- 1Center for Evidence-Based and Translational Medicine, Zhongnan Hospital of Wuhan University, Wuhan, China
- 2Department of Urology, Zhongnan Hospital of Wuhan University, Wuhan, China
Extracellular vesicles (EVs) are natural nanoparticles secreted by cells in the body and released into the extracellular environment. They are associated with various physiological or pathological processes, and considered as carriers in intercellular information transmission, so that EVs can be used as an important marker of liquid biopsy for disease diagnosis and prognosis. EVs are widely present in various body fluids, among which, urine is easy to obtain in large amount through non-invasive methods and has a small dynamic range of proteins, so it is a good object for studying EVs. However, most of the current isolation and detection of EVs still use traditional methods, which are of low purity, time consuming, and poor efficiency; therefore, more efficient and highly selective techniques are urgently needed. Recently, inspired by the nanoscale of EVs, platforms based on nanomaterials have been innovatively explored for isolation and detection of EVs from body fluids. These newly developed nanotechnologies, with higher selectivity and sensitivity, greatly improve the precision of isolation target EVs from urine. This review focuses on the nanomaterials used in isolation and detection of urinary EVs, discusses the advantages and disadvantages between traditional methods and nanomaterials-based platforms, and presents urinary EV-derived biomarkers for prostate cancer (PCa) diagnosis. We aim to provide a reference for researchers who want to carry out studies about nanomaterial-based platforms to identify urinary EVs, and we hope to summarize the biomarkers in downstream analysis of urinary EVs for auxiliary diagnosis of PCa disease in detail.
Introduction
Extracellular vesicles (EVs) are natural nanoparticles with phospholipid bilayer structures that are secreted by cells into the extracellular environment. According to the different formation mechanisms and physiological characteristics, EVs can be divided into three categories: exosomes (30–150 nm), microvesicles (100–1,000 nm), and apoptotic bodies (1,000–5,000 nm) (1, 2). EVs exist in a variety of body fluids, including blood (3), urine (4), pleural fluid (5), breast milk (6), ascites (7), cerebrospinal fluid (8), bronchoalveolar lavage fluid (9), semen (10), and so on. In many of these body fluids, EVs show significant abnormalities under the condition of disease. Since the contents carried by EVs, such as nucleic acids, proteins, and lipids, reflect messages about parental cells and play an important role in the process of antigen delivery, protein and RNA transport, angiogenesis, tumor cell genesis and development, and so on (11–14), EVs are expected to be a new diagnostic biomarker in clinic.
The ideal biomarker should have high reproducibility, stability, sensitivity, specificity, positive and negative predictive values and can be obtained in a non-invasive manner (15). In recent years, urine has been increasingly utilized in the development of biomarkers associated with cancers, because it is readily available in large quantities by non-invasive means and its protein content is much lower than that of blood, which is more conducive to the detection of low-abundance proteins (16). The prostate is close to the urethra in anatomy, so changes in urine composition can indirectly reflect functional changes of prostate. For example, the concentration of EVs in urine will be increased while the person suffers from prostate cancer (PCa) (17). In addition, EVs can also maintain morphological integrity in urine with different permeability (18). Therefore, the cargoes of urinary EVs have great advantages to be used as PCa markers. In recent years, urinary EVs not only have been widely researched as biomarkers, but also their mobility characteristics make them possible to be explored as therapeutic agents and drug carriers (19–22). In order to promote the application of urinary EVs in the fields of disease diagnosis and treatment, obtaining high-yield, high-purity, biologically active, and structurally complete EVs is an important basis for subsequent analysis.
At present, many techniques have been developed for urinary EVs enrichment, such as high-throughput bulk methods, including ultracentrifugation (UC), density gradient centrifugation (DG), ultrafiltration (UF), coprecipitation, size-exclusion chromatography (SEC), and so on. And lots of new enrichment methods are innovatively proposed, like microfluidic filtering, contact-free sorting, immunoaffinity (IAF) enrichment, and so on (23). For EVs characterization, most technologies are utilized to detect the physical properties of EVs, such as scanning electron microscopy (SEM), transmission electron microscopy (TEM), dynamic light scattering (DLS), nanoparticle tracking analysis (NTA), resistive pulse sensing (RPS), and flow cytometry (FCM). Although large amount of creative works about urinary EVs have been reported, there is still a long way to go for their clinical applications. Therefore, in order to promote the applications of urinary EVs in clinic, it is necessary to continuously explore highly effective, easily operated, and time-saving methods for obtaining sufficient amount of target EVs from urine.
Since the end of the twentieth century, nanomaterials have cut a striking figure in the biomedical field due to their attractive mechanical, optical, and electromagnetic properties in nanoscale that differ from traditional bulk materials (24). At present, nanomaterials together with mature modification technology have been widely used in medical imaging and disease diagnosis or treatment (25, 26). Nowadays, an increasing number of researchers have used nanomaterials to enrich EVs from body fluids samples with high selectivity and time saving, and many nanotechnologies have been developed to sort and detect EVs with high sensitivity and easy operation. This review focuses on summarizing the nanomaterials for isolation and detection of EVs from urine samples, the whole contents include introducing the attention points of collection, pretreatment, and storage urine samples, presenting platforms based on nanomaterials for isolation and detection of urinary EVs in detail, and listing the potential EV-derived PCa biomarkers.
Collection, Pretreatment, and Storage of Urine Samples
How to collect, pretreat, and store urinary samples is the first and important step to ensure intact EVs without broken, and the quality of urinary samples directly affect the subsequent separation, purification, and detection of EVs. Therefore, the standardization of sample collection, pretreatment, and storage has great significance to improve the comparability of research results and accelerate the clinical application of urinary EVs (27).
Collection of Urine Samples
It is easy to collect a large amount of urine non-invasively, but the composition of urine is very complex and there are intra-individual and inter-individual differences (28). In addition, EVs are sensitive to changes in the biological fluid environment, so a standardized urine collection program is beneficial to maintain the integrity of EVs (29). At present, the types of urine samples commonly used for EVs analysis are morning urine, random urine, and 24 h urine. Zhou et al. measured four exosome-related proteins (TSG101, NHE3, ALIX, and AQP2) in the first morning urine and the second morning urine, respectively. The results showed that the concentrations of exosome-related proteins in these two different urine samples were almost the same, indicating that both the first morning urine and the second morning urine can be used for analysis of urine EVs (30). Random urine is easy to collect, but its composition is easily affected by factors such as diet and renal function. For analysis of EV-related protein, random urine needs to be standardized with indicators such as urine creatinine or urine flow rate (31), which are difficult to ensure consistency. The composition of 24 h urine is relatively stable, but preservatives need to be added, and the requirements of sample collection are complicated, resulting in poor patient compliance.
Studies have shown that performing digital rectal examination (DRE) before collecting urine samples can promote the secretion of EVs into the urethra, and significantly increase the levels of PCa biomarkers, such as prostate-specific antigen (PSA), PCA3, and E-twenty six (ETS)-related gene (ERG) mRNA (32, 33). However, some scholars believed that urinary EVs biomarkers associated with PCa can be identified even without DRE, because DRE complicated the procedure of urine collection and increased discomfort of patients (34, 35). But in terms of the downstream analysis of EVs alone, urine is a dilute solution with relatively low EVs abundance, and it is necessary to increase the EVs concentration to meet the requirements of various subsequent analyses, the method of collecting urine samples, which can increase the secretion of EVs, such as DRE, is surely attractive.
Pretreatment of Urine Samples
After obtaining urine samples, no matter for EVs separation immediately or storage for later studies, the samples should be centrifuged to remove cell debris, and the supernatant could be collected for later use. Uromodulin (UMOD) is the major protein in urine, which will trap EVs with affinity, resulting in a serious decrease in the efficiency and yield of urinary EVs isolation (36). To solve this problem, some researchers suggested to add chemical reagents, such as dithiothreitol (DTT) and 3-[(3-cholamido propyl) dimethyl ammonio]-1-propane sulfonate (CHAPS), to the urine for preventing EVs from combination with UMOD (37, 38). However, this is not absolute, since the addition of DTT to urine only increased the number of EVs, while the content of RNA did not increase significantly (39), so whether to add and which reagents to add should depend on the purpose of downstream analysis of urinary EVs.
Storage of Urine Samples
Currently, the recommended storage temperature for urine samples is −80°C. Zhou et al. compared the effects of urine samples stored at different temperatures (−4°C vs. −20°C vs. −80°C) on the content of EV-associated proteins, and found that urine samples stored at −80°C lost the least EV-associated protein (14%), and extensive vortexing treatment after thawing can achieve 100% recovery (30). It has also been suggested that whether the urine sample was stored at −4 or −80°C has no effect on the recovery rate of EVs when the storage time is <1 week (40). This opinion is consistent with Jacquillet et al., who recommended −4 or −20°C for short-term urine samples storage and −70 or −80°C for long-term urine samples storage (41).
Traditional Techniques for Isolation and Detection of Urinary EVs
Since EVs were confirmed existing in urine in 2004, researchers have carried out a lot of works about urinary EVs (42). In order to promote the possibility of identifying target EVs in urine for cancer diagnosis, treatment, and prognosis, it is necessary to isolate and detect low abundance of EVs in complex urinary environments. In recent years, some conventional methods for EVs isolation and detection have been developed to meet the needs of urinary EVs researches. In the following sections, we will summarize these traditional technologies and their advantages and disadvantages.
Traditional Techniques for Isolation of Urinary EVs
At present, many techniques based on physical or biochemical characteristics of EVs have been developed to separate them from urine; common ones include UC, DG, UF, SEC, IAF, precipitation, and so on. These techniques can be used alone or in a combination of each other to improve the efficiency of separation, but they all have certain limitations. For example, separation methods based on physical characteristics such as UC, DG, SEC, UF, etc. are time-consuming and low in throughput, recovery, and purity (43), while the precipitation method and IAF method are prone to be contaminated by non-EVs related substances (44). The advantages and disadvantages about all of these approaches are shown in Table 1. Up to now, there is still no single optimal urine EVs isolation method, so scientific selection should be designed according to the purpose of downstream analysis.
Traditional Techniques for Detection of Urinary EVs
After the EVs in urine are isolated, the corresponding detection technique needs to be used to evaluate the results of isolation, which is the premise of further downstream analysis of EVs. The ideal EVs detection techniques usually have the following characteristics (57): the range of EVs size that can be detected is 50 nm and above; with a definite limit of detection for characterization of different EVs; with a known sample volume that allows measuring concentration of EVs; with the ability to identify different epitopes on the EV surface. Traditional techniques for measuring the size distribution and quantification of EVs in urine are TEM, DLS, FCM, NTA, RPS, western blotting (WB), enzyme linked immunosorbent assay (ELISA), and so on. Even though all the performances of above detection technologies cannot be integrated in one device, all of them have their own advantages, and we summarized their advantages and disadvantages in Table 2. Some researchers have compared these methods and found that the size and concentration of EVs detected by each method are somewhat different, mainly because the standard of minimum size detected by each method is different (63). Therefore, combining more than two detection methods is recommended in researches.
Nanomaterial-Based Platforms for Isolation of Urinary EVs
A generally accepted fact is that EVs are involved in communication between cells (13), therefore, EVs in urine have great potential as valuable markers for the diagnosis and prognosis of urological cancers. In order to meet the continuous exploration of urinary EVs, simple and efficient isolation methods have become an important basis for medical research on EVs. However, traditional methods are often unable to fully meet the current research needs due to their shortcomings such as low recovery rate, poor specificity, and high dependence on expensive equipment. As a result, in recent years, scholars have introduced nanomaterials into the isolation techniques of urinary EVs to improve the efficient of extraction (64). The most commonly used nanomaterials in these emerging technologies are nanomembrane and nanowires, which are commonly used to create physical barriers based on size separation. Moreover, magnetic nanoparticles, which are often combined with immune affinity for enrichment of protein, can also be used for identifying and separating EVs. In the following sections, the applications of these methods in isolation of urinary EVs are summarized in detail.
Size-Based Isolation Techniques
The application principle of nanomaterials in the size-based EVs isolation method is similar to that of UF, both of which have the possibilities to isolate EVs by separating the nanoparticles through the filter, the particles smaller than pore size of nanomembrane or the spacing between nanowires will be isolated, which is very suitable for the separation of nano-sized EVs from urine (65–72).
Woo et al. designed an integrated centrifugal microfluidic platform, simplifying the isolation steps of EVs from urine (Figure 1A). The main functional components of the platform were two nanomembrane filters used in centrifuge with low g-forces (<500 g), and their pore diameter ratio was 600:20 nm (Filter I: Filter II). Nanofilter I was used to intercept large particles, and then filter out free nucleic acids and proteins through nanofilter II, so that target-sized EVs were enriched on the nanofilter II. The platform completed the entire extraction process within just 30 min with a high recovery of over 95%. In addition, the concentration of mRNA captured by the device was 100 times higher than that of UC, providing a rich material basis for subsequent downstream analysis (65). Double nanomembranes were also used to filter urinary EVs in Liang's research, with the pore size of nanofilms as 300:20 nm. In this platform, two nanomembranes with different pore sizes were used to remove large particles of impurities and soluble proteins, in order to achieve the purpose of purifying EVs from urine (71) (Figure 1B).
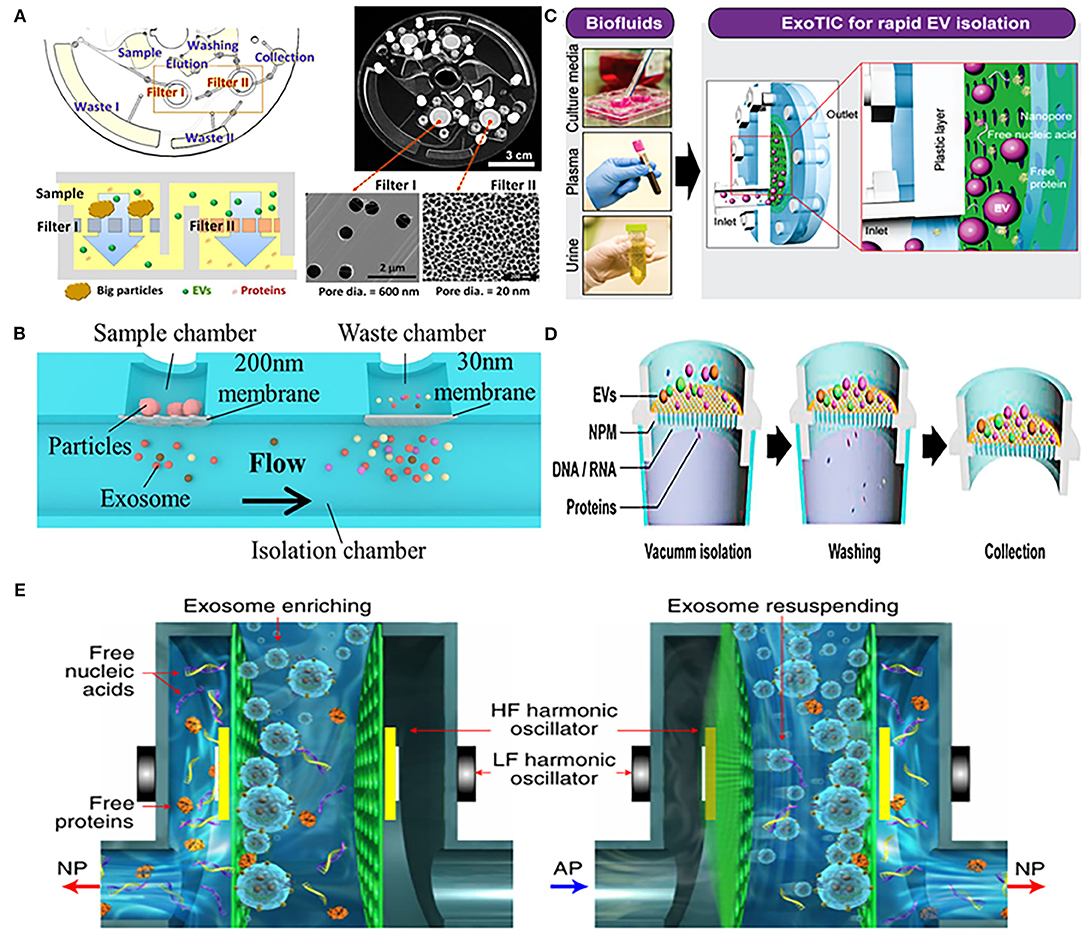
Figure 1. Platforms based on nanomaterials for physical isolation of extracellular vesicles (EVs) from urine. (A) Mechanism of an integrated device (Exodisc) for isolating urinary EVs through two nanofilters with different pore diameters (600:20 nm). Reprinted with permission from Woo et al. (65). (B) Mechanism of urinary exosomes isolation using nanomaterials device combined with double-filtration (200:30 nm). Reprinted with permission from Liang et al. (71). (C) Mechanism of nanomembrane-based modular platform (ExoTIC) for isolating urinary EVs. Reprinted with permission from Liu et al. (66). (D) Mechanism of a nanomaterials device (Exo-POS) combined with a vacuum syringe and nanomembrane for isolating urinary EVs. Reprinted with permission from Deng et al. (67). (E) Mechanism of a nanomaterials device (EXODUC) combined with nanoporous membrane and oscillators for isolating urinary EVs. Reprinted with permission from Chen et al. (68).
Using a similar strategy, Liu et al. proposed a nanomembrane-based high-efficiency modular platform (ExoTIC), which isolated urinary exosomes through washing out free nucleic acids and proteins by the nanomembrane-filter (Figure 1C). This equipment can separate exosomes from urine with high purity and recovery rate, with a throughput of 5 mL/h, and the total operating time is <3 h. In addition, the device can not only enrich the exosomes from urine, but also had the ability of being applied in culture media and plasma, and achieved the yield of exosomes 4–1,000 times higher than that of UC (66). Another high-throughput device was composed of a vacuum syringe and a nanomembrane with a pore size of 20 nm, which further saved the time of separating EVs. Benefited by using vacuum pressure, efficient enrichment of EVs from a 3 mL urine sample within half an hour was achieved (67) (Figure 1D). Different from the aforementioned nanomembrane device, Chen et al. recently reported a new urinary exosomes separation device that combined a nanopore membrane with oscillators (Figure 1E). The device could remove free proteins, nucleic acids, and other small particles by periodic negative pressure oscillations on the nanomembrane. At the same time, the high- and low-frequency harmonic oscillation would be produced by two pairs of oscillators installed on both sides, which can make the particles enriched on the nanofilm resuspended; this method has achieved the purpose of non-blocking in pores of filter and efficient isolation of exosomes. The device can effectively avoid the aggregation of particles and prevents nanoporous membrane from blockage (68).
In addition to nanomembranes, nanowires are also promising filter for EVs isolation. They can not only separate EVs by forming a physical barrier through the tiny gaps, but can also be combined with electrochemistry to enhance the separation performance of the device. Yasui et al. anchored ZnO nanowires on a polydimethylsiloxane (PDMS) substrate to form a highly efficient microfluidic device for urinary EVs separation, which captured EVs of 30–200 nm and enriched them from 1 mL urine taken just 20 min with a recovery of over 99% (Figure 2A). The high-efficiency capture ability of the microfluidic device is not only determined by the size of the particles controlled by the gap between nanowires, but also mainly depends on the nanowire-induced electrostatic. When the urine pH is between 6 and 8, the surface of the ZnO nanowires is positively charged, and the surface of the EVs is negatively charged, so that the nanowires can highly capture EVs in a case of opposites attract (70). This efficient and robust separation technology provides an attractive tool for downstream analysis of EVs.
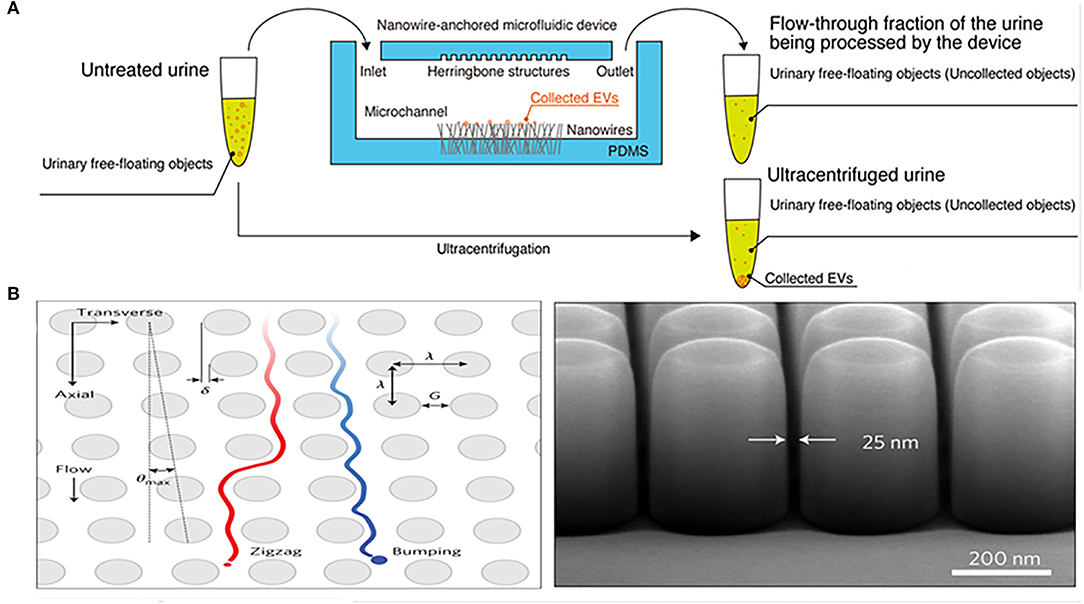
Figure 2. Microfluidic chips based on nanomaterials for isolation of EVs from urine. (A) Mechanism of a microfluidic chip for isolating urinary EVs using electrostatic interactions between the anchored ZnO/Al2O3 core-shell nanowires and EVs. Reprinted with permission from Yasui et al. (70). (B) Mechanism of a microfluidic chip composed of arrays of the deterministic lateral displacement (DLD) pillars for urinary exosomes isolation. Reprinted with permission from Wunsch et al. (72).
Deterministic lateral displacement (DLD) array is an isolation method that combines the laminar flow characteristics of microfluidic channels with the bifurcation of fluid around obstacles. Because it bases on the physical hindrance of obstacles instead of the chemical properties of the analyte for separation, DLD can avoid any protein structure and conformation changes (73). Wunsch et al. designed an optimized nanoscale DLD for the separation of urinary exosomes (Figure 2B). They reduced the minimum gap of the array to 25 nm, allowing for the separation of 20–110 nm particles with clear resolution and the fractionation of polydisperse exosomes particles based on size (72). The device was suitable for trace initial samples, but has a significant disadvantage of low flow rate, which is overcome by another chip integrated with a 1,024 nanoscale DLD arrays (Figure 2C) (69). The throughput of the chip was up to 15 μL/min and the recovery rate of urinary EVs reached 50%. However, such devices often require the application of complex lithography techniques, so it is difficult to apply in different types of samples.
The physical barrier method based on nanomaterials is a promising direction for the development of new EVs isolation techniques. Compared with UC, this type of method greatly shortens the time in separation and improves the recovery efficiency. In addition, according to the inner diameter of the nanoporous membrane, EVs within a set range can be accurately separated, and damage of EVs structure caused by excessive centrifugal gravity can be beneficially avoided, thereby biological composition of EVs could be maintained completely. However, such a physical barrier method also has some limitations, for example, with the accumulation of filtered particles, pore blockage is easy to occur, which affects the durability and filtration efficiency of nanomembrane. Appropriately increasing the effective filtration area of nanomembrane will be a considered method to solve this problem. Second, the size-based isolation methods can easily capture non-desirable particles that have the similar size with EVs, affecting the purity of the extracted EVs. In this case, IAF methods based on specific antibodies or aptamers can be considered for further identification of target EVs.
IAF-Based Isolation Techniques
Different from the size-based theory of the physical barrier method, the IAF isolation methods are based on identifying specific proteins on the surface of EVs, which could isolate specific types of EVs subtypes through strong specific binding with antibodies, and can perfectly exclude cell debris and other proteins that cannot specifically bind to antibodies, overcoming the co-purification problem that exists in traditional methods.
Magnetic nanobeads are often used in enrichment techniques due to their ease of solid-liquid separation and stable magnetic responsiveness (74). Magnetic nanobeads are a kind of popular nanomaterials for separating EVs from urine, the specific antibodies could be stably conjugated on the nanomagnetic beads, and then target the corresponding antigenic epitope on the surface of EVs to capture target EVs. Finally, the magnetic field is widely utilized to separate EVs from other unbound substances in the urine sample (75–78). Three transmembrane proteins, tetraspanin CD9, CD63, and CD81, have been confirmed to be universally expressed in EVs and play important roles in the biogenesis of EVs (79). Therefore, CD9, CD63, and CD81 antibodies are the three most commonly used monoclonal antibodies for the isolation and detection of EVs. Hildonen et al. have confirmed that using these three typical antibodies coupled with nanomagnetic beads to isolate EVs of 30–100 nm from urine had higher purity than UC (75).
Although the combination of magnetic nanobeads and antibodies is widely used in EVs separation, aptamers are also commonly used to combine with magnetic nanobeads. Li et al. combined prostate-specific membrane antigen (PSMA) aptamers with superparamagnetic Fe3O4 nanoparticles, and then modified them with single-stranded DNA to form a superparamagnetic conjunctions complex (Figure 3A). The complex could identify PCa-related exosomes through the specific binding of PSMA aptamers and PSMA-positive exosomes in urine, and then the exosomes would be easily isolated through the restriction sites of the aptamers (76). Another similar aptamer complex (Fe3O4@TiO2-CD63 aptamer) composed of CD63 aptamer and Fe3O4 nanoparticles coated by TiO2 (Figure 3B). The aptamer has dual affinity, including the interaction between TiO2 and the phosphate group of exosomes, and the interaction between CD63 aptamer and the surface protein of exosomes. This dual affinity effects enabled the aptamer to capture urinary exosomes powerfully within just 10 min and the recovery rate is as high as 92.6%, just like the authors' analogy, catching fish with two hands is definitely stronger than catching fish with one hand (77).
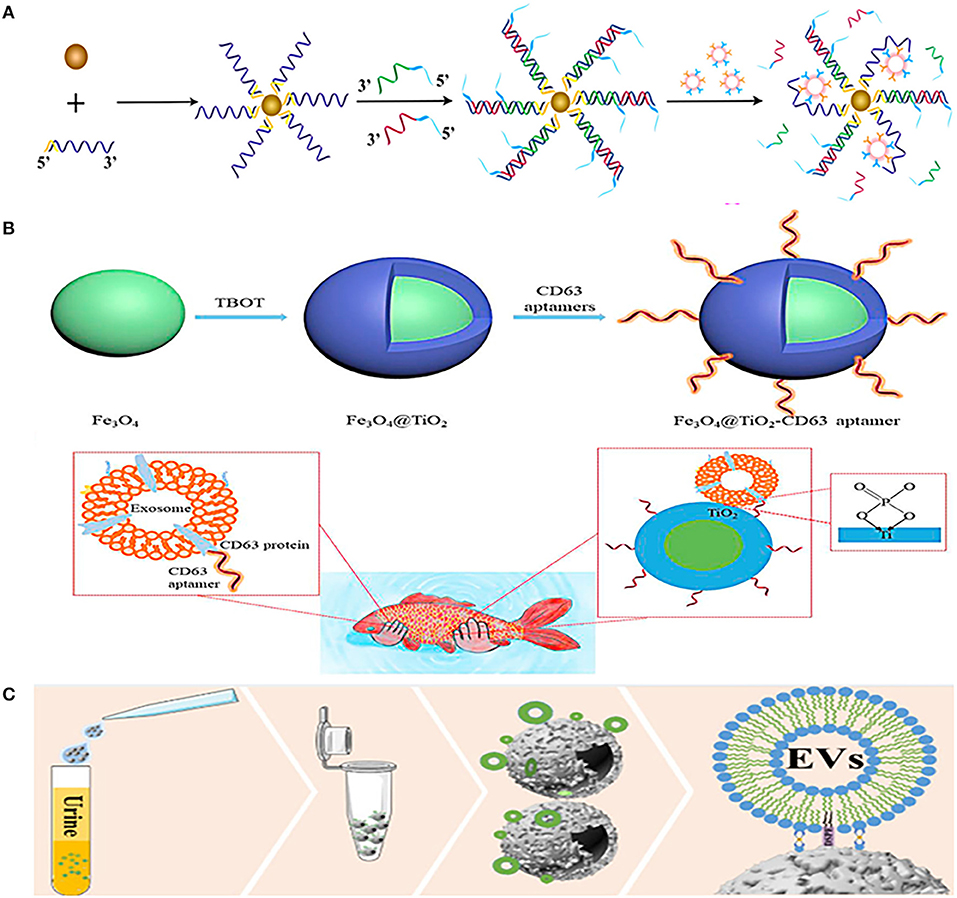
Figure 3. Platforms based on immunoaffinity (IAF) magnetic nanobeads for isolation of urinary EVs. (A) Mechanism of urinary EVs isolation using nanomaterials complexes (superparamagnetic conjunction-molecular beacon [SMC-MB]) combined with prostate-specific membrane antigen (PSMA) aptamers, superparamagnetic Fe3O4 nanoparticles, and single-stranded DNAs. Reprinted with permission from Li et al. (76). (B) Mechanism of urinary EVs isolation using nanomaterials complexes (Fe3O4@TiO2-CD63 aptamer) composed of CD63 aptamer and TiO2 coated on Fe3O4 nanoparticles. Reprinted with permission from Zhang et al. (77). (C) Mechanism of urinary EVs isolation using a dual-function nanomagnetic beads designed with Ti (IV) and 1,2distearoyl-sn-glycero-3-phosphorylethanolamine (DSPE). Reprinted with permission from Sun et al. (78).
Furthermore, a dual-function nanomagnetic beads was designed with Ti (IV) and 1,2distearoyl-sn-glycero-3-phosphorylethanolamine (DSPE) (Figure 3C). Based on the phospholipid bilayer structure of EV membrane, on the one hand, Ti (IV) can bind to the phosphate group in the phospholipid bilayer, and on the other hand, DSPE can be inserted into the phospholipid molecular layer. Thus, the two properties of Ti (IV)-DSPE complex can synergistically capture EVs with a fast (time <1h) and effective (recovery rate >80%) urinary EVs isolation. In addition, quantitative phosphoproteomics analysis of urinary EVs isolated by this method revealed that 121 phosphorylated proteins were upregulated in patients with PCa. These remarkable advantages make it possible for finding new markers in early diagnosis of patients with PCa (78). The same is to use the chelation between the phosphate group of the phospholipid layer on the surface of EVs and Ti (IV), Lou et al. combined it with UF to separate urinary EVs (80). Urine was first concentrated by UF to remove about 25% of urinary protein, and then TiO2-coated magnetic nanobeads were used to capture EVs based on chelation between TiO2 and phosphate groups of phospholipid layer. After EVs were captured, NH3H2O was used to replace phosphate buffer saline (PBS), and the pH value of buffer was adjusted to alkaline to reverse the interaction between TiO2 and phosphate groups to release captured EVs, and finally use magnetic purification of EVs. The yield of the metabolites of EVs obtained by this method is equivalent to that of UC with 467 types of lipid metabolites of urinary EVs have been successfully detected, making it a potential alternative to UC for metabolites analysis of urinary EVs.
The IAF method based on magnetic nanobeads is currently a hot spot in the development of urinary EVs isolation techniques, which can separate high-purity EVs subgroups, but this kind of method also has some limitations, for example, the antibodies used are often expensive, and there is considerable heterogeneity in the expression levels of biomarkers on the surface of EVs between different individuals (79). What's more, currently, there is no systematic and complete classification of EVs (27), so separation based on the characteristics of existing subtypes may miss many undiscovered EVs subtypes. Moreover, the existing markers cannot effectively distinguish different subtypes, such as exosomes and microvesicles. Although it is well known that their mechanisms of occurrence are different, there is still no reliable marker for distinguishing them so far. Therefore, to complete EVs typing system is still an urgent task.
Nanomaterial-Based Platforms for Detection of Urinary EVs
With the continuous development and application of urinary EVs, the traditional EVs detection techniques have been unable to fully meet the existing requirements. In recent years, new detection methods have emerged one after another, among which nanomaterials are frequently applied in various devices to achieve efficient and simple EVs detection (65, 70, 71, 75, 76, 81–83) (Table 3). In the next part, we will summarize the application of these newly techniques in urinary EVs detection.
On-chip ELISA is an easy way to quantify EVs, which has gradually become popular in recent years. An integrated dual-filtration microfluidic system developed by Liang et al. can not only efficiently recover EVs in urine, but also directly quantify EVs by on-chip ELISA (Figure 4A). After the EVs of 20–300 nm were enriched on the nanomembrane, the biotinylated anti-CD63 was used to identify the specific protein on the surface of EVs, and then it was labeled with streptavidin-horseradish peroxidase (HRP), and the final results could be simply transmitted to the computer for data analysis through mobile phone imaging (71). A similar dual-function microfluidic device with two different nanofilters also has been developed to perform ELISA directly on-disc after enriching EVs from urine, it can complete protein detection within just 30 min with a throughput of 16.7 μL/min (Figure 4B) (65). Compared with traditional ELISA, on-chip ELISA has the advantages of high throughput, simple operation, low cost, and high sensitivity, which provides potential for its clinical application.
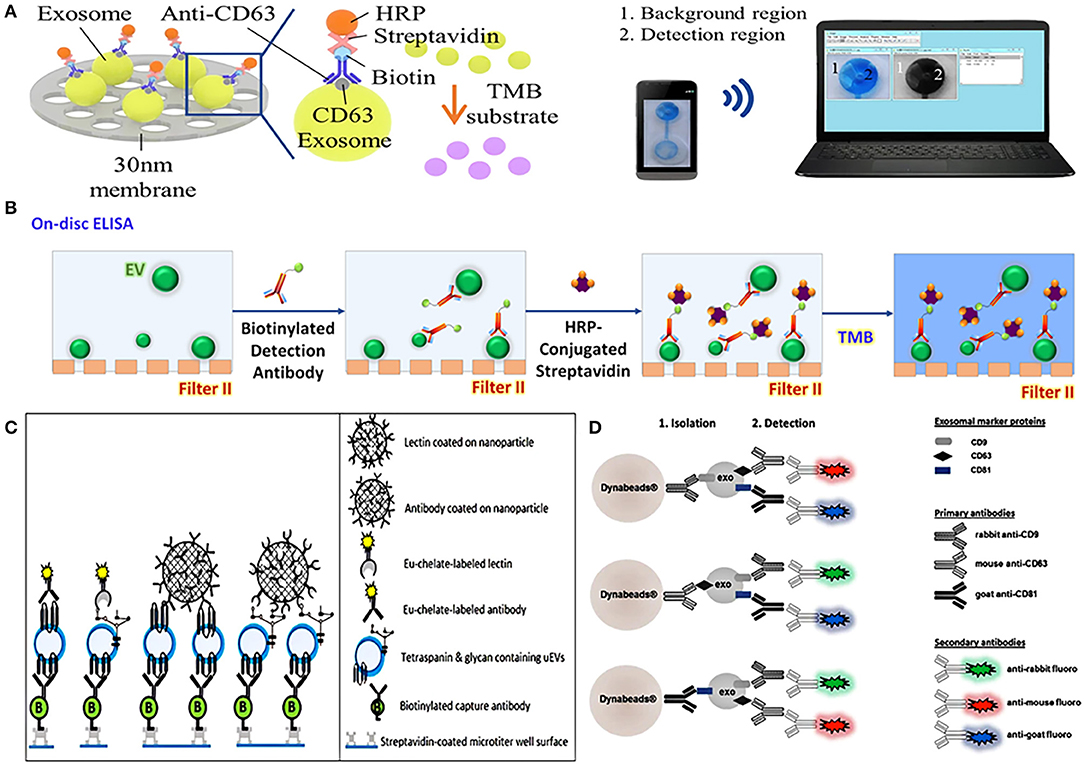
Figure 4. Platforms based on nanomaterials for urinary EVs direct detection. (A) Schematic representation of urinary exosomes detection using on-chip ELISA with biotinylated anti-CD63 and streptavidin-HRP. Reprinted with permission from Liang et al. (71). (B) Schematic of a dual-function nanomaterials device (Exodisc) to detect urinary EVs through on-chip ELISA with biotinylated anti-CD9 and streptavidin-HRP. Reprinted with permission from Woo et al. (65). (C) Schematic of nanoparticles-based device combined Eu3+-doped nanoparticles with time-resolved fluorescence immunoassay (TRFIA) for detecting urinary EVs. Reprinted with permission from Islam et al. (83). (D) Schematic representation of nanoparticles complexes combined nanomagnetic beads, three specific antibodies of rabbit-anti CD9, mouse-anti CD63, and goat-anti CD81, and the corresponding secondary antibodies for detecting urinary EVs. Reprinted with permission from Hildonen et al. (75).
It is a common EVs detection method to combine nanomaterials with visual signals such as fluorescence reaction to achieve quantitative characterization. Islam et al. combined Eu3+-doped nanoparticles with time-resolved fluorescence immunoassay (NP-TRFIA) to develop a stable, simple, and highly sensitive urinary EVs detection method, which can detect specific proteins and polysaccharides on the surface of EVs through antibodies and lectins that were labeled by Eu3+-chelate (Figure 4C). In addition, the device can also identify the differential expression of PCa-related proteins on the surface of EVs, showing its great potential in PCa diagnosis (83). Hildonen et al. utilized magnetic nanobeads to couple with three specific antibodies of rabbit-anti CD9, mouse-anti CD63, and goat-anti CD81 to identify exosomes, and then used secondary antibodies with different colored fluorescent pigments to specifically match primary antibodies. When the matching was completed, the fluorescent effect on the surface of the nanomagnetic beads was triggered, and exosomes can then be detected by different fluorescence (75) (Figure 4D). A similar detection method, combining nanomagnetic beads-based immunocapture technology with traditional FCM, can directly detect multiple different protein markers on the surface of EVs in urine without extracting EVs, which is more sensitive to individual proteins than WB (82).
Li et al. have developed a device combining superparamagnetic conjunction (SMC) and molecular beacon to integrate the separation and quantification of urinary exosomes together (Figure 5A). First, superparamagnetic Fe3O4 nanoparticles, PSMA adaptor, and single-stranded DNA (ssDNA) were combined to form the SMC complex. After adding the complex to urine, the strong affinity between PCa exosomes and PSMA adaptor will replace the combination between ssDNA and PSMA adaptor, thus ssDNA would be completely released. In this way, the captured exosomes can be indirectly quantified by detecting the amount of ssDNA. The quantification of ssDNA depends on two hairpin DNA probes (HP1 and HP2). When ssDNA (the second half is complementary to HP1) binds to HP1, HP2 will squeeze out ssDNA and bind to HP1 due to the stronger affinity of HP2 and HP1, forming a HP1-HP2 complex to turn on the fluorescent signal for detection. By using this method, PSMA-positive exosomes in urine can be specifically captured and detected, the detection limit of this method is as low as 100 particles/μL, which has a potential application in diagnosis of PCa (76).
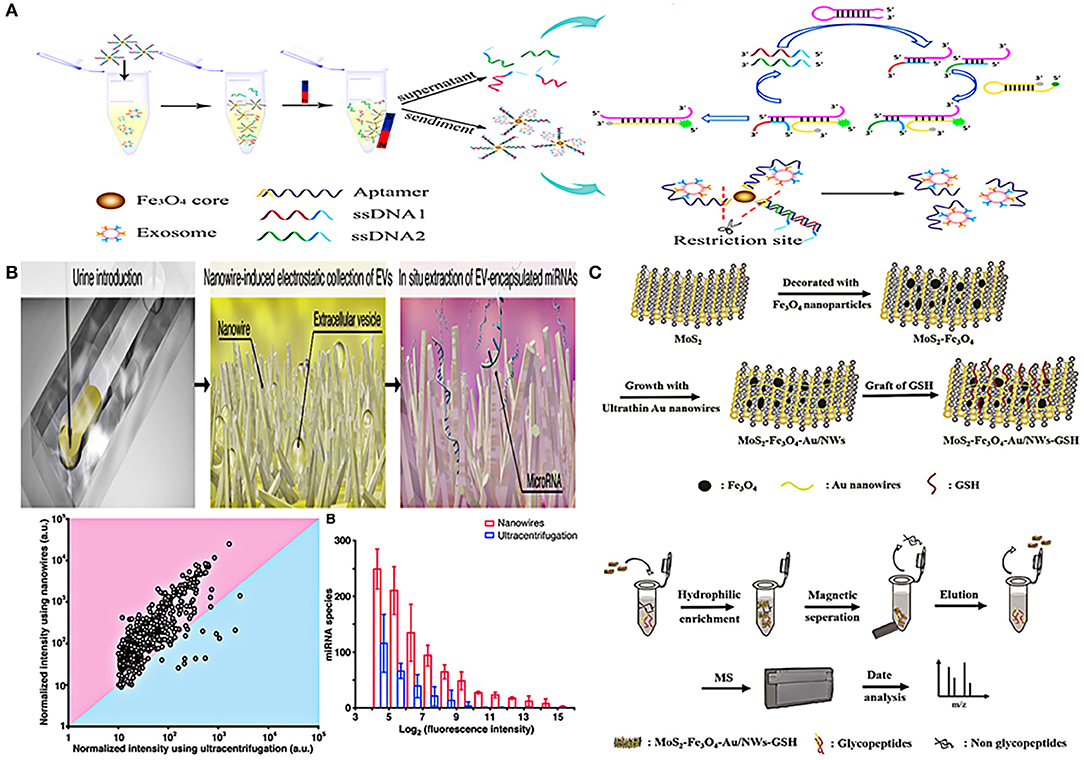
Figure 5. Platforms based on nanomaterials for urinary EVs indirect detection. (A) Schematic representation of urinary EVs indirect detection using nano-complexes (SMC-MB) with molecular beacon. Reprinted with permission from Li et al. (76). (B) Schematic of a microfluidic device integrated with nanowires in the microchannels performed in situ miRNA extraction. Reprinted with permission from Yasui et al. (70). (C) Schematic representation of composite material combined MoS2, superparamagnetic Fe3O4 nanoparticles, ultra-thin Au nanowires, and glutathione for enriching and detecting N-glycopeptides of urinary exosomes. Reprinted with permission from Zhang et al. (81).
In addition to magnetic nanobeads, nanowires are also skillfully designed for urinary EVs detection. Yasui et al. demonstrated a microfluidic device integrated with nanowires in the microchannels for separation of urinary EVs and their miRNA identification (Figure 5B). The device can perform in situ miRNA extraction from the captured EVs, and the entire process (EVs enrichment and miRNA extraction from 1 mL urine) can be completed in just 40 min. In addition, although the initial sample volume required by this technique was 20 times less than that of UC, more miRNA species with different sequences can be extracted than UC method. This approach provided a potential tool for researchers to identify urine miRNA markers and made the early diagnosis of urological cancer possible (70). A magnetic hydrophilic material, which combined MoS2, superparamagnetic Fe3O4 nanoparticles, ultra-thin Au nanowires, and glutathione, was developed to enrich the N-glycopeptides of urinary exosomes based on hydrophilic interaction chromatography (HILIC). After enrichment, non-glycopeptides were removed by magnetic separation, then the obtained N-glycopeptides were deglycosylated, and finally purified exosome N-glycopeptides was collected after two elutions. This complex material can be combined with biomass general analysis to achieve high sensitivity and selectivity of enrichment and detection (81) (Figure 5C).
Even though nanomaterials are used more and more widely in urinary EVs detection, which provides some innovative ways for simplifying steps, reducing costs, and improving efficiency for EVs detection, there are some certain limitations. For example, calculating the purity of extracted EVs by detecting their surface biomarkers is not completely reliable, because some commonly used surface biomarkers of EVs are also present in large quantities of cells (84), which will be simultaneously detected, leading to the overestimation of EVs purity. So, simultaneous detection of multiple EVs markers and setting negative controls of non-EV proteins would be a considerable method to efficiently improve the accuracy of EVs detection. In addition, the IAF method based on magnetic nanobeads is usually used together with fluorescent labels, especially when using multiple fluorescent labels, the displayed fluorescence may overlap, which increases the difficulty to position and distinguish target particles accurately (85). While using quantum dots may solve this problem, quantum dots are a kind of fluorescent nanomaterials and more stable than organic fluorescent materials (86), which may have wide development and application in the field of EVs detection in the future.
Urinary EVs as Potential Biomarkers for PCa
In 2020, PCa has the highest cancer-related incidence in countries with a low human development index and is the fifth leading cause of cancer death in men, which has brought great problems to men's life and health (87). The etiologies of PCa are numerous and complex, which make early accurate diagnosis difficult (88, 89). As we all know, PSA is a common indicator used in PCa detection, and population-based PSA screening for patients with PCa can reduce the mortality to a certain extent. However, the European Society for Medical Oncology (ESMO) still does not recommend PSA detection due to its lack of specificity. For example, benign prostatic hyperplasia (BPH) can also lead to elevated PSA levels, which may lead to overdiagnosis and overtreatment (88). On the other hand, PCa is multifocal, so the biopsy results may lack representativeness, and false negative may occur due to the biopsy missing cancer foci (90). Therefore, there is an urgent need to find new and more effective biomarkers for PCa screening, diagnosis, and follow-up.
Urinary EVs have a similar cargo to their donor cancer cells and can reflect pathophysiological processes within the tissue of origin, allowing them to be potential as a marker for early diagnosis of urological cancer (91). In addition, compared with circulating tumor cells, the abundance of EVs is higher, and EVs can maintain good stability due to the protection of the lipid bilayer, therefore, urinary EVs are potential biomarkers for the diagnosis and prognosis of urological cancer (92). Some researchers agree that EVs in urine may be derived from prostate cells, because PSA, PSMA, and transglutaminase-4 (TGM4), which were prostate-specific molecules, have been found in urinary EVs (93, 94). Besides, PCa-derived EVs in urine have been proved to be a role in the progression of PCa and can be used to monitor the disease (95). Either proteins and nucleic acids or lipids and metabolites of urinary EVs can all be used as markers of PCa to distinguish between normal and disease state (94, 96–98). Next, we will mainly give an overview of proteins, miRNA, and mRNA of urinary EVs, which have the potential to be developed as PCa diagnostic and prognostic biomarkers (Table 4).
Protein Biomarkers in Urinary EVs Related to PCa
Mass spectrometry (MS) is one of the common methods for protein analysis. Øverbye et al. demonstrated the advantages of multiplexing biomarkers by analyzing the proteome of urinary EVs based on MS technology. Their results showed that the area under the curve (AUC) was 0.87 when TM256 was used alone as a marker, while TM256 combining with LAMTOR1, the AUG was increased to 0.94 (94). Wang et al. combined liquid chromatography (LC)-MS/MS with floatation-based density gradient to analyze the protein in urinary EVs of patients with PCa before and after local treatment, and they found that 13 of the 3,686 EVs proteins were significantly reduced after local treatment of PCa. In addition, this study also indicated that protein in urinary EVs could reflect prostate tissue-derived protein, which would provide a certain support for using urinary EVs as a biomarker of PCa (103). Another study also evaluated the ability of urinary EVs protein markers for PCa diagnosis by using WB and ELISA. Flotillin 2 showed a strong discrimination when WB was used for protein analysis, with an AUC of 0.914. While ELISA results showed that the discriminating ability of flotillin 2 decreased (AUC = 0.65), but it showed good sensitivity (68%) and specificity (93%) when combined with PARK7 for PCa diagnosis. This study suggested that urinary EVs protein markers based on immunological analysis also have good PCa diagnostic value, making them easier to be applied in clinical practice (102).
It has been observed that FABP5 was significantly overexpressed in patients with PCa (P = 0.009), and it was significantly associated with high Gleason score GS (P = 0.011), and its ability to predict patients with PCa with GS≥6 and GS≥7 was higher than serum PSA (100). Another study found that the appropriate protein combination panel (PPAP+PSA+CD63+SPHM+GLPK5) of urinary EVs also could be used for differentiating high- and low-grade PCa, which could distinguish PCa with GS ≤ 7 (3 + 4) and GS≥7 (4 + 3) well (AUC = 0.70). Moreover, the study also showed that the ADSV-TGM4 protein combination can identify benign and malignant prostate tumors (101). Welton et al. analyzed the proteomics of urinary EVs in patients with metastatic PCa and found that FGF19, IGFBP2, IGFBP5, CCL16, CD226 antigens, and so on were significantly elevated in the progression disease, which had the potential to suggest an ineffective treatment (99).
MiRNA Biomarkers in Urinary EVs Related to PCa
Many of the urinary EVs markers (miR-19b, miR-196a-5p, miR-501-3p, miR-21, miR-375) can be used to distinguish patients with PCa from healthy men, which were found after miRNA analysis of urinary EVs isolated by UC, but some studies have proved that EVs isolated based on hydrostatic filtration dialysis (HFD) (109), lectin-induced sedimentation (105), and Vn96 (111) can also be used for miRNA analysis. Some potential EVs markers (miR-145, miR-141-5p, miR-21-5p, miR-574-3p, miR-375-3p) have been found for PCa screening and diagnosis. What's more, the miRNA analysis approach is as important as the isolation method. Kim et al. used hydrogel-based hybridization chain reaction (HCR), which has the function of multiplex signal amplification, to perform a ratiometric analysis of miRNA in urinary EVs, and found that the ratio of mir-6090 to mir-3665 was statistically different between patients with PCa and healthy men (P < 0.0001), which provided a supplementary diagnostic marker for PCa (112). In another study, miRCURY LNA miRNA quantitative PCR (qPCR) panel was used to analyze miRNA expression in urinary EVs of healthy controls, BPH, and patients with PCa, and 5 miRNA pairs (miR-30a: miR-125b; miR-425: miR331; miR-29b: miR-21; miR-191: miR-200a; miR-331: miR-106b) were found to identify PCa with 100% specificity and 97.5% accuracy (110).
Not only mature miRNAs, but also the isoforms of miRNA have the potential to diagnose PCa. Koppers-Lalic et al. found that the three miRNA isoforms of miR-21, miR-204, and miR-375 were highly different expressed in healthy controls and patients with PCa, which had a better diagnostic performance than PSA (AUC: 0.866 vs. 0.707) (104). Except for miRNAs of urinary EVs that have the ability to distinguish healthy controls and patients with PCa, miR-2909 can be used to distinguish bladder cancer from PCa and can also be used as a non-invasive marker for differentiating the severity of PCa (108). In addition, Shin et al. constructed a “Prostate Cancer Metastasis Risk Scoring (PCA-MRS)” model, which consists of three miRNAs (miR-21, miR-451, and miR-636) and preoperative PSA. The model, with an AUC of 0.925, had better distinguishing ability than GS, and could effectively predict the biochemical recurrence free survival of patients with PCa based on the score of the model. It has been proved that unique miRNAs of urinary EVs can also be valuable markers for predicting metastasis and prognosis in patients with PCa (114).
MRNA Biomarkers in Urinary EVs Related to PCa
Due to the protection of the phospholipid bilayer, the mRNA of urinary EVs can be stored stably without being hydrolyzed by a large amount of RNA hydrolase in the urine, making the mRNA of EVs to have the potential to become a marker (15). Royo et al. found that while comparing with patients with BPH, the abundance of CDH3 in the urinary EVs of patients with PCa decreased significantly, and this trend was consistent with the change trend of mRNA in PCa cells (116). Similarly, for patients with PCa after prostatectomy, the GATA2 and TMPRSS2: ERG expression levels of urinary EVs also showed a significant downward trend or even disappeared, which were related to the expression levels of GATA2 and TMPRSS2: ERG in prostate tissue. In addition, when these two mRNAs were used in combination with PCA3, the ability to recognize aggressive PCa can be improved and 91.2% of unnecessary biopsies can be avoided, which helps to reduce the over-diagnosis of patients with PCa (35, 117). Moreover, several combination panels, such as the PCA3 and PCGEM1 gene panels and the combination of ERG, PCA3, SPDEF genes, and standard of care (SOC), were also capable of identifying high-grade PCa, and all of them have better diagnostic performance than using SOC alone (34, 115, 118).
Conclusions
Urinary EVs are a promising source of biomarkers with non-invasive and readily available. In order to meet the in-depth exploration of urinary EVs, nanomaterials have been introduced into the isolation and detection techniques to improve efficiency, which provides an important technical premise for the scientific research and clinical application of urinary EVs. EVs capture and detection techniques based on nanomaterials have higher sensitivity and specificity than traditional technologies, and can greatly save the samples and operation time. Moreover, the recovery rate and purity of EVs can also be highly improved. Especially, based on the rapid development of nanotechnologies in recent years, isolation and detection technologies could be highly integrated to specifically recognize EVs derived from a specific tumor cell, like PCa cells, in urine. These methods facilitate the molecular understanding of disease-specific urinary EVs and complete non-invasive biomarkers for early diagnosis and disease surveillance of cancer. In order to further promote the development of clinical assays of urinary EVs and transform experimental research to clinical applications, it is necessary to verify the performance of nanomaterials within larger sample size and more cancer types. Although the isolation and detection techniques of urinary EVs based on nanomaterials are not yet mature, their unique performance helps to solve the shortcomings of traditional methods and brings breakthroughs in the field of clinical science. In addition, urinary EV-related cargoes have shown great potential in diagnosis, disease detection, and prognosis of patients with PCa, but the complex processing of biomarkers identification has limited their clinical applications. In the future, more efforts should be paid to develop urinary EVs diagnostic platform with good biocompatibility, high stability, and reproducibility for clinical applications.
Author Contributions
NW, XH, and Y-SZ summarized all contents and wrote the manuscript. SY and CF reviewed and revised the manuscript. L-LZ and X-TZ conceived the contents, supervised, reviewed, and revised the manuscript, and provided funding acquisition. All authors contributed to the article and approved the submitted version.
Funding
This work was supported by the Health Commission of Hubei Province scientific research project (WJ2021Q041), and the Program of Excellent Doctoral (Postdoctoral) of Zhongnan Hospital of Wuhan University (Grant No. ZNYB2020026).
Conflict of Interest
The authors declare that the research was conducted in the absence of any commercial or financial relationships that could be construed as a potential conflict of interest.
Publisher's Note
All claims expressed in this article are solely those of the authors and do not necessarily represent those of their affiliated organizations, or those of the publisher, the editors and the reviewers. Any product that may be evaluated in this article, or claim that may be made by its manufacturer, is not guaranteed or endorsed by the publisher.
Acknowledgments
We greatly thank the authors whose works were included in this study.
References
1. Van Niel G, D'Angelo G, Raposo G. Shedding light on the cell biology of extracellular vesicles. Nat Rev Mol Cell Biol. (2018) 19:213–28. doi: 10.1038/nrm.2017.125
2. Hu T, Wolfram J, Srivastava S. Extracellular Vesicles in Cancer Detection: Hopes and Hypes. Trends Cancer. (2021) 7:122–33. doi: 10.1016/j.trecan.2020.09.003
3. Kuo WP, Tigges JC, Toxavidis V, Ghiran I. Red Blood Cells: A Source of Extracellular Vesicles. Meth Mol Biol. (2017) 1660:15–22. doi: 10.1007/978-1-4939-7253-1_2
4. Urabe F, Kimura T, Ito K, Yamamoto Y, Tsuzuki S, Miki J, et al. Urinary extracellular vesicles: a rising star in bladder cancer management. Transl Androl Urol. (2021) 10:1878–89. doi: 10.21037/tau-20-1039
5. Kim Y, Shin S, Lee KA. Exosome-based detection of EGFR T790M in plasma and pleural fluid of prospectively enrolled non-small cell lung cancer patients after first-line tyrosine kinase inhibitor therapy. Cancer Cell Int. (2021) 21:50. doi: 10.1186/s12935-021-01761-x
6. Miyake H, Lee C, Chusilp S, Bhalla M, Li B, Pitino M, et al. Human breast milk exosomes attenuate intestinal damage. Pediatr Surg Int. (2020) 36:155–63. doi: 10.1007/s00383-019-04599-7
7. Hu Y, Qi C, Liu X, Zhang C, Gao J, Wu Y, et al. Malignant ascites-derived exosomes promote peritoneal tumor cell dissemination and reveal a distinct miRNA signature in advanced gastric cancer. Cancer Lett. (2019) 457:142–50. doi: 10.1016/j.canlet.2019.04.034
8. Muraoka S, Jedrychowski MP, Yanamandra K, Ikezu S, Gygi SP, Ikezu T. Proteomic profiling of extracellular vesicles derived from cerebrospinal fluid of Alzheimer's disease patients: a pilot study. Cells. (2020) 9:1959. doi: 10.3390/cells9091959
9. Carnino JM, Lee H, Jin Y. Isolation and characterization of extracellular vesicles from Broncho-alveolar lavage fluid: a review and comparison of different methods. Respir Res. (2019) 20:240. doi: 10.1186/s12931-019-1210-z
10. Welch JL, Kaddour H, Winchester L, Fletcher CV, Stapleton JT, Okeoma CM. Semen extracellular vesicles from HIV-1-infected individuals inhibit HIV-1 replication In Vitro, and extracellular vesicles carry antiretroviral drugs In Vivo. J Acquir Immune Defic syndr (1999). (2020) 83:90–8. doi: 10.1097/QAI.0000000000002233
11. Jabbari N, Nawaz M, Rezaie J. Bystander effects of ionizing radiation: conditioned media from X-ray irradiated MCF-7 cells increases the angiogenic ability of endothelial cells. Cell Commun Signal. (2019) 17:165. doi: 10.1186/s12964-019-0474-8
12. Raposo G, Stoorvogel W. Extracellular vesicles: exosomes, microvesicles, and friends. J Cell Biol. (2013) 200:373–83. doi: 10.1083/jcb.201211138
13. Wortzel I, Dror S, Kenific CM, Lyden D. Exosome-mediated metastasis: communication from a distance. Dev Cell. (2019) 49:347–60. doi: 10.1016/j.devcel.2019.04.011
14. Doyle LM, Wang MZ. Overview of extracellular vesicles, their origin, composition, purpose, and methods for exosome isolation and analysis. Cells. (2019) 8:727. doi: 10.3390/cells8070727
15. Dhondt B, Van Deun J, Vermaerke S, de Marco A, Lumen N, De Wever O, et al. Urinary extracellular vesicle biomarkers in urological cancers: From discovery towards clinical implementation. Int J Biochem Cell Biol. (2018) 99:236–56. doi: 10.1016/j.biocel.2018.04.009
16. Filella X, Foj L. Prostate cancer detection and prognosis: from prostate specific antigen (PSA) to exosomal biomarkers. Int J Mol Sci. (2016) 17:1784. doi: 10.3390/ijms17111784
17. Bryzgunova OE, Zaripov MM, Skvortsova TE, Lekchnov EA. Grigor'eva AE, Zaporozhchenko IA, et al. Comparative Study of Extracellular Vesicles from the Urine of Healthy Individuals and Prostate Cancer Patients. PloS ONE. (2016) 11:e0157566. doi: 10.1371/journal.pone.0157566
18. Mitchell PJ, Welton J, Staffurth J, Court J, Mason MD, Tabi Z, et al. Can urinary exosomes act as treatment response markers in prostate cancer? J Transl Med. (2009) 7:4. doi: 10.1186/1479-5876-7-4
19. Li X, Corbett AL, Taatizadeh E, Tasnim N, Little JP, Garnis C, et al. Challenges and opportunities in exosome research-Perspectives from biology, engineering, and cancer therapy. APL Bioeng. (2019) 3:011503. doi: 10.1063/1.5087122
20. Babaei M, Rezaie J. Application of stem cell-derived exosomes in ischemic diseases: opportunity and limitations. J Transl Med. (2021) 19:196. doi: 10.1186/s12967-021-02863-w
21. Nikfarjam S, Rezaie J, Kashanchi F, Jafari R. Dexosomes as a cell-free vaccine for cancer immunotherapy. J Exp Clin Cancer Res. (2020) 39:258. doi: 10.1186/s13046-020-01781-x
22. Rezaie J, Aslan C, Ahmadi M, Zolbanin NM, Kashanchi F, Jafari R. The versatile role of exosomes in human retroviral infections: from immunopathogenesis to clinical application. Cell Biosci. (2021) 11:19. doi: 10.1186/s13578-021-00537-0
23. Shao H, Im H, Castro CM, Breakefield X, Weissleder R, Lee H. New technologies for analysis of extracellular vesicles. Chem Rev. (2018) 118:1917–50. doi: 10.1021/acs.chemrev.7b00534
24. Yang J, Zhang C. Regulation of cancer-immunity cycle and tumor microenvironment by nanobiomaterials to enhance tumor immunotherapy. Wiley Interdiscip Rev Nanomed Nanobiotechnol. (2020) 12:e1612. doi: 10.1002/wnan.1612
25. Abd Elkodous M, El-Sayyad GS, Abdelrahman IY, El-Bastawisy HS, Mohamed AE, Mosallam FM, et al. Therapeutic and diagnostic potential of nanomaterials for enhanced biomedical applications. Colloids Surf B, Biointerfaces. (2019) 180:411–28. doi: 10.1016/j.colsurfb.2019.05.008
26. Zhang K, Gao YJ, Yang PP Qi GB, Zhang JP, Wang L, et al. Self-assembled fluorescent organic nanomaterials for biomedical imaging. Adv Healthc Mater. (2018) 7:e1800344. doi: 10.1002/adhm.201800344
27. Théry C, Witwer KW, Aikawa E, Alcaraz MJ, Anderson JD, Andriantsitohaina R, et al. Minimal information for studies of extracellular vesicles 2018 (MISEV2018): a position statement of the International Society for Extracellular Vesicles and update of the MISEV2014 guidelines. J Extracell Vesicles. (2018) 7:1535750. doi: 10.1080/20013078.2018.1535750
28. Street JM, Koritzinsky EH, Glispie DM, Star RA, Yuen PS. Urine exosomes: an emerging trove of biomarkers. Adv Clin Chem. (2017) 78:103–22. doi: 10.1016/bs.acc.2016.07.003
29. Witwer KW, Buzás EI, Bemis LT, Bora A, Lässer C, Lötvall J, et al. Standardization of sample collection, isolation and analysis methods in extracellular vesicle research. J Extracell Vesicles. (2013) 2:20360. doi: 10.3402/jev.v2i0.20360
30. Zhou H, Yuen PS, Pisitkun T, Gonzales PA, Yasuda H, Dear JW, et al. Collection, storage, preservation, and normalization of human urinary exosomes for biomarker discovery. Kidney Int. (2006) 69:1471–6. doi: 10.1038/sj.ki.5000273
31. Pisitkun T, Johnstone R, Knepper MA. Discovery of urinary biomarkers. Mol Cell Proteomics. (2006) 5:1760–71. doi: 10.1074/mcp.R600004-MCP200
32. Hendriks RJ, Dijkstra S, Jannink SA, Steffens MG, van Oort IM, Mulders PF, et al. Comparative analysis of prostate cancer specific biomarkers PCA3 and ERG in whole urine, urinary sediments and exosomes. Clin Chem Lab Med. (2016) 54:483–92. doi: 10.1515/cclm-2015-0599
33. Dijkstra S, Birker IL, Smit FP, Leyten GH, de Reijke TM, van Oort IM, et al. Prostate cancer biomarker profiles in urinary sediments and exosomes. J Urol. (2014) 191:1132–8. doi: 10.1016/j.juro.2013.11.001
34. Donovan MJ, Noerholm M, Bentink S, Belzer S, Skog J, O'Neill V, et al. A molecular signature of PCA3 and ERG exosomal RNA from non-DRE urine is predictive of initial prostate biopsy result. Prostate Cancer Prostatic Dis. (2015) 18:370–5. doi: 10.1038/pcan.2015.40
35. Motamedinia P, Scott AN, Bate KL, Sadeghi N, Salazar G, Shapiro E, et al. Urine exosomes for non-invasive assessment of gene expression and mutations of prostate cancer. PLoS ONE. (2016) 11:e0154507. doi: 10.1371/journal.pone.0154507
36. Xu X, Barreiro K, Musante L, Kretz O, Lin H, Zou H, et al. Management of Tamm-Horsfall protein for reliable urinary analytics. Proteomics Clin Appl. (2019) 13:e1900018. doi: 10.1002/prca.201900018
37. Fernández-Llama P, Khositseth S, Gonzales PA, Star RA, Pisitkun T, Knepper MA. Tamm-Horsfall protein and urinary exosome isolation. Kidney Int. (2010) 77:736–42. doi: 10.1038/ki.2009.550
38. Musante L, Saraswat M, Duriez E, Byrne B, Ravidà A, Domon B, et al. Biochemical and physical characterisation of urinary nanovesicles following CHAPS treatment. PLoS ONE. (2012) 7:e37279. doi: 10.1371/journal.pone.0037279
39. Cheng L, Sharples RA, Scicluna BJ, Hill AF. Exosomes provide a protective and enriched source of miRNA for biomarker profiling compared to intracellular and cell-free blood. J Extracell Vesicles. (2014) 3:23743. doi: 10.3402/jev.v3.23743
40. Cheruvanky A, Zhou H, Pisitkun T, Kopp JB, Knepper MA, Yuen PS, et al. Rapid isolation of urinary exosomal biomarkers using a nanomembrane ultrafiltration concentrator. Am J Physiol Renal Physiol. (2007) 292:F1657–61. doi: 10.1152/ajprenal.00434.2006
41. Jacquillet G, Hoorn EJ, Vilasi A, Unwin RJ. Urinary vesicles: in splendid isolation. Nephrol Dial Transplant. (2013) 28:1332–5. doi: 10.1093/ndt/gfs599
42. Pisitkun T, Shen RF, Knepper MA. Identification and proteomic profiling of exosomes in human urine. Proc Natl Acad Sci USA. (2004) 101:13368–73. doi: 10.1073/pnas.0403453101
43. Tauro BJ, Greening DW, Mathias RA Ji H, Mathivanan S, Scott AM, et al. Comparison of ultracentrifugation, density gradient separation, and immunoaffinity capture methods for isolating human colon cancer cell line LIM1863-derived exosomes. Methods. (2012) 56:293–304. doi: 10.1016/j.ymeth.2012.01.002
44. Lane RE, Korbie D, Trau M, Hill MM. Purification protocols for extracellular vesicles. Meth Mol Biol. (2017) 1660:111–30. doi: 10.1007/978-1-4939-7253-1_10
45. Lourenço C, Constâncio V, Henrique R, Carvalho Â, Jerónimo C. Urinary extracellular vesicles as potential biomarkers for urologic cancers: an overview of current methods and advances. Cancers. (2021) 13:1529. doi: 10.3390/cancers13071529
46. Yang XX, Sun C, Wang L, Guo XL. New insight into isolation, identification techniques and medical applications of exosomes. J Control Release. (2019) 308:119–29. doi: 10.1016/j.jconrel.2019.07.021
47. Musante L, Saraswat M, Ravidà A, Byrne B, Holthofer H. Recovery of urinary nanovesicles from ultracentrifugation supernatants. Nephrol Dial Transplant. (2013) 28:1425–33. doi: 10.1093/ndt/gfs564
48. Théry C, Amigorena S, Raposo G, Clayton A. Isolation and characterization of exosomes from cell culture supernatants and biological fluids. Curr Protocols Cell Biol. (2006) Chapter 3:Unit 3.22. doi: 10.1002/0471143030.cb0322s30
49. Dong L, Zieren RC, Horie K, Kim CJ, Mallick E, Jing Y, et al. Comprehensive evaluation of methods for small extracellular vesicles separation from human plasma, urine and cell culture medium. J Extracell Vesicles. (2020) 10:e12044. doi: 10.1002/jev2.12044
50. Ramirez MI, Amorim MG, Gadelha C, Milic I, Welsh JA, Freitas VM, et al. Technical challenges of working with extracellular vesicles. Nanoscale. (2018) 10:881–906. doi: 10.1039/C7NR08360B
51. Vergauwen G, Dhondt B, Van Deun J, De Smedt E, Berx G, Timmerman E, et al. Confounding factors of ultrafiltration and protein analysis in extracellular vesicle research. Sci Rep. (2017) 7:2704. doi: 10.1038/s41598-017-02599-y
52. Sidhom K, Obi PO, Saleem A. A review of exosomal isolation methods: is size exclusion chromatography the best option? Int J Mol Sci. (2020) 21:6466. doi: 10.20944/preprints202007.0485.v2
53. Lane RE, Korbie D, Trau M, Hill MM. Optimizing size exclusion chromatography for extracellular vesicle enrichment and proteomic analysis from clinically relevant samples. Proteomics. (2019) 19:e1800156. doi: 10.1002/pmic.201800156
54. Sun Z, Yang J, Li H, Wang C, Fletcher C, Li J, et al. Progress in the research of nanomaterial-based exosome bioanalysis and exosome-based nanomaterials tumor therapy. Biomaterials. (2021) 274:120873. doi: 10.1016/j.biomaterials.2021.120873
55. Boriachek K, Islam MN, Möller A, Salomon C, Nguyen NT, Hossain MSA, et al. Biological functions and current advances in isolation and detection strategies for exosome nanovesicles. Small. (2018) 14:99. doi: 10.1002/smll.201702153
56. Weng Y, Sui Z, Shan Y, Hu Y, Chen Y, Zhang L, et al. Effective isolation of exosomes with polyethylene glycol from cell culture supernatant for in-depth proteome profiling. The Analyst. (2016) 141:4640–6. doi: 10.1039/C6AN00892E
57. Coumans FAW, Brisson AR, Buzas EI, Dignat-George F, Drees EEE, El-Andaloussi S, et al. Methodological Guidelines to Study Extracellular Vesicles. Circ Res. (2017) 120:1632–48. doi: 10.1161/CIRCRESAHA.117.309417
58. Merchant ML, Rood IM, Deegens JKJ, Klein JB. Isolation and characterization of urinary extracellular vesicles: implications for biomarker discovery. Nat Rev Nephrol. (2017) 13:731–49. doi: 10.1038/nrneph.2017.148
59. Ko J, Carpenter E, Issadore D. Detection and isolation of circulating exosomes and microvesicles for cancer monitoring and diagnostics using micro-/nano-based devices. The Analyst. (2016) 141:450–60. doi: 10.1039/C5AN01610J
60. Kowal EJK, Ter-Ovanesyan D, Regev A, Church GM. Extracellular vesicle isolation and analysis by western blotting. Meth Mol Biol. (2017) 1660:143–52. doi: 10.1007/978-1-4939-7253-1_12
61. Wu X, Showiheen SAA, Sun AR, Crawford R, Xiao Y, Mao X, et al. Exosomes extraction and identification. Meth Mol Biol. (2019) 2054:81–91. doi: 10.1007/978-1-4939-9769-5_4
62. Logozzi M, Di Raimo R, Mizzoni D, Fais S. Immunocapture-based ELISA to characterize and quantify exosomes in both cell culture supernatants and body fluids. Meth Enzymol. (2020) 645:155–80. doi: 10.1016/bs.mie.2020.06.011
63. Van der Pol E, Coumans FA, Grootemaat AE, Gardiner C, Sargent IL, Harrison P, et al. Particle size distribution of exosomes and microvesicles determined by transmission electron microscopy, flow cytometry, nanoparticle tracking analysis, and resistive pulse sensing. J Thromb Haemost. (2014) 12:1182–92. doi: 10.1111/jth.12602
64. Shao B, Xiao Z. Recent achievements in exosomal biomarkers detection by nanomaterials-based optical biosensors—a review. Anal Chim Acta. (2020) 1114:74–84. doi: 10.1016/j.aca.2020.02.041
65. Woo HK, Sunkara V, Park J, Kim TH, Han JR, Kim CJ, et al. Exodisc for rapid, size-selective, and efficient isolation and analysis of nanoscale extracellular vesicles from biological samples. ACS Nano. (2017) 11:1360–70. doi: 10.1021/acsnano.6b06131
66. Liu F, Vermesh O, Mani V, Ge TJ, Madsen SJ, Sabour A, et al. The exosome total isolation chip. ACS Nano. (2017) 11:10712–23. doi: 10.1021/acsnano.7b04878
67. Deng Z, Wang Y, Hu L, Zhu Q, Chen Y, Chen JJ, et al. A facile, rapid, high-throughput extracellular vesicles analytical platform for cancer detection. Anal Chim Acta. (2020) 1138:132–40. doi: 10.1016/j.aca.2020.08.053
68. Chen Y, Zhu Q, Cheng L, Wang Y, Li M, Yang Q, et al. Exosome detection via the ultrafast-isolation system: EXODUS. Nat Methods. (2021) 18:212–8. doi: 10.1038/s41592-020-01034-x
69. Smith JT, Wunsch BH, Dogra N, Ahsen ME, Lee K, Yadav KK, et al. Integrated nanoscale deterministic lateral displacement arrays for separation of extracellular vesicles from clinically-relevant volumes of biological samples. Lab Chip. (2018) 18:3913–25. doi: 10.1039/C8LC01017J
70. Yasui T, Yanagida T, Ito S, Konakade Y, Takeshita D, Naganawa T, et al. Unveiling massive numbers of cancer-related urinary-microRNA candidates via nanowires. Sci Adv. (2017) 3:e1701133. doi: 10.1126/sciadv.1701133
71. Liang LG, Kong MQ, Zhou S, Sheng YF, Wang P, Yu T, et al. An integrated double-filtration microfluidic device for isolation, enrichment and quantification of urinary extracellular vesicles for detection of bladder cancer. Sci Rep. (2017) 7:46224. doi: 10.1038/srep46224
72. Wunsch BH, Smith JT, Gifford SM, Wang C, Brink M, Bruce RL, et al. Nanoscale lateral displacement arrays for the separation of exosomes and colloids down to 20 nm. Nat Nanotechnol. (2016) 11:936–40. doi: 10.1038/nnano.2016.134
73. Salafi T, Zhang Y, Zhang Y. A review on deterministic lateral displacement for particle separation and detection. Nano-Micro Lett. (2019) 11:77. doi: 10.1007/s40820-019-0308-7
74. Ríos Á, Zougagh M. Recent advances in magnetic nanomaterials for improving analytical processes. TrAC Trends Anal Chem. (2016) 84:72–83. doi: 10.1016/j.trac.2016.03.001
75. Hildonen S, Skarpen E, Halvorsen TG, Reubsaet L. Isolation and mass spectrometry analysis of urinary extraexosomal proteins. Sci Rep. (2016) 6:36331. doi: 10.1038/srep36331
76. Li P, Yu X, Han W, Kong Y, Bao W, Zhang J, et al. Ultrasensitive and reversible nanoplatform of urinary exosomes for prostate cancer diagnosis. ACS sensors. (2019) 4:1433–41. doi: 10.1021/acssensors.9b00621
77. Zhang N, Sun N, Deng C. Rapid isolation and proteome analysis of urinary exosome based on double interactions of Fe(3)O(4)@TiO(2)-DNA aptamer. Talanta. (2021) 221:121571. doi: 10.1016/j.talanta.2020.121571
78. Sun J, Han S, Ma L, Zhang H, Zhan Z, Aguilar HA, et al. Synergistically bifunctional paramagnetic separation enables efficient isolation of urine extracellular vesicles and downstream phosphoproteomic analysis. ACS Appl Mater Interfaces. (2021) 13:3622–30. doi: 10.1021/acsami.0c19400
79. Jørgensen M, Bæk R, Pedersen S, Søndergaard EK, Kristensen SR, Varming K. Extracellular Vesicle (EV) Array: microarray capturing of exosomes and other extracellular vesicles for multiplexed phenotyping. J Extracell Vesicles. (2013) 2:20920. doi: 10.3402/jev.v2i0.20920
80. Lou D, Wang Y, Yang Q, Hu L, Zhu Q. Ultrafiltration combing with phospholipid affinity-based isolation for metabolomic profiling of urinary extracellular vesicles. J Chromatogr A. (2021) 1640:461942. doi: 10.1016/j.chroma.2021.461942
81. Zhang H, Lv Y, Du J, Shao W, Jiao F, Xia C, et al. A GSH Functionalized magnetic ultra-thin 2D-MoS(2) nanocomposite for HILIC-based enrichment of N-glycopeptides from urine exosome and serum proteins. Anal Chim Acta. (2020) 1098:181–9. doi: 10.1016/j.aca.2019.11.012
82. Campos-Silva C, Suárez H, Jara-Acevedo R, Linares-Espinós E, Martinez-Piñeiro L, Yáñez-Mó M, et al. High sensitivity detection of extracellular vesicles immune-captured from urine by conventional flow cytometry. Sci Rep. (2019) 9:2042. doi: 10.1038/s41598-019-38516-8
83. Islam MK, Syed P, Lehtinen L, Leivo J, Gidwani K, Wittfooth S, et al. A Nanoparticle-based approach for the detection of extracellular vesicles. Sci Rep. (2019) 9:10038. doi: 10.1038/s41598-019-46395-2
84. Yoshioka Y, Konishi Y, Kosaka N, Katsuda T, Kato T, Ochiya T. Comparative marker analysis of extracellular vesicles in different human cancer types. J Extracell Vesicles. (2013) 2:20424. doi: 10.3402/jev.v2i0.20424
85. Singh RD, Shandilya R, Bhargava A, Kumar R, Tiwari R, Chaudhury K, et al. Quantum dot based nano-biosensors for detection of circulating cell free miRNAs in lung carcinogenesis: from biology to clinical translation. Front Genet. (2018) 9:616. doi: 10.3389/fgene.2018.00616
86. Dobhal G, Ayupova D, Laufersky G, Ayed Z, Nann T, Goreham RV. Cadmium-free quantum dots as fluorescent labels for exosomes. Sensors. (2018) 18:3308. doi: 10.3390/s18103308
87. Sung H, Ferlay J, Siegel RL, Laversanne M, Soerjomataram I, Jemal A, et al. Global cancer statistics 2020: GLOBOCAN estimates of incidence and mortality worldwide for 36 cancers in 185 countries. CA Cancer J Clin. (2021) 71:209–49. doi: 10.3322/caac.21660
88. Parker C, Castro E, Fizazi K, Heidenreich A, Ost P, Procopio G, et al. Prostate cancer: ESMO clinical practice guidelines for diagnosis, treatment and follow-up. Ann Oncol. (2020) 31:1119–34. doi: 10.1016/j.annonc.2020.06.011
89. Zi H, He SH, Leng XY, Xu XF, Huang Q, Weng H, et al. Global, regional, and national burden of kidney, bladder, and prostate cancers and their attributable risk factors, 1990–2019. Mil Med Res. (2021) 8:60. doi: 10.1186/s40779-021-00354-z
90. Kasivisvanathan V, Rannikko AS, Borghi M, Panebianco V, Mynderse LA, Vaarala MH, et al. MRI-Targeted or standard biopsy for prostate-cancer diagnosis. N Engl J Med. (2018) 378:1767–77. doi: 10.1056/NEJMoa1801993
91. Soung YH, Ford S, Zhang V, Chung J. Exosomes in cancer diagnostics. Cancers. (2017) 9:8. doi: 10.3390/cancers9010008
92. Stahl PD, Raposo G. Extracellular vesicles: exosomes and microvesicles, integrators of homeostasis. Physiology. (2019) 34:169–77. doi: 10.1152/physiol.00045.2018
93. Drake RR, Kislinger T. The proteomics of prostate cancer exosomes. Expert Rev Proteomics. (2014) 11:167–77. doi: 10.1586/14789450.2014.890894
94. Øverbye A, Skotland T, Koehler CJ, Thiede B, Seierstad T, Berge V, et al. Identification of prostate cancer biomarkers in urinary exosomes. Oncotarget. (2015) 6:30357–76. doi: 10.18632/oncotarget.4851
95. Miyahira AK, Sharp A, Ellis L, Jones J, Kaochar S, Larman HB, et al. Prostate cancer research: the next generation; report from the 2019 coffey-holden prostate cancer academy meeting. Prostate. (2020) 80:113–32.
96. Işin M, Uysaler E, Özgür E, Köseoglu H, Sanli Ö, Yücel Ö B, et al. Exosomal lncRNA-p21 levels may help to distinguish prostate cancer from benign disease. Front Genet. (2015) 6:168. doi: 10.3389/fgene.2015.00168
97. Clos-Garcia M, Loizaga-Iriarte A, Zuñiga-Garcia P, Sánchez-Mosquera P, Rosa Cortazar A, González E, et al. Metabolic alterations in urine extracellular vesicles are associated to prostate cancer pathogenesis and progression. J Extracell Vesicles. (2018) 7:1470442. doi: 10.1080/20013078.2018.1470442
98. Skotland T, Ekroos K, Kauhanen D, Simolin H, Seierstad T, Berge V, et al. Molecular lipid species in urinary exosomes as potential prostate cancer biomarkers. Eur J Cancer. (2017) 70:122–32. doi: 10.1016/j.ejca.2016.10.011
99. Welton JL, Brennan P, Gurney M, Webber JP, Spary LK, Carton DG, et al. Proteomics analysis of vesicles isolated from plasma and urine of prostate cancer patients using a multiplex, aptamer-based protein array. J Extracell Vesicles. (2016) 5:31209. doi: 10.3402/jev.v5.31209
100. Fujita K, Kume H, Matsuzaki K, Kawashima A, Ujike T, Nagahara A, et al. Proteomic analysis of urinary extracellular vesicles from high Gleason score prostate cancer. Sci Rep. (2017) 7:42961. doi: 10.1038/srep42961
101. Sequeiros T, Rigau M, Chiva C, Montes M, Garcia-Grau I, Garcia M, et al. Targeted proteomics in urinary extracellular vesicles identifies biomarkers for diagnosis and prognosis of prostate cancer. Oncotarget. (2017) 8:4960–76. doi: 10.18632/oncotarget.13634
102. Wang L, Skotland T, Berge V, Sandvig K, Llorente A. Exosomal proteins as prostate cancer biomarkers in urine: from mass spectrometry discovery to immunoassay-based validation. Eur J Pharm Sci. (2017) 98:80–5. doi: 10.1016/j.ejps.2016.09.023
103. Dhondt B, Geeurickx E, Tulkens J, Van Deun J, Vergauwen G, Lippens L, et al. Unravelling the proteomic landscape of extracellular vesicles in prostate cancer by density-based fractionation of urine. J Extracell Vesicles. (2020) 9:1736935. doi: 10.1080/20013078.2020.1736935
104. Koppers-Lalic D, Hackenberg M, de Menezes R, Misovic B, Wachalska M, Geldof A, et al. Non-invasive prostate cancer detection by measuring miRNA variants (isomiRs) in urine extracellular vesicles. Oncotarget. (2016) 7:22566–78. doi: 10.18632/oncotarget.8124
105. Samsonov R, Shtam T, Burdakov V, Glotov A, Tsyrlina E, Berstein L, et al. Lectin-induced agglutination method of urinary exosomes isolation followed by mi-RNA analysis: Application for prostate cancer diagnostic. Prostate. (2016) 76:68–79. doi: 10.1002/pros.23101
106. Foj L, Ferrer F, Serra M, Arévalo A, Gavagnach M, Giménez N, et al. Exosomal and non-exosomal urinary miRNAs in prostate cancer detection and prognosis. Prostate. (2017) 77:573–83. doi: 10.1002/pros.23295
107. Rodríguez M, Bajo-Santos C, Hessvik NP, Lorenz S, Fromm B, Berge V, et al. Identification of non-invasive miRNAs biomarkers for prostate cancer by deep sequencing analysis of urinary exosomes. Mol Cancer. (2017) 16:156. doi: 10.1186/s12943-017-0726-4
108. Wani S, Kaul D, Mavuduru RS, Kakkar N, Bhatia A. Urinary-exosomal miR-2909: A novel pathognomonic trait of prostate cancer severity. J Biotechnol. (2017) 259:135–9. doi: 10.1016/j.jbiotec.2017.07.029
109. Xu Y, Qin S, An T, Tang Y, Huang Y, Zheng L. MiR-145 detection in urinary extracellular vesicles increase diagnostic efficiency of prostate cancer based on hydrostatic filtration dialysis method. Prostate. (2017) 77:1167–75. doi: 10.1002/pros.23376
110. Bryzgunova OE, Zaporozhchenko IA, Lekchnov EA, Amelina EV, Konoshenko MY, Yarmoschuk SV, et al. Data analysis algorithm for the development of extracellular miRNA-based diagnostic systems for prostate cancer. PLoS ONE. (2019) 14:e0215003. doi: 10.1371/journal.pone.0215003
111. Davey M, Benzina S, Savoie M, Breault G, Ghosh A, Ouellette RJ. Affinity captured urinary extracellular vesicles provide mRNA and miRNA biomarkers for improved accuracy of prostate cancer detection: a pilot study. Int J Mol Sci. (2020) 21:8330. doi: 10.3390/ijms21218330
112. Kim J, Shim JS, Han BH, Kim HJ, Park J, Cho IJ, et al. Hydrogel-based hybridization chain reaction (HCR) for detection of urinary exosomal miRNAs as a diagnostic tool of prostate cancer. Biosens Bioelectron. (2021) 192:113504. doi: 10.1016/j.bios.2021.113504
113. Matsuzaki K, Fujita K, Tomiyama E, Hatano K, Hayashi Y, Wang C, et al. MiR-30b-3p and miR-126-3p of urinary extracellular vesicles could be new biomarkers for prostate cancer. Transl Androl Urol. (2021) 10:1918–27. doi: 10.21037/tau-20-421
114. Shin S, Park YH, Jung SH, Jang SH, Kim MY, Lee JY, et al. Urinary exosome microRNA signatures as a noninvasive prognostic biomarker for prostate cancer. NPJ Genomic Med. (2021) 6:45. doi: 10.1038/s41525-021-00212-w
115. McKiernan J, Donovan MJ, O'Neill V, Bentink S, Noerholm M, Belzer S, et al. A novel urine exosome gene expression assay to predict high-grade prostate cancer at initial biopsy. JAMA Oncol. (2016) 2:882–9. doi: 10.1001/jamaoncol.2016.0097
116. Royo F, Zuñiga-Garcia P, Torrano V, Loizaga A, Sanchez-Mosquera P, Ugalde-Olano A, et al. Transcriptomic profiling of urine extracellular vesicles reveals alterations of CDH3 in prostate cancer. Oncotarget. (2016) 7:6835–46. doi: 10.18632/oncotarget.6899
117. Woo J, Santasusagna S, Banks J, Pastor-Lopez S, Yadav K, Carceles-Cordon M, et al. Urine extracellular vesicle GATA2 mRNA discriminates biopsy result in men with suspicion of prostate cancer. J Urol. (2020) 204:691–700. doi: 10.1097/JU.0000000000001066
Keywords: urinary extracellular vesicles, nanomaterials, isolation, detection, biomarkers, prostate cancer
Citation: Wang N, Yuan S, Fang C, Hu X, Zhang Y-S, Zhang L-L and Zeng X-T (2022) Nanomaterials-Based Urinary Extracellular Vesicles Isolation and Detection for Non-invasive Auxiliary Diagnosis of Prostate Cancer. Front. Med. 8:800889. doi: 10.3389/fmed.2021.800889
Received: 24 October 2021; Accepted: 13 December 2021;
Published: 14 January 2022.
Edited by:
Surasak Saokaew, University of Phayao, ThailandReviewed by:
Jafar Rezaie, Urmia University of Medical Sciences, IranAcharaporn Duangjai, University of Phayao, Thailand
Copyright © 2022 Wang, Yuan, Fang, Hu, Zhang, Zhang and Zeng. This is an open-access article distributed under the terms of the Creative Commons Attribution License (CC BY). The use, distribution or reproduction in other forums is permitted, provided the original author(s) and the copyright owner(s) are credited and that the original publication in this journal is cited, in accordance with accepted academic practice. No use, distribution or reproduction is permitted which does not comply with these terms.
*Correspondence: Ling-Ling Zhang, llzhang@whu.edu.cn; Xian-Tao Zeng, zengxiantao1128@whu.edu.cn; zengxiantao1128@163.com