- 1Department of Radiation Biology, Institute for Cancer Research, The Norwegian Radium Hospital, Oslo University Hospital, Oslo, Norway
- 2Department of Physics, University of Oslo, Oslo, Norway
- 3Institute for Clinical Medicine, University of Oslo, Oslo, Norway
- 4ARTBIO AS, Oslo, Norway
- 5Department of Oncology, The Norwegian Radium Hospital, Oslo University Hospital, Oslo, Norway
- 6Division of Radiology and Nuclear Medicine, Oslo University Hospital, Oslo, Norway
Metastases are the primary cause of death among cancer patients and efficacious new treatments are sorely needed. Targeted alpha-emitting radiopharmaceuticals that are highly cytotoxic may fulfill this critical need. The focus of this paper is to describe and explore a novel technology that may improve the therapeutic effect of targeted alpha therapy by combining two radionuclides from the same decay chain in the same solution. We hypothesize that the dual targeting solution containing bone-seeking 224Ra and cell-directed complexes of progeny 212Pb is a promising approach to treat metastatic cancers with bone and soft tissue lesions as well as skeletal metastases of mixed lytic/osteoblastic nature. A novel liquid 224Ra/212Pb-generator for rapid preparation of a dual targeting solution is described. Cancer cell targeting monoclonal antibodies, their fragments, synthetic proteins or peptides can all be radiolabeled with 212Pb in the 224Ra-solution in transient equilibrium with daughter nuclides. Thus, 224Ra targets stromal elements in sclerotic bone metastases and 212Pb-chelated-conjugate targets tumor cells of metastatic prostate cancer or osteosarcoma. The dual targeting solution may also be explored to treat metastatic breast cancer or multiple myeloma after manipulation of bone metastases to a more osteoblastic phenotype by the use of bisphosphonates, denosumab, bortezomib or hormone therapy prior to treatment. This may improve targeting of bone-seeking 224Ra and render an augmented radiation dose deposited within metastases. Our preliminary preclinical studies provide conceptual evidence that the dual 224Ra-solution with bone or tumor-targeted delivery of 212Pb has potential to inhibit cancer metastases without significant toxicity. In some settings, the use of a booster dose of purified 212Pb-conjugate alone could be required to elevate the effect of this tumor cell directed component, if needed, e.g., in a fractionated treatment regimen, where the dual targeting solution will act as maintenance treatment.
Introduction
Wide-spread (skeletal, lymph and/or visceral) metastases are responsible for ∼70% of cancer mortality worldwide (1, 2). Understanding and developing targeted therapies for metastatic cancers remain a large unmet medical need. Therapeutic nuclear medicine is emerging rapidly as an additional treatment modality in oncology (3–5). Recently approved beta-emitting 177Lu-DOTATATE (Lutathera®, 2018) targeting somatostatin-2 receptors in patients with metastatic neuroendocrine tumors and 177Lu-PSMA-617 (Pluvicto®, 2022) targeting prostate-specific membrane antigen (PSMA) in patients with metastatic castration-resistant prostate cancer (mCRPC) will clearly shift targeted radionuclide therapy (TRT) into the mainstream of cancer treatment. Nevertheless, some patients either do not respond or following initially good response develop resistance to 177Lu-based therapies, despite sufficient expression of target proteins (6, 7). These patients may, however, respond to targeted alpha therapy (TAT) with 225Ac (6, 7). Both preclinical and clinical studies have clearly demonstrated that alpha-emitting radiopharmaceuticals are more efficient in tumor cell killing and less damaging to the surrounding normal tissue than beta-emitting radiopharmaceuticals (5, 8–11). Alpha particles deliver a high amount of ionization over a short range (< 100 μm in water/tissue, < 40 μm in bone), inducing more complex double-strand DNA breaks that are harder to repair than single-strand breaks induced by beta particles (10, 11). Alpha-emitting radionuclides are particularly suited for the elimination of single cells and cancer micrometastases (10). TRT with beta- or alpha- emitting radionuclides improve the quality of life and delay disease progression (12, 13), but they are most likely not curative. Further improvements are warranted to enhance the therapeutic benefit. Combining TRT with potentially synergistic agents (chemotherapy, immune checkpoint inhibitors, PARP inhibitors, etc.) or with other radiopharmaceuticals is being evaluated in ongoing clinical trials (14–17). The focus of this paper is to describe and explore a novel technology platform that may improve the therapeutic effect of TRT by combining two radionuclides from the same decay chain; one TAT component targeting the stromal elements of osteoblastic skeletal metastases and the other by selective cell-surface binding to cancer cells in extraskeletal and skeletal metastases.
Dual targeting strategies: Alpha and beta radiopharmaceuticals
It has been demonstrated that tandem therapy with beta-emitting 177Lu-PSMA-617 and alpha-emitting 225Ac-PSMA-617 is an effective treatment approach for mCRPC patients (18–20). In addition, the combination of 177Lu-PSMA-I&T and 225Ac-J591 for progressive mCRPC (33 patients) is being evaluated in an ongoing phase I/II clinical study in the United States (ClinicalTrials.gov Identifier: NCT04886986). It has been hypothesized that additive radiation to PSMA-positive cells should occur when administering the radiopharmaceuticals concurrently since the monoclonal antibody (mAb) J591 and the small molecule ligand PSMA-I&T have different PSMA binding sites (21). Additionally, the team hypothesized that 225Ac-J591 could deliver antitumor activity without xerostomia (21, 22), that is the most common side effect of PSMA-TAT with small molecule ligands (13). However, at the present time, the insufficient availability and radiopharmaceutical aspects of 225Ac limit the wide clinical applications of 225Ac (23–25).
The first, and so far, only approved alpha-emitting radiopharmaceutical 223RaCl2 (Xofigo®, 2013) is used to treat mCRPC that has spread only to the bone (3, 26). Ra-223 binds to osteoblastic stromal elements of bone metastases during mineralization since 223Ra is a calcium mimetic that binds to hydroxyapatite in the bone matrix in areas of high bone turnover. Such osteoblastic bone metastases are predominant in patients with mCRPC (27–29). The spatial distribution of the hydroxyapatite within an osteoblastic tumor facilitates a volume distribution of 223Ra (30). Due to the bone-seeking characteristics of 223Ra, its clinical use is limited to patients with osteoblastic bone (sclerotic, new bone deposition, or formation) metastases (31). Biologically stable complex between a bifunctional chelator with 223Ra and a tumor-targeting vector (small molecule, peptide, mAb, or its fragment) is essential to treat extraskeletal metastases (lymph nodes and visceral). Unfortunately, 223Ra, like other alkaline earth metals, does not form stable complexes in vivo (32–34). A phase I/II study, the AlphaBet trial, evaluating the combination of 177Lu-PSMA-I&T and 223Ra to target PSMA-expressing cancer cells and bone metastasis in 36 mCRPC patients has recently been started in Australia (NCT05383079).
Dual targeting strategy: A cancer cell-surface seeker targeted 227Th and stromal bone-seeker 223Ra
Another radionuclide that attracts interest is 227Th that can be linked to a variety of mAbs. These 227Th-immunoconjugates have shown promising preclinical results (30, 35, 36). Furthermore, 227Th acts as an in vivo generator of bone-seeking 223Ra (Figure 1) that can be additionally exploited to improve therapeutic effects in sclerotic bone metastases (30, 37). Dual bone-targeting strategy by bone-targeted 227Th and 223Ra was introduced in 2004 (38, 39). Radium-223 produced from 227Th decay is a cation that can easily penetrate into sclerotic metastasis. Henriksen et al. suggested to use 227Th-polyphosphonate compounds, DOTMP [1,4,7,10 tetraazacyclododecane N, N′, N″, N″ 1,4,7,10-tetra(methylene) phosphonic acid] or DTMP [diethylene triamine N, N′, N″ penta(methylene) phosphonic acid] to deliver alpha particle radiation to primary bone cancer or skeletal metastases from solid cancers (38). They proposed that the total radioactivity in bone should increase as 227Th decays and 223Ra appears, if the 227Th–labeled bone-seeker solution was free from 223Ra at the time of administration (38). Washiyama et al. studied the biodistribution of bone-seeking 227Th-EDTMP (ethylenediamine-tetramethylenephosphonic acid) and its daughter 223Ra in mice and found high uptake of 227Th-EDTMP and long retention of 223Ra in bones (39). They concluded that even if 223Ra escapes from the bone after 227Th decay, it redistributes to bone, as there are no physical or biological differences between 223Ra injected intravenously and that generated in vivo after 227Th injection (39). In 2008, a dual targeting approach was introduced for the treatment of soft tissue and bone metastases: 227Th-chelator-mAb targeting cancer cell surface antigens and 223Ra targeting osteoblastic stroma (30, 37). The main advantage of 227Th is high availability from beta decay of 227Ac (36). The 18.7 day half-life of 227Th is long enough for proper radiopharmaceutical preparation, transportation and administration. However, a therapeutic window allowing treatment with 227Th-conjugates with acceptable toxicity may exist due to 223Ra ingrowth. A few clinical trials evaluating 227Th-conjugates targeting CD22, mesothelin, PSMA and human epidermal growth factor 2 (HER-2) are registered at clinicaltrials.gov (NCT02581878, NCT03507452, NCT03724747, NCT04147819), but the results are not yet available.
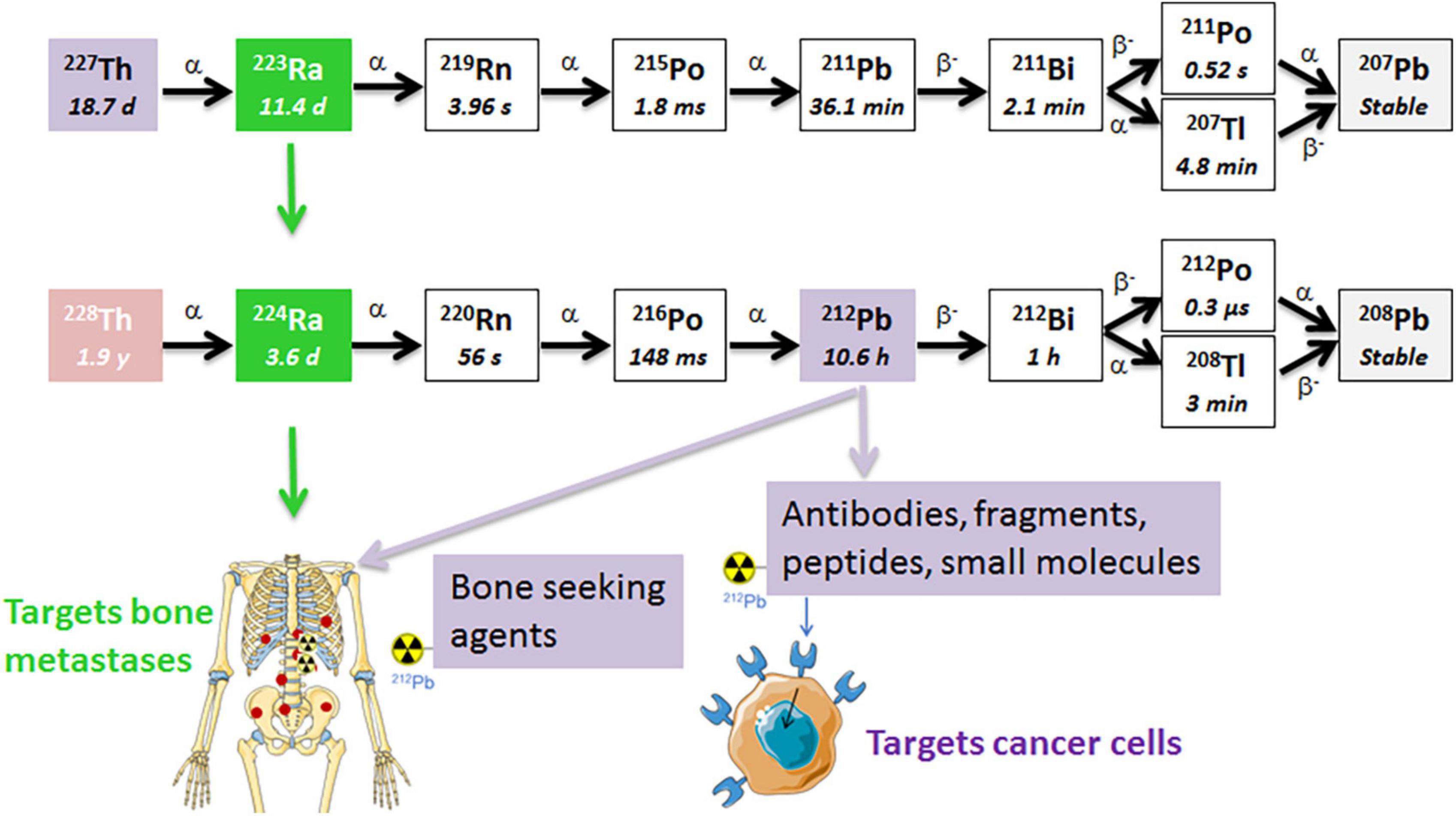
Figure 1. The decay chains of 227Th and 228Th. The radium isotopes are chemically similar to calcium and are natural bone-seekers, and thus, will target osteoblastic bone metastases. The progeny 212Pb in the 224Ra decay chain has a suitable half-life for chelation by a tumor-specific ligand/mAb that targets cancer cells (41, 54, 55). The short half-life of progeny 211Pb in the 223Ra decay chain is not practical for conjugation to a targeting ligand since the majority of the decay will occur before the ligand reaches the tumor sites. α, alpha particle; β, beta particle.
Dual targeting strategy: A bone-seeker 224Ra and a cancer cell-seeker targeted 212Pb in one solution
Two radionuclides from the same decay chain, 224Ra and 212Pb, with its alpha emitting daughter 212Bi (Figure 1), are attractive for cancer therapy due to availability and physical half-lives. These radionuclides can be produced by the end user in clinically relevant amounts from 228Th generators (40–42). The 1.9 year half-life of 228Th allows long-term use of the generator. Similarly to 223Ra, the lack of suitable chelators has limited 224Ra to bone-targeting applications. Both radium isotopes have similar chemical and decay properties, total energies, and biodistribution (41, 43–45). Importantly, 224Ra was widely used decades ago as a pain-relieving treatment of the chronic inflammatory rheumatic disease ankylosing spondylitis (46–49). Administration of total activities of 5.6–11.1 MBq 224Ra (1 MBq of 224Ra chloride solution per weekly injection) to these patients between 1945 and 1975 had neither negative impact on the survival, nor increased significantly the overall rate of second malignancies, as compared to the control population after a mean follow-up time of 24 years (46). The incidence rates of leukemias were 0.014 and 0.009 [hazard ratio 2.56 (95% confidence interval (CI) 0.89–7.54)] in in patients treated and non-treated with 224Ra, respectively (46, 48, 50). Such long-term follow up of a large non-cancer patient population seems very relevant from a radiation safety perspective. The life expectancies in patients with metastatic cancer are significantly shorter.
The daughter nuclides of 224Ra, namely 220Rn (t1/2 ≈ 56 s), 212Pb (t1/2 ≈ 10.6 h), and 212Bi (t1/2 ≈ 1 h), have longer half-lives than those of 223Ra (Figure 1). Lead-212 is suitable for radiolabeling of mAbs, peptides, or other targeting vectors conjugated with appropriate bifunctional chelators. Conjugates of 212Pb/212Bi have already been tested in clinical trials for cancer treatment (51–53). Moreover, these daughter nuclides can be conjugated to chelated targeting agents in the radiopharmaceutical solution of 224Ra in equilibrium with progeny (Figure 2), such as EDTMP for retention of progeny in bone, or a cancer-specific ligand/mAb with a bifunctional chelator TCMC (S-2-(4-isothiocyanatobenzyl)-1,4,7,10-tetraaza-1,4,7,10-tetra(2-carbamoylmethyl)cyclododecane) for cancer cell targeting (41, 54, 55). We hypothesize that the resulting solution will have dual TAT properties: (1) Unbound bone-seeking 224Ra will target metastatic cells on the endosteal surface of bone as well as the stromal elements of osteoblastic skeletal metastases killing these cells; and (2) tumor cell-surface seeking 212Pb-TCMC-targeting agent that will kill the circulating cancer cells and micrometastases by selective binding and deposition of DNA breaking alpha radiation to the cancer cells. The aim of the dual targeting approach is to direct as much as possible of the ionizing radiation of the 224Ra decay chain to the entire spectrum of metastases.
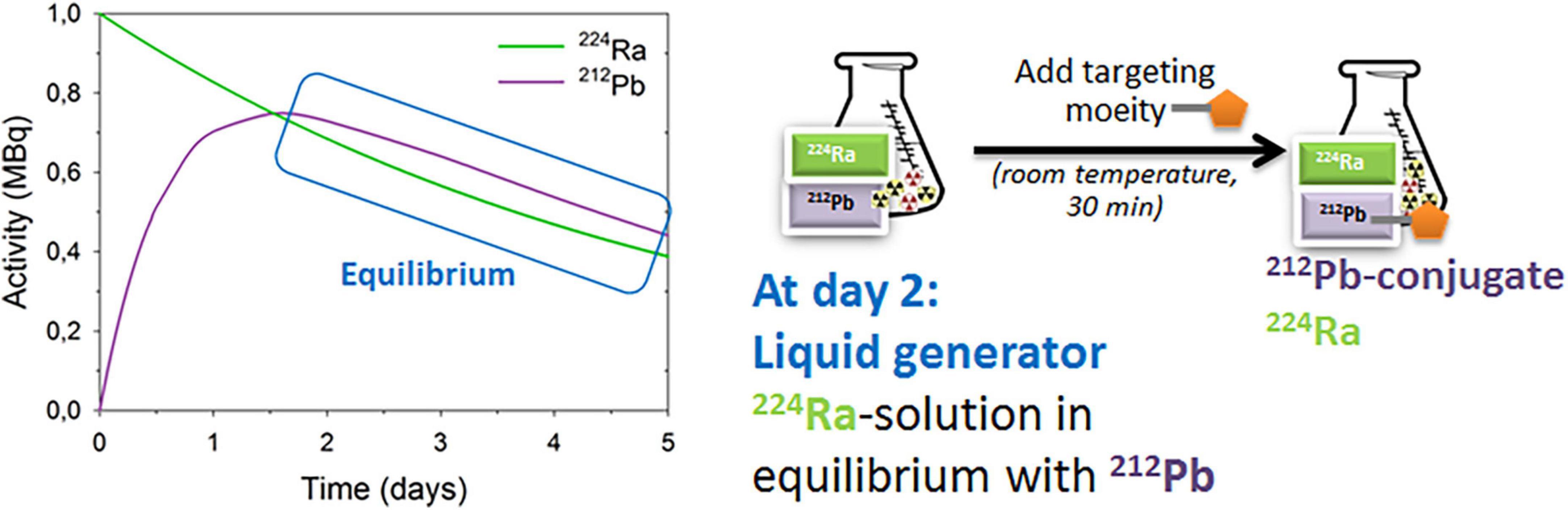
Figure 2. The 224Ra-liquid generator for preparation of dual alpha targeting solution. For details see references (41, 54, 55).
Dual targeting technology: The 224Ra/212Pb liquid generator
A 224Ra-liquid generator for the preparation of dual targeting solution (Figure 2) was developed and patented by Larsen (54). Targeting moieties can be rapidly (≤ 1 h) and efficiently labeled with 212Pb in the 224Ra-solution in transient equilibrium with progenies (41, 55). Additionally, up to 80% free 212Bi can also be conjugated (41). Similar binding and uptake abilities of the 212Pb-labeled PSMA-targeting ligand NG001 in 224Ra-solution or in 212Pb-solution were observed in vitro and in vivo (41, 56, 57). Biodistribution studies of 224Ra with free 212Pb, 212Pb-NG001, and 212Pb-PSMA-617 were tested in athymic nude mice with C4-2 xenografts (41). Importantly, a high uptake of 224Ra in the femur and skull in all groups was shown, demonstrating that 212Pb can be chelated to ligands without compromising the bone-seeking properties of radium in the radiopharmaceutical solution containing the radionuclides (41).
Diffusion of 224Ra progenies and “dose-smoothening effect”
As mentioned above, 224Ra has longer lived progenies, 220Rn and 212Pb, than the isotopes of the same elements in the 223Ra series (Figure 1). Theoretically, these daughter nuclides, especially noble gas 220Rn, will diffuse from the target, because of differing physical half-lives and biological affinities. The first 220Rn progeny, 216Po, has a half-life of 148 ms and decays within very close vicinity to the creation site (58). The mean diffusion length of 216Po in water (soft tissue) is only around 4 μm (58). Lloyd et al. studied retention and distribution of 224Ra and its daughters in beagle dogs, and concluded that the majority of 220Rn produced in bone by 224Ra decay stays in bone (59). It has been demonstrated that 220Rn redistribution leads to toxicity to non-targeted tissues only when extremely high activities of 224Ra were given to patients or animals (47, 59–61). Napoli et al. studied the diffusion and re-adsorption of 224Ra progenies from 224Ra-labeled calcium carbonate microparticles (61). These particles were chosen since radium’s calcium-mimetic properties allow the adsorption of 224Ra onto their surface. It has been demonstrated that 220Rn can escape from the particles, however, it can diffuse from 224Ra-labeled calcium carbonate microparticles only around 300–400 μm in water. It was also documented that the microparticles have the ability to re-adsorb almost all 212Pb generated in the liquid phase from escaped 220Rn (61). If we assume that these particles resemble bone or an osteoblastic bone metastasis, the obtained results may explain the low leakage of 212Pb from bone into systemic circulation, thereby reducing the risk of unwanted radiation exposure of distant tissues. The diffusion of 220Rn up to a few hundred micrometers can extend the effective range of the shorter-range alpha particles from 224Ra itself and may cause a “dose-smoothening” effect in the metastases. Ra-223 is initially shown to be deposited on bone surface and with time is incorporated into the volume of the bone (59). However, areas with “uncalcifying” stroma containing cancer cells in bone metastases (29) will most likely not be eradicated. This contribution from 220Rn may overcome one major limitation of the approved bone-seeking radiopharmaceutical Xofigo when it comes to the short range (only up to 40 μm in bone) of alpha particles in skeletal metastases.
Diffusing alpha-emitters radiation therapy (DaRT) is a novel brachytherapy employing implantable 224Ra enriched seeds for the treatment of solid tumors (58, 62, 63). The 224Ra progenies are shown to diffuse 5–7 mm from the seed and are reported as responsible for the therapeutic effect (63). The efficacy and safety of DaRT have been found to be promising in preclinical and clinical studies (62, 63). DaRT is now in clinical trials for many different cancer types (Table 1).
As mentioned above three of the four alpha particles in the decay chain of 224Ra will decay within a radius of about 400 μm from the 224Ra atom in tissues, while the 212Pb due to the long half-life can potentially diffuse up to several mm in tissues as indicated by the DART technology. If decay takes place in the skeleton, association of 212Pb to hydroxyapatite may considerably limit diffusion range.
Dual targeting alpha therapy and cancers with bone metastases
Bone is the third most frequent site of cancer metastases and the organ-system involved in multiple myeloma (64–66). Bone metastases are especially common in prostate and breast cancer (Table 2). These metastases frequently result in skeletal-related events such as increased pain, hypercalcemia, bone fractures and spinal cord compression, which cause considerable morbidity and reduced quality of life (1, 66, 67). Bone metastases occur as osteolytic lesions, characterized by destruction of normal bone, or as osteoblastic metastases, characterized by formation of new bone matrix (Table 2). The majority of patients with advanced prostate cancer have osteoblastic bone metastases (29). However, some of these patients may have a mixed phenotype or even osteolytic lesions (29).
Dual targeting alpha therapy seems the most suitable for prostate cancer and osteosarcoma since radium localizes in osteoblastic active zones, including on skeletal surfaces and in osteoblastic metastases (77, 78). For cancers without extraskeletal metastases, 212Pb can be chelated to organic phosphates, e.g., EDTMP, which are incorporated into the bone matrix (79), whereas for cancers with extraskeletal metastases, 212Pb can be chelated to small molecules or mAbs targeting cancer cells.
Stromal manipulations: From osteolytic to osteoblastic
The skeletal lesions in multiple myeloma, breast, renal, and lung cancer patients are most commonly osteolytic (Table 2). Additionally, patients with these cancers may have extraskeletal metastases. A few clinical trials are registered to explore the potential of 223Ra, mainly in combination with other drugs (Table 3). However, bisphosphonates, denosumab, bortezomib, and antihormonal therapies may alter the bone matrix of the disease and lead to a more avid target for radium (80, 81). It is documented that the administration of bisphosphonates alters the lytic/blastic ratio in bone lesions toward a more blastic phenotype, and increase uptake of bone-seeking beta-emitting radiopharmaceuticals, such as 89Sr and 153Sm-EDTMP (81). Bortezomib and other proteasome inhibitors can also restore the impaired osteoblast activity (81–83). Denosumab is a mAb that binds the cytokine receptor activator of NFκB ligand (RANKL) that is an essential factor initiating bone turnover (84). RANKL inhibition blocks osteoclast maturation, function and survival, thus reducing bone resorption (84). It has been demonstrated that breast cancer patients after chronic bisphosphonate therapy and multiple myeloma patients after bortezomib treatment had increased 99Tm-labeled methylene diphosphonate (MTD) uptake in osseous bone metastases (81).
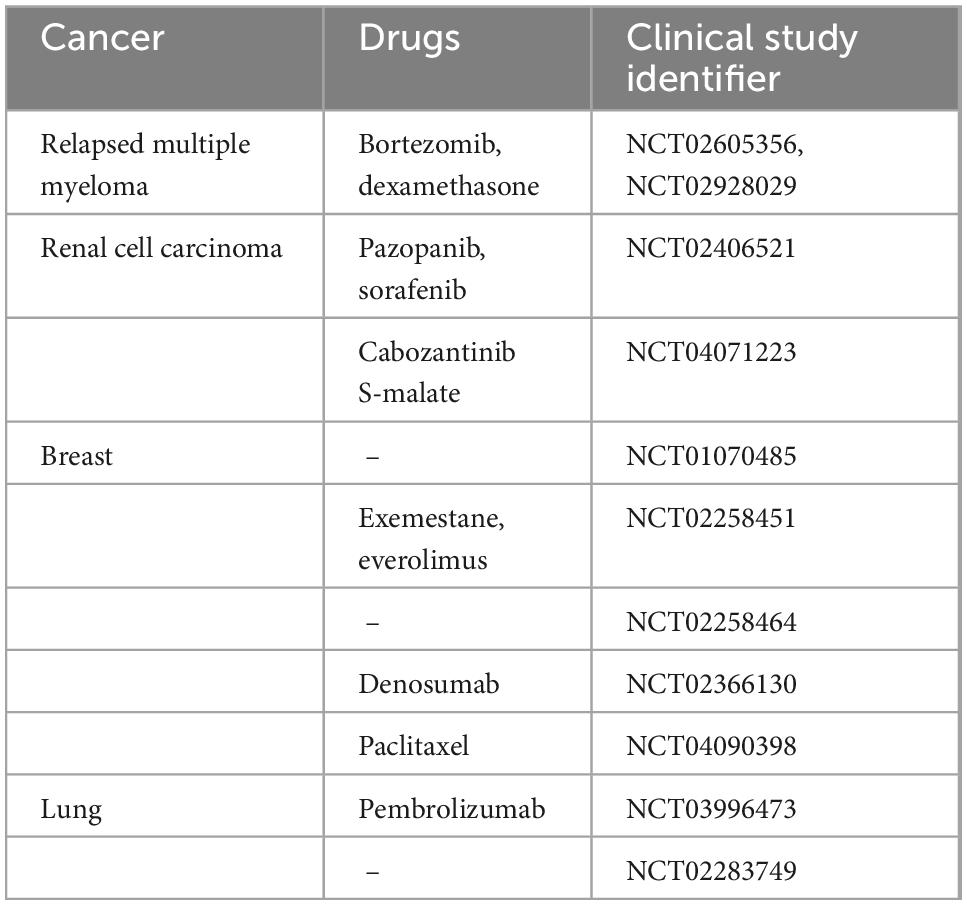
Table 3. List of clinical trials with Xofigo alone or in combination with other drugs in cancers with dominant osteolytic lesions.
Ra-223 has been used for breast cancer patients with bone-dominant disease with osteoblastic and osteolytic lesions (85–87). Coleman et al. have demonstrated that 223Ra targeted osteoblastic, but not osteolytic lesions, in breast cancer patients with bone-dominant disease (85). The results are not surprising because the balance between osteoblastic and osteolytic lesions have not been taken into account (87). However, these studies have demonstrated that 223Ra is safe (85–87), with the potential to be combined with other therapies after pretreatment with bisphosphonates and denosumab.
Suominen et al. investigated the effect of 223Ra, bortezomib and their combination in the syngeneic 5TGM1 mouse multiple myeloma model in vivo (82). The combination of bortezomib and 223Ra improved the incorporation of 223Ra into multiple myeloma bone lesions, decreased synergistically the area of osteolytic lesions and decreased tumor burden and restored body weights in mice (82).
Preclinical studies of dual targeting alpha therapy
Dual targeting alpha therapy seems more suitable for breast cancer patients than 223Ra alone because a 212Pb-conjugate potentially can target breast cancer cells all over the body or alternatively be made bone directed (i.e., dual bone targeting). Preclinical results demonstrated that a single dose of dual bone 224Ra-solution with EDTMP prolonged survival time and lowered incidence of paralysis and bone metastases in nude mice with breast cancer micrometastases (55). Epidermal growth-factor receptor (EGFR) is overexpressed in 15–70% of breast cancer (88, 89), and thus, is an attractive candidate for dual targeting alpha therapy.
Example 1
To test the proof of concept of our dual targeting approach the EGFR-targeting mAb cetuximab (CTX) and bone-targeting EDTMP were chosen for our pilot studies (unpublished results). The mAb or EDTMP were labeled with 212Pb in 224Ra solutions in equilibrium with progenies (pH adjusted to 5–6 by 0.5 M C2H7NO2 or C2H3NaO2). TCMC-mAb was added to a final concentration of 0.1–1 mg/ml. The solutions were mixed on a Thermomixer (Eppendorf, Hamburg) for 30 min at 37°C. Radiochemical purity of the samples was determined by instant thin layer chromatography (Tec-control, Biodex, Medical Systems, Shirley, NY), and only products with purities ≥ 95% were used in the experiments.
The anti-cancer effects of 224Ra-solutions with TCMC-CTX or EDTMP were investigated in 6 weeks old female athymic Nude-Foxn1nu mice (bred at the Comparative Medicine Department, Oslo University Hospital) with breast cancer metastasis. MDA-MB-231-luciferase (Luc) expressing breast cancer cells (2 × 105 cells/100 μl PBS per mouse) were injected into the left ventricle of mouse heart (intracardiac injection). Sodium chloride (0.9% NaCl, control), 300 kBq/kg 224Ra & 212Pb-EDTMP, or 300 kBq/kg 224Ra&212Pb-TCMC-CTX were intravenously administered to mice 2 days after cell injection. Tumor metastases were monitored by bioluminescence imaging in an IVIS Spectrum in vivo imaging system (PerkinElmer, Waltham, MA) 24, 31, and 38 days after intravenous injection of compounds. Each mouse was injected intraperitoneally with 0.2 ml D-luciferin (Biosynth AG, Staad, Switzerland) dissolved in Dulbecco’s PBS (20 mg/ml) 10 min prior to imaging. During imaging, mice were under gas anesthesia (∼3.5% Sevoflurane in oxygen at 0.5 L/min; Baxter, IL, USA). All bioluminescence data are displayed in radiance (photons/s/cm2/str) under identical acquisition conditions. Mice were euthanized by cervical dislocation when cachexia, paraplegia or any signs of severe sickness or discomfort was observed. The studies were approved by the Institutional Committee on Research Animal Care (Department of Comparative Medicine, Oslo University Hospital) and the Norwegian Food Safety Authority (Brumunddal, Norway, approval: FOTS ID 22197).
Dual targeting alpha therapy extended survival in EDTMP and cetuximab group compared to the control [0.9% sodium chloride (NaCl)] group, and lowered the incidence of bone and extraskeletal metastases (Figure 3). The preliminary studies provide conceptual and strong evidence that dual targeting 224Ra-solution with bone or tumor-targeted delivery of 212Pb has potential to inhibit cancer metastases without significant toxicity. Several molecular targets are being explored to target HER2, estrogen receptor and progesterone receptor for nuclear medicine imaging (90, 91), and they can be suitable for dual targeting alpha therapy of breast cancer after stromal manipulation.
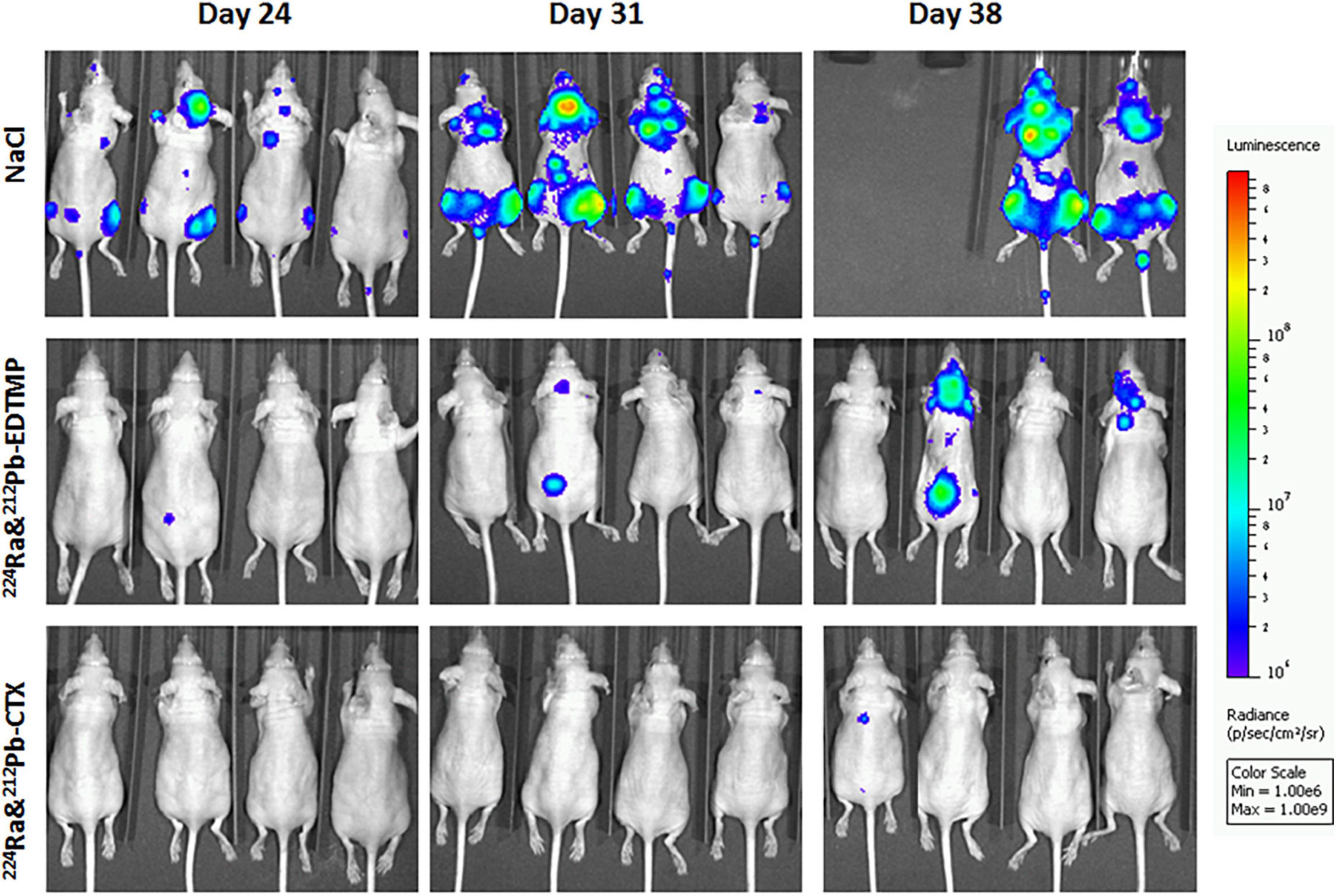
Figure 3. The influence of dual targeting alpha solution on breast cancer metastases growth in mice. Tumor metastases were monitored by bioluminescence imaging in the different therapy groups 24, 31, and 38 days after intravenous injection of 0.9% sodium chloride (NaCl, control), 300 kBq/kg 224Ra&212Pb-EDTMP, or 300 kBq/kg 224Ra&212Pb-TCMC-cetuximab (CTX). MDA-MB-231-Luc breast cancer cells (2 × 105 cells/mouse) were injected intracardially into athymic Nude-Foxn1nu mice 2 days before the treatment. The mice are positioned in the same order at all-time points. The studies were approved by the Institutional Committee on Research Animal Care (Department of Comparative Medicine, Oslo University Hospital) and the Norwegian Food Safety Authority (Brumunddal, Norway, approval: FOTS ID 22197).
Example 2
In prostate cancers, EGFR is weakly expressed in neoplastic cells while it is highly expressed in metastatic lesions (92, 93). The effectiveness of 224Ra-solution with 212Pb-TCMC-CTX directed against EGFR-positive multicellular LNCaP spheroids, an in vitro model for micrometastatic cancer, was investigated (unpublished data).
Ra-224-solution with CTX effectively stopped the growth of LNCaP spheroids relative to the equivalent dose of 224Ra-solution alone or RTX (unpublished data, Figure 4).
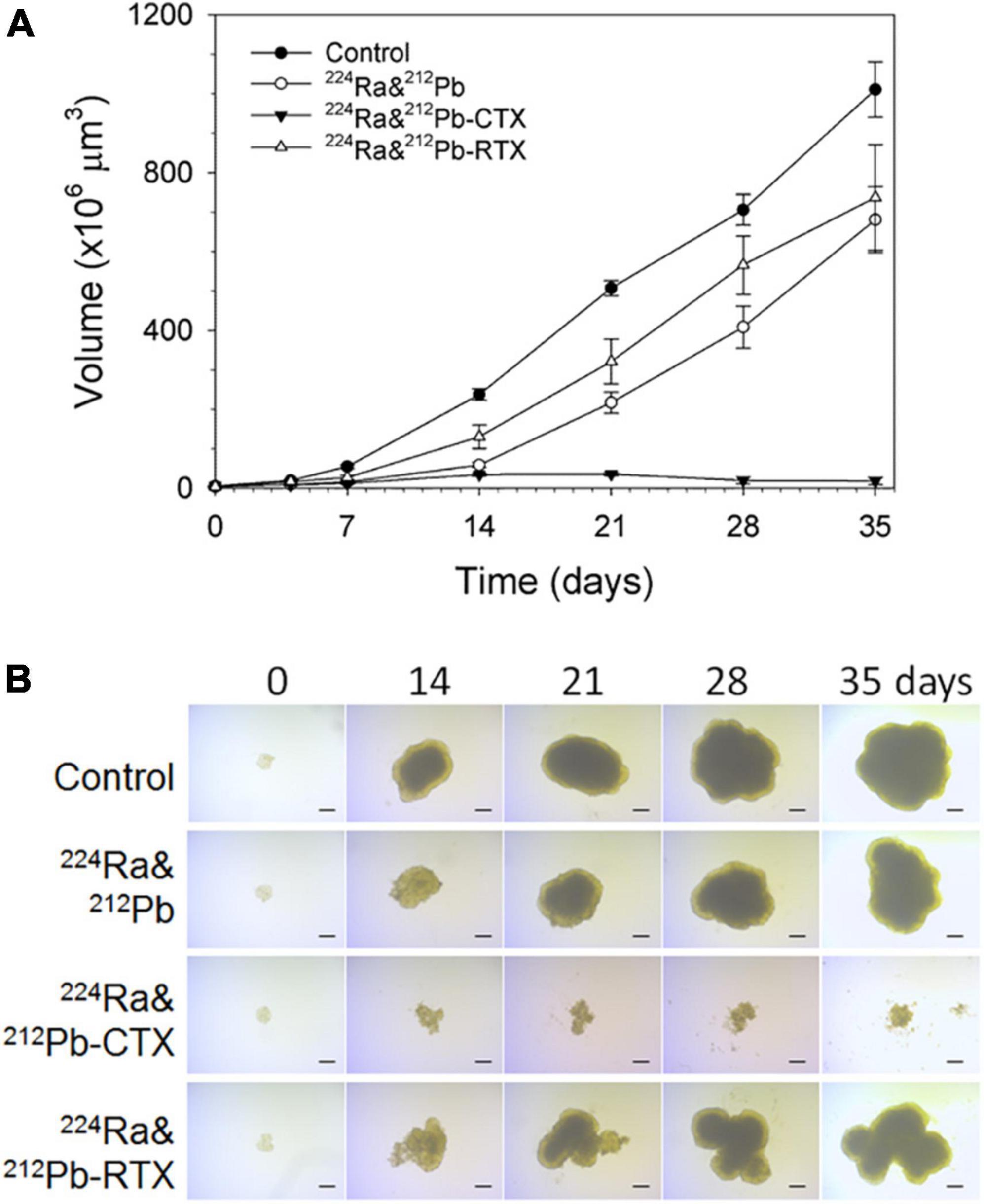
Figure 4. The influence of dual targeting alpha solution on prostate cancer multicellular LNCaP spheroid growth after incubation for 4 h. (A) Growth of spheroids treated with 1 kBq/ml 224Ra&212Pb, 224Ra&212Pb-TCMC-cetuximab (CTX), and 224Ra&212Pb-TCMC- rituximab (RTX, negative control) groups was measured for up to 35 days and is presented as volume (×106 μm3) ± SD. The images of LNCaP spheroids were measured for up to 35 days. (B) Representative microscope images (×4 magnification) were taken at the predefined study end point of 35 days using a bright-field microscope with AxioVision Rel. 4.8 software. LNCaP spheroids were generated by cultivation of cells in liquid overlay in 1.5% agarose-coated flat bottom 96-well plates (94). Cell suspensions of 500 cells in 100 μL medium were added to each well, followed by centrifugation of the plates at 470 × g for 15 min. After an initial incubation time of 3 days, spheroids with diameter of ∼229 μm were formed.
Transferring dual targeting solution into clinic
Several combination treatments with TAT have been proposed (95–98). Their goal is to increase efficacy by using therapies with different action mechanisms together with TAT keeping toxic effects to a minimum. Dual targeting solution may allow increasing therapeutic efficacy and reducing toxicities of 224Ra and its progenies to normal organs. Transferring dual targeting solution into clinic will be more complicated due to the complexity of the product (224Ra, its progenies, targeting agent, and chelator). A few therapy cycles of dual targeting alpha therapy, similarly to single alpha therapies, will be needed. In some cases, a booster dose of cancer cell-seeker targeted 212Pb alone will be required. At the same time, the non-toxic administered activity of 224Ra and/or 212Pb may be chosen based on earlier or ongoing clinical studies. As was mentioned earlier, 224Ra was used in the treatment of ankylosing spondylitis, and long-term toxicity and carcinogenicity data in humans exist (46–48). Additionally, the seeds with 224Ra (Table 1) and bio-degradable calcium carbonate microparticles with 224Ra (NCT03732768) are under clinical investigations for cancer treatment. A few clinical trials investigating 212Pb-conjugates are registered on Clinicaltrials.gov (Table 4). Phase I studies of 212Pb-TCMC-trastuzumab and 212Pb-DOTAMTATE demonstrated safety and feasibility in patients with HER-2 expressing malignancies (99) and somatostatin receptor (SSTR) expressing neuroendocrine tumors (52).
Summary of dual targeting technology
The dual targeting solutions taking advantage of both the bone seeking 224Ra and cell directed complexes of 212Pb seems a promising approach to treat metastatic cancers presenting with bone and soft tissue lesions and also of skeletal metastases of mixed lytic/osteogenic nature. The radioactivity of the solutions will probably be dictated by the tolerability to the longer lived 224Ra. In this regard, the knowledge of long-term and short-term toxicity of 224Ra in the previous mentioned ankylosing spondylitis series may be important in determining the suitable activity levels. In some settings the use of a booster dose of purified 212Pb-radioligand alone could be a possible tactic to elevate the effect of this component, if needed, e.g., in fractionated scheduled treatment regimen, where the dual targeting solution then will act as maintenance treatment. It could be a regulatory challenge to develop such a combined product. Anyhow, some clinical data of the purified 212Pb-ligand alone would be required.
Cell lines
The cell lines present in this study were obtained from ATCC (LNCaP cell line) and Cell Biolabs Inc. (MDA-MB-231-Luc cell line).
Data availability statement
The raw data supporting the conclusions of this article will be made available by the authors, without undue reservation.
Ethics statement
The animal study was reviewed and approved by the Norwegian Food Safety Authority (Brumunddal, Norway, approval: FOTS ID 22197).
Author contributions
AJ, ØB, and RL: conceptualization. AJ, VS, ØB, and RL: designing the work and interpretation of results. AJ and RL: in vitro, in vivo experiments, and analyzing data. AJ, VS, ØB, M-ER, and RL: drafting the manuscript and revising it critically for important intellectual content. All authors have read and agreed to the published version of the manuscript.
Funding
This research was funded by the Norwegian Research Council and Artbio AS [Industrial Ph.D. project number 260639 (Vilde Stenberg), Oslo, Norway], and the South-Eastern Norway Regional Health Authority (project number 2020028, Oslo, Norway).
Acknowledgments
We would like to thank Li-Wei Ma for his support in the execution and follow-up of the multicellular tumor spheroid and animal experiments described in this manuscript.
Conflict of interest
ØB and RL hold ownership interest in ARTBIO AS.
The remaining authors declare that the research was conducted in the absence of any commercial or financial relationships that could be construed as a potential conflict of interest.
Publisher’s note
All claims expressed in this article are solely those of the authors and do not necessarily represent those of their affiliated organizations, or those of the publisher, the editors and the reviewers. Any product that may be evaluated in this article, or claim that may be made by its manufacturer, is not guaranteed or endorsed by the publisher.
References
1. Coleman R, Hadji P, Body J, Santini D, Chow E, Terpos E, et al. Bone health in cancer: ESMO Clinical Practice Guidelines. Ann Oncol. (2020) 31:1650–63. doi: 10.1016/j.annonc.2020.07.019
2. Dillekås H, Rogers M, Straume O. Are 90% of deaths from cancer caused by metastases? Cancer Med. (2019) 8:5574–6. doi: 10.1002/cam4.2474
3. Herrmann K, Schwaiger M, Lewis J, Solomon S, McNeil B, Baumann M, et al. Radiotheranostics: a roadmap for future development. Lancet Oncol. (2020) 21:e146–56. doi: 10.1016/s1470-204530821-6
4. Solnes L, Shokeen M, Pandit-Taskar N. Novel agents and future perspectives on theranostics. Semin Radiat Oncol. (2021) 31:83–92. doi: 10.1016/j.semradonc.2020.07.010
5. Jadvar H, Colletti P. Targeted α-therapy in non-prostate malignancies. Eur J Nucl Med Mol Imaging. (2021) 49:47–53. doi: 10.1007/s00259-021-05405-0
6. Zhang J, Kulkarni H, Baum R. 225Ac-DOTATOC-targeted somatostatin receptor α-therapy in a patient with metastatic neuroendocrine tumor of the thymus, refractory to β-radiation. Clin Nucl Med. (2021) 46:1030–1. doi: 10.1097/rlu.0000000000003792
7. Kratochwil C, Bruchertseifer F, Giesel F, Weis M, Verburg F, Mottaghy F, et al. 225Ac-PSMA-617 for PSMA-targeted α-radiation therapy of metastatic castration-resistant prostate cancer. J Nucl Med. (2016) 57:1941–4. doi: 10.2967/jnumed.116.178673
8. Marcu L, Bezak E, Allen B. Global comparison of targeted alpha vs targeted beta therapy for cancer: in vitro, in vivo and clinical trials. Crit Rev Oncol Hematol. (2018) 123:7–20. doi: 10.1016/j.critrevonc.2018.01.001
9. Brechbiel M. Targeted alpha-therapy: past, present, future? Dalton Trans. (2007) 43:4918–28. doi: 10.1039/b704726f
10. Pouget J, Constanzo J. Revisiting the radiobiology of targeted alpha therapy. Front Med. (2021) 8:692436. doi: 10.3389/fmed.2021.692436
11. Ruigrok E, Tamborino G, de Blois E, Roobol S, Verkaik N, De Saint-Hubert M, et al. In vitro dose effect relationships of actinium-225- and lutetium-177-labeled PSMA-I&T. Eur J Nucl Med Mol Imaging. (2022) 49:3627–38. doi: 10.1007/s00259-022-05821-w
12. Sartor O, de Bono J, Chi K, Fizazi K, Herrmann K, Rahbar K, et al. Lutetium-177-PSMA-617 for metastatic castration-resistant prostate cancer. N Engl J Med. (2021) 385:1091–103. doi: 10.1056/NEJMoa2107322
13. Ma J, Li L, Liao T, Gong W, Zhang C. Efficacy and safety of 225Ac-PSMA-617-targeted alpha therapy in metastatic castration-resistant prostate cancer: a systematic review and meta-analysis. Front Oncol. (2022) 12:796657. doi: 10.3389/fonc.2022.796657
14. Gafita A, Marcus C, Kostos L, Schuster D, Calais J, Hofman M. Predictors and real-world use of prostate-specific radioligand therapy: PSMA and beyond. Am Soc Clin Oncol Educ Book. (2022) 42:1–17. doi: 10.1200/edbk_350946
15. Yordanova A, Ahmadzadehfar H. Combination therapies with PRRT. Pharmaceuticals. (2021) 14:1005. doi: 10.3390/ph14101005
16. Aicher A, Sindrilaru A, Crisan D, Thaiss W, Steinacker J, Beer M, et al. Short-interval, low-dose peptide receptor radionuclide therapy in combination with PD-1 checkpoint immunotherapy induces remission in immunocompromised patients with metastatic merkel cell carcinoma. Pharmaceutics. (2022) 14:1466. doi: 10.3390/pharmaceutics14071466
17. Hallqvist A, Svensson J, Hagmarker L, Marin I, Rydén T, Beauregard J, et al. Optimizing the schedule of PARP inhibitors in combination with 177Lu-DOTATATE: a dosimetry rationale. Biomedicines. (2021) 9:1570. doi: 10.3390/biomedicines9111570
18. Khreish F, Ebert N, Ries M, Maus S, Rosar F, Bohnenberger H, et al. (225)Ac-PSMA-617/(177)Lu-PSMA-617 tandem therapy of metastatic castration-resistant prostate cancer: pilot experience. Eur J Nucl Med Mol Imaging. (2020) 47:721–8. doi: 10.1007/s00259-019-04612-0
19. Rosar F, Hau F, Bartholomä M, Maus S, Stemler T, Linxweiler J, et al. Molecular imaging and biochemical response assessment after a single cycle of [225Ac]Ac-PSMA-617/[177Lu]Lu-PSMA-617 tandem therapy in mCRPC patients who have progressed on [177Lu]Lu-PSMA-617 monotherapy. Theranostics. (2021) 11:4050–60. doi: 10.7150/thno.56211
20. Rosar F, Krause J, Bartholomä M, Maus S, Stemler T, Hierlmeier I, et al. Efficacy and safety of [225Ac]Ac-PSMA-617 Augmented [177Lu]Lu-PSMA-617 radioligand therapy in patients with highly advanced mCRPC with poor prognosis. Pharmaceutics. (2021) 13:722. doi: 10.3390/pharmaceutics13050722
21. Miyahira A, Soule H. The history of prostate-specific membrane antigen as a theranostic target in prostate cancer: the cornerstone role of the prostate cancer foundation. J Nucl Med. (2022) 63:331–8. doi: 10.2967/jnumed.121.262997
22. Tagawa S, Sun M, Sartor O, Thomas C, Singh S, Bissassar M, et al. editors. Phase I study of 225Ac-J591 for men with metastatic castration-resistant prostate cancer (mCRPC). J Clin Oncol. (2021) 39:5015.
23. Robertson A, Ramogida C, Schaffer P, Radchenko V. Development of 225Ac radiopharmaceuticals: TRIUMF perspectives and experiences. Curr Radiopharm. (2018) 11:156–72. doi: 10.2174/1874471011666180416161908
24. Morgenstern A, Apostolidis C, Bruchertseifer F. Supply and clinical application of actinium-225 and bismuth-213. Semin Nucl Med. (2020) 50:119–23. doi: 10.1053/j.semnuclmed.2020.02.003
25. Thiele N, Wilson J. Actinium-225 for targeted α therapy: coordination chemistry and current chelation approaches. Cancer Biother Radiopharm. (2018) 33:336–48. doi: 10.1089/cbr.2018.2494
26. Parker C, Nilsson S, Heinrich D, Helle S, O’Sullivan J, Fosså S, et al. Alpha emitter radium-223 and survival in metastatic prostate cancer. N Engl J Med. (2013) 369:213–23. doi: 10.1056/NEJMoa1213755
27. Suominen M, Rissanen J, Käkönen R, Fagerlund K, Alhoniemi E, Mumberg D, et al. Survival benefit with radium-223 dichloride in a mouse model of breast cancer bone metastasis. J Natl Cancer Inst. (2013) 105:908–16. doi: 10.1093/jnci/djt116
28. Suominen M, Wilson T, Käkönen S, Scholz A. The mode-of-action of targeted alpha therapy radium-223 as an enabler for novel combinations to treat patients with bone metastasis. Int J Mol Sci. (2019) 20:3899. doi: 10.3390/ijms20163899
29. Roudier M, Morrissey C, True L, Higano C, Vessella R, Ott S. Histopathological assessment of prostate cancer bone osteoblastic metastases. J Urol. (2008) 180:1154–60. doi: 10.1016/j.juro.2008.04.140
30. Bruland ØS, Dahle J, Olsen D, Larsen R. Targeted high-LET therapy of bone metastases. In: Stigbrand T, Carlsson J, Adams G, editors. Targeted Radionuclide Tumor Therapy Biological Aspects. Dordrecht: Springer (2008). p. 181–94.
31. Bruland ØS, Nilsson S, Fisher D, Larsen R. High-linear energy transfer irradiation targeted to skeletal metastases by the alpha-emitter 223Ra: adjuvant or alternative to conventional modalities? Clin Cancer Res. (2006) 12(20 Pt 2):6250s–7s. doi: 10.1158/1078-0432.Ccr-06-0841
32. Henriksen G, Hoff P, Larsen R. Evaluation of potential chelating agents for radium. Appl Radiat Isot. (2002) 56:667–71. doi: 10.1016/s0969-804300282-2
33. Abou D, Thiele N, Gutsche N, Villmer A, Zhang H, Woods J, et al. Towards the stable chelation of radium for biomedical applications with an 18-membered macrocyclic ligand. Chem Sci. (2021) 12:3733–42. doi: 10.1039/d0sc06867e
34. Gott M, Yang P, Kortz U, Stephan H, Pietzsch H, Mamat C. A 224Ra-labeled polyoxopalladate as a putative radiopharmaceutical. Chem Commun. (2019) 55:7631–4. doi: 10.1039/c9cc02587a
35. Hagemann U, Wickstroem K, Hammer S, Bjerke R, Zitzmann-Kolbe S, Ryan O, et al. Advances in precision oncology: targeted thorium-227 conjugates as a new modality in targeted alpha therapy. Cancer Biother Radiopharm. (2020) 35:497–510. doi: 10.1089/cbr.2020.3568
36. Frantellizzi V, Cosma L, Brunotti G, Pani A, Spanu A, Nuvoli S, et al. Targeted alpha therapy with thorium-227. Cancer Biother Radiopharm. (2020) 35:437–45. doi: 10.1089/cbr.2019.3105
37. Dahle J, Larsen R. Targeted alpha-particle therapy with 227Th-labeled antibodies. Curr Radiopharm. (2008) 1:209–14. doi: 10.1097/MNM.0b013e328354df7c
38. Henriksen G, Bruland O, Larsen R. Thorium and actinium polyphosphonate compounds as bone-seeking alpha particle-emitting agents. Anticancer Res. (2004) 24:101–5.
39. Washiyama K, Amano R, Sasaki J, Kinuya S, Tonami N, Shiokawa Y, et al. 227Th-EDTMP: a potential therapeutic agent for bone metastasis. Nucl Med Biol. (2004) 31:901–8. doi: 10.1016/j.nucmedbio.2004.05.001
40. Westrøm S, Generalov R, Bønsdorff T, Larsen R. Preparation of 212Pb-labeled monoclonal antibody using a novel 224Ra-based generator solution. Nucl Med Biol. (2017) 51:1–9. doi: 10.1016/j.nucmedbio.2017.04.005
41. Stenberg V, Juzeniene A, Bruland ØS, Larsen R. In situ generated 212Pb-PSMA ligand in a 224Ra-solution for dual targeting of prostate cancer sclerotic stroma and PSMA-positive cells. Curr Radiopharm. (2020) 13:130–41. doi: 10.2174/1874471013666200511000532
42. Li R, Stenberg V, Larsen R. A novel experimental generator for production of high purity lead-212 for use in radiopharmaceuticals. J Nucl Med. (2022). doi: 10.2967/jnumed.122.264009
43. Jiang W, Ulmert D, Simons B, Abou D, Thorek D. The impact of age on radium-223 distribution and an evaluation of molecular imaging surrogates. Nucl Med Biol. (2018) 62–3:1–8. doi: 10.1016/j.nucmedbio.2018.05.003
44. Henriksen G, Breistøl K, Bruland ØS, Fodstad Ø, Larsen R. Significant antitumor effect from bone-seeking, alpha-particle-emitting 223Ra demonstrated in an experimental skeletal metastases model. Cancer Res. (2002) 62:3120–5.
45. Schumann S, Eberlein U, Müller J, Scherthan H, Lassmann M. Correlation of the absorbed dose to the blood and DNA damage in leukocytes after internal ex-vivo irradiation of blood samples with Ra-224. EJNMMI Res. (2018) 8:77. doi: 10.1186/s13550-018-0422-4
46. Priest N, Dauer L, Hoel D. Administration of lower doses of radium-224 to ankylosing spondylitis patients results in no evidence of significant overall detriment. PLoS One. (2020) 15:e0232597. doi: 10.1371/journal.pone.0232597
47. Lassmann M, Nosske D, Reiners C. Therapy of ankylosing spondylitis with 224Ra-radium chloride: dosimetry and risk considerations. Radiat Environ Biophys. (2002) 41:173–8. doi: 10.1007/s00411-002-0164-5
48. Wick R, Nekolla E, Gaubitz M, Schulte T. Increased risk of myeloid leukaemia in patients with ankylosing spondylitis following treatment with radium-224. Rheumatology. (2008) 47:855–9. doi: 10.1093/rheumatology/ken060
49. Nekolla E, Kreisheimer M, Kellerer A, Kuse-Isingschulte M, Gössner W, Spiess H. Induction of malignant bone tumors in radium-224 patients: risk estimates based on the improved dosimetry. Radiat Res. (2000) 153:93–103. doi: 10.1667/0033-7587(2000)153[0093:iombti]2.0.co;2
50. Wick R, Nekolla E, Gössner W, Kellerer A. Late effects in ankylosing spondylitis patients treated with 224Ra. Radiat Res. (1999) 152(6 Suppl.):S8–11.
51. Meredith R, Torgue J, Azure M, Shen S, Saddekni S, Banaga E, et al. Pharmacokinetics and imaging of 212Pb-TCMC-trastuzumab after intraperitoneal administration in ovarian cancer patients. Cancer Biother Radiopharm. (2014) 29:12–7. doi: 10.1089/cbr.2013.1531
52. Delpassand E, Tworowska I, Esfandiari R, Torgue J, Hurt J, Shafie A, et al. Targeted alpha-emitter therapy with 212Pb-DOTAMTATE for the treatment of metastatic SSTR-expressing neuroendocrine tumors: first-in-human, dose-escalation clinical trial. J Nucl Med. (2022) 63:1326–33. doi: 10.2967/jnumed.121.263230
53. Kokov K, Egorova B, German M, Klabukov I, Krasheninnikov M, Larkin-Kondrov A, et al. 212Pb: production approaches and targeted therapy applications. Pharmaceutics. (2022) 14:189. doi: 10.3390/pharmaceutics14010189
54. Larsen R. Radiopharmaceutical Solutions with Advantageous Properties. US10434198B2US10434198B2. (2019). Available online at: https://patents.google.com/patent/US10434198B2/en
55. Juzeniene A, Bernoulli J, Suominen M, Halleen J, Larsen R. Antitumor activity of novel bone-seeking, α-emitting 224Ra-solution in a breast cancer skeletal metastases model. Anticancer Res. (2018) 38:1947–55. doi: 10.21873/anticanres.12432
56. Stenberg V, Juzeniene A, Chen Q, Yang X, Bruland ØS, Larsen R. Preparation of the alpha-emitting prostate-specific membrane antigen targeted radioligand [212Pb]Pb-NG001 for prostate cancer. J Labelled Comp Radiopharm. (2020) 63:129–43. doi: 10.1002/jlcr.3825
57. Stenberg V, Tornes A, Nilsen H, Revheim M, Bruland ØS, Larsen R, et al. Factors influencing the therapeutic efficacy of the PSMA targeting radioligand 212Pb-NG001. Cancers. (2022) 14:2784. doi: 10.3390/cancers14112784
58. Arazi L. Diffusing alpha-emitters radiation therapy: approximate modeling of the macroscopic alpha particle dose of a point source. Phys Med Biol. (2020) 65:015015. doi: 10.1088/1361-6560/ab5b73
59. Lloyd R, Mays C, Taylor G, Atherton D, Bruenger F, Jones C. Radium-224 retention, distribution, and dosimetry in beagles. Radiat Res. (1982) 92:280–95.
60. Muggenburg B, Hahn F, Griffith W Jr, Lloyd R, Boecker B. The biological effects of radium-224 injected into dogs. Radiat Res. (1996) 146:171–86.
61. Napoli E, Bønsdorff T, Jorstad I, Bruland ØS, Larsen R, Westrøm S. Radon-220 diffusion from 224Ra-labeled calcium carbonate microparticles: some implications for radiotherapeutic use. PLoS One. (2021) 16:e0248133. doi: 10.1371/journal.pone.0248133
62. Bellia S, Feliciani G, Duca M, Monti M, Turri V, Sarnelli A, et al. Clinical evidence of abscopal effect in cutaneous squamous cell carcinoma treated with diffusing alpha emitters radiation therapy: a case report. J Contemp Brachytherapy. (2019) 11:449–57. doi: 10.5114/jcb.2019.88138
63. Feliciani G, Bellia S, Del Duca M, Mazzotti G, Monti M, Stanganelli I, et al. A new approach for a safe and reproducible seeds positioning for diffusing alpha-emitters radiation therapy of squamous cell skin cancer: a feasibility study. Cancers. (2022) 14:240. doi: 10.3390/cancers14010240
64. Huang J, Shen J, Li X, Rengan R, Silvestris N, Wang M, et al. Incidence of patients with bone metastases at diagnosis of solid tumors in adults: a large population-based study. Ann Transl Med. (2020) 8:482. doi: 10.21037/atm.2020.03.55
65. Bãdilã A, Rãdulescu D, Niculescu A, Grumezescu A, Rãdulescu M, Rãdulescu A. Recent advances in the treatment of bone metastases and primary bone tumors: an up-to-date review. Cancers. (2021) 13:4229. doi: 10.3390/cancers13164229
66. Macedo F, Ladeira K, Pinho F, Saraiva N, Bonito N, Pinto L, et al. Bone metastases: an overview. Oncol Rev. (2017) 11:321. doi: 10.4081/oncol.2017.321
67. Woodward E, Jagdev S, McParland L, Clark K, Gregory W, Newsham A, et al. Skeletal complications and survival in renal cancer patients with bone metastases. Bone. (2011) 48:160–6. doi: 10.1016/j.bone.2010.09.008
68. Brozovich A, Garmezy B, Pan T, Wang L, Farach-Carson M, Satcher R. All bone metastases are not created equal: revisiting treatment resistance in renal cell carcinoma. J Bone Oncol. (2021) 31:100399. doi: 10.1016/j.jbo.2021.100399
69. Hameed A, Brady J, Dowling P, Clynes M, O’Gorman P. Bone disease in multiple myeloma: pathophysiology and management. Cancer Growth Metastasis. (2014) 7:33–42. doi: 10.4137/cgm.S16817
70. Eda H, Santo L, David Roodman G, Raje N. Bone disease in multiple myeloma. Cancer Treat Res. (2016) 169:251–70. doi: 10.1007/978-3-319-40320-5_14
71. Knapp B, Devarakonda S, Govindan R. Bone metastases in non-small cell lung cancer: a narrative review. J Thorac Dis. (2022) 14:1696–712. doi: 10.21037/jtd-21-1502
72. Hendriks L, Hermans B, van den Beuken-van Everdingen M, Hochstenbag M, Dingemans A. Effect of bisphosphonates, denosumab, and radioisotopes on bone pain and quality of life in patients with non-small cell lung cancer and bone metastases: a systematic review. J Thorac Oncol. (2016) 11:155–73. doi: 10.1016/j.jtho.2015.10.001
73. Gong L, Xu L, Yuan Z, Wang Z, Zhao L, Wang P. Clinical outcome for small cell lung cancer patients with bone metastases at the time of diagnosis. J Bone Oncol. (2019) 19:100265. doi: 10.1016/j.jbo.2019.100265
74. Megyesfalvi Z, Tallosy B, Pipek O, Fillinger J, Lang C, Klikovits T, et al. The landscape of small cell lung cancer metastases: organ specificity and timing. Thorac Cancer. (2021) 12:914–23. doi: 10.1111/1759-7714.13854
75. Garcia-Torralba E, Spada F, Lim K, Jacobs T, Barriuso J, Mansoor W, et al. Knowns and unknowns of bone metastases in patients with neuroendocrine neoplasms: a systematic review and meta-analysis. Cancer Treat Rev. (2021) 94:102168. doi: 10.1016/j.ctrv.2021.102168
76. Sheng G, Gao Y, Yang Y, Wu H. Osteosarcoma and metastasis. Front Oncol. (2021) 11:780264. doi: 10.3389/fonc.2021.780264
77. Larsen R, Saxtorph H, Skydsgaard M, Borrebaek J, Jonasdottir T, Bruland O, et al. Radiotoxicity of the alpha-emitting bone-seeker 223Ra injected intravenously into mice: histology, clinical chemistry and hematology. In Vivo. (2006) 20:325–31.
78. Pandit-Taskar N, Larson S, Carrasquillo J. Bone-seeking radiopharmaceuticals for treatment of osseous metastases, part 1: α therapy with 223Ra-dichloride. J Nucl Med. (2014) 55:268–74. doi: 10.2967/jnumed.112.112482
79. Tomblyn M. The role of bone-seeking radionuclides in the palliative treatment of patients with painful osteoblastic skeletal metastases. Cancer Control. (2012) 19:137–44. doi: 10.1177/107327481201900208
80. Humm J, Sartor O, Parker C, Bruland O, Macklis R. Radium-223 in the treatment of osteoblastic metastases: a critical clinical review. Int J Radiat Oncol Biol Phys. (2015) 91:898–906. doi: 10.1016/j.ijrobp.2014.12.061
81. Sartor O, Hoskin P, Bruland O. Targeted radio-nuclide therapy of skeletal metastases. Cancer Treat Rev. (2013) 39:18–26. doi: 10.1016/j.ctrv.2012.03.006
82. Suominen M, Mäki-Jouppila J, Huhtinen A, Sjöholm B, Rissanen J, Luostarinen A, et al. Additive benefits of radium-223 dichloride and bortezomib combination in a systemic multiple myeloma mouse model. Int J Mol Sci. (2021) 22:5570. doi: 10.3390/ijms22115570
83. Heider U, Kaiser M, Müller C, Jakob C, Zavrski I, Schulz C, et al. Bortezomib increases osteoblast activity in myeloma patients irrespective of response to treatment. Eur J Haematol. (2006) 77:233–8. doi: 10.1111/j.1600-0609.2006.00692.x
84. Hanley D, Adachi J, Bell A, Brown V. Denosumab: mechanism of action and clinical outcomes. Int J Clin Pract. (2012) 66:1139–46. doi: 10.1111/ijcp.12022
85. Coleman R, Aksnes A, Naume B, Garcia C, Jerusalem G, Piccart M, et al. A phase IIa, nonrandomized study of radium-223 dichloride in advanced breast cancer patients with bone-dominant disease. Breast Cancer Res Treat. (2014) 145:411–8. doi: 10.1007/s10549-014-2939-1
86. Takalkar A, Adams S, Subbiah V. Radium-223 dichloride bone-targeted alpha particle therapy for hormone-refractory breast cancer metastatic to bone. Exp Hematol Oncol. (2014) 3:23. doi: 10.1186/2162-3619-3-23
87. Winter M, Coleman R, Kendall J, Palmieri C, Twelves C, Howell S, et al. A phase IB and randomised phase IIA trial of CApecitabine plus radium-223 (Xofigo™) in breast cancer patients with BONe metastases: CARBON trial results. J Bone Oncol. (2022) 35:100442. doi: 10.1016/j.jbo.2022.100442
88. Maennling A, Tur M, Niebert M, Klockenbring T, Zeppernick F, Gattenlöhner S, et al. Molecular targeting therapy against EGFR family in breast cancer: progress and future potentials. Cancers. (2019) 11:1826. doi: 10.3390/cancers11121826
89. Lorusso V, Forcignano R, Cinieri S, Tinelli A, Porcelli L, Quatrale A, et al. Which role for EGFR therapy in breast cancer? Front Biosci. (2012) 4:31–42. doi: 10.2741/s249
90. Altunay B, Morgenroth A, Mottaghy F. Use of radionuclide-based imaging methods in breast cancer. Semin Nucl Med. (2022) 52:561–73. doi: 10.1053/j.semnuclmed.2022.04.003
91. Sharma R, Mukherjee A, Mitra J, Sarma H. Unravelling the potential of lutetium-177 labeled pertuzumab targeting HER 2 receptors for theranostic applications. Int J Radiat Oncol Biol Phys. (2022). doi: 10.1016/j.ijrobp.2022.07.029
92. Carlsson J, Shen L, Xiang J, Xu J, Wei Q. Tendencies for higher co-expression of EGFR and HER2 and downregulation of HER3 in prostate cancer lymph node metastases compared with corresponding primary tumors. Oncol Lett. (2013) 5:208–14. doi: 10.3892/ol.2012.996
93. Day K, Lorenzatti Hiles G, Kozminsky M, Dawsey S, Paul A, Broses L, et al. HER2 and EGFR overexpression support metastatic progression of prostate cancer to bone. Cancer Res. (2017) 77:74–85. doi: 10.1158/0008-5472.Can-16-1656
94. Stenberg V, Larsen R, Ma L, Peng Q, Juzenas P, Bruland ØS, et al. Evaluation of the PSMA-binding ligand 212Pb-NG001 in multicellular tumour spheroid and mouse models of prostate cancer. Int J Mol Sci. (2021) 22:4815. doi: 10.3390/ijms22094815
95. Damiana T, Dalm S. Combination therapy, a promising approach to enhance the efficacy of radionuclide and targeted radionuclide therapy of prostate and breast cancer. Pharmaceutics. (2021) 13:674. doi: 10.3390/pharmaceutics13050674
96. Abou D, Fears A, Summer L, Longtine M, Benabdallah N, Riddle R, et al. Improved radium-223 therapy with combination epithelial sodium channel blockade. J Nucl Med. (2021) 62:1751–8. doi: 10.2967/jnumed.121.261977
97. Reissig F, Runge R, Naumann A, Kotzerke J. Cisplatin – A more efficient drug in combination with radionuclides? Nuklearmedizin. (2022) 61:325–32. doi: 10.1055/a-1759-1749
98. Milenic D, Baidoo K, Shih J, Wong K, Brechbiel M. Evaluation of platinum chemotherapy in combination with HER2-targeted α-particle radiation. Cancer Biother Radiopharm. (2013) 28:441–9. doi: 10.1089/cbr.2012.1423
Keywords: cancer, lead-212, radiopharmaceutical, radium-224, radium-223, targeted radionuclide therapy (TRT), targeted alpha particle therapy (TAT)
Citation: Juzeniene A, Stenberg VY, Bruland ØS, Revheim M-E and Larsen RH (2023) Dual targeting with 224Ra/212Pb-conjugates for targeted alpha therapy of disseminated cancers: A conceptual approach. Front. Med. 9:1051825. doi: 10.3389/fmed.2022.1051825
Received: 23 September 2022; Accepted: 05 December 2022;
Published: 17 January 2023.
Edited by:
Dawei Jiang, Huazhong University of Science and Technology, ChinaReviewed by:
Tom A. Bäck, University of Gothenburg, SwedenReinier Hernandez, University of Wisconsin-Madison, United States
Hanyi Fang, Huazhong University of Science and Technology, China
Copyright © 2023 Juzeniene, Stenberg, Bruland, Revheim and Larsen. This is an open-access article distributed under the terms of the Creative Commons Attribution License (CC BY). The use, distribution or reproduction in other forums is permitted, provided the original author(s) and the copyright owner(s) are credited and that the original publication in this journal is cited, in accordance with accepted academic practice. No use, distribution or reproduction is permitted which does not comply with these terms.
*Correspondence: Asta Juzeniene, YXN0YWpAcnItcmVzZWFyY2gubm8=