- 1Lawrence Berkeley National Laboratory, Chemical Sciences Division, Berkeley, CA, United States
- 2Department of Nuclear Engineering, University of California, Berkeley, Berkeley, CA, United States
Targeted alpha therapy is an oncological treatment, where cytotoxic doses of alpha radiation are locally delivered to tumor cells, while the surrounding healthy tissue is minimally affected. This therapeutic strategy relies on radiopharmaceuticals made of medically relevant radionuclides chelated by ligands, and conjugated to targeting vectors, which promote the drug accumulation in tumor sites. This review discusses the state-of-the-art in the development of radiopharmaceuticals for targeted alpha therapy, breaking down their key structural components, such as radioisotope, targeting vector, and delivery formulation, and analyzing their pros and cons. Moreover, we discuss current drawbacks that are holding back targeted alpha therapy in the clinic, and identify ongoing strategies in field to overcome those issues, including radioisotope encapsulation in nanoformulations to prevent the release of the daughters. Lastly, we critically discuss potential opportunities the field holds, which may contribute to targeted alpha therapy becoming a gold standard treatment in oncology in the future.
1 Introduction
Therapeutic agents based on radionuclides hold great potential in oncology, as they allow to deliver highly cytotoxic doses of ionizing radiation to cancer cells, while minimizing damage to surrounding tissue (1, 2). Hence, targeted radiotherapy has been proposed to treat a wide range of cancers, including micrometastases, and tumors resistant to other treatments (3). Although targeted radiotherapy has been primarily explored in oncology, other potential uses include the treatment of viral and bacterial infections (4–7). In order to deliver the radioactive dose to the tumor site and spar healthy tissue, the radionuclides are complexed by chelating agents conjugated to targeting moieties, such as monoclonal antibodies (Figure 1) (1). To date, the U.S. Food and Drug administration (FDA) has approved two radioimmunoconjugate drugs to treat non-Hodgkin lymphoma: 90Y-ibritumomab tiuxetan and 131I-tositumomab, sold under the commercial names of Zevalin and Bexxar, respectively (8, 9), which rely on β-particle emissions. The latter was discontinued in 2014 due to manufacturing and commercial issues. Furthermore, the FDA and the European Medicines Agency (EMA) have also approved two targeting radiotherapeutics based on small molecules or peptides. The two radiopharmaceuticals are 177Lu-vipivotide tetraxetan (previously known as 177Lu-PSMA-617, and sold under the commercial name of Pluvicto) and 177Lu-oxodotreotide (sold under the commercial name of Lutathera) for the treatment of prostate-specific membrane antigen (PSMA)-positive metastatic castration-resistant prostate cancers and certain digestive tract cancers, respectively (10, 11).
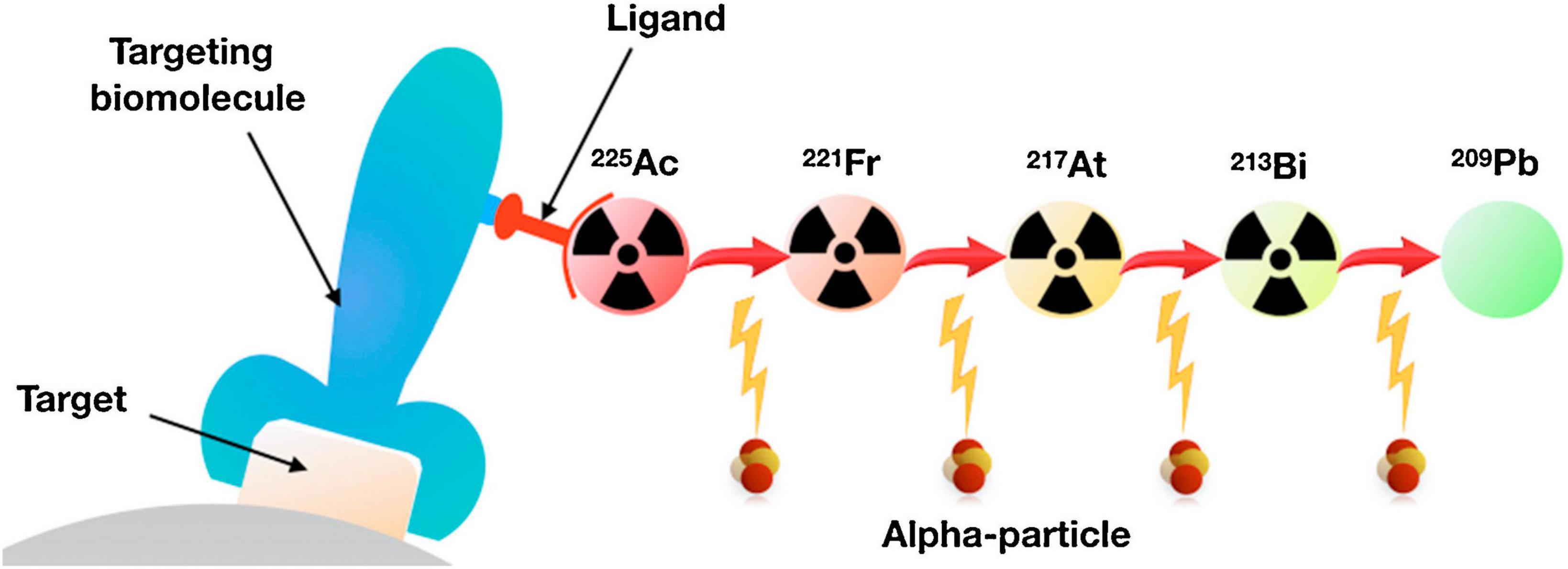
Figure 1. Schematic representation of targeted radiotherapy. In targeted alpha therapy, the targeting vector is conjugated with a ligand chelating an α-emitter, such as 225Ac, which emits four α-particles down to stable 209Pb. Adapted with permission from ref 2. Copyright 2018 Elsevier.
The early success of β-emitters in cancer therapeutics has brought attention to α-emitting radionuclides, since they can potentially deliver greater and more confined cytotoxic dose. α-particles have higher linear energy transfer (50–230 keV/μm), which causes DNA break clusters, compared to β-particles (0.2 keV/μm), which yield individual and repairable DNA lesions (12). Studies with new-generation microbeam devices have further demonstrated that α-radiation cytotoxicity is also mediated by disruptions of other subcellular targets beyond the nucleus, including mitochondria, lysosomes, and cell membranes (13). In addition to the direct cytotoxicity of α-particles, α-emitters also cause biological effects through immunological and bystander effects (14, 15). Despite some of these mechanisms of action, such as bystander effects, depend on the generation of reactive oxygen species (16), high linear energy transfer radiation is less sensitive to oxygenation level and cell proliferation. Hence, the damage caused by α-emitters is more difficult to overcome than the one caused by β-emitters. Moreover, α-particles have shorter path lengths in biological tissues than β-particles do (50–100 and 1,000–10,000 μm, respectively), limiting the delivered dose to a narrower region (17). Hence, the dose confinement of α-particles may help to minimize cytotoxic damage outside the tumor region. Currently, only one α-emitting agent has been approved by the FDA, namely radium-223 dichloride, sold under the commercial name of Xofigo (formerly Alpharadin) (18). 223RaCl2 is used for the treatment of prostate cancer with metastatic bone disease, however, it does not rely on targeting agents to be delivered to the tumor site. Instead, radium acts as a calcium-mimetic cation that binds to hydroxyapatite deposition regions, including bone metastases in prostate cancer patients (19, 20). Although β-emitters, such as 89Sr and 153Sm, only provide pain palliation in bone-metastatic prostate cancer patients (21, 22), 223Ra yields both survival benefits as well as pain reduction when added to best standard of care (23, 24). Thus, 223Ra results exemplify the benefits of α-radiation compared to β-radiation in oncological settings.
Regarding targeted alpha therapy, early works focused on evaluating the therapeutic performance of immunoconjugates chelating single α-emitting isotopes (25). In recent years, however, radionuclides that emit multiple α-particles in the decay chain have received increasing interest, since they act as in vivo α-generators, enhancing the delivered dose (26). Hence, most ongoing clinical trials explore the use of radiopharmaceuticals radiolabeled with 225Ac or 227Th (Table 1). Although pre-clinical and clinical studies have highlighted the therapeutic benefits of these conjugates, there are still challenges that need to be solved, such as kinetics and stability of the complexes (27), and retention of the daughters (28, 29). In an α-decay, the recoiling daughter breaks the chemical bonds by which it is bound to the ligand. Free radioisotopes and metals, particularly f-block elements, have high binding affinities for biological receptors (30, 31), resulting in their endogenous chelation and subsequent deposition in tissues (32, 33). Internal contamination with radiometals (and their decay products) cause metal- and radiotoxicity, as multiple biological processes get disrupted (34–39). Therefore, minimizing daughter release is a fundamental step to extend the use of targeted alpha therapy beyond metastatic non-responding patients (28). While the design of new ligands is unlikely to solve the recoil issue (as the energies that the chelators need to withstand are too large), new nanoformulations, which encapsulate the α-emitting isotopes, have shown to enhance daughter retention (40, 41).
In this review, we analyze the current progress in the development of radiopharmaceuticals for targeted alpha therapy, from in vitro studies to clinical settings. We describe different oncological challenges this therapy tries to overcome, and then identify the design principles of radiopharmaceuticals that allow to overcome those issues, including targeting vectors, radionuclides, and delivery formulations. Finally, we discuss future research opportunities that the field may hold as well as challenges that need to be solved before targeted alpha therapy becomes a mainstream treatment in the clinic.
2 Radionuclides used in targeted alpha therapy
2.1 Radium-223
It is the first α-emitting isotope FDA-approved to treat cancer (18). 223Ra has a half-life of 11.4 days, and it can be obtained from 227Ac generators (42). It emits four α-particles and two β-particles as part of its decay chain down to stable 207Pb. The multiple α-emissions provide higher cytotoxic (and potentially therapeutic) effects, but they make the radiolabeling more challenging. One of its main drawbacks is the generation of 219Rn gas during its decay (43, 44).
2.2 Actinium-225
It is a radionuclide with a half-life of 10 days that produces six daughters during its decay chain down to stable 209Bi (12). Each 225Ac decay results on four α-emissions with energies between 5.8 and 8.4 MeV. 225Ac is advantageous over the FDA-approved 223Ra because it does not emit high-energy Ɣ-radiation. Furthermore, the half-life in the several days range allows for radionuclide central production and subsequent distribution, rather than on-site generation. Current 225Ac use in targeted alpha therapy is limited by the lack of chelating ligands capable of withstand the recoil energies (45).
2.3 Thorium-227
It is a radionuclide with a half-life of 18.7 days that decays to 223Ra through α-emission (46). Because the decay chain of 227Th contains five α-emissions, the radionuclide can act as in vivo α-generator during targeted alpha therapy, enhancing the dose delivered to the tumors (26). As in the case of 225Ac, applications of 227Th are limited by the capacities of the chelating agents to withstand the high recoil energies.
2.4 Bismuth-212 and -213
212Bi is a radioisotope with a half-life of 60.55 min that decays down to stable 208Pb through 208Tl (36%) and 212Po (64%) (12), with both decay routes emitting α- and β-particles. 212Bi is obtained through the decay of 228Th, but its short half-life complicates the radiolabeling process and sample preparation. Nevertheless, recent developments in 224Ra (half-life of 3.6 days) generators have partially overcome this issue, since they can produce both 212Pb and 212Bi with good yields (47). In addition to 212Bi short half-life, the radionuclide is also challenging to work with because one of its daughters (208Tl) emits high energy Ɣ-radiation (2.6 MeV).
Regarding 213Bi, it has a half-life of 45.6 min and can be produced in an 225Ac and 213Bi generator, which yields clinically useful radionuclide for 10 days (12). As in the previous radioisotope in this list, 213Bi short half-life also limits the preparation of therapeutic radioimmunoconjugates (48). Nevertheless, 213Bi decay chain includes a 440 keV Ɣ-emission that can be used to image tumor uptake and calculate dosimetry (49).
2.5 Lead-212
It is a radionuclide with a half-life of 10.6 h, which is produced during the 228Th decay, and commonly obtained from 224Ra generators (47). 212Pb is a β-emitter that serves as in vivo generator of the clinically relevant 212Bi, extending the use of the latter beyond its 60.55 min half-life (50). Simulations based on a Monte Carlo model showed that 212Pb and 225Ac have similar relative biological effectiveness when considering the entire decay chains (51). 212Pb, however, presents similar challenges that 212Bi does, such as a decay chain that contains a daughter (208Tl) that emits high energy Ɣ-radiation (2.6 MeV) (52).
2.6 Astatine-211
Astatine is the heaviest naturally occurring halogen, and one of its isotopes (211At) was proposed, more than fifty years ago, as substitute of iodine isotopes during specific inactivation of sensitized lymphocytes (53). 211At decay is branched, where the first path (42%) is by α-emission, resulting in the production of 207Bi, which is followed by electron capture to stable 207Pb (12). The second route (58%) is by electron capture, yielding 211Po, followed by emission of α-particles to stable 207Pb. The radiological properties of 211At are favorable for targeted alpha therapy, since the half-life of 211At is 7.2 h [long enough for most radiolabeling procedures to obtain the radioimmunoconjugates (12)], more than 99% of 211At radiation energy originates from α-emissions (54), and one of its daughters (211Pu) emits X-rays (77–92 keV), which can be used for imaging (55). While the other radioisotopes used for targeted alpha therapy are metals and their radiolabeling rely on metalation processes, At is a halogen. Hence, 211At radiolabeling is based on reactions with stannyl derivatives, iodonium salts, or boronic derivatives, among others (56). Nevertheless, 211At use in targeted alpha therapy is limited by its low availability and supply (57).
3 Dosimetry
Although dosimetry was initially developed for protection against radiation (58), nowadays it is also used for optimization of radiotherapy. The biological effect of radiation depends on the absorbed dose, which is defined as the amount of energy absorbed per unit of tissue mass (59), the fractionation and the spread of the exposure, among others (60, 61). While radiobiology and dosimetry for external-beam radiotherapy are well-established, their direct extrapolation to targeted radiotherapy is problematic, as the characteristics of the latter are rather different (i.e., mixed and heterogeneous irradiation, long exposure times, and low absorbed dose rates) (62). Therefore, new radiobiological understanding and dosimetry tools specific to targeted radiotherapy are necessary. In this regard, despite patient-specific dosimetry is slowly being implemented in clinical settings (63), radiobiological knowledge is still lacking to meet certain clinical needs (64). To that end, recent coordinated efforts are calling to collectively promote and foster advances in radiobiology with the aim to improve targeted radiotherapy outcomes (64).
In the case of targeted alpha therapy, dosimetry calculations are more challenging than in other types of targeted radiotherapy, since the daughters need to be considered, and they may have different pharmacokinetic profiles and chemical properties (65). Hence, each decay down to the stable isotope needs to be assessed. Roeske et al. developed a model that predicts dosimetry of α-emitters, which takes into consideration multiple factors, including the decay site, the daughter half-lives and their potential biodistribution, the blood time, and the tumor uptake (65). Because most of radionuclides used in targeted alpha therapy emit Ɣ-radiation (or some characteristic x-ray or bremsstrahlung radiation), biodistribution and pharmacokinetic information can be obtained for dosimetry calculations through clinical imaging (25). The spatial resolution of those images, however, tends to be poor, due to the injected activity for targeted alpha therapy is lower than the one used for imaging, yielding subpar signal-to-noise ratios (25, 66).
4 Radiopharmaceutical development: From pre-clinical to clinical studies
In targeted alpha therapy, the radionuclides are delivered to cancer cells through a wide variety of formulations. Most radiopharmaceuticals are made of radiolabeled antibodies, peptides, or small targeting molecules (Figure 2) (26, 67). A recent strategy includes incorporating the radionuclides into liposomes or nanoconstructs as a mean to enhance tumor uptake and decrease daughters redistribution (41). The therapeutic performance of these nanoformulations, however, has only been studied in pre-clinical settings, and no clinical trials have been performed.
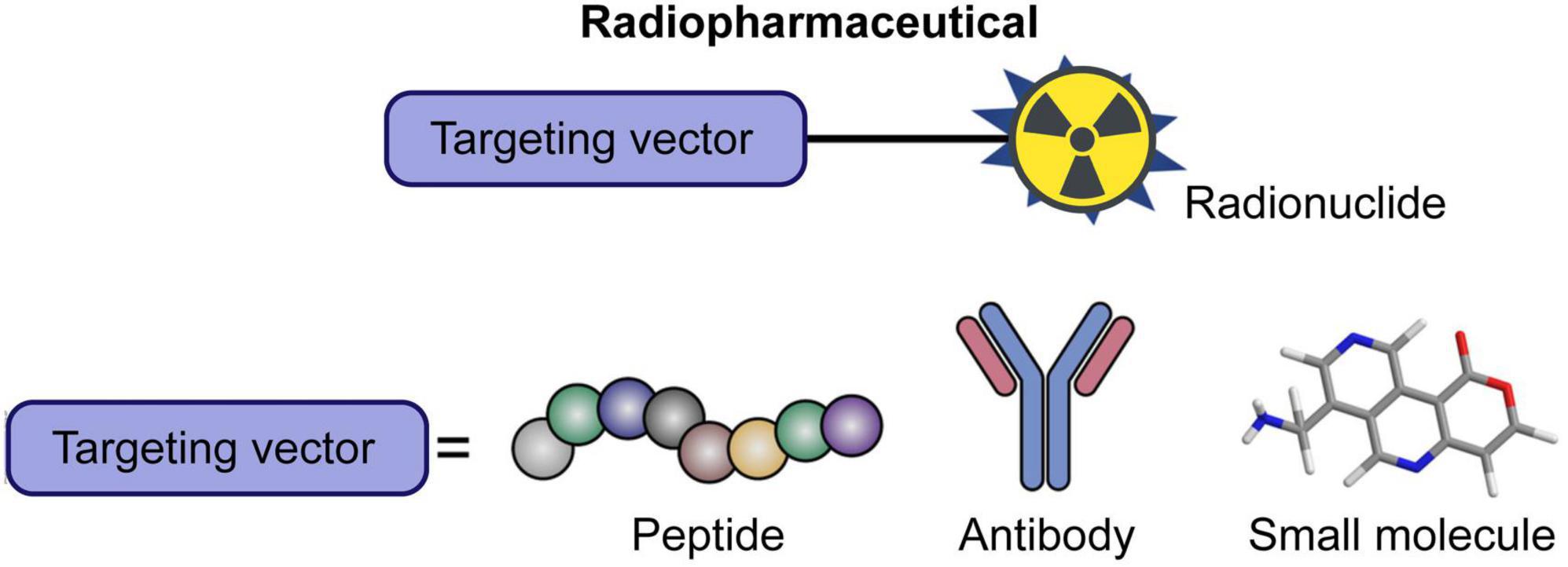
Figure 2. Schematic representation of radiopharmaceutical for targeted alpha therapy. The main targeting vectors are peptides, antibodies, and small molecules. Modified with permission from ref 45. Copyright 2020 Frontiers Media S.A.
4.1 Small-molecule targeting
Over the last decade, increasing number of studies have focused on the use of small-molecule radiopharmaceuticals for targeting overexpressed antigens in cancer cells. For instance, PSMA is a type II membrane protein with enzymatic activity that is overexpressed on the cell membrane of aggressive prostate cancer and other solid tumors (68). Hence, PSMA is commonly targeted to deliver imaging and therapeutic agents to PSMA-expressing tumoral cells (69, 70). In the case of radiopharmaceuticals, PSMA-617 and PSMA I&T are two small-molecules that act as PSMA inhibitors, which are frequently radiolabeled with clinically relevant radionuclides (71, 72). For example, PSMA-617 labeled with 177Lu (β-emitter, half-life of 6.7 days) has shown promising therapeutic responses against metastatic castration-resistant prostate cancer in a phase II clinical trial (NCT03392428) (73), and is currently undergoing a phase III clinical trial (NCT03511664). The promising results with β-emitters promoted new research efforts toward developing PSMA-based alpha therapy. For instance, PSMA-617 radiolabeled with 225Ac showed therapeutic benefits in patients refractory to 177Lu-PSMA-617 (74, 75). The first clinical PSMA-based targeted alpha therapy study was published in 2016, where two patients with metastatic castration-resistant prostate cancer with challenging clinical situations received 225Ac-PSMA-617 (100 kBq/Kg) every 2 weeks (74). The first patient presented diffuse red marrow infiltration, yielding him unsuitable for 177Lu-PSMA-617 treatment, and the second one was resistant to the 177Lu radiopharmaceutical. Both patients experienced significant improvements after 225Ac-PSMA-617 treatments with prostate-specific antigen decreasing below measurable levels in serum, and complete response based on clinical imaging (Figure 3). It is worth highlighting that blood analysis and/or functional imaging (e.g., 18F-fluorodeoxyglucose-based positron emission tomography, PET) are important to characterize the therapeutic response after targeted radiotherapy, as the surface receptors may be downregulated after therapy, impairing molecular imaging. Furthermore, no hematologic toxicity was reported, and the only meaningful side effect was xerostomia. Based on the positive results, the same authors did a follow up study with 14 metastatic castration-resistant prostate cancer patients to optimize the treatment dose (76). For advanced-stage patients, a cycle of 225Ac-PSMA-617 (100 kBq/Kg) every 8 weeks showed the most optimal response when considering both therapeutic performance and toxicity. As the previous study, severe xerostomia was the dose-limiting side effect. Since then, the standardized treatment of 225Ac-PSMA-617 has been applied as last-line therapy to end-stage metastatic castration-resistant prostate cancer patients in other studies (75, 77). In one of those studies, 82% of chemotherapy-naïve patients showed above 90% serum prostate specific antigen decline, including 41% of patients having undetectable serum antigen levels, and remained in remission 12 months after the treatment (75). The first clinical data of 225Ac-PSMA-I&T have been published, showing comparable biochemical responses to 225Ac-PSMA-617 during the treatment of metastatic castration-resistant prostate cancer patients (78, 79). Recently, a pilot study with patients with metastatic prostate cancer, who received 225Ac-PSMA-617, reported two cases where patients developed 225Ac therapy-associated chronic kidney disease (80). Both patients had prior impaired renal function, which worsened after 225Ac therapy. This study highlighted the need to carefully assess and monitor kidney function on patients receiving 225Ac- PSMA-617, specially in cases with preexisting kidney impairment. An alternative to decrease therapy-associated toxicity, particularly xerostomia, is a tandem protocol, where both 225Ac-PSMA-617 and 177Lu-PSMA-617 are co-administrated. Tandem targeted therapy has shown similar initial response rates than 225Ac-PSMA-617 monotherapy (81), however, it still has stronger side effects compared to 177Lu-PSMA-617 monotherapy (82).
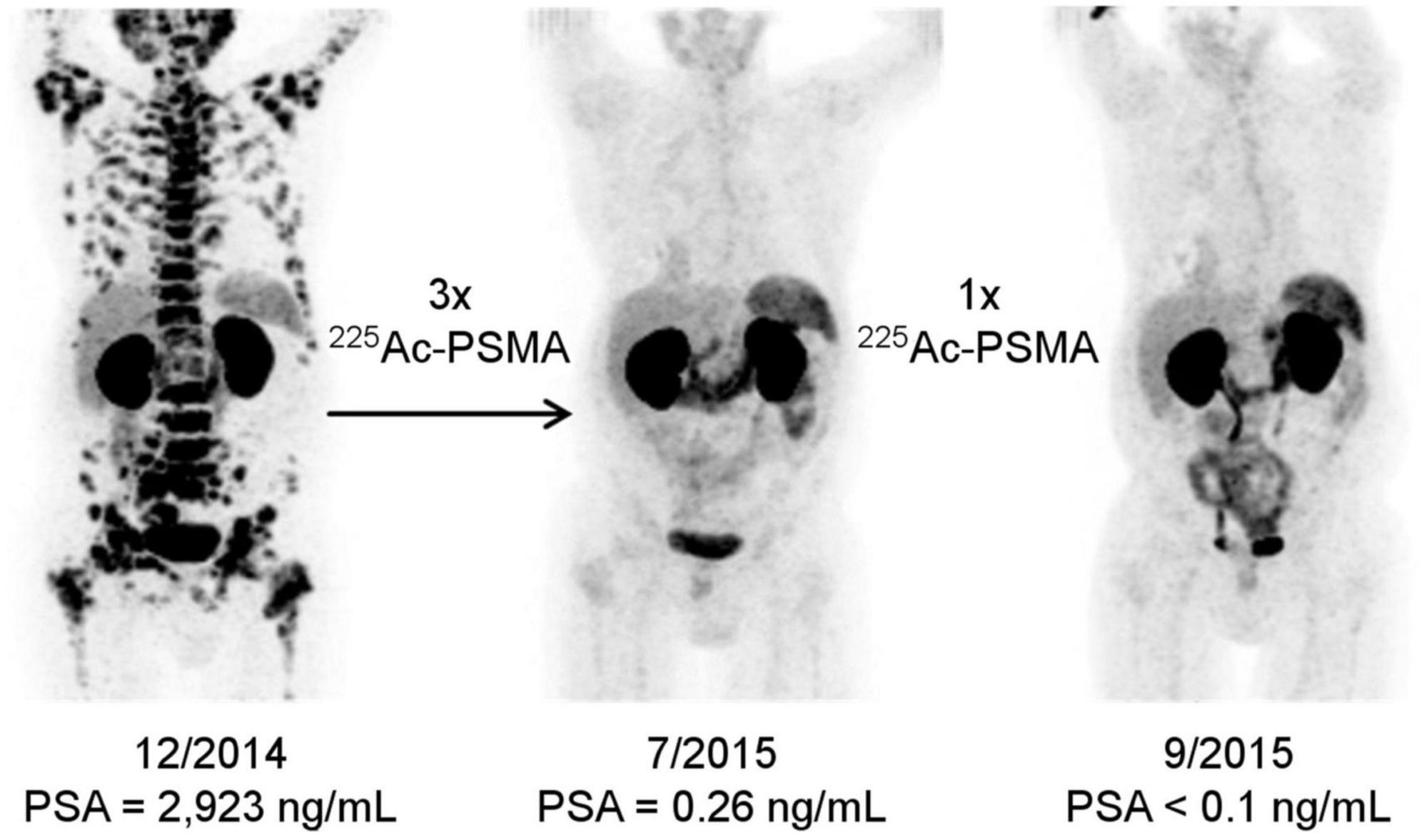
Figure 3. PET/CT scans of patient after receiving 225Ac-PSMA-617 treatments. Tumor spread before treatment (left), restaging 2 months after third 225Ac-PSMA-617 cycle (center), restaging 2 months after an additional consolidation cycle (right). Adapted with permission from ref 53. Copyright 2016 Society of Nuclear Medicine and Molecular Imaging.
The PSMA-617 and PSMA I&T constructs have also been used to chelate other clinically relevant α-emitters, such 213Bi. For instance, Nonnekens et al. compared the double-strand DNA breaks induced by 213Bi-PSMA I&T and 213Bi-JVZ-008 (a PSMA nanobody) in mice bearing prostate cancer xenografts (83). 213Bi-PSMA I&T showed higher tumor uptake and double-strand DNA breaks than its nanobody counterpart. Regarding PSMA-617, there is only one clinical study of 213Bi-PSMA-617, where a patient with metastatic castration-resistant prostate cancer was treated with the radiopharmaceutical (84). The patient, who was progressive under conventional therapy, received two cycles of 213Bi-PSMA-617. Remarkable molecular imaging response was observed by PET after 11 months. Moreover, the patient biochemistry notably improved with prostate specific antigen levels decreasing from 237 μg/L down to 43 μg/L. Nevertheless, a follow up study, which estimated the dosimetry of 213Bi-PSMA-617, showed that although the radioconstruct can reach dose levels acceptable for clinical applications, it has higher perfusion-dependent off-target radiation than 225Ac-PSMA-617 (85).
A novel strategy in the field of radiopharmaceuticals includes labeling the drugs with theranostic pairs, where one radionuclide provides therapeutic performance and the other one is used for imaging/diagnostic purposes (86, 87). As an example, PSMA-targeting ligands have been combined with 212Pb (parent of α-emitter 212Bi) and 203Pb (Ɣ-emitter with a half-life of 52 h), which can be used as agent for single photon emission computed tomography (SPECT) (88). Several new PSMA-targeting ligands have been developed for 212Pb and/or 203Pb, which provide rapid tumor uptake in mice bearing xenografts (89) and favorable antitumor responses (52). In clinical settings, the Ɣ-emitting 203Pb has been used as imaging surrogate to estimate the dosimetry of PSMA-targeting 212Pb drugs through planar scintigraphy scans (Figure 4) (90). 211At is another α-emitter that has shown significant tumor growth inhibition in xenograft models (91). This work has been recently expanded by developing new 211At therapeutic conjugates that display enhanced in vivo stability and tumor accumulation (92).
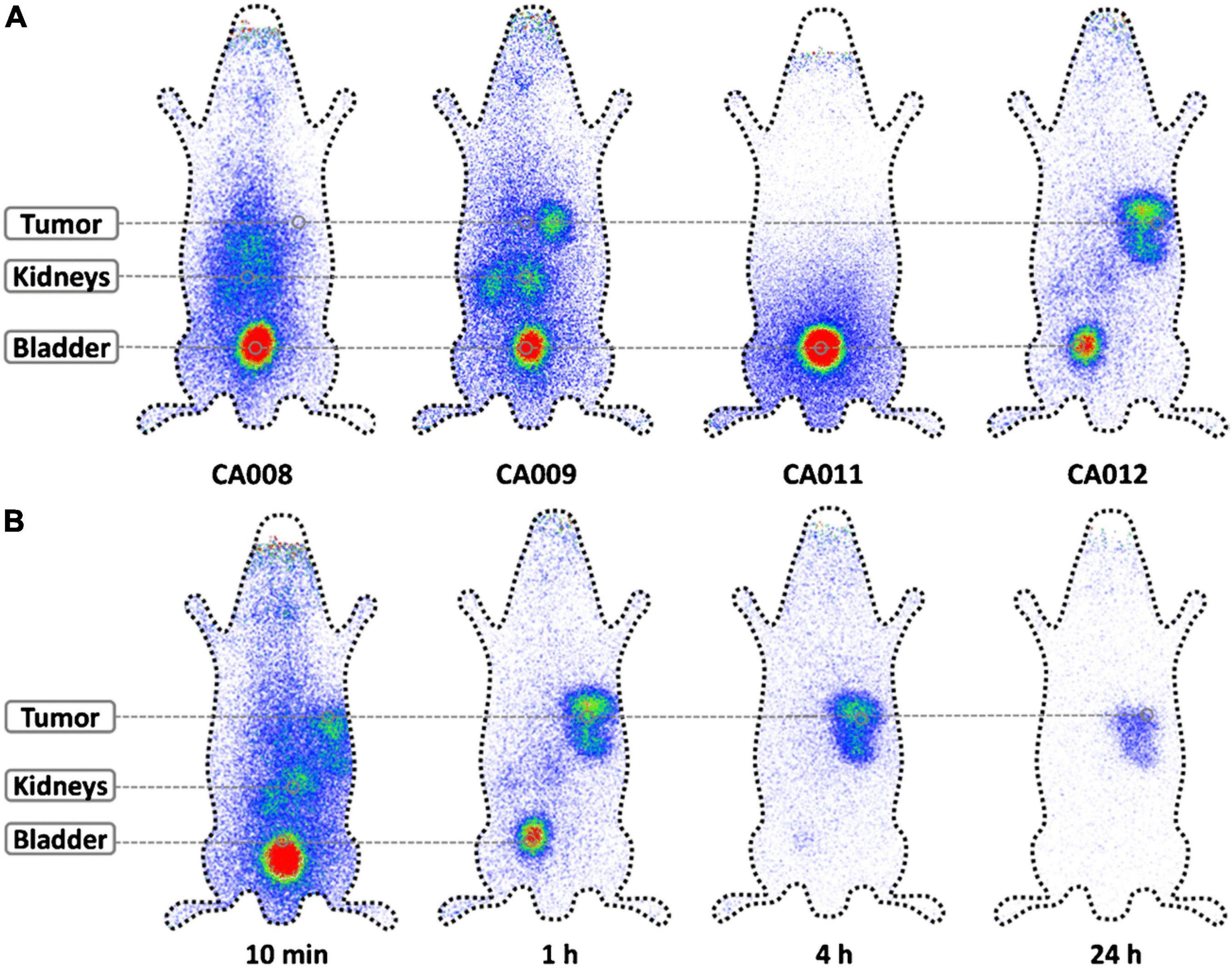
Figure 4. Theranostic pairs used for pharmacokinetics and dosimetry estimations. The Ɣ-emitting 203Pb was used as imaging surrogate of therapeutic 213Pb to estimate the pharmacokinetics and dosimetry of different PSMA-targeting radiopharmaceuticals. Planar scintigraphy scans of (A) different radioconjugates 1 h after injection, and (B) 203Pb-CA01 at different time points. Adapted with permission from ref 66. Copyright 2018 Springer Nature.
As mentioned earlier, the main dose-limiting side effect of radiopharmaceuticals using small molecules to target PSMA is severe xerostomia, which results from high salivary gland uptake (93). Recent awareness about xerostomia has shift radiopharmaceutical development toward other targeting vectors. For instance, Kelly et al. enhanced 225Ac ligand clearance by binding serum albumin to the PSMA-targeting molecules (94). Alternatively, anti-PSMA antibodies have also been used to target the antigen, maximizing selective tumor uptake, and preserving therapeutic performance in vivo (95).
4.2 Peptide targeting
The use of peptides in radiopharmaceuticals dates back to early 1990s, when somatostatin receptor targeting peptides were used in the clinical imaging of neuroendocrine and other somatostatin-positive tumors (26, 67). 111In-DTPA-DPhe1-octreotide was the first radiopharmaceutical to reach the market (96). Hence, the next natural step was to explore those same peptides for therapeutic applications. After initially studying the tumor suppression performance of 111In, which decays through electron capture, the field shifted toward other β-emitters, including 90Y and 177Lu (26). As a result of those efforts, 177Lu DOTATATE (an eight amino acid peptide covalently bonded to a DOTA chelator and labeled with 177Lu) was approved by the FDA for the treatment of somatostatin receptor-positive gastroenteropancreatic neuroendocrine tumors (97). The approval was based on a phase III clinical trial results (NCT01578239), which demonstrated 177Lu DOTATATE treatment yielded longer progression-free survival and higher response rate compared to standard of care. These results have encouraged new research toward developing similar formulations using α-emitters. For instance, DOTAMTATE (another somatostatin analog conjugated to a DOTA chelator unit) was labeled with 212Pb, and its therapeutic performance evaluated in animal models (98). After receiving three treatment cycles of 370 kBq 212Pb DOTAMTATE every 2 weeks, 79% of the mice were tumor free at the end of the 31-week study. Since then, a phase 1 clinical trial has been initiated to study 212Pb DOTAMTATE (commercially known as AlphaMedix) in adults suffering from neuroendocrine tumors (99). Preliminary results have shown favorable safety profiles, although full evaluations of safety and clinical performance are still in progress.
4.3 Antibody targeting
Antibodies have been the most commonly used targeting vector in targeted alpha therapy (27). Among the different classes of antibody to choose from, IgGs are the preferred ones because of their long circulation half-life (between 2 and 5 days depending on structure) and efficient elimination through liver and reticuloendothelial system (100). Moreover, antibody-based radiotherapy has benefited from current technology that allows to obtain IgGs with well-defined binding and selectivity against specific antigens (100). Similar to small molecule and peptide-based radiotherapy, early studies using antibodies as delivery vehicles focused on β-emitters, such as 131I (101, 102). More recently, the α-emitter 211At was explored as alternative to 131I during the ablation of bone marrow in preparation for transplantation. A synergistic treatment combining 211At-anti-CD45 immunoconjugates and bone marrow transplantation expanded survival in disseminated murine leukemia models (103). Minimal serological toxicity was observed after treatment, with recovery of white blood cell counts after 4 weeks. This study was followed by a second one that adjusted the reaction conditions and the quality control methods used to obtain the radioimmunoconjugate in laboratory settings to current good manufacturing practice production (as enforced by the FDA) (104). As a result of this progress, the 211At-radioimmunoconjugate has moved to a phase I/II clinical trial (NCT03128034) for the treatment of patients with relapsed or refractory high-risk acute leukemia before donor stem cell transplant.
Lintuzumab (also known as HuM195) is another monoclonal antibody used to target leukemia cells (105). Particularly, lintuzumab binds to a cell surface glycoprotein (CD33) found on the majority of myeloid leukemia cells, and myelomonocytic and erythroid progenitor cells (106). Hence, HuM195 has been one of the most explored antibodies for the development of radioimmunoconjugates against blood cancers. For example, an early pre-clinical work explored the dose-dependent cytotoxicity of 213Bi-HuM195 in vitro and the safety profile in vivo (107). The authors followed up with a study that characterized the pharmacokinetics and toxicity of 213Bi-HuM195 in mice (108), and another one that scaled up the production of the radioimmunoconjugate from pre-clinical to clinical quantities (109). All these pre-clinical studies provided enough data to move the development of the radiopharmaceutical to clinical settings, where the pharmacokinetics and dosimetry of 213Bi-HuM195 were evaluated in patients with leukemia (49, 110). The drug was also studied in a phase I dose-escalation trial that included 18 patients with advanced myeloid leukemias (111). The study showed reductions in bone marrow blast in 78% patients, although no complete remissions were reported. 213Bi-HuM195 dosage was further evaluated in another phase I/II trial, which determined the maximum tolerated dose and therapeutic effects after the patients had received chemotherapy (112). Because 213Bi is limited by its short half-life, subsequent studies with lintuzumab explored the use of other (longer-lived) radioisotopes, such as 225Ac (113, 114). Single doses of 225Ac-HuM195 in kBq range induced tumor regression and improved survival without toxicity in mice bearing tumor xenografts (113). Due to the release of 225Ac daughters during treatment had been associated with the development of radiation-induced nephritis (115), new strategies to protect renal function were developed. For instance, a low-dose spironolactone (a potassium-sparing diuretic) was administrated during treatment with 225Ac-HuM195, preventing the development of functional and histopathologic changes in the kidneys (116). Based on the pre-clinical data, two phase I clinical trials were undertaken, the first one was a dose escalation study to identify the safety, pharmacology, and biological activity of 225Ac-HuM195 (117), while the second one was a dose-escalation trial combining the radiopharmaceutical with chemotherapy (118). Since then, 225Ac-HuM195 has entered in a phase II clinical trial to establish the response rate in patients aged 60 years old and older with acute myeloid leukemia. Preliminary results showed a response rate of 69% in patients receiving 72 kBq/kg/dose (119). However, due to the high incidence (46%) of grade 4 thrombocytopenia, the dose was decreased to 55.5 kBq/kg for the rest of the clinical trial, which is currently ongoing. Beyond lintuzumab, daratumumab is another myeloma-targeting antibody (120), which has been recently explored for targeted alpha therapy. Nevertheless, although daratumumab radiolabeled with 225Ac has shown promising antitumoral effects both in vitro (121) and in vivo (122), the radiopharmaceutical has yet to move to clinical studies.
Beyond blood cancers, several radiopharmaceuticals have been developed to target solid tumors. For example, insulin-like growth factor receptor (IGF-1R) is an oncogenic protein over-expressed on the surface of a wide range of tumor cells (123). Because drugs targeting the IGF-1R had shown poor antitumoral effects in clinical settings (124), targeted alpha therapy was proposed as a therapeutic strategy against IGF-1R-expressing solid tumors. Hence, AVE1642 (a monoclonal antibody targeting IGF-1R) labeled with 225Ac was used to treat immunodeficient mice with colorectal, radioresistant lung, or prostate tumor xenografts (125). Single doses (between 1.85 and 14.8 kBq) showed high anti-tumor efficacy, as observed by the decrease of tumor volumes. Based on the positive in vivo data as well as previous clinical experience with the antibody itself, the first clinical trial (NCT03746431) was initiated to determine the pharmacokinetics and safety profile of the 225Ac radioimmunoconjugate (126).
Another antigen used in targeted alpha therapy against solid tumors is mesothelin, a membrane glycoprotein involved in cell proliferation that is overexpressed in ovarian, lung, pancreatic and triple-negative breast cancers, among others (127, 128). For example, a single dose administration (250 or 500 kBq/Kg) of a 227Th radioimmunoconjugate that targets mesothelin showed statistically significant antitumor effects in orthotopic bone xenograft models compared to control groups (129). Similar results were also reported when the 227Th radioimmunoconjugate was used to treat mice with other xenograft models, including breast, colorectal, lung, ovarian, and pancreatic tumors (130).
Trastuzumab is another IgG antibody used in the treatment of solid tumors, such as breast, ovarian, and gastric cancers (131). Trastuzumab targets human epidermal growth factor receptor 2 (HER2), a protein involved in cell proliferation that is overexpressed in multiple tumors [e.g., between 20 and 30% of breast cancers (132), and between 15 and 30% of ovarian cancers (133)]. Thus, trastuzumab (sold under commercial name of Herceptin) was approved by the FDA in 2008 to treat HER2-overexpressing metastatic breast cancers. However, the antibody as stand-alone treatment has limited efficacy, since the majority of tumors that initially respond to the treatment develop resistance within a year, becoming progressive again (134). Hence, radiotherapy using trastuzumab as targeting agent was proposed, since the radiopharmaceutical would likely require less antigens in each cancerous cell to be effective (compared to the antibody treatment), and resistance would be unlikely to occur. An early study explored the use of trastuzumab radiolabeled with 213Bi to treat colon and pancreatic xenograft models (135). Although the radiopharmaceutical had anti-tumor effects in both models, only mice bearing human colon carcinomas showed significant survival increases (from 20.5 days to 43 and 59 days after receiving doses of 18.5 and 27.75 MBq, respectively). A follow up study by the same authors compared the therapeutic performance of trastuzumab when radiolabeled with 212Bi, 213Bi, and 212Pb against peritoneal xenografts (136). 212Pb had better therapeutic index compared to the other two radionuclides, and required lower doses (between 370 and 1,480 kBq) to promote effective cytotoxic responses. The therapeutic benefits of 212Pb were also demonstrated in mice bearing human pancreatic carcinoma xenografts, which had been previously reported as unresponsive to 213Bi-trastuzumab (136). Moreover, the mouse survival could be further extended by combining the 212Pb-trastuzumab treatment with the administration of Gemcitabine (a chemotherapeutic agent) (137). All these pre-clinical data resulted on 212Pb-trastuzumab being explored on clinical settings. A phase I clinical study with three patients with HER2-expressing cancers that had been non-responsive to standard therapies received 7.4 MBq/m2 intraperitoneal injections of the radioimmunoconjugate to study pharmacokinetics and toxicity (138). Imaging demonstrated almost no radiopharmaceutical distribution outside the peritoneal cavity, while the administered dose was well tolerated by the patients with minimal toxicity signs. A follow up dose escalation and dosimetry study was performed by the same authors, showing minimal toxicity at more than a year after the patients received 7.4 MBq/m2, and almost no toxicity more than 4 months after the patients received 9.6, 12.6, 16.3, or 21.1 MBq/m2 (139). After a year, the authors reported that all dose levels were well tolerated with drug-related adverse effects being transient, mild, and not dose dependent (140). The mild side-effects included asymptomatic and abnormal laboratory values. However, no late renal, cardiac, or liver toxicity was observed up to a year post administration. Because all doses explored seemed safe, a higher dose (27 MBq/m2) was also investigated, and it was also well tolerated (140).
As the field of targeted alpha therapy started shifting from short-lived radionuclides to longer-lived ones, such as 225Ac and 227Th, so did the pre-clinical research using trastuzumab. An initial study demonstrated the therapeutic effect of trastuzumab radiolabeled with 227Th in breast and ovarian cancer cell lines (141). This study was followed by in vivo studies using different xenograft models, including ovarian, breast, and orthotopic bone cancers (46, 142–144). Normal tissue toxicity could be decreased by splitting the administrated dose (1000 kBq/kg) into several fractions (from a single injection to four injections every 2 or 4 weeks), while preserving the therapeutic effect (46). Furthermore, when the radiopharmaceutical was co-administrated with Olaparib (a chemotherapeutic drug), both treatments showed synergistic effects (145).
4.4 Nanoformulations
Liposomal and inorganic nanoconstructs are currently being investigated as delivery vehicles of radionuclides in pre-clinical settings (41, 146). Nanoformulations are advantageous over traditional delivery systems because they show enhanced cellular uptake (147, 148), high surface-to-volume ratios (149) that results in high loading capabilities, and ease of functionalization (150–152). Moreover, the unique optoelectronic properties of inorganic nanoparticles, which can be controlled through crystal engineering (153–156), can be exploited by other forms of therapy, such as photothermal therapy (157), magnetic hyperthermia (158), or smart drug-release (159), and imaging/diagnostics (160–163). Hence, nanoformulations that combine targeted alpha therapy with another type of therapy could potentially provide synergistic treatments.
One of the first nanoparticles explored for radionuclide delivery were zeolite nanoconstructs. A concern when using 223Ra in targeted alpha therapy is the recirculation of its daughters, particularly 219Rn, which is gaseous. By using porous zeolite nanoparticles as delivery system, between 90 and 95% retention of decay products was achieved (164). Furthermore, the nanoparticles were functionalized with a ligand that targets NK-1, a receptor overexpressed in glioma cells, providing selective cytotoxic effect in vitro. An alternative to loading the radionuclides in porous materials to minimize the release of daughters is encapsulating the α-emitters in shell structures. For instance, nanoparticle shells made of LaPO4 encapsulating 223Ra were able to retain up to 88% of the radionuclide (and its daughters) over 35 days (165).
Similar strategies (i.e., encapsulation with porous nanomaterials or nanoparticle shells) have also been used to improve the retention of 225Ac daughters. For example, mesoporous silica nanoparticles, which have high surface-to-volume ratios because of their porous structure, allowing high radionuclide content per particle, were employed in 225Ac-based targeted alpha therapy against breast cancer cells (Figure 5) (166). The silica nanoparticle pores were loaded with 225Ac complexed by a hydroxypyridonate chelator, which improved radionuclide retention. The nanoparticle surface was functionalized with targeting agents, which promoted accumulation and cytotoxic effects in cancerous cells. Furthermore, in vivo studies in mice demonstrated that the nanoparticles enhanced radionuclide excretion, minimizing internal deposition. Alternatively, gold nanoshells made of 225Ac-doped La0.5Gd0.5PO4 cores and covered by thin gold shells displayed enhanced radionuclide retention (167). The gold nanoconstructs were further functionalized with antibodies and demonstrated targeting capabilities in vivo. A recent work by Karpov et al. further demonstrated the benefits of metal coating on radionuclide retention. The study reported that 225Ac encapsulated in silica cores and coated by gold or titania shells displayed no significant toxicity effects up to 10 days post-administration, as reveled by histological analysis (168). Furthermore, no radionuclide could be detected in non-targeted organs during that period of time. Beyond 225Ac, gold nanoparticles have also been used to deliver other α-emitters, such as 211At (169).
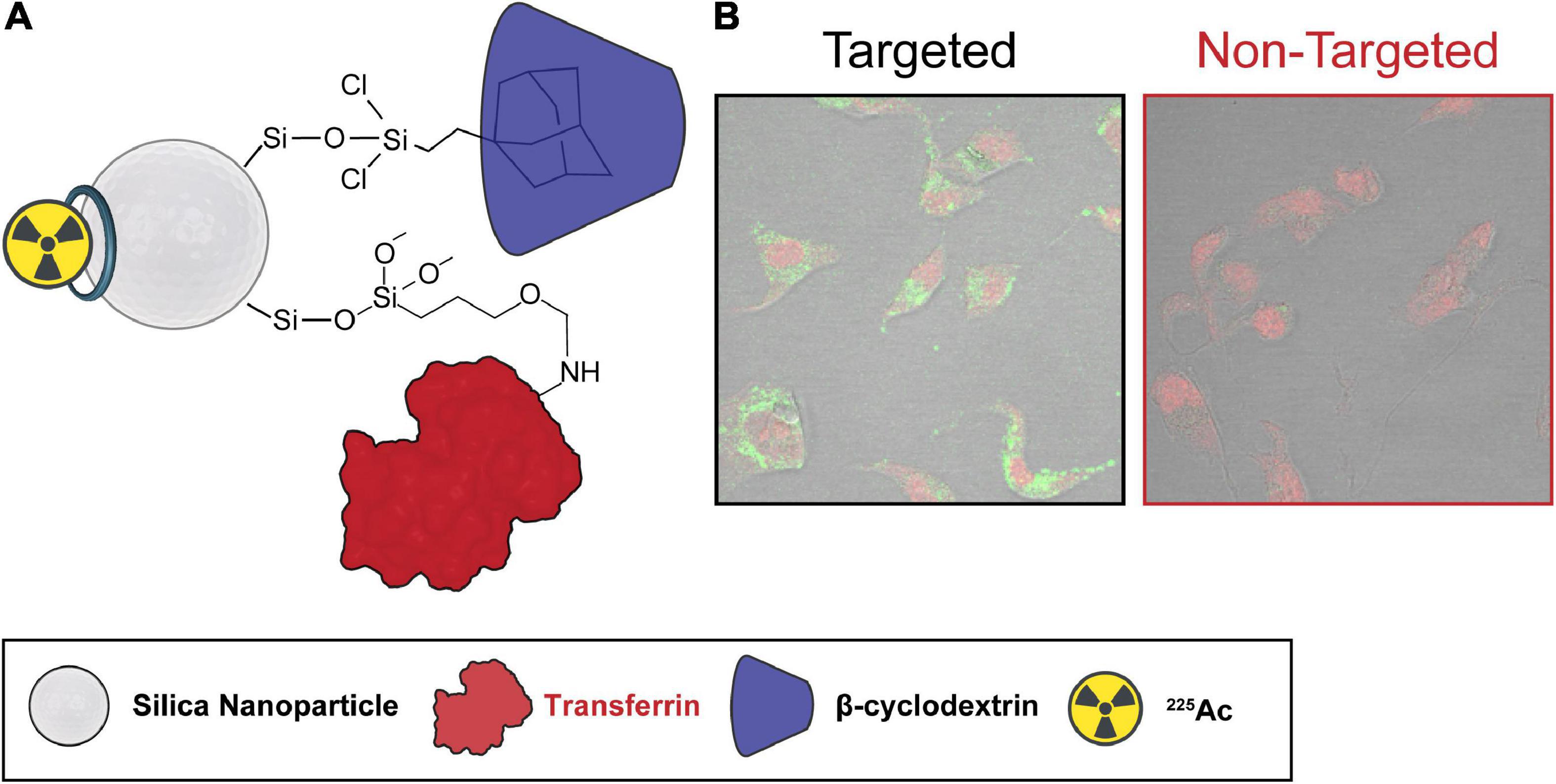
Figure 5. Silica nanoparticles as delivery vehicles for targeted alpha therapy. Silica nanoparticles were used as delivery vehicles of 225Ac for targeted alpha therapy. Transferrin and cyclodextrin were used as targeting and stabilizing agents, respectively. (A) Schematic representation, (B) cellular uptake with and without targeting agent. Adapted with permission from ref 139. Copyright 2020 American Chemical Society.
Lastly, titania nanoparticles radiolabeled with 225Ac and functionalized with peptide fragments targeting NK-1 receptors were used to selectively deliver the α-emitter to glioma cells (170). Alternatively, 225Ac was also incorporated into liposome-based nanoparticles, which could cross the blood-brain barrier and deliver the cytotoxic dose to glioblastoma cells through integrin αVβ3-targeting (171, 172).
5 Challenges and future opportunities
Targeted alpha therapy has demonstrated to be an effective therapeutic strategy against a wide range of cancers, including tumors that were resistant to conventional treatments (26). Nevertheless, targeted alpha therapy has not become a gold standard treatment in oncology, as other therapies have, because of several challenges.
First, implementation of targeted alpha therapy in the clinic requires α-emitters being easily available and at reasonable costs, two conditions that are not currently met (173). For example, the annual production of 225Ac is around 75 GBq, which can only support a few hundred patients per year (174). No single production source is expected to individually achieve the sufficient scale for widespread use of 225Ac in the near future. Nevertheless, medium-energy proton irradiations of 232Th targets and high flux reactor irradiation of 226Ra and 227Ac targets are leading the efforts to yield enough 225Ac production for large patient population treatment (174). Overall, there are ongoing technical efforts (both in the private and public sectors) to overcome the production and supply issues associated with targeted alpha therapy radioisotopes (175). Those include developing new production avenues for these key medical radioisotopes (176–178).
Second, despite the absence of resistance mechanisms to α-radiation, cancerous cells may develop coping strategies against the delivery of the radionuclides, such as down-regulating the expression of surface proteins that are being targeted by the radiopharmaceuticals. Hence, expanding the current library of targeting agents and epitopes will be necessary to face tumors with acquired resistance, as well as cancers that inherently show low antigen expression.
Third, dosimetry calculations for the medical radioisotopes and daughters are still challenging because of the wide-range of factors that need to be considered. For example, the intra- and inter-tumor heterogeneity, including variation in antigen expression and vascularization, strongly affects the interaction between the radiopharmaceutical and the cancer cells. Hence, imaging techniques that provide this type of information are necessary, as well as modeling methods capable to account for all the different factors. Autoradiograph can image the tumor with high resolution, but it has to be done ex vivo (179). Some groups exploited the Ɣ-emission during 225Ac decay to image the radiopharmaceutical in the tumor by SPECT (180–182). Nevertheless, the low activities used during targeted alpha therapy render the imaging of 225Ac by SPECT very challenging (66). In this situation, theranostic pairs, where one radioisotope provides therapeutic benefits and the other one imaging/diagnostic information, are a valuable alternative. However, theranostic imaging does not provide information regarding the redistribution and impact of the daughters.
Four, the α-emitters, particularly those with multiple α-decays in their decay chain, are limited by the recoil effect, which causes the release of part of the daughters from the radiopharmaceutical (29, 183). This is problematic for two main reasons. First, the instability of the radiopharmaceuticals complicates the exact determination of their radiochemical purity. Second, the uncontrolled circulation and deposition of the daughters may damage healthy tissue and induce radiological poisoning. Because this issue is unlikely to be solved by new chelators, a novel method to minimize the release of the daughters is encapsulating the radionuclides in nanoconstructs (41). These nanoformulations, however, have been primarily tested in pre-clinical settings, and they are still far from being used in patients.
Regarding nanoformulations, it is also worth noting that nanoparticles are routinely used in the clinic for cancer therapy and imaging (e.g., Doxil, Abraxane, Hensify, Oncaspar, 99mTc colloids) (184). In therapy, nanomedicines with long circulation times are usually preferred, as those allow for larger accumulation of the nanoformulations at the pathological sites, which tend to yield better therapeutic outcomes (185). In the case of targeted alpha therapy is unclear whether short or long circulation times should be favored, as longer circulation times could result in higher therapeutic effects but larger possibility of radionuclide internal deposition. Nevertheless, there are currently nanoformulation designs with long (e.g., Doxil, Hensify) and short (e.g., Cornell dots) circulation times either clinically approved or in clinical trials. Therefore, both pharmacokinetic designs are achievable, and future studies will have to define which option is better suited for targeted alpha therapy.
6 Summary and outlook
Targeted alpha therapy is a very promising treatment in oncology, since it can focalize highly cytotoxic doses to cancer cells, while sparring the surrounding tissue. This therapy relies on the delivery of α-emitters to the tumor sites guided by targeting agents. In this context, α-emissions are advantageous over other types of radiation, since they have higher linear energy transfers and shorter path ranges, yielding higher therapeutic performance with lower potential side effects. Despite the positive results of targeted alpha therapy in both pre-clinical and clinical studies, where tumors resistant to conventional treatments were partially or completely eradicated, there are still challenges that need to be addressed (e.g., radionuclide scarcity, precise dosimetry calculations, and daughter release from the drug) before targeted alpha therapy can be routinely used in patients.
In this comprehensive review, we have summarized the state-of-the-art in the development of radiopharmaceuticals for targeted alpha therapy, identifying their key structural components. The pros and cons of the different radionuclides (e.g., isotopes with one or multiple α-emissions in their decay chain) as well the targeting vectors (e.g., small molecules, peptides, and antibodies) commonly used in the field have been critically discussed. Moreover, we have highlighted ongoing strategies to overcome some of the main pitfalls the therapy currently presents, such as encapsulating the radionuclides in nanoformulations to prevent the release of the daughters. Lastly, we have discussed potential opportunities the field holds, which may contribute to targeted alpha therapy becoming a gold standard treatment in oncology in the future. Hence, we believe this review will assist other scientists to understand the current status of the field, allowing them to recognize promising research directions that may be important in the future.
Author contributions
RP and RA wrote the manuscript. Both authors contributed to the article and approved the submitted version.
Funding
This work was supported by the University of California Contractor Supporting Research Program at Lawrence Berkeley National Laboratory under U.S. Department of Energy Contract (No. DE-AC02-05CH11231).
Conflict of interest
The authors declare that the research was conducted in the absence of any commercial or financial relationships that could be construed as a potential conflict of interest.
Publisher’s note
All claims expressed in this article are solely those of the authors and do not necessarily represent those of their affiliated organizations, or those of the publisher, the editors and the reviewers. Any product that may be evaluated in this article, or claim that may be made by its manufacturer, is not guaranteed or endorsed by the publisher.
References
1. Lacoeuille F, Arlicot N, Faivre-Chauvet A. Targeted alpha and beta radiotherapy: an overview of radiopharmaceutical and clinical aspects. Méd Nucl. (2018) 42:32–44. doi: 10.1016/j.mednuc.2017.12.002
2. Makvandi M, Dupis E, Engle JW, Nortier FM, Fassbender ME, Simon S, et al. Alpha-emitters and targeted alpha therapy in oncology: from basic science to clinical investigations. Target Oncol. (2018) 13:189–203. doi: 10.1007/s11523-018-0550-9
3. Guerra Liberal FDC, O’sullivan JM, Mcmahon SJ, Prise KM. Targeted alpha therapy: current clinical applications. Cancer Biother Radiopharm. (2020) 35:404–17. doi: 10.1089/cbr.2020.3576
4. Dadachova E, Kitchen SG, Bristol G, Baldwin GC, Revskaya E, Empig C, et al. Pre-clinical evaluation of a 213Bi-Labeled 2556 antibody to HIV-1 gp41 glycoprotein in HIV-1 mouse models as a reagent for HIV eradication. PLoS One. (2012) 7:e31866. doi: 10.1371/journal.pone.0031866
5. Helal M, Dadachova E. Radioimmunotherapy as a novel approach in HIV, bacterial, and fungal infectious diseases. Cancer Biother Radiopharm. (2018) 33:330–5. doi: 10.1089/cbr.2018.2481
6. Pallares RM, Abergel RJ. Diagnostic, prognostic, and therapeutic use of radiopharmaceuticals in the context of SARS-CoV-2. ACS Pharmacol Transl Sci. (2021) 4:1–7. doi: 10.1021/acsptsci.0c00186
7. Pallares RM, Flick M, Shield KM, Bailey TA, Velappan N, Lillo AM, et al. Development of an actinium-225 radioimmunoconjugate for targeted alpha therapy against SARS-CoV-2. N J Chem. (2022) 46:15795–8. doi: 10.1039/D2NJ02617A
8. Milenic DE, Brechbiel MW. Targeting of radio-isotopes for cancer therapy. Cancer Biol Ther. (2004) 3:361–70. doi: 10.4161/cbt.3.4.790
9. Goldsmith SJ. Radioimmunotherapy of lymphoma: bexxar and zevalin. Semin Nucl Med. (2010) 40:122–35. doi: 10.1053/j.semnuclmed.2009.11.002
10. Hennrich U, Kopka K. Lutathera® : the first FDA- and EMA-approved radiopharmaceutical for peptide receptor radionuclide therapy. Pharmaceuticals. (2019) 12:114. doi: 10.3390/ph12030114
11. Keam SJ. Lutetium Lu 177 vipivotide tetraxetan: first approval. Mol Diagn Ther. (2022) 26:467–75. doi: 10.1007/s40291-022-00594-2
12. Couturier O, Supiot S, Degraef-Mougin M, Faivre-Chauvet A, Carlier T, Chatal J-F, et al. Cancer radioimmunotherapy with alpha-emitting nuclides. Eur J Nucl Med Mol Imaging. (2005) 32:601–14. doi: 10.1007/s00259-005-1803-2
13. Pouget J-P, Constanzo J. Revisiting the radiobiology of targeted alpha therapy. Front Med. (2021) 8:692436. doi: 10.3389/fmed.2021.692436
14. Leung CN, Canter BS, Rajon D, Bäck TA, Fritton JC, Azzam EI, et al. Dose-dependent growth delay of breast cancer xenografts in the bone marrow of mice treated 223Ra: the role of bystander effects and their potential for therapy. J Nucl Med. (2020) 61:89. doi: 10.2967/jnumed.119.227835
15. Lejeune P, Cruciani V, Berg-Larsen A, Schlicker A, Mobergslien A, Bartnitzky L, et al. Immunostimulatory effects of targeted thorium-227 conjugates as single agent and in combination with anti-PD-L1 therapy. J Immunother Cancer. (2021) 9:e002387. doi: 10.1136/jitc-2021-002387
16. Li J, He M, Shen B, Yuan D, Shao C. Alpha particle-induced bystander effect is mediated by ROS via a p53-dependent SCO2 pathway in hepatoma cells. Int J Radiat Biol. (2013) 89:1028–34. doi: 10.3109/09553002.2013.817706
17. Pouget J-P, Navarro-Teulon I, Bardiès M, Chouin N, Cartron G, Pèlegrin A, et al. Clinical radioimmunotherapy—the role of radiobiology. Nat Rev Clin Oncol. (2011) 8:720–34. doi: 10.1038/nrclinonc.2011.160
18. Laughhunn L, Botkin C, Hubble W, Hewing D, Turner J, Muzaffar R, et al. Xofigo: an exciting new therapy for prostate cancer. J Nucl Med. (2014) 55:2707.
19. Henriksen G, Fisher DR, Roeske JC, Bruland ØS, Larsen RH. Targeting of osseous sites with α-emitting 223Ra: comparison with the β-Emitter 89Sr in mice. J Nucl Med. (2003) 44:252.
20. Suominen MI, Fagerlund KM, Rissanen JP, Konkol YM, Morko JP, Peng Z, et al. Radium-223 inhibits osseous prostate cancer growth by dual targeting of cancer cells and bone microenvironment in mouse models. Clin Cancer Res. (2017) 23:4335. doi: 10.1158/1078-0432.CCR-16-2955
21. Sartor O, Reid RH, Hoskin PJ, Quick DP, Ell PJ, Coleman RE, et al. Samarium-153-lexidronam complex for treatment of painful bone metastases in hormone-refractory prostate cancer. Urology. (2004) 63:940–5. doi: 10.1016/j.urology.2004.01.034
22. Wang C, Li W, Drabek D, Okba NMA, Van Haperen R, Osterhaus ADME, et al. A human monoclonal antibody blocking SARS-CoV-2 infection. Nat Commun. (2020) 11:2251. doi: 10.1038/s41467-020-16256-y
23. Parker C, Nilsson S, Heinrich D, Helle SI, O’sullivan JM, Fosså SD, et al. Alpha emitter radium-223 and survival in metastatic prostate cancer. N Engl J Med. (2013) 369:213–23. doi: 10.1056/NEJMoa1213755
24. Sartor O, Coleman R, Nilsson S, Heinrich D, Helle SI, O’sullivan JM, et al. Effect of radium-223 dichloride on symptomatic skeletal events in patients with castration-resistant prostate cancer and bone metastases: results from a phase 3, double-blind, randomised trial. Lancet Oncol. (2014) 15:738–46. doi: 10.1016/S1470-2045(14)70183-4
25. Elgqvist J, Frost S, Pouget J-P, Albertsson P. The potential and hurdles of targeted alpha therapy – clinical trials and beyond. Front Oncol. (2014) 3:324. doi: 10.3389/fonc.2013.00324
26. Sgouros G, Bodei L, Mcdevitt MR, Nedrow JR. Radiopharmaceutical therapy in cancer: clinical advances and challenges. Nat Rev Drug Discov. (2020) 19:589–608. doi: 10.1038/s41573-020-0073-9
27. Parker C, Lewington V, Shore N, Kratochwil C, Levy M, Lindén O, et al. Targeted alpha therapy, an emerging class of cancer agents: a review. JAMA Oncol. (2018) 4:1765–72. doi: 10.1001/jamaoncol.2018.4044
28. De Kruijff MR, Wolterbeek TH, Denkova GA. A critical review of alpha radionuclide therapy—how to deal with recoiling daughters? Pharmaceuticals. (2015) 8:321–36. doi: 10.3390/ph8020321
29. Kozempel J, Mokhodoeva O, Vlk M. Progress in targeted alpha-particle therapy. what we learned about recoils release from in vivo generators. Molecules. (2018) 23:581. doi: 10.3390/molecules23030581
30. Deblonde GJP, Sturzbecher-Hoehne M, Mason AB, Abergel RJ. Receptor recognition of transferrin bound to lanthanides and actinides: a discriminating step in cellular acquisition of f-block metals. Metallomics. (2013) 5:619–26. doi: 10.1039/c3mt20237b
31. Pallares RM, Panyala NR, Sturzbecher-Hoehne M, Illy M-C, Abergel RJ. Characterizing the general chelating affinity of serum protein fetuin for lanthanides. J Biol Inorg Chem. (2020) 25:941–8. doi: 10.1007/s00775-020-01815-x
32. Beyer GJ, Bergmann R, Schomäcker K, Rösch F, Schäfer G, Kulikov EV, et al. Comparison of the Biodistribution of 225Ac and Radio-Lanthanides as Citrate Complexes. Isotopenpraxis. (1990) 26:111–4. doi: 10.1080/10256019008624245
33. Pallares RM, An DD, Deblonde GJP, Kullgren B, Gauny SS, Jarvis EE, et al. Efficient discrimination of transplutonium actinides by in vivo models. Chem Sci. (2021) 12:5295–301. doi: 10.1039/D0SC06610A
34. Mizukami-Murata S, Murata Y, Iwahashi H. Chemical toxicity of thorium in Saccharomyces cerevisiae. Japanese J Environ Toxicol. (2006) 9:87–100.
35. Pallares RM, An DD, Hébert S, Faulkner D, Loguinov A, Proctor M, et al. Multidimensional genome-wide screening in yeast provides mechanistic insights into europium toxicity. Metallomics. (2021) 13:mfab061. doi: 10.1093/mtomcs/mfab061
36. Pallares RM, Faulkner D, An DD, Hébert S, Loguinov A, Proctor M, et al. Genome-wide toxicogenomic study of the lanthanides sheds light on the selective toxicity mechanisms associated with critical materials. Proc Natl Acad Sci USA. (2021) 118:e2025952118. doi: 10.1073/pnas.2025952118
37. Pallares RM, An DD, Hébert S, Faulkner D, Loguinov A, Proctor M, et al. Delineating toxicity mechanisms associated with MRI contrast enhancement through a multidimensional toxicogenomic profiling of gadolinium. Mol Omics. (2022) 18:237–48. doi: 10.1039/D1MO00267H
38. Pallares RM, An DD, Hébert S, Loguinov A, Proctor M, Villalobos JA, et al. Identifying toxicity mechanisms associated with early lanthanide exposure through multidimensional genome-wide screening. ACS Omega. (2022) 7:34412–9. doi: 10.1021/acsomega.2c04045
39. Pallares RM, Jarvis E, An DD, Wu CH, Chang PY, Abergel RJ. Toxicogenomic assessment of organ-specific responses following plutonium internal contamination. Environ Adv. (2022) 8:100245. doi: 10.1016/j.envadv.2022.100245
40. Majkowska-Pilip A, Gawêda W, Żelechowska-Matysiak K, Wawrowicz K, Bilewicz A. Nanoparticles in targeted alpha therapy. Nanomaterials. (2020) 10:1366. doi: 10.3390/nano10071366
41. Pallares RM, Abergel RJ. Nanoparticles for targeted cancer radiotherapy. Nano Res. (2020) 13:2887–97. doi: 10.1007/s12274-020-2957-8
42. Atcher RW, Friedman AM, Huizenga JR, Spencer RP. A radionuclide generator for the production of211Pb and its daughters. J Radioanalyt Nucl Chem. (1989) 135:215–21. doi: 10.1007/BF02164974
43. Stabin MG, Siegel JA. Radiation dose and hazard assessment of potential contamination events during use of 223Ra dichloride in radionuclide therapy. Health Phys. (2015) 109:212–7. doi: 10.1097/HP.0000000000000310
44. Nagata K, Shirasaki K, Toyoshima A, Ooe K, Yamamura T, Shinohara A, et al. Dispersal rate of Radon-219 from aqueous radium-223 solution containing sodium Chloride/Citrate. Radiat Saf Manag. (2020) 19:1–9. doi: 10.12950/rsm.190328
45. Davis IA, Glowienka KA, Boll RA, Deal KA, Brechbiel MW, Stabin M, et al. Comparison of 225actinium chelates: tissue distribution and radiotoxicity. Nucl Med Biol. (1999) 26:581–9. doi: 10.1016/S0969-8051(99)00024-4
46. Heyerdahl H, Abbas N, Brevik EM, Mollatt C, Dahle J. Fractionated therapy of HER2-expressing breast and ovarian cancer xenografts in mice with targeted alpha emitting 227Th-DOTA-p-benzyl-trastuzumab. PLoS One. (2012) 7:e42345. doi: 10.1371/journal.pone.0042345
47. Atcher RW, Friedman AM, Hines JJ. An improved generator for the production of 212Pb and 212Bi from 224Ra. Int J Rad Appl Instrum A. (1988) 39:283–6. doi: 10.1016/0883-2889(88)90016-0
48. Wadas TJ, Pandya DN, Solingapuram Sai KK, Mintz A. Molecular targeted α-particle therapy for oncologic applications. Am J Roentgenol. (2014) 203:253–60. doi: 10.2214/AJR.14.12554
49. Sgouros G, Ballangrud ÅM, Jurcic JG, Mcdevitt MR, Humm JL, Erdi YE, et al. Pharmacokinetics and dosimetry of an alpha-particle emitter labeled antibody: 213Bi-HuM195 (anti-CD33) in patients with leukemia. J Nucl Med. (1999) 40:1935.
50. Mausner LF, Srivastava SG, Straub RF. In Vivo Generator for Radioimmunotherapy. USA patent application USH545H. (1987).
51. Ackerman NL, De La Fuente Rosales L, Falzone N, Vallis KA, Bernal MA. Targeted alpha therapy with 212Pb or 225Ac: change in RBE from daughter migration. Phys Med. (2018) 51:91–8. doi: 10.1016/j.ejmp.2018.05.020
52. Banerjee SR, Minn I, Kumar V, Josefsson A, Lisok A, Brummet M, et al. Preclinical evaluation of 203/212Pb-labeled low-molecular-weight compounds for targeted radiopharmaceutical therapy of prostate cancer. J Nucl Med. (2020) 61:80. doi: 10.2967/jnumed.119.229393
53. Smit JA, Myburgh JA, Neirinckx RD. Specific inactivation of sensitized lymphocytes in vitro using antigens labelled with astatine-211. Clin Exp Immunol. (1973) 14:107–16.
54. Larsen RH, Murud KM, Akabani G, Hoff P, Bruland ØS, Zalutsky MR. 211At- and 131I-labeled bisphosphonates with high in vivo stability and bone accumulation. J Nucl Med. (1999) 40:1197.
55. Johnson EL, Turkington TG, Jaszczak RJ, Gilland DR, Vaidyanathan G, Greer KL, et al. Quantitation of 211At in small volumes for evaluation of targeted radiotherapy in animal models. Nucl Med Biol. (1995) 22:45–54. doi: 10.1016/0969-8051(94)00077-W
56. Maingueneau C, Berdal M, Eychenne R, Gaschet J, Chérel M, Gestin J-F, et al. 211At and 125I-Labeling of (Hetero)aryliodonium ylides: astatine wins again. Chemistry. (2022) 28:e202104169. doi: 10.1002/chem.202104169
57. Zalutsky MR, Reardon DA, Pozzi OR, Vaidyanathan G, Bigner DD. Targeted α-particle radiotherapy with 211At-labeled monoclonal antibodies. Nucl Med Biol. (2007) 34:779–85. doi: 10.1016/j.nucmedbio.2007.03.007
58. International Commission on Radiological Protection [ICRP]. Report of Committee IV on Evaluation of Radiation Doses to Body Tissues from Internal Contamination due to Occupational Exposure. Oxford: Pergamon Press (1968).
60. Bentzen SM, Dörr W, Gahbauer R, Howell RW, Joiner MC, Jones B, et al. Bioeffect modeling and equieffective dose concepts in radiation oncology – Terminology, quantities and units. Radiother Oncol. (2012) 105:266–8. doi: 10.1016/j.radonc.2012.10.006
61. Billena C, Khan AJ. A current review of spatial fractionation: back to the future? Int J Radiat Oncol Biol Phys. (2019) 104:177–87. doi: 10.1016/j.ijrobp.2019.01.073
62. Pouget J-P, Lozza C, Deshayes E, Boudousq V, Navarro-Teulon I. Introduction to radiobiology of targeted radionuclide therapy. Front Med. (2015) 2:12. doi: 10.3389/fmed.2015.00012
63. Sgouros G, Bolch WE, Chiti A, Dewaraja YK, Emfietzoglou D, Hobbs RF, et al. ICRU REPORT 96, dosimetry-guided radiopharmaceutical therapy. J ICRU. (2021) 21:1–212. doi: 10.1177/14736691211060117
64. Pouget J-P, Konijnenberg M, Eberlein U, Glatting G, Gabina PM, Herrmann K, et al. An EANM position paper on advancing radiobiology for shaping the future of nuclear medicine. Eur J Nucl Med Mol Imaging. (2022). doi: 10.1007/s00259-022-05934-2x [Epub ahead of print].
65. Roeske JC, Aydogan B, Bardies M, Humm JL. Small-scale dosimetry: challenges and future directions. Semin Nucl Med. (2008) 38:367–83. doi: 10.1053/j.semnuclmed.2008.05.003
66. Robertson AKH, Ramogida CF, Rodríguez-Rodríguez C, Blinder S, Kunz P, Sossi V, et al. Multi-isotope SPECT imaging of the225Ac decay chain: feasibility studies. Phys Med Biol. (2017) 62:4406–20. doi: 10.1088/1361-6560/aa6a99
67. Hoppenz P, Els-Heindl S, Beck-Sickinger AG. Peptide-drug conjugates and their targets in advanced cancer therapies. Front Chem. (2020) 8:571. doi: 10.3389/fchem.2020.00571
69. Huang CT, Guo X, Baøinka C, Lupold SE, Pomper MG, Gabrielson K, et al. Development of 5D3-DM1: a novel anti-prostate-specific membrane antigen antibody-drug conjugate for PSMA-positive prostate cancer therapy. Mol Pharm. (2020) 17:3392–402. doi: 10.1021/acs.molpharmaceut.0c00457
70. Rosenfeld L, Sananes A, Zur Y, Cohen S, Dhara K, Gelkop S, et al. Nanobodies targeting prostate-specific membrane antigen for the imaging and therapy of prostate cancer. J Med Chem. (2020) 63:7601–15. doi: 10.1021/acs.jmedchem.0c00418
71. Rahbar K, Afshar-Oromieh A, Jadvar H, Ahmadzadehfar H. PSMA theranostics: current status and future directions. Mol Imaging. (2018) 17:1536012118776068. doi: 10.1177/1536012118776068
72. Juzeniene A, Stenberg VY, Bruland ØS, Larsen RH. Preclinical and clinical status of PSMA-targeted alpha therapy for metastatic castration-resistant prostate cancer. Cancers. (2021) 13:779. doi: 10.3390/cancers13040779
73. Hofman MS, Emmett L, Sandhu S, Iravani A, Joshua AM, Goh JC, et al. [177Lu]Lu-PSMA-617 versus cabazitaxel in patients with metastatic castration-resistant prostate cancer (TheraP): a randomised, open-label, phase 2 trial. Lancet. (2021) 397:797–804.
74. Kratochwil C, Bruchertseifer F, Giesel FL, Weis M, Verburg FA, Mottaghy F, et al. 225Ac-PSMA-617 for PSMA-targeted α-radiation therapy of metastatic castration-resistant prostate cancer. J Nucl Med. (2016) 57:1941. doi: 10.2967/jnumed.116.178673
75. Sathekge M, Bruchertseifer F, Knoesen O, Reyneke F, Lawal I, Lengana T, et al. 225Ac-PSMA-617 in chemotherapy-naive patients with advanced prostate cancer: a pilot study. Eur J Nucl Med Mol Imaging. (2019) 46:129–38. doi: 10.1007/s00259-018-4167-0
76. Kratochwil C, Bruchertseifer F, Rathke H, Bronzel M, Apostolidis C, Weichert W, et al. Targeted α-therapy of metastatic castration-resistant prostate cancer with 225Ac-PSMA-617: dosimetry estimate and empiric dose finding. J Nucl Med. (2017) 58:1624. doi: 10.2967/jnumed.117.191395
77. Kratochwil C, Bruchertseifer F, Rathke H, Hohenfellner M, Giesel FL, Haberkorn U, et al. Targeted alpha- therapy of metastatic castration-resistant prostate cancer with (225)Ac-PSMA-617: swimmer-plot analysis suggests efficacy regarding duration of tumor control. J Nucl Med. (2018) 59:795. doi: 10.2967/jnumed.117.203539
78. Ilhan H, Gosewisch A, Böning G, Völter F, Zacherl M, Unterrainer M, et al. Response to 225Ac-PSMA-I&T after failure of long-term 177Lu-PSMA RLT in mCRPC. Eur J Nucl Med Mol Imaging. (2021) 48:1262–3. doi: 10.1007/s00259-020-05023-2
79. Zacherl MJ, Gildehaus FJ, Mittlmeier L, Böning G, Gosewisch A, Wenter V, et al. First clinical results for PSMA targeted alpha therapy using 225Ac-PSMA-I&T in advanced mCRPC patients. J Nucl Med. (2021) 62:669. doi: 10.2967/jnumed.120.251017
80. Pelletier K, Côté G, Fallah-Rad N, John R, Kitchlu A. CKD After 225Ac-PSMA617 therapy in patients with metastatic prostate cancer. Kidney Int Rep. (2021) 6:853–6. doi: 10.1016/j.ekir.2020.12.006
81. Kulkarni H, Zhang J, Langbein T, Schuchardt C, Singh A, Mueller D, et al. Radioligand therapy using combination of Ac-225 and Lu-177 labelled PSMA ligands for progressive end-stage metastatic prostate cancer: effective trade-off between response and toxicity. J Nucl Med. (2019) 60:464. doi: 10.1055/s-0039-1683560
82. Langbein T, Kulkarni HR, Schuchardt C, Mueller D, Volk GF, Baum RP. Salivary gland toxicity of PSMA-targeted radioligand therapy with 177Lu-PSMA and combined 225Ac- and 177Lu-labeled PSMA ligands (TANDEM-PRLT) in advanced prostate cancer: a single-center systematic investigation. Diagnostics. (2022) 12:1926. doi: 10.3390/diagnostics12081926
83. Nonnekens J, Chatalic KLS, Molkenboer-Kuenen JDM, Beerens CEMT, Bruchertseifer F, Morgenstern A, et al. 213Bi-Labeled prostate-specific membrane antigen-targeting agents induce DNA double-strand breaks in prostate cancer xenografts. Cancer Biother Radiopharm. (2017) 32:67–73. doi: 10.1089/cbr.2016.2155
84. Sathekge M, Knoesen O, Meckel M, Modiselle M, Vorster M, Marx S. 213Bi-PSMA-617 targeted alpha-radionuclide therapy in metastatic castration-resistant prostate cancer. Eur J Nucl Med Mol Imaging. (2017) 44:1099–100. doi: 10.1007/s00259-017-3657-9
85. Kratochwil C, Schmidt K, Afshar-Oromieh A, Bruchertseifer F, Rathke H, Morgenstern A, et al. Targeted alpha therapy of mCRPC: dosimetry estimate of 213Bismuth-PSMA-617. Eur J Nucl Med Mol Imaging. (2018) 45:31–7. doi: 10.1007/s00259-017-3817-y
86. Qaim SM, Scholten B, Neumaier B. New developments in the production of theranostic pairs of radionuclides. J Radioanalyt Nucl Chem. (2018) 318:1493–509. doi: 10.1007/s10967-018-6238-x
87. Qaim SM. Theranostic radionuclides: recent advances in production methodologies. J Radioanalyt Nucl Chem. (2019) 322:1257–66. doi: 10.1007/s10967-019-06797-y
88. Máthé D, Szigeti K, Hegedûs N, Horváth I, Veres DS, Kovács B, et al. Production and in vivo imaging of 203Pb as a surrogate isotope for in vivo 212Pb internal absorbed dose studies. Appl Radiat Isotopes. (2016) 114:1–6. doi: 10.1016/j.apradiso.2016.04.015
89. Stenberg VY, Juzeniene A, Chen Q, Yang X, Bruland ØS, Larsen RH. Preparation of the alpha-emitting prostate-specific membrane antigen targeted radioligand [212Pb]Pb-NG001 for prostate cancer. J Labelled Comp Radiopharm. (2020) 63:129–43. doi: 10.1002/jlcr.3825
90. Dos Santos JC, Schäfer M, Bauder-Wüst U, Lehnert W, Leotta K, Morgenstern A, et al. Development and dosimetry of 203Pb/212Pb-labelled PSMA ligands: bringing “the lead” into PSMA-targeted alpha therapy? Eur J Nucl Med Mol Imaging. (2019) 46:1081–91. doi: 10.1007/s00259-018-4220-z
91. Kiess AP, Minn I, Vaidyanathan G, Hobbs RF, Josefsson A, Shen C, et al. (2S)-2-(3-(1-Carboxy-5-(4-211At-Astatobenzamido)Pentyl)Ureido)-pentanedioic acid for PSMA-targeted α-particle radiopharmaceutical therapy. J Nucl Med. (2016) 57:1569. doi: 10.2967/jnumed.116.174300
92. Vaidyanathan G, Mease RC, Minn I, Choi J, Chen Y, Shallal H, et al. Synthesis and preliminary evaluation of 211At-labeled inhibitors of prostate-specific membrane antigen for targeted alpha particle therapy of prostate cancer. Nucl Med Biol. (2021) 94-95:67–80. doi: 10.1016/j.nucmedbio.2021.01.002
93. Taïeb D, Foletti J-M, Bardiès M, Rocchi P, Hicks RJ, Haberkorn U. PSMA-targeted radionuclide therapy and salivary gland toxicity: why does it matter? J Nucl Med. (2018) 59:747. doi: 10.2967/jnumed.118.207993
94. Kelly JM, Amor-Coarasa A, Ponnala S, Nikolopoulou A, Williams C, Thiele NA, et al. A single dose of 225Ac-RPS-074 induces a complete tumor response in an lncap xenograft model. J Nucl Med. (2019) 60:649. doi: 10.2967/jnumed.118.219592
95. Hammer S, Hagemann UB, Zitzmann-Kolbe S, Larsen A, Ellingsen C, Geraudie S, et al. Preclinical efficacy of a PSMA-targeted thorium-227 conjugate (PSMA-TTC), a targeted alpha therapy for prostate cancer. Clin Cancer Res. (2020) 26:1985. doi: 10.1158/1078-0432.CCR-19-2268
96. Virgolini I, Traub T, Novotny C, Leimer M. New trends in peptide receptor radioligands. Q J Nucl Med Mol Imaging. (2001) 45:153–9.
97. Strosberg J, El-Haddad G, Wolin E, Hendifar A, Yao J, Chasen B, et al. Phase 3 trial of 177Lu-dotatate for midgut neuroendocrine tumors. N Engl J Med. (2017) 376:125–35. doi: 10.1056/NEJMoa1607427
98. Stallons TAR, Saidi A, Tworowska I, Delpassand ES, Torgue JJ. Preclinical investigation of 212Pb-DOTAMTATE for peptide receptor radionuclide therapy in a neuroendocrine tumor model. Mol Cancer Ther. (2019) 18:1012. doi: 10.1158/1535-7163.MCT-18-1103
99. Delpassand E, Tworowska I, Shanoon F, Nunez R, Flores Ii L, Muzammil A, et al. First clinical experience using targeted alpha-emitter therapy with 212Pb-DOTAMTATE (AlphaMedix) in patients with SSTR(+) neuroendocrine tumors. J Nucl Med. (2019) 60:559.
100. Wohlrab J. Pharmacokinetic characteristics of therapeutic antibodies. J Dtsch Dermatol Ges. (2015) 13:530–4. doi: 10.1111/ddg.12648
101. Goldenberg DM, Deland F, Kim E, Bennett S, Primus FJ, Van Nagell JR, et al. Use of radiolabeled antibodies to carcinoembryonic antigen for the detection and localization of diverse cancers by external photoscanning. N Engl J Med. (1978) 298:1384–8. doi: 10.1056/NEJM197806222982503
102. Mahé M-A, Fumoleau P, Fabbro M, Guastalla J-P, Faurous P, Chauvot P, et al. A phase II study of intraperitoneal radioimmunotherapy with Iodine-131-labeled monoclonal antibody OC-125 in patients with residual ovarian carcinoma. Clin Cancer Res. (1999) 5:3249-53s.
103. Orozco JJ, Bäck T, Kenoyer A, Balkin ER, Hamlin DK, Wilbur DS, et al. Anti-CD45 radioimmunotherapy using 211At with bone marrow transplantation prolongs survival in a disseminated murine leukemia model. Blood. (2013) 121:3759–67. doi: 10.1182/blood-2012-11-467035
104. Li Y, Hamlin DK, Chyan M-K, Wong R, Dorman EF, Emery RC, et al. cGMP production of astatine-211-labeled anti-CD45 antibodies for use in allogeneic hematopoietic cell transplantation for treatment of advanced hematopoietic malignancies. PLoS One. (2018) 13:e0205135. doi: 10.1371/journal.pone.0205135
105. Caron PC, Jurcic JG, Scott AM, Finn RD, Divgi CR, Graham MC, et al. A phase 1B trial of humanized monoclonal antibody M195 (anti-CD33) in myeloid leukemia: specific targeting without immunogenicity. Blood. (1994) 83:1760–8. doi: 10.1182/blood.V83.7.1760.bloodjournal8371760
106. Simmons D, Seed B. Isolation of a cDNA encoding CD33, a differentiation antigen of myeloid progenitor cells. J Immunol. (1988) 141:2797.
107. Juricic JG, Caron PC, Nikula TK, Papadopoulos EB, Finn RD, Gansow OA, et al. Radiolabeled anti-CD33 monoclonal antibody M195 for myeloid leukemias. Cancer Res. (1995) 55:5908-10s.
108. Nikula TK, Mcdevitt MR, Finn RD, Wu C, Kozak RW, Garmestani K, et al. Alpha-emitting bismuth cyclohexylbenzyl DTPA constructs of recombinant humanized anti-CD33 antibodies: pharmacokinetics, bioactivity, toxicity and chemistry. J Nucl Med. (1999) 40:166.
109. Mcdevitt MR, Finn RD, Ma D, Larson SM, Scheinberg DA. Preparation of α-emitting 213Bi-labeled antibody constructs for clinical use. J Nucl Med. (1999) 40:1722.
110. Kolbert KS, Hamacher KA, Jurcic JG, Scheinberg DA, Larson SM, Sgouros G. Parametric images of antibody pharmacokinetics in Bi213-HuM195 therapy of leukemia. J Nucl Med. (2001) 42:27.
111. Jurcic JG, Larson SM, Sgouros G, Mcdevitt MR, Finn RD, Divgi CR, et al. Targeted α particle immunotherapy for myeloid leukemia. Blood. (2002) 100:1233–9. doi: 10.1182/blood.V100.4.1233.h81602001233_1233_1239
112. Rosenblat TL, Mcdevitt MR, Mulford DA, Pandit-Taskar N, Divgi CR, Panageas KS, et al. Sequential cytarabine and α-particle immunotherapy with Bismuth-213–Lintuzumab (HuM195) for acute myeloid leukemia. Clin Cancer Res. (2010) 16:5303. doi: 10.1158/1078-0432.CCR-10-0382
113. Mcdevitt MR, Ma D, Lai LT, Simon J, Borchardt P, Frank RK, et al. Tumor therapy with targeted atomic nanogenerators. Science. (2001) 294:1537. doi: 10.1126/science.1064126
114. Mcdevitt MR, Ma D, Simon J, Frank RK, Scheinberg DA. Design and synthesis of 225Ac radioimmunopharmaceuticals. Appl Radiat Isotopes. (2002) 57:841–7. doi: 10.1016/S0969-8043(02)00167-7
115. Jaggi JS, Seshan SV, Mcdevitt MR, Laperle K, Sgouros G, Scheinberg DA. Renal tubulointerstitial changes after internal irradiation with α-particle–emitting actinium daughters. J Am Soc Nephrol. (2005) 16:2677. doi: 10.1681/ASN.2004110945
116. Jaggi JS, Seshan SV, Mcdevitt MR, Sgouros G, Hyjek E, Scheinberg DA. Mitigation of radiation nephropathy after internal α-particle irradiation of kidneys. Int J Radiat Oncol Biol Phys. (2006) 64:1503–12. doi: 10.1016/j.ijrobp.2005.11.036
117. Jurcic JG, Rosenblat TL, Mcdevitt MR, Pandit-Taskar N, Carrasquillo JA, Chanel SM, et al. Phase I trial of the targeted alpha-particle nano-generator actinium-225 (225Ac)-Lintuzumab (Anti-CD33; HuM195) in acute myeloid leukemia (AML). Blood. (2011) 118:768. doi: 10.1182/blood.V118.21.768.768
118. Jurcic JG, Levy MY, Park JH, Ravandi F, Perl AE, Pagel JM, et al. Phase I trial of targeted alpha-particle therapy with actinium-225 (225Ac)-lintuzumab and low-dose cytarabine (LDAC) in patients age 60 or older with untreated acute myeloid leukemia (AML). Blood. (2016) 128:4050. doi: 10.1182/blood.V128.22.4050.4050
119. Atallah E, Berger M, Jurcic J, Roboz G, Tse W, Mawad R, et al. A phase 2 study of actinium-225 (225Ac)-lintuzumab in older patients with untreated acute myeloid leukemia (AML). J Med Imaging Radiat Sci. (2019) 50:S109. doi: 10.1016/j.jmir.2019.11.118
120. Tai Y-T, De Weers M, Li X-F, Song W, Nahar S, Bakker JM, et al. Daratumumab, a novel potent human anti-CD38 monoclonal antibody, induces significant killing of human multiple myeloma cells: therapeutic implication. Blood. (2009) 114:608. doi: 10.1182/blood.V114.22.608.608
121. Berger MS, Thomas K, Ekaterina D, Malo M, Jiao R, Allen K, et al. Actinium labeled daratumumab demonstrates enhanced killing of multiple myeloma cells over naked daratumumab. Blood. (2017) 130:4427.
122. Dadachova E, Dawicki W, Allen K, Jiao R, Malo M, Ludwig D. 225Ac-CD38 antibody targeting is effective and well tolerated in experimental models of lymphoma and multiple myeloma. J Nucl Med. (2019) 60:1410.
123. Ouban A, Muraca P, Yeatman T, Coppola D. Expression and distribution of insulin-like growth factor-1 receptor in human carcinomas. Hum Pathol. (2003) 34:803–8. doi: 10.1016/S0046-8177(03)00291-0
124. Hartog H, Wesseling J, Boezen HM, Van Der Graaf WTA. The insulin-like growth factor 1 receptor in cancer: old focus, new future. Eur J Cancer. (2007) 43:1895–904. doi: 10.1016/j.ejca.2007.05.021
125. Grinshtein N, Simms R, Hu M, Storozhuk Y, Moran M, Burak E, et al. IGF-1R targeted alpha therapeutic FPI-1434 causes DNA Double-stranded breaks and induces regression in preclinical models of human cancer. J Med Imaging Radiat Sci. (2019) 50:S86. doi: 10.1016/j.jmir.2019.11.061
126. Juergens RA, Zukotynski KA, Juneau D, Krnezich L, Simms R, Forbes J, et al. A phase I study of [225Ac]-FPI-1434 radioimmunotherapy in patients with IGF-1R expressing solid tumors. J Clin Oncol. (2019) 37:TS3152. doi: 10.1200/JCO.2019.37.15_suppl.TPS3152
127. Hassan R, Bera T, Pastan I. Mesothelin: a new target for immunotherapy. Clin Cancer Res. (2004) 10:3937. doi: 10.1158/1078-0432.CCR-03-0801
128. Hassan R, Ho M. Mesothelin targeted cancer immunotherapy. Eur J Cancer. (2008) 44:46–53. doi: 10.1016/j.ejca.2007.08.028
129. Hagemann UB, Hagelin E-M, Wickstroem K, Sponheim K, Smeets R, Karlsson J, et al. A novel high energy alpha-pharmaceutical: in vitro and in vivo potency of a mesothelin-targeted thorium-227 conjugate (TTC) in a model of bone disease. Cancer Res. (2016) 76:591. doi: 10.1158/1538-7445.AM2016-591
130. Hagemann UB, Ellingsen C, Schuhmacher J, Kristian A, Mobergslien A, Cruciani V, et al. Mesothelin-targeted thorium-227 conjugate (MSLN-TTC): preclinical evaluation of a new targeted alpha therapy for mesothelin-positive cancers. Clin Cancer Res. (2019) 25:4723. doi: 10.1158/1078-0432.CCR-18-3476
131. Mckeage K, Perry CM. Trastuzumab. Drugs. (2002) 62:209–43. doi: 10.2165/00003495-200262010-00008
132. Wolff AC, Hammond MEH, Schwartz JN, Hagerty KL, Allred DC, Cote RJ, et al. American society of clinical oncology/college of American pathologists guideline recommendations for human epidermal growth factor receptor 2 testing in breast cancer. Arch Pathol Lab Med. (2007) 131:18–43.
133. Verri E, Guglielmini P, Puntoni M, Perdelli L, Papadia A, Lorenzi P, et al. HER2/neu oncoprotein overexpression in epithelial ovarian cancer: evaluation of its prevalence and prognostic significance. Oncology. (2005) 68:154–61. doi: 10.1159/000086958
134. Nahta R, Esteva FJ. Herceptin: mechanisms of action and resistance. Cancer Lett. (2006) 232:123–38. doi: 10.1016/j.canlet.2005.01.041
135. Milenic DE, Garmestani K, Brady ED, Albert PS, Ma D, Abdulla A, et al. Targeting of HER2 antigen for the treatment of disseminated peritoneal disease. Clin Cancer Res. (2004) 10:7834. doi: 10.1158/1078-0432.CCR-04-1226
136. Milenic DE, Garmestani K, Brady ED, Albert PS, Ma D, Abdulla A, et al. α-Particle radioimmunotherapy of disseminated peritoneal disease using a 212Pb-labeled radioimmunoconjugate targeting HER2. Cancer Biother Radiopharm. (2005) 20:557–68. doi: 10.1089/cbr.2005.20.557
137. Milenic DE, Garmestani K, Brady ED, Albert PS, Abdulla A, Flynn J, et al. Potentiation of high-LET radiation by gemcitabine: targeting HER2 with trastuzumab to treat disseminated peritoneal disease. Clin Cancer Res. (2007) 13:1926. doi: 10.1158/1078-0432.CCR-06-2300
138. Meredith RF, Torgue J, Azure MT, Shen S, Saddekni S, Banaga E, et al. Pharmacokinetics and imaging of 212Pb-TCMC-trastuzumab after intraperitoneal administration in ovarian cancer patients. Cancer Biother Radiopharm. (2013) 29:12–7. doi: 10.1089/cbr.2013.1531
139. Meredith R, Torgue J, Shen S, Fisher DR, Banaga E, Bunch P, et al. Dose escalation and dosimetry of first-in-human α radioimmunotherapy with 212Pb-TCMC-trastuzumab. J Nucl Med. (2014) 55:1636. doi: 10.2967/jnumed.114.143842
140. Meredith RF, Torgue JJ, Rozgaja TA, Banaga EP, Bunch PW, Alvarez RD, et al. Safety and outcome measures of first-in-human intraperitoneal α radioimmunotherapy with 212Pb-TCMC-trastuzumab. Am J Clin Oncol. (2018) 41:716–21. doi: 10.1097/COC.0000000000000353
141. Heyerdahl H, Krogh C, Borrebæk J, Larsen Å, Dahle J. Treatment of HER2-expressing breast cancer and ovarian cancer cells with alpha particle-emitting 227Th-trastuzumab. Int J Radiat Oncol Biol Phys. (2011) 79:563–70. doi: 10.1016/j.ijrobp.2010.08.038
142. Helen H, Nasir A, Kristine S, Camilla M, Oyvind B, Jostein D. Targeted alpha therapy with 227Th-trastuzumab of intraperitoneal ovarian cancer in nude mice. Curr Radiopharm. (2013) 6:106–16. doi: 10.2174/18744710113069990018
143. Karlsson J, Hagemann UB, Schatz C, Grant D, Ellingsen C, Kristian A, et al. HER2-targeted thorium-227 conjugate (HER2-TTC): efficacy in a HER2 positive orthotopic bone model. Cancer Res. (2017) 77:5857. doi: 10.1158/1538-7445.AM2017-5857
144. Karlsson J, Hagemann UB, Schatz C, Grant D, Kristian A, Ellingsen C, et al. HER2-targeted thorium-227 conjugate (HER2-TTC): efficacy in preclinical models of trastuzumab and T-DM1 resistance. Cancer Res. (2017) 77:5859. doi: 10.1158/1538-7445.AM2017-5859
145. Wickstroem K, Karlsson J, Ellingsen C, Cruciani V, Kristian A, Hagemann UB, et al. Synergistic effect of a HER2 targeted thorium-227 conjugate in combination with olaparib in a BRCA2 deficient xenograft model. Pharmaceuticals. (2019) 12:155. doi: 10.3390/ph12040155
146. Pallares RM, Abergel RJ. Transforming lanthanide and actinide chemistry with nanoparticles. Nanoscale. (2020) 12:1339–48. doi: 10.1039/C9NR09175K
147. Yue J, Pallares RM, Cole LE, Coughlin EE, Mirkin CA, Lee A, et al. Smaller CpG-conjugated gold nanoconstructs achieve higher targeting specificity of immune activation. ACS Appl Mater Interfaces. (2018) 10:21920–6. doi: 10.1021/acsami.8b06633
148. Pallares RM, Choo P, Cole LE, Mirkin CA, Lee A, Odom TW. Manipulating immune activation of macrophages by tuning the oligonucleotide composition of gold nanoparticles. Bioconjug Chem. (2019) 30:2032–7. doi: 10.1021/acs.bioconjchem.9b00316
149. Xie D, Wang MP, Qi WH. A simplified model to calculate the surface-to-volume atomic ratio dependent cohesive energy of nanocrystals. J Phys Condensed Matter. (2004) 16:L401–5. doi: 10.1088/0953-8984/16/36/L01
150. Tiwari PM, Vig K, Dennis VA, Singh SR. Functionalized gold nanoparticles and their biomedical applications. Nanomaterials. (2011) 1:31–63. doi: 10.3390/nano1010031
151. Sedlmeier A, Gorris HH. Surface modification and characterization of photon-upconverting nanoparticles for bioanalytical applications. Chem Soc Rev. (2015) 44:1526–60. doi: 10.1039/C4CS00186A
152. Pallares RM, Carter KP, Zeltmann SE, Tratnjek T, Minor AM, Abergel RJ. Selective lanthanide sensing with gold nanoparticles and hydroxypyridinone chelators. Inorg Chem. (2020) 59:2030–6. doi: 10.1021/acs.inorgchem.9b03393
153. Laurent S, Forge D, Port M, Roch A, Robic C, Vander Elst L, et al. Magnetic iron oxide nanoparticles: synthesis, stabilization, vectorization, physicochemical characterizations, and biological applications. Chem Rev. (2008) 108:2064–110. doi: 10.1021/cr068445e
154. Pallares RM, Su X, Lim SH, Thanh NTK. Fine-tuning of gold nanorod dimensions and plasmonic properties using the Hofmeister effects. J Mater Chem C. (2016) 4:53–61. doi: 10.1039/C5TC02426A
155. Pallares RM, Wang Y, Lim SH, Thanh NTK, Su X. Growth of anisotropic gold nanoparticles in photoresponsive fluid for UV sensing and erythema prediction. Nanomedicine. (2016) 11:2845–60.
156. Pallares RM, Stilson T, Choo P, Hu J, Odom TW. Using good’s buffers to control the anisotropic structure and optical properties of spiky gold nanoparticles for refractive index sensing. ACS Appl Nano Mater. (2019) 2:5266–71. doi: 10.1021/acsanm.9b01117
157. Alkilany AM, Thompson LB, Boulos SP, Sisco PN, Murphy CJ. Gold nanorods: their potential for photothermal therapeutics and drug delivery, tempered by the complexity of their biological interactions. Adv Drug Deliv Rev. (2012) 64:190–9. doi: 10.1016/j.addr.2011.03.005
158. Pankhurst QA, Thanh NTK, Jones SK, Dobson J. Progress in applications of magnetic nanoparticles in biomedicine. J Phys D Appl Phys. (2009) 42:224001. doi: 10.1088/0022-3727/42/22/224001
159. Goodman AM, Hogan NJ, Gottheim S, Li C, Clare SE, Halas NJ. Understanding resonant light-triggered DNA release from plasmonic nanoparticles. ACS Nano. (2017) 11:171–9. doi: 10.1021/acsnano.6b06510
160. Hilderbrand SA, Shao F, Salthouse C, Mahmood U, Weissleder R. Upconverting luminescent nanomaterials: application to in vivo bioimaging. Chem Commun. (2009):4188–90. doi: 10.1039/b905927j
161. Shin T-H, Choi Y, Kim S, Cheon J. Recent advances in magnetic nanoparticle-based multi-modal imaging. Chem Soc Rev. (2015) 44:4501–16. doi: 10.1039/C4CS00345D
162. Pallares RM, Thanh NTK, Su X. Tunable plasmonic colorimetric assay with inverse sensitivity for extracellular DNA quantification. Chem Commun. (2018) 54:11260–3. doi: 10.1039/C8CC05465G
163. Pallares RM, Thanh NTK, Su X. Sensing of circulating cancer biomarkers with metal nanoparticles. Nanoscale. (2019) 11:22152–71. doi: 10.1039/C9NR03040A
164. Piotrowska A, Mȩczyńska-Wielgosz S, Majkowska-Pilip A, Koźmiński P, Wójciuk G, Cêdrowska E, et al. Nanozeolite bioconjugates labeled with 223Ra for targeted alpha therapy. Nucl Med Biol. (2017) 47:10–8. doi: 10.1016/j.nucmedbio.2016.11.005
165. Rojas JV, Woodward JD, Chen N, Rondinone AJ, Castano CH, Mirzadeh S. Synthesis and characterization of lanthanum phosphate nanoparticles as carriers for 223Ra and 225Ra for targeted alpha therapy. Nucl Med Biol. (2015) 42:614–20. doi: 10.1016/j.nucmedbio.2015.03.007
166. Pallares RM, Agbo P, Liu X, An DD, Gauny SS, Zeltmann SE, et al. Engineering mesoporous silica nanoparticles for targeted alpha therapy against breast cancer. ACS Appl Mater Interfaces. (2020) 12:40078–84. doi: 10.1021/acsami.0c11051
167. Mclaughlin MF, Woodward J, Boll RA, Wall JS, Rondinone AJ, Kennel SJ, et al. Gold coated lanthanide phosphate nanoparticles for targeted alpha generator radiotherapy. PLoS One. (2013) 8:e54531. doi: 10.1371/journal.pone.0054531
168. Karpov TE, Muslimov AR, Antuganov DO, Postovalova AS, Pavlov DA, Usov YV, et al. Impact of metallic coating on the retention of 225Ac and its daugthers within core–shell nanocarriers. J Colloid Interface Sci. (2022) 608:2571–83. doi: 10.1016/j.jcis.2021.10.187
169. Dziawer L, Koźmiński P, Mȩczyńska-Wielgosz S, Pruszyński M, Łyczko M, Wąs B, et al. Gold nanoparticle bioconjugates labelled with 211At for targeted alpha therapy. RSC Adv. (2017) 7:41024–32. doi: 10.1039/C7RA06376H
170. Cêdrowska E, Pruszynski M, Majkowska-Pilip A, Mȩczyńska-Wielgosz S, Bruchertseifer F, Morgenstern A, et al. Functionalized TiO2 nanoparticles labelled with 225Ac for targeted alpha radionuclide therapy. J Nanopart Res. (2018) 20:83. doi: 10.1007/s11051-018-4181-y
171. Sattiraju A, Pandya D, Wadas T, Xiong X, Sun Y, Jung Y, et al. Alpha particle enhanced permeabilization of the blood tumor barrier using alpha-v beta-3 (αvβ3) specific nanoparticles. J Nucl Med. (2016) 57:633.
172. Sattiraju A, Xiong X, Pandya DN, Wadas TJ, Xuan A, Sun Y, et al. Alpha particle enhanced blood brain/tumor barrier permeabilization in glioblastomas using integrin alpha-v beta-3–targeted liposomes. Mol Cancer Ther. (2017) 16:2191. doi: 10.1158/1535-7163.MCT-16-0907
173. Andrew Kyle Henderson R, Caterina Fortunata R, Paul S, Valery R. Development of 225Ac radiopharmaceuticals: TRIUMF perspectives and experiences. Curr Radiopharm. (2018) 11:156–72. doi: 10.2174/1874471011666180416161908
174. Engle JW. The production of Ac-225. Curr Radiopharm. (2018) 11:173–9. doi: 10.2174/1874471011666180418141357
175. Nelson BJB, Andersson JD, Wuest F. Targeted alpha therapy: progress in radionuclide production, radiochemistry, and applications. Pharmaceutics. (2021) 13:49. doi: 10.3390/pharmaceutics13010049
176. Zhuikov BL. Production of medical radionuclides in Russia: status and future—a review. Appl Radiat Isotopes. (2014) 84:48–56. doi: 10.1016/j.apradiso.2013.11.025
177. Hoehr C, Bénard F, Buckley K, Crawford J, Gottberg A, Hanemaayer V, et al. Medical isotope production at TRIUMF – from imaging to treatment. Phys Procedia. (2017) 90:200–8. doi: 10.1016/j.phpro.2017.09.059
178. John K, Birnbaum E, Boll R, Brown A, Brugh M, Cooley J, et al. US DOE tri-lab research effort to provide accelerator-produced 225Ac for radiotherapy: 2018 update. J Nucl Med. (2018) 59:1044.
179. Al Darwish R, Staudacher AH, Bezak E, Brown MP. Autoradiography imaging in targeted alpha therapy with timepix detector. Comput Math Methods Med. (2015) 2015:612580. doi: 10.1155/2015/612580
180. Usmani S, Rasheed R, Al Kandari F, Marafi F, Naqvi SAR. 225Ac prostate-specific membrane antigen posttherapy α imaging: comparing 2 and 3 photopeaks. Clin Nucl Med. (2019) 44:401–3. doi: 10.1097/RLU.0000000000002525
181. Vatsa R, Sood A, Vadi SK, Das CK, Kaur K, Parmar M, et al. 225Ac-PSMA-617 radioligand posttherapy imaging in metastatic castrate-resistant prostate cancer patient using 3 photopeaks. Clin Nucl Med. (2020) 45:437–8. doi: 10.1097/RLU.0000000000003031
182. Gosewisch A, Schleske M, Gildehaus FJ, Berg I, Kaiser L, Brosch J, et al. Image-based dosimetry for 225Ac-PSMA-I&T therapy using quantitative SPECT. Eur J Nucl Med Mol Imaging. (2021) 48:1260–1. doi: 10.1007/s00259-020-05024-1
183. Singh Jaggi J, Kappel BJ, Mcdevitt MR, Sgouros G, Flombaum CD, Cabassa C, et al. Efforts to control the errant products of a targeted in vivo generator. Cancer Res. (2005) 65:4888. doi: 10.1158/0008-5472.CAN-04-3096
184. Anselmo AC, Mitragotri S. Nanoparticles in the clinic: an update post COVID-19 vaccines. Bioengineer Transl Med. (2021) 6:e10246. doi: 10.1002/btm2.10246
Keywords: targeted alpha therapy, radiopharmaceuticals, immunoconjugates, targeted radiotherapy, actinium-225
Citation: Pallares RM and Abergel RJ (2022) Development of radiopharmaceuticals for targeted alpha therapy: Where do we stand? Front. Med. 9:1020188. doi: 10.3389/fmed.2022.1020188
Received: 25 August 2022; Accepted: 05 December 2022;
Published: 22 December 2022.
Edited by:
Gabriele Multhoff, Technical University of Munich, GermanyReviewed by:
Michael Rod Zalutsky, Duke University, United StatesAdriano Duatti, University of Ferrara, Italy
Copyright © 2022 Pallares and Abergel. This is an open-access article distributed under the terms of the Creative Commons Attribution License (CC BY). The use, distribution or reproduction in other forums is permitted, provided the original author(s) and the copyright owner(s) are credited and that the original publication in this journal is cited, in accordance with accepted academic practice. No use, distribution or reproduction is permitted which does not comply with these terms.
*Correspondence: Rebecca J. Abergel, ✉ YWJlcmdlbEBiZXJrZWxleS5lZHU=
†Present Address: Roger M. Pallares, Institute for Experimental Molecular Imaging, RWTH Aachen University Hospital, Aachen, Germany
‡ORCID: Roger M. Pallares, orcid.org/0000-0001-7423-8706; Rebecca J. Abergel, orcid.org/0000-0002-3906-8761