- 1Istituto di Fisica Applicata “Nello Carrara”, Consiglio Nazionale delle Ricerche (IFAC-CNR), Florence, Italy
- 2Skin Centers, Avezzano, Italy
- 3Laser Cutaneous Cosmetic and Plastic Surgery Unit, Villa Donatello Clinic, Florence, Italy
- 4Department of Dermatology and Venereology, Dermatology Clinic, Maggiore Hospital, University of Trieste, Trieste, Italy
- 5El.En Group, Calenzano, Italy
Introduction
Aging and sunlight exposure induce different changes in facial skin. Furthermore, collagen synthesis and the dermal lifespan of fibroblasts are reduced. As a result, an overall reduction of collagen and elastic fibers in the dermis leads to deep wrinkles, skin laxity, and post-inflammatory hyperpigmentation (PHI), characteristics typical of photoaged skin (1, 2). To date, several types of lasers for skin aging are marketed.
Manstein (3) was the first to introduce the concept of fractional photothermolysis or fractional resurfacing Carbon Dioxide (CO2) laser. This new approach is based on the generation of microscopic columns of thermal damage (both in ablation and in coagulation) at the interface between epidermis and dermis, which stimulate a wound healing response (4, 5) and collagen synthesis (6, 7). Furthermore, in vivo experiments conducted by Cohen (8) showed that fractional treatments with 1,540 nm affect coagulation, collagen production, and dermal remodeling, leading to skin rejuvenation and benefits in acne scars and stretch marks.
It has also been observed that a solution of near-infrared (NIR) sources in which coagulation is not sought is a 1540 nm fractional erbium-glass laser system, which has many advantages over other lasers, including regulation of penetration depth (2–3 mm) (9). However, these conditions cannot induce tissue alterations but only reversible action, which has significant biological effects on biostimulation: it causes an increment in blood flow, cytokines, and growth factor changes. Generally speaking, changes in growth factors and cytokines might be essential in the pathogenesis of the 1540 nm fractional erbium-glass laser-induced wound healing, hair regrowth and cellular metabolism (10). Fractional ablative lasers have a higher safety profile than traditional ones (5). However, only the fractional ablative CO2 laser requires higher energies to reach different reticular depths, which could induce hyperpigmentation and energy-related prolonged bleeding. In addition, these post-treatment reactions should be associated with acute inflammatory responses to skin heat damage leading to increased side effects, such as persistent erythema, skin changes, PHI, scarring, and prolonged healing time (11, 12). On the other hand, the lower absorption coefficient of 1540 and 1550 nm devices results in a greater maximum depth of 1400 μm (13).
This different mode of delivering laser energy reduces the post-treatment erythema and recovery times (14). Furthermore, the thermal injury generated by non-ablative laser remains spatially confined to the dermis, while the surrounding skin enables fast recovery of damaged tissue. Complete re-epithelialization is usually observed within 24 to 48 h (5, 15).
Fractional CO2 laser treatment stimulates a molecular cascade that can lead to wound healing, collagen remodeling and scar regression (10, 16–20). These molecular processes also evoke an increase in type III collagen synthesis (21), and histological analysis clearly shows neocollagenesis, epidermal thickening and an increase in elastic fibers (22).
It is well known that during the aging process, due to photoaging, the skin shows a reduction in type I and type III collagen (23). The synergistic combination of ablative and non-ablative laser sources could improve the effect on the tissue. The simultaneous emission of the CO2 laser and the bipolar radiofrequency is a viable and possible alternative, which provides epidermal coagulation and tissue remodeling due to the production of new collagen in the dermis. This methodology can modulate the coagulation and ablative effects, affecting healing times compared to typical CO2 laser stimulation (24, 25), reducing patient downtime and pain, and improving skin rejuvenation results (26, 27).
This dual wavelength system aims to couple the ablative aspect of CO2 laser and the deep non-ablative e non-coagulative properties to enhance the effects of CO2 laser while limiting the side effects. For this purpose, the DuoGlide system (DEKA Mela, Florence, Italy) was used in this study. This technology combines the two wavelengths (CO2 and 1540 nm), but the possibility of excluding the CO2 allowed us to perform an in vitro study of the non-coagulative thermal effect of 1540 nm.
The effect of the 1540 nm wavelength on cultured fibroblast is known to upregulate the expression of collagen-related synthesis genes and, at the same time, to downregulate matrix proteins production (28–30). On these bases, our research aimed to evaluate the photobiomodulation effect of the 1540 nm wavelength treatment on the proliferation of cultured fibroblast and their ability to express type I and III collagen.
Materials and methods
Laser device description
The DuoGlide system (DEKA Mela Srl, Florence, Italy) is a laser with a new design that incorporates a 10.6 μm Carbon Dioxide (CO2) laser device (60 W) and a 1540 nm laser (10 W), which can be used with the fractional scanning units (μScan DOT). This scanner can deliver one or both wavelengths (1540 nm and 10.6 μm) in a sequential emission mode on the same microthermal zone (DOT) separated by healthy tissue (DOT spacing) DOT; this allows for a tunable balance between ablation and coagulation depths and for delivery of new and more efficient treatments. Furthermore, the second wavelength of 1540 nm conveyed through the new miniaturized scanning systems can achieve homogeneous, continuous and non-coagulative heating of the entire scanning area, reaching further and deeper into the dermis (not gently reachable with the ablative laser alone), thanks to spots of the order of 1000 μm emitted on the same axis as the DOT and thanks to the use of the typical CO2 spacing parameters (~500 μm) used in the literature for dermatological applications (25).
Cell culture
Adult Human Dermal Fibroblast cells (HDFa, Lot# 2207322) were purchased from Thermo Fisher Scientific (Waltham, Massachusetts, USA) and used following the recommendation of the manufacturer. HDFa were cultivated in Dulbecco Modified Eagle Medium (DMEM, PAN-Biotech GmbH, Aidenbach, Germany) added with 10% of Fetal Bovine Serum (FBS), 1% of Glutamine and Streptomycin (PAN-Biotech GmbH, Aidenbach, Germany). Cells were kept under standard culture conditions (37 °C and 5% CO2) and the DMEM was refreshed every 48 h.
Sample irradiation
To perform a colourimetric assay, HDFa cells were counted using a Neubauer chamber (Karl Hecht Assistent GmbH, Sondheim vor der Rhön, Germany), and 8 x 103 cells were seeded in 96-multiwell plates (Greiner Bio-One Italia, Milan, Italy). Before the experiments, cells were seeded in rows and columns for each multiwell in alternate wells to avoid double or partial irradiation. Next, each sample was maintained for 24 h in standard culture conditions in DMEM without FBS. Then, HDFa cells were subjected to irradiation.
Cytotoxicity and proliferation assay
Cellular viability was evaluated 24 and 48 h after the treatment using the Cell Counting Kit-8 (CCK-8) assay (Sigma-Aldrich, Milan, Italy). The CCK-8 uses WST-8 reagent, which is bioreduced by mitochondrial dehydrogenases and becomes WST-8 formazan with an orange color soluble in the tissue culture medium (31, 32). Proliferation was measured by Sulforodhamine B-based assay (SRB, Sigma-Aldrich, Milan, Italy) 24 and 48 h after irradiation. This test is based on the capability of the protein-dye sulforhodamine B to bind electrostatically and pH-dependent on basic amino acid residues on cells. The quantification of the bound dye can serve as an approximation of total cell biomass, thus cell proliferation (33–35). Absorbance at 450 nm and 570 nm for CCK-8 and SRB, respectively (reference wavelength at 630 nm), were read using an automatic microplate absorbance reader equipped with SkanIt software (Multiskan FC Micro-plate Photometer, Thermo Fisher Scientific, Milan, Italy). Each experiment was performed at least in triplicate.
Immunocytochemical staining and fluorescence quantification
HDFa cells were cultivated in 35 mm2 dishes suitable for confocal acquisition (Ibidi GmbH, Martinsried, Germany). Cells were left homogeneously adhered to the bottom of the petri dish to avoid bias during the subsequent analysis steps. The immunocytochemical protocol was previously described (36). Briefly, a 3.6% paraformaldehyde solution was used to fix HDFa cells. After two washes in phosphate buffer saline (PBS), cells were permeabilised using Triton-X100 for 10 min at room temperature. The unspecific sites were blocked using 10% goat serum diluted in PBS and 0.1% Tween20 (PBST). Primary antibodies were diluted as follows: anti-type I collagen (1:400) and anti-type III collagen (1:200), both in PBST solution. Secondary antibodies AlexaFluor555 and AlexaFluor647 were diluted at 1:500 in PBST. All the antibodies were purchased from AbCam (Cambridge, UK) and used according to the manufacturer's instructions. Fixed cells were incubated with the secondary antibodies for control experiments to exclude non-specific binding. A mounting medium with DAPI (4′,6-diamidino-2-phenylindole, Sigma-Aldrich, Milan, Italy) was used to stain cell nuclei and mount the coverslip. Immunocytochemical images were obtained by SP8 laser scanning confocal microscope (Leica Microsystems, Mannheim, Germany) using a 20x dry objective (NA 0.4). All the photos from treated and control samples were acquired keeping the same microscope parameters (gain, laser intensity, scan area and speed. The collected images obtained were analyzed with open-source software [ImageJ (37)]. Each image was separated into two channels corresponding to the fluorescence signal for a specific type of collagen (type I or type III). The fluorescence intensity analysis was performed over the entire image area (38).
Statistical analysis
The CCK-8 and SRB assay data were expressed as mean±SD. In addition, the Kruskal-Wallis test followed by Dunn's test for multiple comparisons were performed. The data obtained from immunofluorescence analysis were described as mean±SEM. The Mann-Whitney two-tailed test was selected. Statistical significance was set for all the experimental results at *p < 0.05. All the data were analyzed using the commercial software GraphPad Prism 8th edition (San Diego, CA, USA).
Results
Evaluation of cytotoxicity and cell proliferation after cell irradiation
Data obtained from the CCK-8 assay showed that all the tested fluences (J/cm2) (2.1, 2.8, 3.5, 4.2) did not affect cellular viability 24 and 48 h after treatment (Figure 1A). On the other hand, the SRB assay demonstrated that the application of 3.5 and 2.8 J/cm2 induced a significant increase in cell proliferation compared to the untreated samples. This increase occurred 24 h after the treatment, but was not observed after 48 h (Figure 1B).
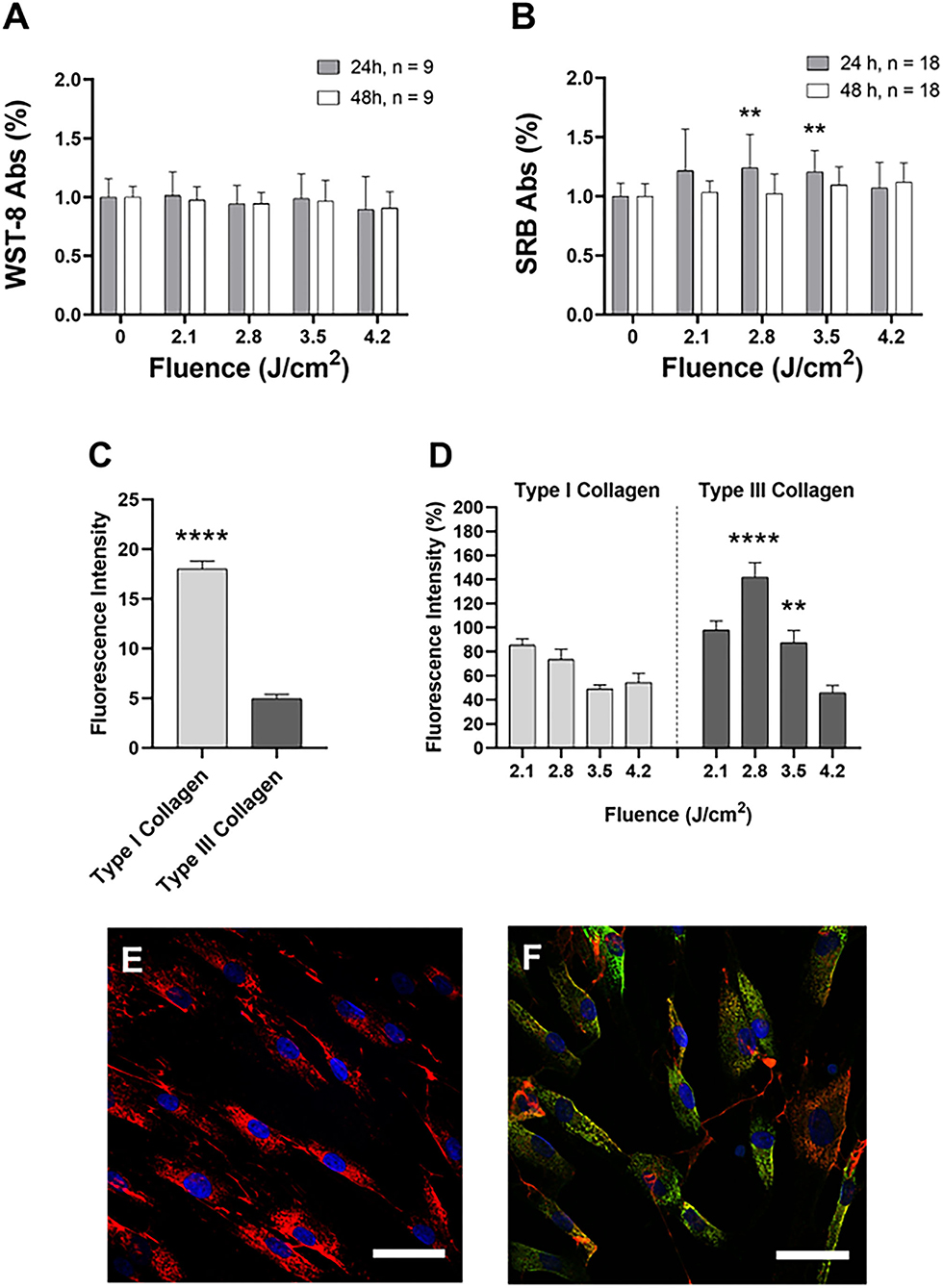
Figure 1. Cell viability (A) and proliferation (B) after 24 h (gray) and 48 h (white) after the irradiation. Data are expressed as mean ± SD, n = 9 (A) and n = 18 (B). Statistical analysis: **p < 0.01 (vs. 0 fluence). The one-way ANOVA, Kruskal-Wallis test followed by Dunn's test of multiple comparisons post-hoc. (C) Fluorescence intensity of type I and type III collagen in untreated cells: collagen was reported in light gray, type III collagen in dark gray. Data are expressed as mean±SEM, n = 20. Statistical analysis: Mann-Whitney two-tailed test, ****p < 0.0001. (D) Fluorescence intensity of type I collagen (light gray) and type III collagen (dark gray) compared at the same applied dose. Data are expressed as mean ± SD, n = 20. Statistical analysis: Mann-Whitney two-tailed test, **p < 0.01; ****p < 0.0001 (vs. the same fluence). Example of confocal images of untreated (E) and treated (F) HDFa cells. Type I collagen: red; type III collagen: green; cell nuclei: blue. Representative images were acquired with a 63x oil objective (NA 1.4). Scalebar: 100 μm.
Confocal analysis of type I and type III collagen
Firstly, we evaluated the fluorescence intensity of the basal expression of collagen in HDFa cells not subjected to irradiation. Figure 1C shows that type I collagen is significantly higher than type III collagen. In Figure 1D, the application of 3.5 and 2.8 J/cm2 induced a significant increase in fluorescence intensity related to type III collagen.
Discussion
The extracellular matrix (ECM) is responsible to the physiological properties of the skin and its architectural organization; the ECM consists of many elements, including collagen fibers. Fibroblasts synthesize collagen so that the skin contains typically from 80 to 85% type I collagen and from 10 to 15% type III collagen. Chronologically aged skin reduces collagen types I and III due to skin photoaging (23). Radiation activates cellular mechanisms which cause clinical manifestations of skin photoaging, such as wrinkles, pigmentation, telangiectasias and neoplasm (39, 40) Nitrous proteins and Cytochrome C oxidase are always considered the main targets of light (41–43). However, recent studies have shown that the cryptochromes and opsins (44, 45) are responsible for the cellular response to visible and UV light. The hypothesis therefore could be that a photon of light could hit several molecular targets by activating molecular targets such as reactive oxygen species (ROS), adenosine triphosphate (ATP) and ionized channels (44, 46) thus activating multiple signaling cascades. It is already well established that NIR light, as well as the visible spectrum, can induce a versatile and a significant range of changes in the expression pathways of several genes, leading to modifications in cell differentiation and proliferation, as well as collagen synthesis (47–49). Indeed, a positive effect on the induction of type I collagen production with 1,320 nm wavelength was also observed during the wound healing process (50).
The results obtained in this study are in line with the scientific literature concerning the rearrangement of types I and III collagen after laser therapy, suggesting a neocollagenesis activation (51, 52). Indeed, our results revealed a significant increase in type III collagen expression improvement, confirming the laser-induced neocollagenesis effect. Furthermore, our data demonstrate that irradiation with medium fluences of 1540 nm modulates cell proliferation. Concerning this evidence, even if there is no evidence in the literature of the possible effects of the laser in the various phases of the fibroblasts cell cycle, our findings indicate an increase in the cellular activity of the mitochondrial electrical potential following laser treatment.
Author contributions
Conceptualization: FR and LP. Methodology and data curation: GM. Validation: GM, LP, DP, PB, CC, GC, and FR. Formal analysis and writing—original draft preparation: GM and LP. Investigation: GM, LP, and IF. Writing—review and editing: FR and IF. Supervision and funding acquisition: FR. All authors have read and agreed to the published version of the manuscript.
Conflict of interest
LP and IF are employed at El.En. Group.
The remaining authors declare that the research was conducted in the absence of any commercial or financial relationships that could be construed as a potential conflict of interest.
The handling editor GT declared a shared affiliation with the author CC at the time of review.
Publisher's note
All claims expressed in this article are solely those of the authors and do not necessarily represent those of their affiliated organizations, or those of the publisher, the editors and the reviewers. Any product that may be evaluated in this article, or claim that may be made by its manufacturer, is not guaranteed or endorsed by the publisher.
References
1. Farage MA, Miller KW, Elsner P, Maibach HI. Intrinsic and extrinsic factors in skin ageing: a review. Int J Cosmet. (2008) 30:87–95. doi: 10.1111/j.1468-2494.2007.00415.x
2. Rittié L, Fisher G.J. UV-light-induced signal cascades and skin aging. Ageing Res Rev. (2002) 1:705–20. doi: 10.1016/S1568-1637(02)00024-7
3. Manstein D, Herron GS, Sink RK, Tanner H, Anderson RR. Fractional photothermolysis: a new concept for cutaneous remodeling using microscopic patterns of thermal injury. Lasers Surg Med. (2004) 34:426–38. doi: 10.1002/lsm.20048
4. Laubach HJ, Tannous Z, Anderson RR, Manstein D. Skin responses to fractional photothermolysis. Lasers Surg Med. (2006) 38:142–9. doi: 10.1002/lsm.20254
5. Alexiades-Armenakas MR, Dover JS, Arndt KA. The spectrum of laser skin resurfacing: Nonablative, fractional, and ablative laser resurfacing. J Am Acad Dermatol. (2008) 58:719–37. doi: 10.1016/j.jaad.2008.01.003
6. Hantash BM, Bedi VP, Kapadia B, Rahman Z, Jiang K, Tanner H, et al. In vivo histological evaluation of a novel ablative fractional resurfacing device. Lasers Surg Med. (2007) 39, 96–107. doi: 10.1002/lsm.20468
7. Anderson RR, Donelan MB, Hivnor C, Greeson E, Ross EV, Shumaker PR, et al. Laser treatment of traumatic scars with an emphasis on ablative fractional laser resurfacing: consensus report. JAMA Dermatol. (2014) 150:187–93. doi: 10.1001/jamadermatol.2013.7761
8. Solomon-Cohen E, Lapidoth M, Mimouni D, Akerman L, Slodownik D, Hodak E, et al. 1540-nm fractional erbium: glass laser is a safe and effective modality for non-ablative facial rejuvenation. J Cosmet Dermatol. (2021) 20:1679–83. doi: 10.1111/jocd.13958
9. Modena DAO, Miranda ACG, Grecco C, Liebano RE, Cordeiro RCT, Guidi RM. Efficacy, safety, and guidelines of application of the fractional ablative laser erbium YAG 2940 nm and non-ablative laser erbium glass in rejuvenation, skin spots, and acne in different skin phototypes: a systematic review. Lasers Med Sci. (2020) 35:1877–88. doi: 10.1007/s10103-020-03046-7
10. Alhattab MK, Al Abdullah MJ, Al-janabi MH, Aljanaby WA, Alwakeel HA. The effect of 1540-nm fractional erbium-glass laser in the treatment of androgenic alopecia. J Cosmet Dermatol. (2020) 19:878–83. doi: 10.1111/jocd.13122
11. Tarijian AL, Goldberg DJ. Fractional ablative laser skin resurfacing: a review. J Cosmet Laser Ther. (2011) 13:262–4. doi: 10.3109/14764172.2011.630083
12. Cannarozzo G, Sannino M, Tamburi F, Chiricozzi A, Saraceno R, Morini C, et al. Deep pulse fractional CO2 laser combined with a radiofrequency system: results of a case series. Photomed Laser Surg. (2014) 32:409–12. doi: 10.1089/pho.2014.3733
13. Chen SX, Cheng J, Watchmaker J, Dover JS, Chung HJ. Review of lasers and energy-based devices for skin rejuvenation and scar treatment with histologic correlations. Dermatol Surg. (2022) 48:441–8. doi: 10.1097/DSS.0000000000003397
14. Neaman KC, Baca ME, Piazza RC, VanderWoude DL, Renucci JD. Outcomes of fractional CO2 laser application in aesthetic surgery: a retrospective review. Aesthetic Surg J. (2010) 30:845–52. doi: 10.1177/1090820X10386930
15. Bedi VP, Chan KF, Sink RK, Hantash BH, Herron GS, Rahman Z, et al. The effects of pulse energy variations on the dimensions of microscopic thermal treatment zones in non-ablative fractional resurfacing. Lasers Surg Med. (2007) 39:145–55. doi: 10.1002/lsm.20406
16. Shumaker PR, Kwan JM, Landers JT, Uebelhoer NS. Functional improvements in traumatic scars and scar contractures using an ablative fractional laser protocol. J Trauma Acute Care Surg. (2012) 73:S116–21. doi: 10.1097/TA.0b013e318260634b
17. Waibel JS, Gianatasio C, Rudnick A, Siegel A. Use of lasers in wound healing: how to best utilize laser technology to prevent scar formation. Curr Dermatol Rep. (2018) 7:303–10. doi: 10.1007/s13671-018-0240-y
18. Fournier N, Dahan S, Barneon G, Diridollou S, Lagarde JM, Gall Y, et al. Nonablative remodeling: clinical, histologic, ultrasound imaging, and profilometric evaluation of a 1540 nm Er:glass laser. Dermatol Surg. (2001) 27:799–806. doi: 10.1046/j.1524-4725.2001.00355.x
19. Souil E, Capon A, Mordon S, Dinh-Xuan AT, Polla BS, Bachelet M. Treatment with 815-nm diode laser induces long-lasting expression of 72-kDa heat shock protein in normal rat skin. Br J Dermatol. (2001) 144, 260–6. doi: 10.1046/j.1365-2133.2001.04010.x
20. Ayuk SM, Abrahamse H, Houreld NN. The role of matrix metalloproteinases in diabetic wound healing in relation to photobiomodulation. J Diabetes Res. (2016) 2016. doi: 10.1155/2016/2897656
21. Haedersdal M, Moreau KER, Beyer DM, Nymann P, Alsbjorn B. Fractional non-ablative 1540 nm laser resurfacing for thermal burn scars: a randomized controlled trial. Lasers Surg Med. (2009) 41:189–195. doi: 10.1002/lsm.20756
22. De Angelis F, Franco R. Fractional nonablative 1540-nm laser treatment of striae distensae in Fitzpatrick skin types II to IV: clinical and histological results. Aesthet Surg J. (2011) 31:411–9. doi: 10.1177/1090820X11402493
23. El-Domyati M, Attia S, Saleh F, Brown D, Birk DE, Gasparro F, et al. Intrinsic aging vs. photoaging: a comparative histopathological, immunohistochemical, and ultrastructural study of skin. Exp Dermatol. (2002) 11:398–405. doi: 10.1034/j.1600-0625.2002.110502.x
24. Cameli N, Mariano M, Serio M, Ardigò M. Preliminary comparison of fractional laser with fractional laser plus radiofrequency for the treatment of acne scars and photoaging. Dermatol Surg. (2014) 40:553–561. doi: 10.1111/dsu.12470
25. Tenna, S, Cogliandro, A, Piombino, L, Filoni, A, Persichetti, P. Combined use of fractional CO2 laser and radiofrequency waves to treat acne scars: a pilot study on 15 patients. J Cosmet Laser Ther. (2012) 87:166–171. doi: 10.3109/14764172.2012.699678
26. Kislevitz M, Lu KB, Wamsley C, Hoopman J, Kenkel J, Akgul Y. Novel use of non-invasive devices and microbiopsies to assess facial skin rejuvenation following laser treatment. Lasers Surg Med. (2020) 52:822–830. doi: 10.1002/lsm.23233
27. Mezzana P, Valeriani M, Valeriani R. Combined fractional resurfacing (10600 nm/1540 nm): tridimensional imaging evaluation of a new device for skin rejuvenation. J Cosmet Laser Ther. (2016) 18:397–402. doi: 10.1080/14764172.2016.1202417
28. Hopps E, Lo Presti R, Montana M, Noto D, Averna MR, Caimi G. Gelatinases and their tissue inhibitors in a group of subjects with metabolic syndrome. J Investig Med. (2013) 61:978–83. doi: 10.2310/JIM.0b013e318294e9da
29. Menghini R, Uccioli L, Vainieri E, Pecchioli C, Casagrande V, Stoehr R, et al. Expression of tissue inhibitor of metalloprotease 3 is reduced in ischemic but not neuropathic ulcers from patients with type 2 diabetes mellitus. Acta Diabetol. (2013) 50:907–10. doi: 10.1007/s00592-013-0478-6
30. Ayuk SM, Abrahamse H, Houreld NN. Photobiomodulation alters matrix protein activity in stressed fibroblast cells in vitro. J Biophotonics. (2018) 11:e201700127. doi: 10.1002/jbio.201700127
31. Jo HY, Kim Y, Park HW, Moon HE, Bae S, Kim J, et al. The unreliability of MTT assay in the cytotoxic test of primary cultured glioblastoma cells. Exp Neurobiol. (2015) 24:235–245. doi: 10.5607/en.2015.24.3.235
32. Krassovka J, Borgschulze A, Sahlender B, Lögters T, Windolf J, Grotheer V. Blue light irradiation and its beneficial effect on Dupuytren's fibroblasts. PLoS ONE. (2019) 14:e0209833. doi: 10.1371/journal.pone.0209833
33. Orellana E, Kasinski A. Sulforhodamine B (SRB) assay in cell culture to investigate cell proliferation. Bio-protocol. (2016) 21:e1984. doi: 10.21769/BioProtoc.1984
34. Vichai V, Kirtikara K. Sulforhodamine B colorimetric assay for cytotoxicity screening. Nat Protoc. (2006) 1:1112–6. doi: 10.1038/nprot.2006.179
35. Skehan P, Storeng R, Scudiero D, Monks A, Mcmahon J, Vistica D, et al. New colorimetric cytotoxicity assay for anticancer-drug screening. JNCI J Natl Cancer Inst. (1990) 82:1107–12. doi: 10.1093/jnci/82.13.1107
36. Magni G, Banchelli M, Cherchi F, Coppi E, Fraccalvieri M, Rossi M, et al. Experimental study on blue light interaction with human keloid-derived fibroblasts. Biomedicines. (2020) :573. doi: 10.3390/biomedicines8120573
37. Schindelin J, Arganda-Carreras I, Frise E, Kaynig V, Longair M, Pietzsch T, et al. Fiji: an open-source platform for biological-image analysis. Nat Methods. (2012) 9:676–82. doi: 10.1038/nmeth.2019
38. Zerbinati N, Calligaro A. Calcium hydroxylapatite treatment of human skin: evidence of collagen turnover through picrosirius red staining and circularly polarized microscopy. Clin Cosmet Investig Dermatol. (2018) 11:29. doi: 10.2147/CCID.S143015
39. Kraft DC, Deocaris CC, Rattan SIS. Proteasomal oscillation during mild heat shock in aging human skin fibroblasts. Ann N Y Acad Sci. (2006) 1067:224–7. doi: 10.1196/annals.1354.028
40. Manela-Azulay M, Cuzzi T, Pinheiro JCA, Azulay DR, Rangel G.B. Objective methods for analyzing outcomes in research studies on cosmetic dermatology. An Bras Dermatol. (2010) 85:65–71. doi: 10.1590/S0365-05962010000100009
41. Mignon C, Botchkareva NV, Uzunbajakava NE, Tobin DJ. Photobiomodulation devices for hair regrowth and wound healing: a therapy full of promise but a literature full of confusion. Exp Dermatol. (2016) 25:745–9. doi: 10.1111/exd.13035
42. Karu TI. Cellular and molecular mechanisms of photobiomodulation (low-power laser therapy). IEEE J Sel Top Quantum Electron. (2014) 20. doi: 10.1109/JSTQE.2013.2273411
43. Chung H, Dai T, Sharma SK, Huang YY, Carroll JD, Hamblin MR. The nuts and bolts of low-level laser (Light) therapy. Ann Biomed Eng. (2012) 40:516–33. doi: 10.1007/s10439-011-0454-7
44. Hamblin MR, Hamblin MR. Mechanisms and applications of the anti-inflammatory effects of photobiomodulation. AIMS Biophys. (2017) 4:337–61. doi: 10.3934/biophy.2017.3.337
45. Garza ZCF, Born M, Hilbers PAJ, van Riel NAW, Liebmann J. Visible blue light therapy: molecular mechanisms and therapeutic opportunities. Curr Med Chem. (2017) 25:5564–77. doi: 10.2174/0929867324666170727112206
46. Wang Y, Huang YY, Wang Y, Lyu, P, Hamblin MR. Red (660 nm) or near-infrared (810 nm) photobiomodulation stimulates, while blue (415 nm), green (540 nm) light inhibits proliferation in human adipose-derived stem cells. Sci Rep. (2017) 7:1–10. doi: 10.1038/s41598-017-07525-w
47. Kim JE, Woo YJ, Sohn KM, Jeong KH, Kang H. Wnt/β-catenin and ERK pathway activation: a possible mechanism of photobiomodulation therapy with light-emitting diodes that regulate the proliferation of human outer root sheath cells. Lasers Surg Med. (2017) 49:940–7. doi: 10.1002/lsm.22736
48. Sassoli C, Chellini F, Squecco R, Tani A, Idrizaj E, Nosi D, et al. Low intensity 635 nm diode laser irradiation inhibits fibroblast–myofibroblast transition reducing TRPC1 channel expression/activity: new perspectives for tissue fibrosis treatment. Lasers Surg Med. (2016) 48:318–332. doi: 10.1002/lsm.22441
49. Mamalis A, Garcha, M, Jagdeo J. Light emitting diode-generated blue light modulates fibrosis characteristics: fibroblast proliferation, migration speed, and reactive oxygen species generation. Lasers Surg Med. (2015) 47:210–5. doi: 10.1002/lsm.22293
50. Nelson BR, Majmudar G, Griffiths CEM, Gillard MO, Dixon AE, Tavakkol A, et al. Clinical improvement following dermabrasion of photoaged skin correlates with synthesis of collagen I. Arch Dermatol. (1994) 130:1136–42. doi: 10.1001/archderm.1994.01690090060008
51. El-Domyati M, Abd-El-Raheem T, Abdel-Wahab H, Medhat W, Hosam W, et al. Fractional versus ablative erbium:yttrium-aluminum-garnet laser resurfacing for facial rejuvenation: an objective evaluation. J Am Acad Dermatol. (2013) 68:103–12. doi: 10.1016/j.jaad.2012.09.014
Keywords: carbon dioxide laser, skin rejuvenation, collagen synthesis, human dermal fibroblasts, confocal microscopy
Citation: Magni G, Piccolo D, Bonan P, Conforti C, Crisman G, Pieri L, Fusco I and Rossi F (2022) 1540-nm fractional laser treatment modulates proliferation and neocollagenesis in cultured human dermal fibroblasts. Front. Med. 9:1010878. doi: 10.3389/fmed.2022.1010878
Received: 03 August 2022; Accepted: 29 August 2022;
Published: 18 October 2022.
Edited by:
Giusto Trevisan, University of Trieste, ItalyReviewed by:
Gianluca Avallone, University of Turin, ItalyAntonio Caldaria, University of Ferrara, Italy
Copyright © 2022 Magni, Piccolo, Bonan, Conforti, Crisman, Pieri, Fusco and Rossi. This is an open-access article distributed under the terms of the Creative Commons Attribution License (CC BY). The use, distribution or reproduction in other forums is permitted, provided the original author(s) and the copyright owner(s) are credited and that the original publication in this journal is cited, in accordance with accepted academic practice. No use, distribution or reproduction is permitted which does not comply with these terms.
*Correspondence: Irene Fusco, aS5mdXNjb0BkZWthLml0
†ORCID: Irene Fusco orcid.org/0000-0001-7264-8808