- 1Emergency and Trauma College, Hainan Medical University, Haikou, China
- 2Key Laboratory of Emergency and Trauma of Ministry of Education, Hainan Medical University, Haikou, China
- 3Department of Oncology, Southwest Hospital, Third Military Medical University (Army Medical University), Chongqing, China
- 4Research Unit of Island Emergency Medicine, Chinese Academy of Medical Sciences (No. 2019RU013), Hainan Medical University, Haikou, China
- 5School of Public Health, Hainan Medical University, Haikou, China
- 6Emergency Medicine Center, Sichuan Provincial People's Hospital, University of Electronic Science and Technology of China, Chengdu, China
The introduction of the Sepsis 3.0 guidelines in 2016 improved our understanding of sepsis diagnosis and therapy. Personalized treatment strategies and nursing methods for sepsis patients are recommended in the “Save Sepsis Campaign” in 2021. However, mortality in sepsis patients remains high. Patients with sepsis-related acute respiratory distress syndrome account for around 30% of them, with fatality rates ranging from 30 to 40%. Pathological specimens from individuals with sepsis-related ARDS frequently demonstrate widespread alveolar damage, and investigations have revealed that pulmonary epithelial and pulmonary endothelial injury is the underlying cause. As a result, the purpose of this work is to evaluate the mechanism and research progress of pulmonary epithelial and pulmonary endothelial damage in sepsis-related ARDS, which may provide new directions for future research, diagnosis, and therapy.
Introduction
Sepsis, one of the most prevalent complications in the ICU, has a high fatality rate due to its complicated molecular underpinnings. Sepsis was described in 2016 as a “life-threatening organ failure produced by an unbalanced host response to infection” (1). Sepsis is frequently characterized by a dysregulated host response to invading pathogens; this systemic inflammatory response can result in disseminated intravascular coagulation, multiple organ dysfunction syndromes (MODS), and mortality (2). With the incremental development of sepsis diagnosis, treatment, and management over the last several decades, and the ongoing updating of recommendations, the mortality rate of sepsis has significantly declined (about 20–30 percent) (3). However, early detection of sepsis, prevention of multiple organ failure, and improved prognosis remain pressing concerns.
Sepsis frequently causes organ dysfunction and damage, such as acute kidney injury (AKI), acute lung injury (ALI), and ALI can be exacerbated by acute respiratory distress syndrome (ARDS). As a result, ARDS is often regarded as a deadly consequence of severe sepsis, with sepsis accounting for around 32% of all cases. The major histological hallmark of ARDS is severe diffuse alveolar damage, which is frequently driven by endothelial dysfunction and local inflammation. As a diverse illness, ARDS frequently manifests as sudden exacerbations of non-cardiogenic pulmonary edema, severe hypoxemia, and the requirement for mechanical ventilation (4, 5).
The focus of this review is on the pathophysiology and current research on sepsis-associated ARDS. It also goes through the biomarkers that play a role in sepsis-related ARDS, which may provide new directions for future research, diagnosis, and therapy.
The potential mechanisms of sepsis-related ARDS
A significant pathogenic characteristic of ARDS is the damage to vascular endothelial (VE) cells, alveolar epithelial cells, and epigenetics. However, the complex pathways underlying sepsis-related ARDS remain unknown. We have illustrated some potential mechanisms in Figure 1.
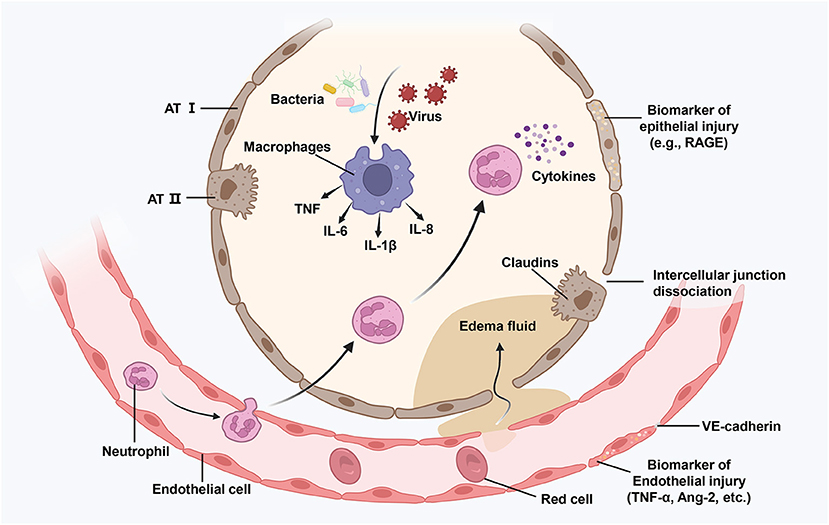
Figure 1. Illustration of an injured alveolus. Various damage factors (such as attack by bacteria and viruses) can directly or indirectly cause damage to the distal alveolar structure and the related microvascular regions. During the exudative phase, alveolar macrophages get activated, resulting in the release of powerful pro-inflammatory mediators and chemokines (such as TNF, IL-6, and IL-8) that promote the accumulation of neutrophils and monocytes. Activated neutrophils (such as cytokines) further promote damage by releasing toxic mediators. The resulting damage causes the loss of barrier functions as well as interstitial an intra-alveolar flooding. ATI, alvcolar type I cell; TNG, tumor necrosis factor; IL-6, interlcukin-6; Ang-2, angiopoietin 2; RAGE, receptor for advance glycation end-products.
VE injury
Although the nature and mechanism of endothelial injury in ARDS remain unknown, new research suggests that it is linked to inflammatory responses, VE-cadherin alteration, apoptosis, or other cell death pathways (such as pyroptosis and autophagy), and oxidative stress.
Inflammatory responses
In patients with sepsis, the immune system is usually in disequilibrium. Antigen-presenting cells activate a variety of signaling pathways between immune cells under external and internal stimulations, resulting in the release of inflammatory mediators such as interleukin (IL)-1, IL-6, IL-8, tumor necrosis factor-alpha (TNF-α), and the release of pro-inflammatory signals accelerates the vascular endothelial dysfunction, which, in turn, promotes the inflow of inflammatory cells (such as neutrophils, macrophages, monocytes, lymphocytes, and lymphocytes), forming a vicious pro-inflammatory cycle, which ultimately aggravates and amplifies lung or systemic inflammation (6, 7). Therefore, pathogen-derived inflammatory mediators and activated immune cells not only trigger immunological responses but also cause host cell harm in sepsis.
The activation and destruction of endothelial cells can result in the production of pro-inflammatory signaling molecules (such as platelet-activating factor, angiopoietin 2, tumor necrosis factor, VE growth factor, inflammasome product IL-1, and others) and the accumulation of leukocytes, resulting in the recruitment of neutrophils and macrophages, activation of alveolar epithelial cells, and effector T cells (5, 8), Leukocyte aggregation is most commonly seen in the form of neutrophil-platelet aggregates, which have complicated thrombo-inflammatory properties (9). This can lead to increased protein permeability in the pulmonary vascular system, which can lead to hypovolemia and multiple organ failure. Neutrophil extracellular traps (NETs), for example, have been linked to the disruption of alveolar-capillary and epithelial barriers in recent research on acute lung damage and ARDS, as well as having inflammatory effects on the lung and other organs (10).
In addition, alveolar macrophages (AM) can cooperate with other immune cells to regulate lung inflammation, and AM cell death plays an important role in the development process of lung inflammation (11–14). On one hand, a variety of proinflammatory cytokines (e.g., inflammasomes and IL-1 β) can activate or amplify the lung injury response of macrophages, T cells, and other immune cells (15–17). On the other hand, AM cell death or pyroptosis promotes neutrophil migration into the lungs, increases the concentration of cytokines (e.g., IL-6, TNF-α, and IL-1β) in the alveoli, and aggravates lung injury (18). Thus, the interaction between inflammation and cell death is expected to further affect and accelerate the progression of ARDS.
In addition, the bacterial endotoxin lipopolysaccharide (LPS), which is a typical activator of sepsis-induced lung injury, can activate the overexpression and release of a variety of pro-inflammatory proteins, resulting in severe cellular or organ damage.
Disruption of VE-cadherin
The synergistic effect of VE-cadherin and endothelial receptor kinase (TIE2), which is regulated by VE protein tyrosine phosphatase, ensures the integrity of VE cells (VE-PTP) (4, 19, 20). Several variables influence and regulate the activity of VE-cadherin and the stability of adhesive junctions, including cytoskeletal interactions, GTPases, phosphorylation, and dephosphorylation (21). Dissociation of VE-PTP from VE-cadherin has been linked to enhanced alveolar-capillary permeability in inflammatory acute lung damage, as well as endothelial cell junction relaxation and inflammatory alveolar protein leakage, according to research (9, 22). The inflammatory response can destabilize VE-cadherin by releasing a range of small molecules (such as angiopoietin 2 and VE growth factor). Furthermore, actin filaments that generate tension and actin stress fibers that generate tension work together to influence the stability of VE cell connections (23, 24). Loss of intercellular adhesion during actomyosin contraction causes gaps to emerge between endothelial cells. These mechanisms combine to promote endothelial and epithelial permeability, which contributes to edematous fluid buildup and hypoxemia.
Apoptosis or other cell death pathways
The clinical significance of neutrophil apoptosis sensitivity in the etiology of ARDS is unknown. ARDS has been linked to the influx of neutrophils into the alveoli in several studies. Apoptotic neutrophils amass in the alveoli as a result of decreased AM proliferation, resulting in secondary necrosis and the release of inflammatory mediators (25, 26). In addition, the buildup of a significant number of neutrophils plays a key role in the release of additional inflammatory mediators and pro-inflammatory factors throughout the ALI process (27).
Necroptosis has been studied extensively as a crucial contributor to apoptosis in ARDS. Under the stimulation of a death signal, receptor-interacting protein kinase 1 (RIPK1) and RIPK3 govern necrotic cell death, which can be suppressed by necrostatin-1 (NEC-1) (28, 29). The presence of HMGB1 in bronchoalveolar lavage of patients with acute lung damage generally indicates necroptosis. Similarly, numerous intracellular bacteria and viruses elicit necroptosis in the lungs and have a role in sepsis-induced ARDS development (30).
Pyroptosis plays a role in the pathophysiology of ARDS as well. Lipopolysaccharide, which is primarily mediated by the cysteine protease (Caspase) family, affects endothelial cell pyroptosis in animal models (such as Caspase-1, Caspase-4, Caspase-11). Gasdermin D can be cleaved by activated Caspase-1 to generate the N-terminus or C-terminus of Gasdermin D, which is a direct executive protein of pyroptosis. Gasdermin D's N-terminus attaches to phospholipid proteins on the cell membrane, creating a hole that allows a flood of inflammatory substances to escape the cell (31).
In conclusion, apoptosis overexpression is important in the development of acute lung damage and ARDS.
Oxidative stress
Oxidative stress is frequently associated with ARDS. A large number of cytokines and inflammatory cells can be released during the inflammatory response of sepsis, and a large number of reactive oxygen species (ROS) can be generated through the oxidative stress response, causing varying degrees of damage to the structure and function of cells, such as mitochondrial damage (32). As per a past report, when cells are exposed to bacteria, leukocyte respiration increases, which kills the pathogens by producing ROS, superoxide, hydrogen peroxide, and hydroxyl radicals (33). NADPH oxidase (NOX) is an enzyme that uses NADPH to catalyze the reduction of oxygen to produce superoxide (34). NOX is commonly referred to as a “professional ROS producer”. Currently, seven NOX isoforms are known, namely, NOX1, NOX2, NOX3, NOX4, NOX5, Duox1, and Duox2 (35). Among these, only NOX1, NOX2, and NOX4 are expressed in the vasculature, and all of them have been implicated in ROS-mediated vascular diseases (36). The ROS produced by LPS exposure has been demonstrated to be NOX1-dependent in macrophages and NOX2-dependent in LPS- challenged lungs (37, 38). The LPS activating the toll-like receptor 4 (TLR4) receptor induces NOX-mediated ROS generation (39), which in turn activates pro-inflammatory signaling factors such as the TNF-α and NF-κB (40, 41). Protein interaction with C-kinase 1 (PICK1) affects pulmonary vascular glutathione synthesis by influencing the substrate-specific component xCT of the pulmonary cystine/glutamate transporter, resulting in severe oxidative stress, according to an animal study on sepsis (42).
Alveolar epithelial injury
One of the key hallmarks of ARDS is alveolar epithelial injury, and the severity of epithelial cell injury is a significant factor in ARDS severity.
The early stage of lung injury, commonly known as the exudative phase of ARDS, is characterized by innate immune cell-mediated disruption of the alveolar endothelial cell barrier and accumulation of protein-rich edema fluid in the alveolar interstitium and alveolus (5). Macrophages in the alveoli generate pro-inflammatory substances, which attract neutrophils, monocytes, and macrophages, as well as activate alveolar epithelial cells and effector T cells, causing inflammation and tissue damage (43). Second, alveolar endothelial cells with enhanced permeability allow proteins and fluids to collect in the pulmonary interstitium, resulting in interstitial edema. The edema fluid is transmitted to the alveolar fluid at this moment due to the alveolar epithelium's normal tight barrier being compromised (4).
Dissociation of intercellular junctions
When endothelial cells mount a proinflammatory or procoagulant response to infection in neighboring epithelial cells, the alveolar epithelial-endothelial barrier occurs independently of endothelial cells, according to Kirsty et al. (44). Barrier injury, on the other hand, is linked to the breakdown of epithelial cells' tight junctions. The alveolar epithelium's tight junctions are critical for regulating fluid in the lung's distal space, and transmembrane tight junction proteins called Claudins play a significant role (45).
Claudins 3, 4, 5, 7, 8, 15, and 18 were all expressed in the distal lung. Claudins 3, 4, and 7 are mostly found in alveolar type II cells, whereas claudin-5 is found in nearly all alveolar epithelial cells (46). The loss of the tight junction protein claudin-4 is one of them, and it's linked to barrier destruction (44). Claudin-5 disrupts the function of the alveolar epithelial barrier by interfering with the interaction of claudin-18 with the scaffold protein ZO-1 (45).
Epithelial cell death
Lung epithelial cells are usually regarded as the lung's first line of defense, and epithelial cell death is the most prominent aspect of alveolar damage in ARDS, which can be induced directly by bacterial and viral invasion, acidic media, hyperoxia, hypoxia, and mechanical alterations (47, 48). Inflammatory macrophages can promote cell death through methods such as the production of tumor necrosis factors and similar apoptosis-inducing ligands, while neutrophil-derived mediators can induce cell death through many pathways, including the release of TNF (49, 50).
Epigenetics
The term “epigenetics” describes the regulatory systems that manage gene expression but are unrelated to changes in the DNA sequence. These changes include non-coding RNA control of transcription, DNA methylation, and histone changes (51). The confluence of genetics and environment is where epigenetic alterations, which affect gene expression in response to external stress, occur. Epigenetic control may be the key factor in the pathogenesis of sepsis, as per several recent types of research on immunology and human sepsis (52).
Epigenetics and sepsis-related immune suppression
Anti-inflammatory and pro-inflammatory symptoms are present in the early stages of sepsis, but, as the condition progresses, immunosuppression frequently predominates, which increases the risk of subsequent infection and death. Immunosuppression induced by sepsis is a complicated phenomenon. Endotoxin tolerance or the body's inability to respond to bacterial endotoxin is one of the current markers of sepsis-associated immunosuppression. Numerous in vitro studies support the idea that epigenetic changes are essential for the development of endotoxin tolerance. In researches by El Gazzar et al. (53, 54), it was demonstrated that the TNF promoter was methylated in monocytes in the stationary phase. The TNF promoter is quickly demethylated upon initial endotoxin exposure, inducing an immunological response. However, the TNF promoter is bound by the histone methyltransferase G9a throughout the endotoxin-tolerance phase, resulting in recurrent methylation of the TNF promoter, which eventually renders the TNF promoter insensitive to endotoxin activation. miRNAs may also play a role in endotoxin tolerance, where miR-125b, miR-146a, miR-221, and miR-579 are involved in controlling the transcriptional expression of TNF (55, 56).
Epigenetics and ARDS
ARDS is a common complication of sepsis, and past studies have demonstrated that DNA methylation plays an important role in its pathogenesis. In an LPS-induced ARDS rat model, the level of 5-methylocytosine was increased, which confirmed the increased DNA methylation level (57). In an epigenomic analysis of lung tissues, more than 1,700 genes exhibited methylation differences (58). Of the 42 differential methylation genes associated with MAPK signaling, seven were found to be associated with ARDS (59). In their recent study, Chen et al. (60) confirmed the METTL3-mediated abnormal n6-methyladenosine mRNA expression in the septic lungs. Moreover, decreased METTL3 levels could exacerbate lung endothelial injury and inflammatory responses in sepsis-related ARDS. These findings provide a new direction in the research of whether sepsis-related ARDS can develop METTL3 as a biomarker or as a therapeutic intervention point.
Biomarkers of sepsis-related ARDS
A variety of biomarkers can be used to determine the severity of ARDS and the characteristics of each stage. The ideal biomarker would be based on the more precise pathophysiological pathways that have been investigated thus far. It must be extremely dependable, repeatable, disease-specific, and sensitive. The procedure is easy and low-cost in clinical practice, and short-term volatility must also be considered. Blood or plasma, urine, feces, bronchoalveolar lavage fluid, cerebrospinal fluid, bone marrow, and other clinical test specimens are currently used. It can reflect the damage or activation of epithelial cells, endothelial cells, or the coagulation system in the ARDS inflammatory response by detecting the change of a single biomarker in the specimen, which can aid in the diagnosis of the disease or the judgment of the curative effect in the treatment of the disease. Predict the present patient's cure rate or fatality rate.
Interleukin-6
Interleukin-6 (IL-6) was first discovered in 1986 under the name B cell-stimulating factor. T cells produce it, but it can also cause B cells to create antibodies. IL-6 has become a key inflammatory regulator since its discovery, and it is secreted by a variety of cells, the majority of which are found in inflammatory, infectious, and neoplastic disorders (61). IL-6 levels have been discovered to be elevated in critical conditions including sepsis and ARDS, and studies have demonstrated that it plays a key role in the disease's progression. Because IL-6 is required for clearing infections in the immune process and plays an active role as an anti-inflammatory or protective factor in most cases, future research can use IL-6 concentrations in the blood or lung as a biomarker to explain the current status of the disease.
Classical signaling and trans-signaling are the two basic types of IL-6 signaling. In recent years, a third transduction mechanism known as trans-presentation has been found. IL-6 forms the IL-6-IL-6R complex with membrane-bound IL-6R, which subsequently binds to gp130 to form signal transduction via the JAK-STAT pathway in traditional signaling. Only a few types of cells, most notably hepatocytes and some leukocytes such as macrophages and T-cell subsets, express IL-6R, but gp130 is expressed by all cell types. IL-6 does not bind to gp130 on its own; it must first form a complex with IL-6R. This signal transduction pathway is primarily responsible for IL-6's anti-inflammatory and antibacterial actions (62). IL-6R is cleaved off the cell surface and alternatively spliced to produce the soluble receptor sIL-6R in trans-signaling. The capacity of sIL-6R to bind to IL-6, and the resulting IL-6-sIL-6R complex to bind to gp130, can be performed on cells without IL-6R, extending the range of IL-6 on target cells and explaining IL-6's versatility. Protease disintegrin and metalloproteinase domain-containing protein 17 (ADAM-17), which is activated during inflammation or infection, is the major enzyme capable of completing this cleavage event, hence the pro-inflammatory effect is mostly mediated through trans-signaling (63). Trans-presentation is the third signal transduction mode. IL-6 is expected to be delivered to the plasma membrane after engaging with antigen-specific dendritic cells (DCs) and binding to IL-6R on DCs. Under the joint action of transforming growth factor-beta 2(TGF-2) and the IL-6-IL-6R complex on the surface of dendritic cells, it binds to gp130 on the surface of T cells and activates pathogenic Th17 cells (64).
In the course of sepsis or ARDS, IL-6 plays a significant role, and its management can have a favorable influence on the condition. The current study focuses on blocking IL-6, and IL-6R, neutralizing gp130, and interfering with JAK-STAT signaling, and it has yielded some promising results (62). The research on IL-6 as a biomarker in critical diseases, particularly ARDS and COVID-19, has made some headway. It is difficult to determine the concentration of circulating IL-6 and interpret the results. The cytokine peaks at different times in different disorders, making the sample time more restrictive. Changes in circadian rhythm, exercise, certain medicines, and immunometabolism comorbidities can all impact IL-6 levels and release in the bloodstream (65). There are further needs for sample processing, as il-6 and other cytokines are produced from blood cells over time, altering results (66). COVID-19 has swept the globe in the last 2 years, and its severe sufferers are likely to develop ARDS. Due to the lack of a common definition, some of the data gathered in the current COVID-19 research on IL-6 may have varied results. Part of the explanation for this disparity could be the use of clinically-based IL-6 tests, which are notoriously less sensitive than currently available research-grade assays. A recent series of cytokine-focused prospective studies in critically ill COVID-19 patients found that IL-6 concentrations were significantly higher than previously reported using clinical IL-6 measurements, both in absolute terms and relative to other inflammatory airway conditions like ARDS (67–69). IL-6 was found to act as an independent predictor of 28-day mortality in sepsis patients, showing superior predictive power to procalcitonin and hypersensitive C-responsive proteins as per meta-analysis. With the combined application of IL-6 and neutrophil-lymphocyte ratio as a predictive model, the predictive power of death risk in sepsis patients was significantly improved (70). This aspect benefits clinicians for more appropriate and accurate management of patients with sepsis.
Angiopoietin 2
Davis et al. (71) found the vascular receptor tyrosine kinase Tie-2 and its ligand angiopoietin 1 (Ang-1) in the mid-1990s and then used homology screening of a cDNA library to find angiopoietin 2 (Ang-2). Tie-2 receptors can bind to both Ang-1 and Ang-2. Tie-2 receptors are activated and phosphorylated after Ang-1 binds, promoting blood vessel integrity and growth. Ang-2 functions as an antagonist of Ang-1, binding to Tie-2 receptors competitively and blocking Ang-1's actions, boosting inflammatory responses and capillary leakage. Because Ang-2 is implicated in the pathophysiology of a variety of disorders, it could be used as a therapeutic target, and certain Ang-2-targeted therapies have been demonstrated to be effective (72, 73).
ARDS is a leading cause of morbidity and mortality in patients around the world, and despite effective antibiotic treatment, pathogen-body interactions can lead to increased pulmonary endothelial cell permeability, which can lead to protein exudation and edema, and eventually life-threatening lung failure. Ang-2 is the principal cause of enhanced permeability in lung endothelial cells. Ang-2 has been validated as a biomarker for sepsis and ARDS risk assessment in several prior studies (74, 75). Despite considerable knowledge of Ang-2's expression and activity in pulmonary circulation, its significance in the development of pneumonia and ARDS is unknown. Gutbier et al. (76) confirmed that Ang-2 levels were significantly greater in ARDS patients than in healthy people in a prospective analysis of two different cohorts and that using Ang-2 as a particular biomarker could improve the CURB-65/CRB-65 grading system accuracy. International recommendations indicate the CURB-65 and CRB-65 scores as predictors of pneumonia fatality (77). It was discovered that Ang-1, Ang-2, and its receptor Tie-2 were considerably expressed in the lung tissue of patients with pneumonia after evaluating the lung tissue of the deceased patient. The Ang-1 protein is found in a variety of types, including lung parenchymal cells, endothelial cells, and epithelial cells. Ang-2 and Tie-2 proteins, on the other hand, were only found in pulmonary VE cells (76). Villar et al. (78) discovered that Ang-2 plays an essential role in ARDS prediction in septic patients in a multicenter observational study in Spanish intensive care units. In a prospective study, Ang-2 demonstrated a large independent association between severe sepsis and organ injury (79). These studies implied that Ang-2—a biomarker of endothelial dysfunction and damage—may play an important role in future studies on predicting the treatment and prognosis of sepsis.
Receptor for advanced glycation end products
RAGE (receptor for advanced glycation end-products) is an immunoglobulin superfamily multi-ligand pattern recognition receptor. It is mostly found in membrane-bound and soluble forms (sRAGE). Membrane-bound forms can identify a wide range of receptors, activate transcription factors via binding to receptors, and enhance pro-inflammatory factor production. The soluble form is a decoy receptor that suppresses membrane RAGE activation competitively (80). RAGE is extensively expressed in lung tissue under normal circumstances (81). By evaluating the level of RAGE in bronchoalveolar lavage fluid and serum of rats and people with acute lung damage, Uchida et al. (82) confirmed that RAGE is a biomarker of type I alveolar epithelial cell injury and a significant inflammatory mediator as early as 2006. This is critical because epithelial cell injury and inflammatory responses are both involved in the ARDS process, and RAGE is involved in both of these routes (80). Following research, it was discovered that sRAGE is linked to the severity of ARDS (83–85).
Two subtypes of sARGE, known as cRAGE and esRAGE, have been separated in recent years (for endogenous secretory RAGE). To facilitate the shedding of sARGE, inflammatory factors are amplified and result in the formation of cRAGE, which is created on the surface of the cell membrane by proteolytic cleavage at the extracellular and transmembrane boundaries (86). Less than 25% of the total circulating sRAGE is created by alternate splicing of the RAGE pre-mRNA (87, 88), and the specific process governing esRAGE production is currently unknown. Studies have shown that increased sRAGE levels during acute illness predict 90-day death in ARDS patients (89). RAGE is thought to be substantially overexpressed in the lung epithelium and that RAGE signaling may play a key role in the clinical symptoms of lung injury (90). High levels of sRAGE are also linked to potential mortality in sepsis (91). Theoretically, respiratory virus illnesses like Covid-19, which are currently wreaking havoc worldwide, may also be connected. The next study objective may be to construct risk classification and sRAGE thresholds for sRAGE levels in clinical practice, which will help sRAGE as a biomarker to better serve the clinic.
In summary, past studies on the biomarkers of sepsis-related ARDS were mostly focused on two aspects: the biomarkers of VE injury and alveolar epithelial injury. The common ones included IL-6, Ang-2, and sRAGE. Despite the lack of any substantial evidence supporting the specificity of these biomarkers for such diseases, recent studies have hinted that the levels of these biomarkers are associated with an increased risk of sepsis-related ARDS development (92–94). In recent years, the researchers turn their attention to the genetic basis of these relationships. Recent studies suggest that genomic or transcriptome-based biomarkers may facilitate the establishment of predictive or prognostic stratification approaches for sepsis-associated ARDS and may, thereby, facilitate the development of novel therapeutic targets. For example, epigenetic variants and circulating microRNA have become potential biomarkers for the diagnosis or prognosis of sepsis-associated ARDS (95). However, they are possibly limited by various factors such as the sample size, ethnicity, and phenotypic heterogeneity. The current study did not detected any exact association of these novel biomarkers with sepsis-related ARDS (96). Nevertheless, this finding also provides a new direction for further research.
Conclusions
Sepsis-related ARDS is an inherently heterogeneous clinical syndrome. Several potential biomarkers have been investigated so far, with no single biomarker yet identified that can specifically reliably diagnose this disease. Current research indicates that biomarker combinations that respond to different aspects (such as epithelial and endothelial injury, epigenetic variation, and inflammation) are more likely to be applied in clinical settings. Some studies have suggested and tested the combination of several biomarkers to explore the relationship with sepsis-related ARDS (97–101), with some success. For example, Zhao et al. (98) validated an ARDS-mortality prediction model, including the age, surfactant protein D, and interleukin-8, which may be useful for risk assessment in clinical trial enrollment. However, none of these candidate research schemes has yet been clinically applied in such patients. It is therefore important to further study and clarify the potential of these candidate schemes.
Our knowledge of sepsis-related ARDS disorders has grown over the last two decades, and our capacity to detect and treat such patients has steadily increased, saving the lives of a huge number of patients. The death rate of sepsis-related ARDS patients, on the other hand, remains at the forefront of many diseases, and long-term consequences for surviving patients are also a serious issue. Further research in the following areas could assist enhance patient outcomes to change this predicament. Exploring strategies to reduce lung endothelial and epithelial cell damage and finding ways to promote lung endothelial and epithelial cell repair is necessary from a molecular standpoint. On the clinical side, we will actively investigate particular biomarkers closely associated with the disease so that the disease may be diagnosed early in clinical work and early treatment intervention can be carried out to prevent the disease from progressing further.
Author contributions
HG, YC, MC, and CL participated in the conception, drafting, and revision of the manuscript. All authors critically reviewed the paper. All authors have read and approved the final manuscript.
Funding
This work was supported by grants from the Hainan Province Science and Technology Special Fund (ZDKJ202004 and ZDKJ2021038), National Natural Science Foundation of China (81871611), and Finance Science and Technology Program of Sichuan Province (2022YFS0602).
Conflict of interest
The authors declare that the research was conducted in the absence of any commercial or financial relationships that could be construed as a potential conflict of interest.
Publisher's note
All claims expressed in this article are solely those of the authors and do not necessarily represent those of their affiliated organizations, or those of the publisher, the editors and the reviewers. Any product that may be evaluated in this article, or claim that may be made by its manufacturer, is not guaranteed or endorsed by the publisher.
References
1. Singer M, Deutschman CS, Seymour CW, Shankar-Hari M, Annane D, Bauer M, et al. The third international consensus definitions for sepsis and septic shock (Sepsis-3). J Am Med Assoc. (2016) 315:801–10. doi: 10.1001/jama.2016.0287
2. Park I, Kim M, Choe K, Song E, Seo H, Hwang Y, et al. Neutrophils disturb pulmonary microcirculation in sepsis-induced acute lung injury. Eur Respir J. (2019) 53:1800786. doi: 10.1183/13993003.00786-2018
3. Genga K, Russell J. Update of sepsis in the intensive care unit. J Innate Immun. (2017) 9:441–55. doi: 10.1159/000477419
4. Matthay MA, Zemans RL, Zimmerman GA, Arabi YM, Beitler JR, Mercat A, et al. Acute respiratory distress syndrome. Nat Rev Dis Prim. (2019) 5:18. doi: 10.1038/s41572-019-0069-0
5. Thompson BT, Chambers RC, Liu KD. Acute respiratory distress syndrome. N Engl J Med. (2017) 377:562–72. doi: 10.1056/NEJMra1608077
6. Hu Q, Hao C, Tang S. From sepsis to acute respiratory distress syndrome (ARDS): emerging preventive strategies based on molecular and genetic researches. Biosci Rep. (2020) 40:BSR20200830. doi: 10.1042/BSR20200830
7. Mehta Y, Dixit SB, Zirpe K, Sud R, Gopal PB, Koul PA, et al. Therapeutic approaches in modulating the inflammatory and immunological response in patients with sepsis, acute respiratory distress syndrome, and pancreatitis: an expert opinion review. Cureus. (2021) 13:e18393. doi: 10.7759/cureus.18393
8. Aggarwal NR, King LS, D'Alessio FR. Diverse macrophage populations mediate acute lung inflammation and resolution. Am J Physiol Lung Cell Mol Physiol. (2014) 306:L709–25. doi: 10.1152/ajplung.00341.2013
9. Ware LB, Matthay MA. The acute respiratory distress syndrome. J Clin Invest. (2012) 122:2731–40. doi: 10.1172/JCI60331
10. Lefrançais E, Mallavia B, Zhuo H, Calfee CS, Looney MR. Maladaptive role of neutrophil extracellular traps in pathogen-induced lung injury. JCI Insight. (2018) 3:e98178. doi: 10.1172/jci.insight.98178
11. Fan E, Erica KY, Fan J. Regulation of alveolar macrophage death in acute lung inflammation. Respir Res. (2018) 19:50. doi: 10.1186/s12931-018-0756-5
12. Rivera A, Siracusa MC, Yap GS, Gause WC. Innate cell communication kick-starts pathogen-specific immunity. Nat Immunol. (2016) 17:356–63. doi: 10.1038/ni.3375
13. Li Z, Scott MJ, Fan EK, Li Y, Liu J, Xiao G, et al. Tissue damage negatively regulates LPS-induced macrophage necroptosis. (2016) 23:1428–47. doi: 10.1038/cdd.2016.21
14. Yang J, Zhao Y, Zhang P, Li Y, Yang Y, Yang Y, et al. Hemorrhagic shock primes for lung vascular endothelial cell pyroptosis: role in pulmonary inflammation following LPS. Cell Death Dis. (2016) 7:e2363. doi: 10.1038/cddis.2016.274
15. Franklin BS, Bossaller L, De Nardo D, Ratter JM, Stutz A, Engels G, et al. The adaptor ASC has extracellular and 'prionoid' activities that propagate inflammation. Nat Immunol. (2014) 15:727–37. doi: 10.1038/ni.2913
16. Delaleu N, Bickel MJP. Interleukin-1 beta and interleukin-18: regulation and activity in local inflammation. Periodontology. (2004) 35:42–52. doi: 10.1111/j.0906-6713.2004.003569.x
17. Liu B, He R, Zhang L, Hao B, Jiang W, Wang W, et al. Inflammatory caspases drive pyroptosis in acute lung injury. Front Pharmacol. (2021) 12:631256. doi: 10.3389/fphar.2021.631256
18. He X, Qian Y, Li Z, Fan EK, Li Y, Wu L, et al. TLR4-upregulated IL-1β and IL-1RI promote alveolar macrophage pyroptosis and lung inflammation through an autocrine mechanism. Sci Rep. (2016) 6:31663. doi: 10.1038/srep31663
19. Frye M, Dierkes M, Küppers V, Vockel M, Tomm J, Zeuschner D, et al. Interfering with VE-PTP stabilizes endothelial junctions in vivo via Tie-2 in the absence of VE-cadherin. J Exp Med. (2015) 212:2267–87. doi: 10.1084/jem.20150718
20. Juettner VV, Kruse K, Dan A, Vu VH, Khan Y, Le J, et al. VE-PTP stabilizes VE-cadherin junctions and the endothelial barrier via a phosphatase-independent mechanism. J Cell Biol. (2019) 218:1725–42. doi: 10.1083/jcb.201807210
21. Giannotta M, Trani M, Dejana E. VE-cadherin and endothelial adherens junctions: active guardians of vascular integrity. Dev Cell. (2013) 26:441–54. doi: 10.1016/j.devcel.2013.08.020
22. Broermann A, Winderlich M, Block H, Frye M, Rossaint J, Zarbock A, et al. Dissociation of VE-PTP from VE-cadherin is required for leukocyte extravasation and for VEGF-induced vascular permeability in vivo. J Exp Med. (2011) 208:2393–401. doi: 10.1084/jem.20110525
23. Schnoor M, García Ponce A, Vadillo E, Pelayo R, Rossaint J, Zarbock A. Actin dynamics in the regulation of endothelial barrier functions and neutrophil recruitment during endotoxemia and sepsis. Cell Mol Life Sci. (2017) 74:1985–97. doi: 10.1007/s00018-016-2449-x
24. Jones J, Minshall R. Endothelial transcytosis in acute lung injury: emerging mechanisms and therapeutic approaches. Front Physiol. (2022) 13:828093. doi: 10.3389/fphys.2022.828093
25. Mahida RY, Scott A, Parekh D, Lugg ST, Hardy RS, Lavery GG, et al. Acute respiratory distress syndrome is associated with impaired alveolar macrophage efferocytosis. Eur Respir J. (2021) 58:2100829. doi: 10.1183/13993003.00829-2021
26. Mahida RY, Scott A, Parekh D, Lugg ST, Belchamber KB, Hardy RS, et al. In vitroassessment of alveolar macrophage dysfunction using an model of acute respiratory distress syndrome. Front Med. (2021) 8:737859. doi: 10.3389/fmed.2021.737859
27. Chopra M, Reuben JS, Sharma AC. Acute lung injury:apoptosis and signaling mechanisms. Exper Biol Med. (2009) 234:361–71. doi: 10.3181/0811-MR-318
28. Pan L, Yao DC Yu YZ, Li SJ, Chen BJ, Hu GH, et al. Necrostatin-1 protects against oleic acid-induced acute respiratory distress syndrome in rats. Biochem Biophys Res Commun. (2016) 478:1602–8. doi: 10.1016/j.bbrc.2016.08.163
29. Sauler M, Bazan IS, Lee PJ. Cell death in the lung: the apoptosis-necroptosis axis. Annu Rev Physiol. (2019) 81:375–402. doi: 10.1146/annurev-physiol-020518-114320
30. Rodrigue-Gervais IG, Labbé K, Dagenais M, Dupaul-Chicoine J, Champagne C, Morizot A, et al. Cellular inhibitor of apoptosis protein cIAP2 protects against pulmonary tissue necrosis during influenza virus infection to promote host survival. Cell Host Microbe. (2014) 15:23–35. doi: 10.1016/j.chom.2013.12.003
31. Mitra S, Exline M, Habyarimana F, Gavrilin MA, Baker PJ, Masters SL, et al. Microparticulate caspase 1 regulates gasdermin d and pulmonary vascular endothelial cell injury. Am J Respir Cell Mol Biol. (2018) 59:56–64. doi: 10.1165/rcmb.2017-0393OC
32. Joffre J, Hellman J. Oxidative stress and endothelial dysfunction in sepsis and acute inflammation. Antioxid Redox Signal. (2021) 35:1291–307. doi: 10.1089/ars.2021.0027
33. Ma H, Huang D, Guo L, Chen Q, Zhong W, Geng Q, et al. Strong correlation between lung ultrasound and chest computerized tomography imaging for the detection of acute lung injury/acute respiratory distress syndrome in rats. (2016) 8:1443–8. doi: 10.21037/jtd.2016.05.15
34. Ago T, Nunoi H, Ito T, Sumimoto H. Mechanism for phosphorylation-induced activation of the phagocyte NADPH oxidase protein p47(phox). Triple replacement of serines 303, 304, and 328 with aspartates disrupts the SH3 domain-mediated intramolecular interaction in p47(phox), thereby activating the oxidase. J Biol Chem. (1999) 274:33644–53. doi: 10.1074/jbc.274.47.33644
35. Geiszt, M. NADPH oxidases: new kids on the block. Cardiovasc Res. (2006) 71:289–99. doi: 10.1016/j.cardiores.2006.05.004
36. Pendyala S, Usatyuk PV, Gorshkova IA, Garcia JG, Natarajan V, Regulation of NADPH oxidase in vascular endothelium: the role of phospholipases, protein kinases, and cytoskeletal proteins. Antioxid Redox Signal. (2009) 11:841–60. doi: 10.1089/ars.2008.2231
37. Maitra U, Singh N, Gan L, Ringwood L, Li L. IRAK-1 contributes to lipopolysaccharide-induced reactive oxygen species generation in macrophages by inducing NOX-1 transcription and Rac1 activation and suppressing the expression of antioxidative enzymes. J Biol Chem. (2009) 284:35403–11. doi: 10.1074/jbc.M109.059501
38. Sato K, Kadiiska MB, Ghio AJ, Corbett J, Fann YC, Holland SM, et al. In vivo lipid-derived free radical formation by NADPH oxidase in acute lung injury induced by lipopolysaccharide: a model for ARDS. FASEB J. (2002) 16:1713–20. doi: 10.1096/fj.02-0331com
39. Imai Y, Kuba K, Neely GG, Yaghubian-Malhami R, Perkmann T, van Loo G, et al. Identification of oxidative stress and Toll-like receptor 4 signaling as a key pathway of acute lung injury. Cell. (2008). 133:235–49. doi: 10.1016/j.cell.2008.02.043
40. Wang Y, Hu H, Yin J, Shi Y, Tan J, Zheng L, et al. TLR4 participates in sympathetic hyperactivity Post-MI in the PVN by regulating NF-κB pathway and ROS production. Redox Biol. (2019) 24:101186. doi: 10.1016/j.redox.2019.101186
41. Zhang SS, Liu M, Liu DN, Yang YL, Du GH, Wang YH, TLR4-IN-C34 inhibits lipopolysaccharide-stimulated inflammatory responses via downregulating TLR4/MyD88/NF-κB/NLRP3 signaling pathway and reducing ROS generation in BV2 cells. Inflammation. (2022) 45:838–50. doi: 10.1007/s10753-021-01588-8
42. Qian M, Lou Y, Wang Y, Zhang M, Jiang Q, Mo Y, et al. PICK1 deficiency exacerbates sepsis-associated acute lung injury and impairs glutathione synthesis via reduction of xCT. Free Radic Biol Med. (2018) 118:23–34. doi: 10.1016/j.freeradbiomed.2018.02.028
43. Weichelt U, Cay R, Schmitz T, Strauss E, Sifringer M, Bührer C, et al. Prevention of hyperoxia-mediated pulmonary inflammation in neonatal rats by caffeine. Eur Respir J. (2013) 41:966–73. doi: 10.1183/09031936.00012412
44. Short KR, Kasper J, Van Der Aa S, Andeweg AC, Zaaraoui-Boutahar F, Goeijenbier M, et al. Influenza virus damages the alveolar barrier by disrupting epithelial cell tight junctions. Eur Respir J. (2016) 47:954–66. doi: 10.1183/13993003.01282-2015
45. Schlingmann B, Overgaard CE, Molina SA, Lynn KS, Mitchell LA, Dorsainvil White S, et al. Regulation of claudin/zonula occludens-1 complexes by hetero-claudin interactions. Nat Commun. (2016) 7:12276. doi: 10.1038/ncomms12276
46. Günzel D, Yu A. Claudins and the modulation of tight junction permeability. Physiol Rev. (2013) 93:525–69. doi: 10.1152/physrev.00019.2012
47. Burgoyne R, Fisher A, Borthwick L. The role of epithelial damage in the pulmonary immune response. Cells. (2021) 10:2763. doi: 10.3390/cells10102763
48. Budinger GS, Mutlu GM, Urich D, Soberanes S, Buccellato LJ, Hawkins K, et al. Epithelial cell death is an important contributor to oxidant-mediated acute lung injury. Am J Respir Crit Care Med. (2011) 183:1043–54. doi: 10.1164/rccm.201002-0181OC
49. Ye C, Li H, Bao M, Zhuo R, Jiang G, Wang W. Alveolar macrophage—derived exosomes modulate severity and outcome of acute lung injury. Aging. (2020) 12:6120–8. doi: 10.18632/aging.103010
50. Högner K, Wolff T, Pleschka S, Plog S, Gruber AD, Kalinke U, et al. Macrophage-expressed IFN-β contributes to apoptotic alveolar epithelial cell injury in severe influenza virus pneumonia. PLoS Pathog. (2013) 9:e1003188. doi: 10.1371/journal.ppat.1003188
51. Portela A, Esteller, M. Epigenetic modifications and human disease. Nat Biotechnol. (2010) 28:1057–68. doi: 10.1038/nbt.1685
52. Tollefsbol TO. Advances in Epigenetic Technology, Vol 791. Totowa, NJ: Humana Press (2011). p. 1–10. doi: 10.1007/978-1-61779-316-5_1
53. El Gazzar M, Liu T, Yoza BK. McCall CE. Dynamic and selective nucleosome repositioning during endotoxin tolerance. J Biol Chem. (2010) 285:1259–71. doi: 10.1074/jbc.M109.067330
54. El Gazzar M, Yoza BK, Chen X, Hu J, Hawkins GA, McCall CE. G9a and HP1 couple histone and DNA methylation to TNFalpha transcription silencing during endotoxin tolerance. J Biol Chem. (2008) 283:32198–208. doi: 10.1074/jbc.M803446200
55. El Gazzar M, McCall CE. MicroRNAs distinguish translational from transcriptional silencing during endotoxin tolerance. J Biol Chem. (2010) 285:20940–51. doi: 10.1074/jbc.M110.115063
56. El Gazzar M, Church A, Liu T, McCall CE. MicroRNA-146a regulates both transcription silencing and translation disruption of TNF-α during TLR4-induced gene reprogramming. J Leukocyte Biol. (2011) 90:509–19. doi: 10.1189/jlb.0211074
57. Shih CC, Liao MH, Hsiao TS, Hii HP, Shen CH, Chen SJ, et al. Procainamide inhibits DNA methylation and alleviates multiple organ dysfunction in rats with endotoxic shock. PLoS ONE. (2016) 11:e0163690. doi: 10.1371/journal.pone.0163690
58. Zhang XQ, Lv CJ, Liu XY, Hao D, Qin J, Tian HH, et al. Genome-wide analysis of DNA methylation in rat lungs with lipopolysaccharide-induced acute lung injury. Mol Med Rep. (2013) 7:1417–24. doi: 10.3892/mmr.2013.1405
59. Zhang R, Miao Q, Wang C, Zhao R, Li W, Haile CN, et al. Genome-wide DNA methylation analysis in alcohol dependence. Addict Biol. (2013) 18:392–403. doi: 10.1111/adb.12037
60. Chen Y, Wu Y, Zhu L, Chen C, Xu S, Tang D. Sepsis-induced acute respiratory distress syndrome. (2022) 13:897487. doi: 10.3389/fimmu.2022.897487
61. Garbers C, Heink S, Korn T, Rose-John S. Interleukin-6: designing specific therapeutics for a complex cytokine. Nat Rev Drug Discov. (2018) 17:395–412. doi: 10.1038/nrd.2018.45
62. McElvaney OJ, Curley GF, Rose-John S, McElvaney NG. Interleukin-6: obstacles to targeting a complex cytokine in critical illness. Lancet Respir Med. (2021) 9:643–54. doi: 10.1016/S2213-2600(21)00103-X
63. Heink S, Yogev N, Garbers C, Herwerth M, Aly L, Gasperi C, et al. Trans-presentation of IL-6 by dendritic cells is required for the priming of pathogenic T17 cells. Nat Immunol. (2017) 18:74–85. doi: 10.1038/ni.3632
64. Uciechowski P, Dempke W. Interleukin-6: a masterplayer in the cytokine network. Oncology. (2020) 98:131–7. doi: 10.1159/000505099
65. Nilsonne G, Lekander M, Åkerstedt T, Axelsson J, Ingre M. Diurnal variation of circulating interleukin-6 in humans: a meta-analysis. PLoS ONE. (2016) 11:e0165799. doi: 10.1371/journal.pone.0165799
66. Keustermans GC, Hoeks SB, Meerding JM, Prakken BJ, de Jager W. Cytokine assays: an assessment of the preparation and treatment of blood and tissue samples. Methods. (2013) 61:10–7. doi: 10.1016/j.ymeth.2013.04.005
67. Del Valle DM, Kim-Schulze S, Huang HH, Beckmann ND, Nirenberg S, Wang B, et al. An inflammatory cytokine signature predicts COVID-19 severity and survival. Nat Med. (2020) 26:1636–43. doi: 10.1038/s41591-020-1051-9
68. Sinha P, Calfee CS, Cherian S, Brealey D, Cutler S, King C, et al. Prevalence of phenotypes of acute respiratory distress syndrome in critically ill patients with COVID-19: a prospective observational study. Lancet Respir Med. (2020) 8:1209–18. doi: 10.1016/S2213-2600(20)30366-0
69. Remy KE, Mazer M, Striker DA, Ellebedy AH, Walton AH, Unsinger J, et al. Severe immunosuppression and not a cytokine storm characterizes COVID-19 infections. JCI Insight. (2020) 5. doi: 10.1172/jci.insight.140329
70. Liu S, Wang X, She F, Zhang W, Liu H, Zhao X, Effects of neutrophil-to-lymphocyte ratio combined with interleukin-6 in predicting 28-day mortality in patients with sepsis. Front Immunol. (2021). 12:639735. doi: 10.3389/fimmu.2021.639735
71. Davis S, Aldrich TH, Jones PF, Acheson A, Compton DL., Jain V, et al. Isolation of angiopoietin-1, a ligand for the TIE2 receptor, by secretion-trap expression cloning. Cell. (1996) 87:1161–9. doi: 10.1016/S0092-8674(00)81812-7
72. Lefere S, Van de Velde F, Hoorens A, Raevens S, Van Campenhout S, Vandierendonck A, et al. Angiopoietin-2 promotes pathological angiogenesis and is a therapeutic target in murine nonalcoholic fatty liver disease. Hepatology. (2019) 69:1087–104. doi: 10.1002/hep.30294
73. Monk BJ, Poveda A, Vergote I, Raspagliesi F, Fujiwara K, Bae DS, et al. Anti-angiopoietin therapy with trebananib for recurrent ovarian cancer (TRINOVA-1): a randomised, multicentre, double-blind, placebo-controlled phase 3 trial. Lancet Oncol. (2014) 15:799–808. doi: 10.1016/S1470-2045(14)70244-X
74. Calfee CS, Gallagher D, Abbott J, Thompson BT, Matthay MA, NHLBI ARDS. Network, plasma angiopoietin-2 in clinical acute lung injury: prognostic and pathogenetic significance. Crit Care Med. (2012) 40:1731–7. doi: 10.1097/CCM.0b013e3182451c87
75. Agrawal A, Matthay MA, Kangelaris KN, Stein J, Chu JC, Imp BM, et al. Plasma angiopoietin-2 predicts the onset of acute lung injury in critically ill patients. Am J Respir Crit Care Med. (2013) 187:736–42. doi: 10.1164/rccm.201208-1460OC
76. Gutbier B, Neuhauß AK, Reppe K, Ehrler C, Santel A, Kaufmann J, et al. Prognostic and pathogenic role of angiopoietin-1 and−2 in pneumonia. Am J Respir Crit Care Med. (2018) 198:220–31. doi: 10.1164/rccm.201708-1733OC
77. Ewig. Management-based risk prediction in community-acquired pneumonia by scores and biomarkers. Eur Respir J. (2013) 41:974–84. doi: 10.1183/09031936.00104412
78. Villar J, Herrán-Monge R, González-Higueras E, Prieto-González M, Ambrós A, Rodríguez-Pérez A, et al. Clinical and biological markers for predicting ARDS and outcome in septic patients. Sci Rep. (2021) 11:22702. doi: 10.1038/s41598-021-02100-w
79. Yu WK, McNeil JB, Wickersham NE, Shaver CM, Bastarache JA, Ware LB, Angiopoietin-2 outperforms other endothelial biomarkers associated with severe acute kidney injury in patients with severe sepsis and respiratory failure. Crit Care. (2021) 25:48. doi: 10.1186/s13054-021-03474-z
80. Guo WA, Knight PR, Raghavendran K. The receptor for advanced glycation end products and acute lung injury/acute respiratory distress syndrome. Intens Care Med. (2012) 38:1588–98. doi: 10.1007/s00134-012-2624-y
81. Fehrenbach H, Kasper M, Tschernig T, Shearman MS, Schuh D, Müller M. Receptor for advanced glycation endproducts (RAGE) exhibits highly differential cellular and subcellular localisation in rat and human lung. Cell Mol Biol (Noisy-le-grand). (1998) 44:1147–57.
82. Uchida T, Shirasawa M, Ware LB, Kojima K, Hata Y, Makita K, et al. Receptor for advanced glycation end-products is a marker of type I cell injury in acute lung injury. Am J Respir Crit Care Med. (2006) 173:1008–15. doi: 10.1164/rccm.200509-1477OC
83. Zhang H, Tasaka S, Shiraishi Y, Fukunaga K, Yamada W, Seki H, et al. Role of soluble receptor for advanced glycation end products on endotoxin-induced lung injury. Am J Respir Crit Care Med. (2008) 178:356–62. doi: 10.1164/rccm.200707-1069OC
84. Su X, Looney MR, Gupta N, Matthay MA. Receptor for advanced glycation end-products (RAGE) is an indicator of direct lung injury in models of experimental lung injury. Am J Physiol Lung Cell Mol Physiol. (2009) 297:L1–5. doi: 10.1152/ajplung.90546.2008
85. Jabaudon M, Blondonnet R, Roszyk L, Bouvier D, Audard J, Clairefond G, et al. Soluble receptor for advanced glycation end-products predicts impaired alveolar fluid clearance in acute respiratory distress syndrome. Am J Respir Crit Care Med. (2015) 192:191–9. doi: 10.1164/rccm.201501-0020OC
86. Zhang L, Bukulin M, Kojro E, Roth A, Metz VV, Fahrenholz F, et al. Receptor for advanced glycation end products is subjected to protein ectodomain shedding by metalloproteinases. J Biol Chem. (2008) 283:35507–16. doi: 10.1074/jbc.M806948200
87. Hudson BI, Carter AM, Harja E, Kalea AZ, Arriero M, Yang H, et al. Identification, classification, and expression of RAGE gene splice variants. FASEB J. (2008) 22:1572–80. doi: 10.1096/fj.07-9909com
88. Yonekura H, Yamamoto Y, Sakurai S, Petrova RG, Abedin MJ., Li H, et al. Novel splice variants of the receptor for advanced glycation end-products expressed in human vascular endothelial cells and pericytes, and their putative roles in diabetes-induced vascular injury. Biochem J. (2003) 370:1097–109. doi: 10.1042/bj20021371
89. Jabaudon M, Blondonnet R, Pereira B, Cartin-Ceba R, Lichtenstern C., Mauri T, et al. Plasma sRAGE is independently associated with increased mortality in ARDS: a meta-analysis of individual patient data. Intensive Care Med. (2018) 44:1388–99. doi: 10.1007/s00134-018-5327-1
90. Erusalimsky, J. The use of the soluble receptor for advanced glycation-end products (sRAGE) as a potential biomarker of disease risk and adverse outcomes. Redox Biol. (2021) 42:101958. doi: 10.1016/j.redox.2021.101958
91. Brodska H, Malickova K, Valenta J, Fabio A, Drabek T. Soluble receptor for advanced glycation end products predicts 28-day mortality in critically ill patients with sepsis. Scand J Clin Lab Invest. (2013) 73:650–60. doi: 10.3109/00365513.2013.849357
92. Van Der Zee P, Rietdijk W, Somhorst P, Endeman H, Gommers D. A systematic review of biomarkers multivariately associated with acute respiratory distress syndrome development and mortality. Crit Care. (2020) 24:243. doi: 10.1186/s13054-020-02913-7
93. Jones TK, Feng R, Kerchberger VE, Reilly JP, Anderson BJ, Shashaty MG, et al. Plasma sRAGE acts as a genetically regulated causal intermediate in sepsis-associated acute respiratory distress syndrome. Am J Respir Crit Care Med. (2020) 201:47–56. doi: 10.1164/rccm.201810-2033OC
94. Reilly JP, Wang F, Jones TK, Palakshappa JA, Anderson BJ, Shashaty MG, et al. Plasma angiopoietin-2 as a potential causal marker in sepsis-associated ARDS development: evidence from Mendelian randomization and mediation analysis. Intens Care Med. (2018) 44:1849–58. doi: 10.1007/s00134-018-5328-0
95. Zheng F, Pan Y, Yang Y, Zeng C, Fang X, Shu Q, et al. Novel biomarkers for acute respiratory distress syndrome: genetics, epigenetics and transcriptomics. Biomark Med. (2022) 16:217–31. doi: 10.2217/bmm-2021-0749
96. Beitler JR, Thompson BT, Baron RM, Bastarache JA, Denlinger LC, Esserman L, et al. Advancing precision medicine for acute respiratory distress syndrome. Lancet Respir Med. (2022) 10:107–20. doi: 10.1016/S2213-2600(21)00157-0
97. Calfee CS, Ware LB, Glidden DV, Eisner MD, Parsons PE, Thompson BT, et al. Use of risk reclassification with multiple biomarkers improves mortality prediction in acute lung injury. Crit. Care Med. (2011) 39:711–7. doi: 10.1097/CCM.0b013e318207ec3c
98. Zhao Z, Wickersham N, Kangelaris KN, May AK, Bernard GR, Matthay MA, et al. External validation of a biomarker and clinical prediction model for hospital mortality in acute respiratory distress syndrome. Intens Care. (2017) 43:1123–31. doi: 10.1007/s00134-017-4854-5
99. Chen C, Shi L, Li Y, Wang X, Yang S. Disease-specific dynamic biomarkers selected by integrating inflammatory mediators with clinical informatics in ARDS patients with severe pneumonia. Cell Biol Toxicol. (2016) 32:169–84. doi: 10.1007/s10565-016-9322-4
100. Ding XF, Li JB, Liang HY, Wang ZY, Jiao TT, Liu Z, et al. Predictive model for acute respiratory distress syndrome events in ICU patients in China using machine learning algorithms: a secondary analysis of a cohort study. J Transl Med. (2019) 17:326. doi: 10.1186/s12967-019-2075-0
101. Sinha P, Delucchi KL, McAuley DF, O'Kane CM, Matthay MA, Calfee CS, et al. Development and validation of parsimonious algorithms to classify acute respiratory distress syndrome phenotypes: a secondary analysis of randomised controlled trials. Lancet Respir Med. (2020) 8:247–57. doi: 10.1016/S2213-2600(19)30369-8
Keywords: sepsis, acute respiratory distress syndrome - ARDS, epithelial injury, endothelial injury, mechanism, biomarkers
Citation: Gong H, Chen Y, Chen M, Li J, Zhang H, Yan S and Lv C (2022) Advanced development and mechanism of sepsis-related acute respiratory distress syndrome. Front. Med. 9:1043859. doi: 10.3389/fmed.2022.1043859
Received: 14 September 2022; Accepted: 31 October 2022;
Published: 14 November 2022.
Edited by:
Shuibang Wang, National Institutes of Health (NIH), United StatesReviewed by:
Francesco Forfori, University of Pisa, ItalySanda Predescu, Rush University Medical Center, United States
Copyright © 2022 Gong, Chen, Chen, Li, Zhang, Yan and Lv. This is an open-access article distributed under the terms of the Creative Commons Attribution License (CC BY). The use, distribution or reproduction in other forums is permitted, provided the original author(s) and the copyright owner(s) are credited and that the original publication in this journal is cited, in accordance with accepted academic practice. No use, distribution or reproduction is permitted which does not comply with these terms.
*Correspondence: Chuanzhu Lv, bHZjaHVhbnpodTY3NyYjeDAwMDQwOzEyNi5jb20=
†These authors have contributed equally to this work and share first authorship