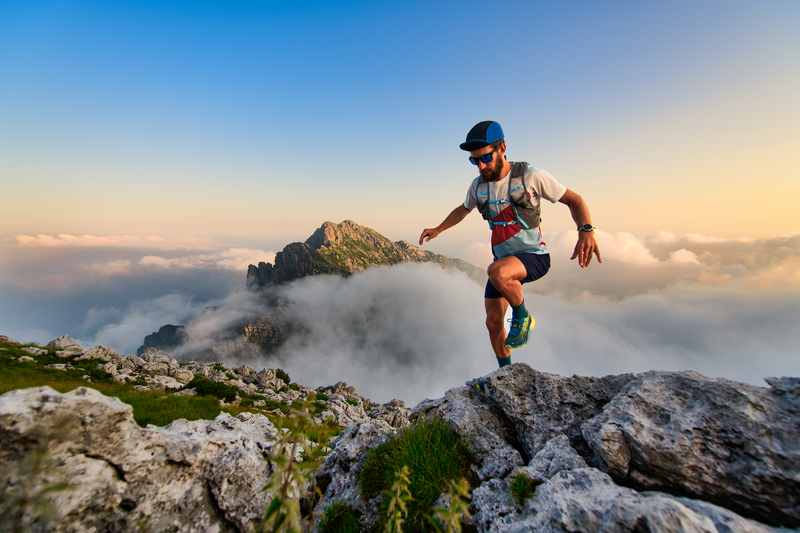
94% of researchers rate our articles as excellent or good
Learn more about the work of our research integrity team to safeguard the quality of each article we publish.
Find out more
ORIGINAL RESEARCH article
Front. Med. , 03 February 2022
Sec. Nephrology
Volume 8 - 2021 | https://doi.org/10.3389/fmed.2021.803874
This article is part of the Research Topic Tissue Microenvironment in Kidney Diseases View all 7 articles
A correction has been applied to this article in:
Corrigendum: HIF-1α Alleviates High-Glucose-Induced Renal Tubular Cell Injury by Promoting Parkin/PINK1-Mediated Mitophagy
It is well-established that mitophagy leads to Diabetic Nephropathy (DN) and renal failure. Mitophagy mediated by a Hypoxia-inducible factor-1α (HIF-1α) plays a beneficial role in many diseases. Nevertheless, the mechanisms underlying HIF-1α-mediated mitophagy in DN remain unclear. This study defines the role of HIF-1α mediated mitophagy in DN. The expression of HIF-1α was upregulated in HK-2 cells in an High-Glucose (HG) environment, and the YC-1 (a specific inhibitor of HIF-1α) further exacerbated the hypoxia-induced mitochondrial dysfunction. Conversely, the HIF-1α-mediated protective effect was strengthened by scavenger N-acetylcysteine (NAC), a type of reactive oxygen species. Moreover, HIF-1α-Parkin/PINK1-mediated mitophagy prevented apoptosis and ROS production in HK-2 cells subjected to HG exposure. In summary, HIF-1α mediated mitophagy on HK-2 cells under HG conditions could alleviate DN, suggesting that it has huge prospects for DN treatment.
The development of the global economy has been paralleled by lifestyle changes, resulting in an elevated incidence of type 2 diabetes mellitus, which has gradually became a serious public health and safety concern worldwide (1). One major complication of diabetes mellitus is the Diabetic nephropathy (DN) (2), which is the primary cause of an end-stage renal disease (3), not only seriously affecting the quality of life of the patient but also imposes a heavy financial burden on society (4). Regrettably, the etiopathogenesis of DN remains to be elucidated, and therapy is limited by the paucity of effective drugs. Accordingly, it is of great significance to thoroughly explore the pathogenesis of DN and to further search for effective therapeutic targets.
Diabetic tubulopathy has an extensive-expression in DN development which contributes to initial renal injury in the pathogenesis of DN (5, 6). Renal tubular hypoxia is reportedly a pathological change found in the early and advanced stages of DN (7), eing an important pathogenic factor causing renal fibrosis. Hypoxia-inducible factor (HIF)-1 is a pivotal molecule that plays an important part in a hypoxic environment. The HIF-1 is widely acknowledged as a heterodimeric transcriptional factor consisting of an oxygen-sensitive α subunit and constitutively expressed β subunit (8–10). An increasing body of evidence suggests that high glucose levels upregulate HIF-1α expression in animal models and human renal proximal tubular cells of type 2 diabetes mellitus nephropathy (11–13). Nevertheless, the precise role of HIF-1α of diabetic nephropathy in the etiopathogenesis remains unclear.
Mitophagy is a special type of autophagy that was first proposed by LeMasters in 2005 (14). Mitophagy selectively clears damaged or unwanted mitochondria and fuses with lysosomes to degrade the redundant mitochondria through different signaling pathways, such as PINK1/parkin and Nix/BNIP3 (6, 15), to maintain the reactive oxygen species (ROS) balance (16). Mitochondrial dysfunction has been documented as an important mechanism in the pathogenesis of renal tubular injury in DN (17, 18), leading to a dysfunctional mitochondrial accumulation, excessive ROS production, renal tubular cell injury, and apoptosis (19). Treatment with mitochondria-targeted antioxidants, such as mitoQ, has been reported to alleviate mitophagy and tubular injury (20). Moreover, studies have corroborated that HIF1α-mediated mitophagy plays a beneficial role in acute kidney injury by inhibiting tubular cell apoptosis and ROS production (21, 22). Nevertheless, whether HIF1α alleviates renal tubular cell injury by promoting mitophagy in an HG environment remains unknown.
In the present study, the renal tubular epithelial cells were subjected to HG exposure. This study explores the effects of HIF1α-mediated mitophagy and ascertain whether the Parkin/PINK1 signaling pathway was involved in this process.
Human proximal tubular epithelial cells HK-2 were cultured in DMEM/F-12 medium supplemented with 10% fetal bovine serum (FBS, Gibco), 1,000 U/L penicillin, and 100 μg/ml streptomycin at 37°C in 5% CO2 air. The cells were treated with normal glucose (NG, 5.5 mM D-glucose), high-glucose (HG, 30 mM D-glucose) for 24 h with or without 10 μM HIF1A inhibitor YC-1, and ROS scavenger NAC (Sigma-Aldrich, A7250, Germany) at a final concentration of 5 mM.
To assess mitochondrial superoxide production, HK-2 cells were incubated with MitoSOX (0.5 μM mol/L, Thermo Fisher, United States) for 15 min. The MitoSOX fluorescence images were obtained by a fluorescence microscope (Olympus CKX53, Japan). The maximum excitation wavelength of MitoSOX was set at 510 nm, and the discharge wavelength was 552–620 nm. Image J software was used to analyze the average fluorescent intensity.
The HK-2 cells were treated with adenovirus expressing GFP-LC3. Two days later, the samples were fixed with 4% paraformaldehyde, permeated with methanol/acetone, and infected with TOM20 antibody (1:200, Abcam, ab186734, United States). The samples were then observed under a fluorescence confocal microscope (LSM510 META, Karl Zeiss, Germany).
Cells were dissolved and lysed in a radioimmunoprecipitation (RIPA) buffer with protease after centrifuging at 13,000 g for 10 min. Mitochondrial fragments were collected using an isolation kit (Nanjing Jiancheng Bioengineering Inc., China). The antibodies used in this study are as follows: Anti-VDAC (1:4000, Abcam, ab14734), Anti-LC3II (1:1500, CST, #3868), Anti-Parkin (1:1000, Abcam, ab77924), Anti-PINK1 (1:1500, Abcam, ab216144), Anti-SQSTM1/P62 (1:5000, CST, #5114), Anti-HIF2a (1:1000, CST, #57921), Anti-HIF1a (1:2000, CST, #3716), Anti-E-Cadherin (1:2000, CST, #14472), Anti-Fibronectin/FN (1:2000, CST, #26836), Anti-a-SMA (1:2000, CST, # 19245), and Anti-SGLT1 (1:2000, Abcam, ab14686). The experiments were repeated more than three times, and the images were captured after incubation in an enhanced chemiluminescence (ECL) reagent. The density of the labeled protein bands which were normalized to β-actin was quantified with a Quantity One software.
Cells were seeded in 6-well plates for 24 h and then transfected with the indicated plasmids. After 24–36 h, the cells were collected and washed with ice-cold PBS 3 times and gently resuspended in a 500 μl binding buffer. Then, the cells were stained with Annexin V/FITC. Subsequently, Propidium iodide (PI) was added into the buffer and incubated for another 10 min in the dark. Finally, the stained cells were analyzed using CytoFLEX (BECKMAN COULTER).
IL-1β and IL-18 levels were quantitatively measured using ELISA test kits (Dakewe, Shenzhen, China; ABclonal, Wuhan, China) in accordance with the instructions of the manufacturer. The absorbance was measured with a Thermo Scientific microplate reader (Model 680, Bio-Rad, Hercules, CA, USA) at 450 nm.
The data were expressed as mean ± SEM. The unpaired two-tailed t-test was used for comparison between two groups. Analysis of variance or repeated analysis of variance was used for multiple comparisons. Graphpad prism® 6.0 software was used for statistical analysis.
To observe the effect of HIF-1α on mitochondrial autophagy under high glucose conditions, we first extracted the mitochondrial component protein from the cell homogenate and then carried out the western blot experiments. The LC3-II was downregulated while the autophagy substrate p62 was upregulated in the high-glucose group compared to the normal glucose group (Figure 1A), suggesting that high glucose could potently inhibit mitophagy. After specific inhibition of HIF-1α with YC-1, mitophagy activation levels were decreased in the HG + YC-1 group compared with the HG group, with reduced LC3-II levels and increased p62 levels (Figure 1A). Given the role of ROS in the regulation of mitophagy, the reactive oxygen species scavenger NAC was added to explore whether HIF-1α regulates mitophagy in HK-2 cells under high-glucose conditions. Importantly, the inhibitory effect of high glucose and YC-1 on mitochondrial autophagy was significantly reversed by NAC treatment (Figure 1A). Confocal microscopy showed that the binding strength between LC3-II and mitochondria was decreased in HK-2 cells under high-glucose conditions and was enhanced by YC-1 treatment but reversed by NAC (Figure 1D). These results indicate that HIF-1α may play a role in mitochondrial autophagy in HK-2 cells exposed to the HG environment.
Figure 1. Effect of HIF-1α on mitophagy-related proteins in HK-2cells subjected to HG exposure. (A–C). The expression of p62 and LC3-II was assessed by Western blot in HK-2 cells treated with normal glucose, high-glucose for 24 h with or without 10 μM HIF-1α inhibitor YC-1, and reactive oxygen species (ROS) scavenger N-Acetyl-L-Cysteine (NAC) at a final concentration of 5 mm.*p < 0.05. vs. indicated group. (D) Mitophagy was assessed using a fluorescence confocal microscope. HG, High Glucose; LC3-II, Microtubule-Associated-Proteinlight-Chain-3 II; HIF-1α, Hypoxia-Inducible Factor-1; YC-1, Lificiguat; ROS, Reactive Oxygen Species; NAC, N-Acetyl-L-Cysteine.
Then, we examined whether parkin/PINK1 is a downstream regulatory factor in HIF1α-related mitophagy. The expression of parkin and PINK1 was significantly decreased in HK-2 cells exposed to the HG environment, and the decrease was further exacerbated by YC-1 (Figure 2A). The parkin and PINK1 expression were upregulated after adding the ROS scavenger NAC, indicating that high glucose levels induced inhibition of the parkin/PINK1 signaling pathway, which was reversed by the HIF-1α inhibitor (Figure 2A). These results showed that parkin/PINK1 is a downstream regulatory factor in an HIF-1α- related mitophagy.
Figure 2. HIF-1α activated parkin/PINK1 pathway in HK-2cells subjected to HG exposure. (A–C) Expression of parkin and PINK1 was measured by Western blot in HK-2 cells treated with normal glucose (NG, 5.5 mM D-glucose), high-glucose (HG, 30mM D-glucose) for 24 h with or without 10 μM HIF-1α inhibitor YC- 1, and ROS scavenger NAC at a final concentration of 5 mm.*p < 0.05. vs. indicated group. HIF-1α, Hypoxia-Inducible Factor-1; Parkin PINK1, Parkin/PTEN Induced Putative Kinase1; YC-1, Lificiguat; ROS, Reactive Oxygen Species; NAC, N-Acetyl-L-Cysteine; HG, High Glucose.
Subsequently, we investigated whether a HIF1α-Parkin/PINK1-mediated mitophagy exerted a protective effect by decreasing apoptosis and ROS production in HK-2 cells exposed to the HG environment. We examined the effect of HIF- 1α on apoptosis under high glucose conditions by flow cytometry after labeling HK-2 cells with annexin V and PI, respectively. Annexin V-positive cells were significantly increased in HK-2 cells exposed to the HG environment, which was enhanced by YC-1 (Figures 3A,B). Similarly, ROS production is upregulated under HG conditions, and YC-1 further enhanced this phenomenon (Figure 3C). Interestingly, the stimulatory effect of YC-1 on apoptosis and ROS production was substantially inhibited by NAC (Figure 3C). This finding suggests that HIF-1α-Parkin/PINK1-mediated mitophagy might exert a protective effect by suppressing apoptosis and ROS production. Importantly, these results demonstrate that HIF-1α-Parkin/PINK1-mediated mitophagy protects renal tubular cells from apoptosis and ROS production when exposed to HG conditions.
Figure 3. HIF-1α-Parkin/PINK1-mediated mitophagy prevented apoptosis and ROS production in HK-2 cells subjected to HG exposure. (A,B). The level of apoptosis was quantified using CytoFLEX after annexin V and PI labeling. Apoptosis levels were significantly increased under high glucose conditions and further increased with YC- 1. This effect was reversed with NAC. *p < 0.05. vs. indicated group. (C,D). Mitochondrial ROS production was measured using Image J software after MitoSOX staining. High glucose and inhibition of HIF-1α significantly increased intracellular ROS levels, while NAC reversed this trend. *p < 0.05. vs. indicated group. HIF-1α, Hypoxia-Inducible Factor-1; Parkin/PINK1, Parkin/PTEN Induced Putative Kinase1; YC-1, Lificiguat; ROS, Reactive Oxygen Species; NAC, N-Acetyl-L-Cysteine; HG, High Glucose.
IL-1b is one of the main components of IL-1b and the main regulator of tissue inflammation. IL-18 is also a member of the proinflammatory cytokine family. ELISA was performed to quantify the levels of these two cytokines. The results showed that the inflammatory factors were significantly increased in the high glucose group, and inhibition of HIF-1α could substantially promote the release of these inflammatory factors (Figures 4A,B). We subsequently observed that NAC reversed the pro-inflammatory effect associated with the HIF-1α inhibitor. These results demonstrate that HIF-1α may reduce HG-mediated inflammation in HK-2 cells.
Figure 4. Inhibition of HIF-1α significantly promoted the degree of HG-induced inflammation. (A,B). Inflammatory cytokines IL-1β and IL-18 in HK-2 cells subjected to HG exposure were quantitatively measured by ELISA. The cytokine levels were further increased with YC- 1 and reversed with NAC. *p < 0.05. vs. indicated group. HIF-1α, Hypoxia-Inducible Factor-1; HG, High Glucose; IL-β, Interleukin-1β; IL-18, Interleukin-18; YC-1, Lificiguat; NAC, N-Acetyl-L-Cysteine.
To investigate the role of HIF-1α on the epithelial-mesenchymal transition process in HK-2 cells exposed to HG environment, we extracted the total protein of HK-2 cells after different treatments and performed Western blot experiments. The results pointed out that the expression of FN and a-SMA were significantly up-regulated, and E-cadherin expression was decreased under high glucose treatment (Figure 5A), suggesting that HK-2 cells underwent epithelial-mesenchymal transition EMT. The expression of FN and α-SMA was further increased, and E-cadherin expression was further decreased after the addition of YC-1 (a specific inhibitor of HIF-1α) (Figure 5A), indicating further enhancement of EMT in HK-2 cells. We further found that NAC (an ROS scavenger) significantly reversed the pro-EMT effect of the HIF-1α inhibitor in a high glucose environment. These results suggest that HIF-1α inhibited epithelial-mesenchymal transition (EMT) in HK-2 cells exposed to HG environment.
Figure 5. HIF-1 α reduced epithelial-mesenchymal transition (EMT) in HK-2 cells subjected to HG exposure. (A–G). Western blot analysis revealed that FN and a-SMA were significantly increased, while E-cadherin expression was significantly decreased in HK-2 cells subjected to HG conditions. EMT, Epithelial-Mesenchymal Transition; HG, High Glucose; FN, Fibronectin; A-SMA, Alpha-Smooth Muscle Actin.
Diabetic renal tubular lesions are widely involved in the development of diabetic kidney disease (DKD). It has been established that during the pathogenesis of DKD, renal tubular injury leads to primary renal injury (23, 24), which is mirrored by an increase in renal tubular injury markers before microalbuminuria. However, the mechanism underlying this phenomenon remains largely unclear.
As one of the important cell organelles, mitochondria provide most of the energy required by the body and are an important source of ROS. Mitochondria are highly dynamic organelles. Its function maintenance is one of the foundations to ensure the normal operation of cells. Mitochondrial homeostasis depends on the balance between mitophagy and mitochondrial biogenesis (25). This balance is crucial to repair damaged mitochondria and ensure normal mitochondrial function (26–28). Mitophagy has been shown to play an indispensable protective role in different disease models of DN and ischemia-reperfusion injury (29). In a mouse model of acute renal injury (AKI) induced by cisplatin, changes in mitochondrial autophagy significantly exacerbated AKI (30). Moreover, inhibiting autophagy in proximal convoluted tubules with chloroquine or knocking out autophagy gene-related 7 (Atg7) molecules led to tissue damage, renal insufficiency, and apoptosis (31). In contrast, rapamycin has been found to activate the mitochondrial autophagy pathway and to reduce cisplatin-induced AKI renal tubular injury. Interestingly, one study showed that an HIF-1α- BNIP 3 mediated mitochondrial autophagy exerts a protective effect against AKI by decreasing renal tubular cell apoptosis, suggesting that HIF-1α plays an important role in hypoxia-induced mitophagy (13, 32). Consistent with the literature, our study indicated that mitophagy plays an important role in DN. Interestingly, recent studies have found that mitophagy is correlated to renal tubular disease in DN. The present study found that HIF-1α alleviates HG-mediated renal tubular cell injury by promoting Parkin/PINK1-mediated mitophagy.
Although HIF-1α plays different roles in many cellular processes [for instance, autophagic degradation, cellular survival, energy homeostasis, and angiogenesis (16)], little is known on the relationship between HIF-1α and autophagy, especially mitophagy. The present study indicated that HIF-1α alleviated high-glucose-induced renal tubular cell injury (33), a phenomenon that was accompanied by a decreased cell apoptosis. The HIF-1α exerted a protective effect in regulating mitophagy via promoting the Parkin/PINK1 signaling pathway (13). Moreover, a decreased ROS synthesis mediated by HIF-1α may account for the enhanced mitophagic activity (34). Taken together, these results exhibit a new mechanism by which HIF-1α protects against hyperglycemia-induced oxidative injury and enhances mitophagic activity, at least partially via Parkin/PINK1 signaling pathway in tubular cells (35).
The PINK1/parkin is the most studied mitophagic pathway (15, 36). The PINK1 leads to the degradation of mitochondrial protein via contact with the surface of the depolarized mitochondrion and inducing Parkin translocation. Zhan et al. observed that a high-glucose environment induced a decreased PINK1/Parkin expression, LC3, and mitophagy in renal tubular epithelial cells in Streptozotocin (STZ)-induced DN mice, which caused mitochondrial ROS (mROS) overproduction, mitochondrial fission, and apoptosis (37). Consistently, Xiao et al. found that db/db mice exhibited reduced PINK1/Parkin and LC3 expression. In the meantime, p62 accumulation in renal tubular epithelial cells results in mitophagy disorders, mitochondrial dysfunction, and apoptosis (38). Nevertheless, the upstream molecular mechanisms of mitophagy in DN have been understudied, warranting further studies.
In 1971, Loschek first demonstrated that the mitochondrial respiratory chain could produce oxygen free radicals, leading to ROS synthesis. The hyperglycemic state in diabetes can lead to an enlarged mitochondrial inner membrane potential difference and formation of an inner membrane hyperpolarossification. Electrons transported from coenzyme Q to cytochromes can reduce oxygen molecules to water and, thus, producing large amounts of ROS. In addition, the inner membrane of mitochondria is highly concentrated in unsaturated fatty acids and hence vulnerable to ROS. Accordingly, the mitochondria are the primary site of ROS synthesis in vivo and are conversely the most sensitive to oxidative damage by ROS (39). We observed that the NAC increased mitophagy and reduced ROS levels under HG conditions, and this phenomenon was exacerbated by inhibition of HIF-1α. The results of this study provide compelling evidence that HG exposure leads to an increased mitophagic activity and the release of large quantities of ROS.
Overall, this study demonstrates that the HIF-1α alleviates high-glucose-mediated renal tubular cell injury by promoting Parkin/PINK1-mediated mitophagy. These findings provide the basis for future studies on the pathogenesis of DN and provide important information for disease prevention and treatment.
The original contributions presented in the study are included in the article/Supplementary Material, further inquiries can be directed to the corresponding author/s.
LY, LT, and YW designed, performed, and analyzed the experiments. LY wrote and revised the manuscript. LY, YW, ZY, and YG carried out the data collection, data analysis, and revised the manuscript. LT revised the manuscript and applied for funding support. LY and LT confirm the authenticity of the raw data. All authors contributed to the article and approved the submitted version.
The present study was supported by the National Natural Science Foundation of China (grant no. U1904134) and Henan Province Young and Middle-aged Health Science and Technology Innovative Talents (Leader) Project (grant no. YXKC2020014).
The authors declare that the research was conducted in the absence of any commercial or financial relationships that could be construed as a potential conflict of interest.
All claims expressed in this article are solely those of the authors and do not necessarily represent those of their affiliated organizations, or those of the publisher, the editors and the reviewers. Any product that may be evaluated in this article, or claim that may be made by its manufacturer, is not guaranteed or endorsed by the publisher.
The Supplementary Material for this article can be found online at: https://www.frontiersin.org/articles/10.3389/fmed.2021.803874/full#supplementary-material
1. Simpson K, Wonnacott A, Fraser DJ, Bowen T. MicroRNAs in diabetic nephropathy: from biomarkers to Therapy. Curr Diab Rep. (2016) 16:35. doi: 10.1007/s11892-016-0724-8
2. Krolewski AS. Progressive renal decline: the new paradigm of diabetic nephropathy in type 1 diabetes. Diabetes Care. (2015) 38:954–62. doi: 10.2337/dc15-0184
3. Yang D, Livingston MJ, Liu Z, Dong G, Zhang M, Chen JK. Autophagy in diabetic kidney disease: regulation, pathological role and therapeutic potential. Cell Mol Life Sci. (2018) 75:669–88. doi: 10.1007/s00018-017-2639-1
4. Liu M, Liu SW, Wang LJ, Bai YM, Zeng XY, Guo HB, et al. Burden of diabetes, hyperglycaemia in China from to 2016: findings from the 1990 to 2016, global burden of disease study. Diabetes Metab. (2019) 45:286–93. doi: 10.1016/j.diabet.2018.08.008
5. Brezniceanu ML, Liu F, Wei CC, Chénier I, Godin N, Zhang SL, et al. Attenuation of interstitial fibrosis and tubular apoptosis in db/db transgenic mice overexpressing catalase in renal proximal tubular cells. Diabetes. (2008) 57:451–9. doi: 10.2337/db07-0013
6. Watanabe J, Takiyama Y, Honjyo J, Makino Y, Fujita Y, Tateno M, et al. Role of IGFBP7 in diabetic nephropathy: TGF-beta1 induces IGFBP7 via Smad2/4 in human renal proximal tubular epithelial cells. PLoS ONE. (2016) 11:e0150897. doi: 10.1371/journal.pone.0150897
7. Miyata T, De Strihou CV. Diabetic nephropathy: a disorder of oxygen metabolism? Nat Rev Nephrol. (2010) 6:83–95. doi: 10.1038/nrneph.2009.211
8. Wang GL, Jiang BH, Semenza GL. Effect of protein kinase and phosphatase inhibitors on expression of hypoxia-inducible factor 1. Biochem Biophys Res Commun. (1995) 216:669–75. doi: 10.1006/bbrc.1995.2674
9. Huang LE, Gu J, Schau M, Bunn HF. Regulation of hypoxia-inducible factor 1alpha is mediated by an O2-dependent degradation domain via the ubiquitin-proteasome pathway. Proc Natl Acad Sci USA. (1998) 95:7987–92. doi: 10.1073/pnas.95.14.7987
10. Wang GL, Jiang BH, Rue EA, Semenza GL. Hypoxia-inducible factor 1 is a basic-helix-loop-helix-PAS heterodimer regulated by cellular O2 tension. Proc Natl Acad Sci USA. (1995) 92:5510–4. doi: 10.1073/pnas.92.12.5510
11. Bessho R, Takiyama Y, Takiyama T, Kitsunai H, Takeda Y, Sakagami H, et al. Hypoxia-inducible factor-1alpha is the therapeutic target of the SGLT2 inhibitor for diabetic nephropathy. Sci Rep. (2019) 9:14754. doi: 10.1038/s41598-019-51343-1
12. Jiang N, Zhao H, Han Y, Li L, Xiong S, Zeng L, et al. HIF-1alpha ameliorates tubular injury in diabetic nephropathy via HO-1-mediated control of mitochondrial dynamics. Cell Prolif. (2020) 53:e12909. doi: 10.1111/cpr.12909
13. Persson P, Palm F. Hypoxia-inducible factor activation in diabetic kidney disease. Curr Opin Nephrol Hypertens. (2017) 26:345–50. doi: 10.1097/MNH.0000000000000341
14. Hamacher-Brady A, Brady NR. Mitophagy programs: mechanisms and physiological implications of mitochondrial targeting by autophagy. Cell Mol Life Sci. (2016) 73:775–95. doi: 10.1007/s00018-015-2087-8
15. Zhu J, Wang KZ, Chu CT. After the banquet: mitochondrial biogenesis, mitophagy, and cell survival. Autophagy. (2013) 9:1663–76. doi: 10.4161/auto.24135
16. Li Q, Liang X, Yang Y, Zeng X, Zhong X, Huang C. Panax notoginseng saponins ameliorate cisplatin-induced mitochondrial injury via the HIF-1alpha/mitochondria/ROS pathway. FEBS Open Bio. (2020) 10:118–26. doi: 10.1002/2211-5463.12760
17. Coughlan MT, Nguyen TV, Penfold SA, Higgins GC, Thallas-Bonke V, Tan SM, et al. Mapping time-course mitochondrial adaptations in the kidney in experimental diabetes. Clin Sci (Lond). (2016) 130:711–20. doi: 10.1042/CS20150838
18. Gou R, Chen J, Sheng S, Wang R, Fang Y, Yang Z, et al. KIM-1 mediates high glucose-induced autophagy and apoptosis in renal tubular epithelial cells. Cell Physiol Biochem. (2016) 38:2479–88. doi: 10.1159/000445598
19. Higgins GC, Coughlan MT. Mitochondrial dysfunction and mitophagy: the beginning and end to diabetic nephropathy? Br J Pharmacol. (2014) 171:1917–42. doi: 10.1111/bph.12503
20. Hou Y, Li S, Wu M, Wei J, Ren Y, Du C, et al. Mitochondria-targeted peptide SS-31 attenuates renal injury via an antioxidant effect in diabetic nephropathy. Am J Physiol Renal Physiol. (2016) 310:F547–59. doi: 10.1152/ajprenal.00574.2014
21. Chen G, Gao X, Wang J, Yang C, Wang Y, Liu Y, et al. Hypoxia-induced microRNA-146a represses Bcl-2 through Traf6/IRAK1 but not Smad4 to promote chondrocyte autophagy. Biol Chem. (2017) 398:499–507. doi: 10.1515/hsz-2016-0211
22. Gong G, Hu L, Liu Y, Bai S, Dai X, Yin L, et al. Upregulation of HIF-1alpha protein induces mitochondrial autophagy in primary cortical cell cultures through the inhibition of the mTOR pathway. Int J Mol Med. (2014) 34:1133–40. doi: 10.3892/ijmm.2014.1850
23. Fiseha T, Tamir Z. Urinary markers of tubular injury in early diabetic nephropathy. Int J Nephrol. (2016) 2016:4647685. doi: 10.1155/2016/4647685
24. Slyne J, Slattery C, McMorrow T, Ryan MP. New developments concerning the proximal tubule in diabetic nephropathy: in vitro models and mechanisms. Nephrol Dial Transplant. (2015) 30(Suppl. 4):iv60–7. doi: 10.1093/ndt/gfv264
25. Lemasters JJ. Selective mitochondrial autophagy, or mitophagy, as a targeted defense against oxidative stress, mitochondrial dysfunction, and aging. Rejuvenation Res. (2005) 8:3–5. doi: 10.1089/rej.2005.8.3
26. Lu H, Li G, Liu L, Feng L, Wang X, Jin H. Regulation and function of mitophagy in development and cancer. Autophagy. (2013) 9:1720–36. doi: 10.4161/auto.26550
27. Shaltouki A, Hsieh CH, Kim MJ, Wang X. Alpha-synuclein delays mitophagy and targeting Miro rescues neuron loss in Parkinson's models. Acta Neuropathol. (2018) 136:607–20. doi: 10.1007/s00401-018-1873-4
28. Tang C, Han H, Yan M, Zhu S, Liu J, Liu Z, et al. PINK1-PRKN/PARK2 pathway of mitophagy is activated to protect against renal ischemia-reperfusion injury. Autophagy. (2018) 14:880–97. doi: 10.1080/15548627.2017.1405880
29. Liang Q, Kobayashi S. Mitochondrial quality control in the diabetic heart. J Mol Cell Cardiol. (2016) 95:57–69. doi: 10.1016/j.yjmcc.2015.12.025
30. Lei R, Zhao F, Tang CY, Luo M, Yang SK, Cheng W. Mitophagy plays a protective role in iodinated contrast-induced acute renal tubular epithelial cells injury. Cell Physiol Biochem. (2018) 46:975–85. doi: 10.1159/000488827
31. Jiang M, Wei Q, Dong G, Komatsu M, Su Y, Dong Z. Autophagy in proximal tubules protects against acute kidney injury. Kidney Int. (2012) 82:1271–83. doi: 10.1038/ki.2012.261
32. Jiao Y, Jiang H, Lu H, Yang Y, Zhang Y, Zhang K, et al. Deficiency of hypoxia inducible factor-1alpha promoted progression of diabetic nephropathy with hypertension. Exp Ther Med. (2018) 16:3658–62. doi: 10.3892/etm.2018.6621
33. Ndibalema AR, Kabuye D, Wen S, Li L, Li X, Fan Q. Empagliflozin protects against proximal renal tubular cell injury induced by high glucose via regulation of hypoxia-inducible factor 1-Alpha. Diabetes Metab Syndr Obes. (2020) 13:1953–67. doi: 10.2147/DMSO.S243170
34. Zhang H, Bosch-Marce M, Shimoda LA, Tan YS, Baek JH, Wesley JB, et al. Mitochondrial autophagy is an HIF-1-dependent adaptive metabolic response to hypoxia. J Biol Chem. (2008) 283:10892–903. doi: 10.1074/jbc.M800102200
35. Bohuslavova R, Cerychova R, Nepomucka K, Pavlinkova G. Renal injury is accelerated by global hypoxia-inducible factor 1 alpha deficiency in a mouse model of STZ-induced diabetes. BMC Endocr Disord. (2017) 17:48. doi: 10.1186/s12902-017-0200-8
36. Wu W, Xu H, Wang Z, Mao Y, Yuan L, Luo W, et al. PINK1-parkin-mediated mitophagy protects mitochondrial integrity and prevents metabolic stress-induced endothelial injury. PLoS ONE. (2015) 10:e0132499. doi: 10.1371/journal.pone.0132499
37. Zhan M, Usman IM, Sun L, Kanwar YS. Disruption of renal tubular mitochondrial quality control by Myo-inositol oxygenase in diabetic kidney disease. J Am Soc Nephrol. (2015) 26:1304–21. doi: 10.1681/ASN.2014050457
38. Xiao LI, Xu X, Zhang F, Wang M, Xu Y, Tang D, et al. The mitochondria-targeted antioxidant MitoQ ameliorated tubular injury mediated by mitophagy in diabetic kidney disease via Nrf2/PINK1. Redox Biol. (2017) 11:297–311. doi: 10.1016/j.redox.2016.12.022
Keywords: HIF-1α, mitophagy, diabetic nephropathy, inflammation, ROS, apoptosis
Citation: Yu L, Wang Y, Guo YH, Wang L, Yang Z, Zhai ZH and Tang L (2022) HIF-1α Alleviates High-Glucose-Induced Renal Tubular Cell Injury by Promoting Parkin/PINK1-Mediated Mitophagy. Front. Med. 8:803874. doi: 10.3389/fmed.2021.803874
Received: 28 October 2021; Accepted: 14 December 2021;
Published: 03 February 2022.
Edited by:
Haiyan Fu, Southern Medical University, ChinaReviewed by:
Xiaojie Wang, Shandong University, ChinaCopyright © 2022 Yu, Wang, Guo, Wang, Yang, Zhai and Tang. This is an open-access article distributed under the terms of the Creative Commons Attribution License (CC BY). The use, distribution or reproduction in other forums is permitted, provided the original author(s) and the copyright owner(s) are credited and that the original publication in this journal is cited, in accordance with accepted academic practice. No use, distribution or reproduction is permitted which does not comply with these terms.
*Correspondence: Lin Tang, dGFuZ2xpbkB6enUuZWR1LmNu
Disclaimer: All claims expressed in this article are solely those of the authors and do not necessarily represent those of their affiliated organizations, or those of the publisher, the editors and the reviewers. Any product that may be evaluated in this article or claim that may be made by its manufacturer is not guaranteed or endorsed by the publisher.
Research integrity at Frontiers
Learn more about the work of our research integrity team to safeguard the quality of each article we publish.