbl.Pdf (Accessed October 3, 2022).
80. Hammer S, Schlicker A, Zitzmann-Kolbe S, Baumgart S, Hagemann U, Scholz A, et al. Darolutamide potentiates the antitumor efficacy of a PSMA-targeted thorium-227 conjugate by a dual mode of action in prostate cancer models. Clin Cancer Res. (2021) 27:4367–78. doi: 10.1158/1078-0432.Ccr-21-0342
81. Schatz C, Zitzmann-Kolbe S, Haendler B, Hennekes H, Hammer S, Scholz A editors. Abstract 3311: darolutamide potentiates the antitumor efficacy of a PSMA-targeted thorium-227 conjugate (PSMA-TTC) in a hormone-independent prostate cancer model. Cancer Res. (2022) 82:3311. doi: 10.1158/1538-7445.AM2022-3311
82. Eychenne R, Cherel M, Haddad F, Guerard F, Gestin J. Overview of the most promising radionuclides for targeted alpha therapy: the “hopeful eight”. Pharmaceutics. (2021) 13:906. doi: 10.3390/pharmaceutics13060906
83. Sathekge M, Bruchertseifer F, Knoesen O, Reyneke F, Lawal I, Lengana T, et al. 225Ac-PSMA-617 in chemotherapy-naive patients with advanced prostate cancer: a pilot study. Eur J Nucl Med Mol Imaging. (2019) 46:129–38. doi: 10.1007/s00259-018-4167-0
84. Sathekge M, Bruchertseifer F, Vorster M, Lawal I, Knoesen O, Mahapane J, et al. Predictors of overall and disease-free survival in metastatic castration-resistant prostate cancer patients receiving 225Ac-PSMA-617 radioligand therapy. J Nucl Med. (2020) 61:62–9. doi: 10.2967/jnumed.119.229229
85. Sathekge M, Bruchertseifer F, Vorster M, Lawal I, Knoesen O, Mahapane J, et al. mCRPC patients receiving 225Ac-PSMA-617 therapy in post androgen deprivation therapy setting: response to treatment and survival analysis. J Nucl Med. (2022) 63:1496–502. doi: 10.2967/jnumed.121.263618
86. Juneau D, Saad F, Berlin A, Metser U, Puzanov I, Lamonica D, et al. Preliminary dosimetry results from a first-in-human phase I study evaluating the efficacy and safety of [225Ac]-FPI-1434 in patients with IGF-1R expressing solid tumors. J Nucl Med. (2021) 62(Suppl. 1):74.
87. Atallah E, Berger M, Jurcic J, Roboz G, Tse W, Mawad R, et al. A phase 2 study of actinium-225 (225Ac)-lintuzumab in older patients with untreated acute myeloid leukemia (AML). J Med Imaging Radiat Sci. (2019) 50(Suppl. 1):S37.
88. Abedin S, Murthy G, Hamadani M, Michaelis L, Runaas L, Carlson K, et al. Lintuzumab-Ac225 in combination with CLAG-M yields high MRD (-) responses in R/R AML with adverse features: interim results of a phase I study. Blood. (2021) 138(Suppl. 1):3414. doi: 10.1182/blood-2021-148746
89. Rosenblat T, McDevitt M, Carrasquillo J, Pandit-Taskar N, Frattini M, Maslak P, et al. Treatment of patients with acute myeloid leukemia with the targeted alpha-particle nanogenerator actinium-225-lintuzumab. Clin Cancer Res. (2022) 28:2030–7. doi: 10.1158/1078-0432.CCR-21-3712
90. Morgenstern A, Apostolidis C, Kratochwil C, Sathekge M, Krolicki L, Bruchertseifer F. An overview of targeted alpha therapy with 225Actinium and 213Bismuth. Curr Radiopharm. (2018) 11:200–8. doi: 10.2174/1874471011666180502104524
91. Sartor O, de Bono J, Chi K, Fizazi K, Herrmann K, Rahbar K, et al. Lutetium-177–PSMA-617 for metastatic castration-resistant prostate cancer. N Engl J Med. (2021) 385:1091–103. doi: 10.1056/NEJMoa2107322
92. Hofman M, Emmett L, Violet J, Y Zhang A, Lawrence N, Stockler M, et al. Therap: a randomized phase 2 trial of 177Lu-PSMA-617 theranostic treatment vs cabazitaxel in progressive metastatic castration-resistant prostate cancer (clinical trial protocol ANZUP 1603). BJU Int. (2019) 124:5–13. doi: 10.1111/bju.14876
93. Kratochwil C, Fendler W, Eiber M, Baum R, Bozkurt M, Czernin J, et al. EANM procedure guidelines for radionuclide therapy with 177Lu-labelled PSMA-ligands (177Lu-PSMA-RLT). Eur J Nucl Med Mol Imaging. (2019) 46:2536–44. doi: 10.1007/s00259-019-04485-3
94. Ballal S, Yadav M, Sahoo R, Tripathi M, Dwivedi S, Bal C. 225 Ac-PSMA-617-targeted alpha therapy for the treatment of metastatic castration-resistant prostate cancer: a systematic review and meta-analysis. Prostate. (2021) 81:580–91. doi: 10.1002/pros.24137
95. Sen I, Thakral P, Tiwari P, Pant V, Das S, Manda D, et al. Therapeutic efficacy of 225Ac-PSMA-617 targeted alpha therapy in patients of metastatic castrate resistant prostate cancer after taxane-based chemotherapy. Ann Nucl Med. (2021) 35:794–810. doi: 10.1007/s12149-021-01617-4
96. Tagawa S, Osborne J, Fernandez E, Thomas C, Niaz M, Ciriaco A, et al. Phase I dose-escalation study of PSMA-targeted alpha emitter 225Ac-J591 in men with metastatic castration-resistant prostate cancer (mCRPC). J Clin Oncol. (2020) 38:5560. doi: 10.1200/JCO.2020.38
Keywords: alpha-particle emitter, DNA damage response, immune checkpoint inhibitors, anti-androgen therapies, alpha emitter, targeted alpha therapy, targeted thorium conjugates, thorium-227
Citation: Karlsson J, Schatz CA, Wengner AM, Hammer S, Scholz A, Cuthbertson A, Wagner V, Hennekes H, Jardine V and Hagemann UB (2023) Targeted thorium-227 conjugates as treatment options in oncology. Front. Med. 9:1071086. doi: 10.3389/fmed.2022.1071086
Received: 15 October 2022; Accepted: 15 December 2022;
Published: 09 January 2023.
Edited by:
Øyvind Bruland, Oslo University Hospital, NorwayReviewed by:
Robert Mairs, University of Glasgow, United KingdomCopyright © 2023 Karlsson, Schatz, Wengner, Hammer, Scholz, Cuthbertson, Wagner, Hennekes, Jardine and Hagemann. This is an open-access article distributed under the terms of the Creative Commons Attribution License (CC BY). The use, distribution or reproduction in other forums is permitted, provided the original author(s) and the copyright owner(s) are credited and that the original publication in this journal is cited, in accordance with accepted academic practice. No use, distribution or reproduction is permitted which does not comply with these terms.
*Correspondence: Urs B. Hagemann, dXJzLmhhZ2VtYW5uQGJheWVyLmNvbQ==
Disclaimer: All claims expressed in this article are solely those of the authors and do not necessarily represent those of their affiliated organizations, or those of the publisher, the editors and the reviewers. Any product that may be evaluated in this article or claim that may be made by its manufacturer is not guaranteed or endorsed by the publisher.
Research integrity at Frontiers
Learn more about the work of our research integrity team to safeguard the quality of each article we publish.