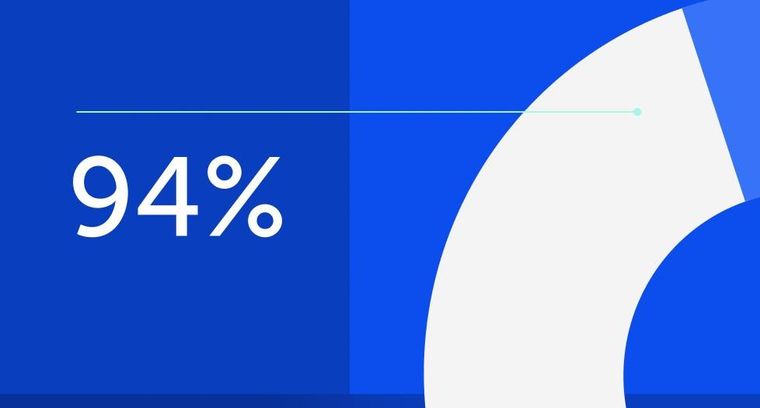
94% of researchers rate our articles as excellent or good
Learn more about the work of our research integrity team to safeguard the quality of each article we publish.
Find out more
REVIEW article
Front. Med., 27 August 2021
Sec. Nuclear Medicine
Volume 8 - 2021 | https://doi.org/10.3389/fmed.2021.723748
This article is part of the Research TopicClinical Impact of Technological Innovations in Nuclear MedicineView all 16 articles
Despite the introduction of new radiotherapy techniques, such as intensity modulated radiation therapy or stereotactic body radiation therapy, radiation induced lung injury remains a significant treatment related adverse event of thoracic radiation therapy. Functional lung avoidance radiation therapy is an emerging concept in the treatment of lung disease to better preserve lung function and to reduce pulmonary toxicity. While conventional ventilation/perfusion (V/Q) lung scintigraphy is limited by a relatively low spatial and temporal resolution, the recent advent of 68Gallium V/Q lung PET/CT imaging offers a potential to increase the accuracy of lung functional mapping and to better tailor lung radiation therapy plans to the individual's lung function. Lung PET/CT imaging may also improve our understanding of radiation induced lung injury compared to the current anatomical based dose–volume constraints. In this review, recent advances in radiation therapy for the management of primary and secondary lung tumors and in V/Q PET/CT imaging for the assessment of functional lung volumes are reviewed. The new opportunities and challenges arising from the integration of V/Q PET/CT imaging in radiation therapy planning are also discussed.
Radiation therapy has an increasing role in the treatment of both primary and secondary lung cancer (1, 2). In recent years, there has been a technologic revolution in radiation therapy (RT) with the introduction of new techniques, like intensity modulated radiation therapy (IMRT), image guided radiation therapy (IGRT), and stereotactic body radiation therapy (SBRT), which have improved the conformation with the target volumes.
However, radiation induced lung injury (RILI) remains a significant treatment related adverse event of thoracic RT (3). Indeed, the incidence of grade ≥ 2 lung toxicities is about 15-20% (3). Accordingly, a current major challenge of thoracic RT is to better preserve lung function and to reduce pulmonary toxicity.
Radiotherapy for the management of pulmonary lesions is currently based on the so-called anatomical planning. Lung volumes are delineated on CT images, and dose constraints are applied to these anatomical volumes, based on the simplistic assumption that the lungs are functionally homogeneous. However, it is well-established that the distribution of regional function into the lungs is non-uniform, especially in patients with lung cancer who frequently present with tobacco-related lung diseases and cancer treatment induced pulmonary disease.
Functional lung avoidance RT is an emerging concept in the treatment of lung disease.
The technique aim at personalizing RT treatment planning to individual's lung functional distribution, by prioritizing delivery of higher dose in non-functional pulmonary regions while sparing functional areas (4). In that purpose, establishing a functional map of the regional ventilation and perfusion in the lungs is required. Several groups have proposed to use conventional lung ventilation/perfusion scintigraphy and showed a potential interest of functional lung avoidance RT to reduce the dose to the functional lung (4). However, the low spatial and temporal resolution of conventional V/Q scintigraphy has limited accurate mapping of lung functional volumes and restrained its use in daily practice (5).
Ventilation/perfusion (V/Q) positron emission tomography/computed tomography (PET/CT) is a novel imaging modality for regional lung function assessment. As compared with conventional V/Q scan, V/Q PET/CT is inherently a vastly superior technology for image acquisition, with higher sensitivity, higher spatial and temporal resolution, superior quantitative capability, and a greater access to respiratory gated acquisition (5, 6). As a consequence, V/Q PET imaging offers an opportunity to improve the accuracy of lung functional mapping and its use for thoracic radiation therapy planning.
The advent of both advanced radiotherapy techniques and high resolution lung functional mapping is a real opportunity to personalize lung radiation therapy plans to an individual's own lung function and to minimize lung toxicity. Lung PET/CT imaging may also improve our understanding of radiation induced lung injury compared to the current anatomical based dose–volume constraints.
In this review, we will discuss recent developments in radiation therapy for the management of primary and secondary lung tumors, and in V/Q PET/CT imaging for the assessment of functional lung volumes. We will also review the new opportunities and challenges arising from the integration of V/Q PET/CT imaging in radiation therapy planning.
Lung cancer is the leading cancer in terms of frequency and mortality, with more than 1.6 million deaths per year (7). The role of curative-intent RT is well-recognized in both early stage (8) and locally advanced (9) non-small cell lung cancer (NSCLC).
In recent years, there have been efforts to diagnose lung cancers at earlier and more curable stages using annual low-dose computed tomography (CT) for at-risk populations (10). In the United States, these screening efforts have resulted in up to 30% of lung cancer cases being diagnosed at an early stage, i.e., stage I or II (11). These early tumors are amenable to surgical treatment, with local control rates of up to 96% and overall survival (OS) rates of ~50-60% at 3 years (12). Unfortunately, an important limitation to surgical treatment of these patients is that many have underlying pulmonary or cardiac comorbidities (13). In current practice, RT is the preferred alternative for these patients with early stage (preferably stage I disease with tumor size ≤ 3 cm) NSCLC who are unfit or refuse radical surgery (13).
Approximately 30% of NSCLC patients are diagnosed with locally advanced disease (stage III). This is a heterogeneous group that includes a large number of clinical presentations, often with a significant tumor burden (T3-T4 and N2-N3). Their management requires consultation within a multidisciplinary team. Radical chemoradiation, especially concurrent chemoradiation, and maintenance immune checkpoint-inhibitors (durvalumab) is the standard of care of unresectable stage III NSCLC (14, 15).
Historically, patients diagnosed with distant metastases secondary to solid tumors were considered incurable and the gold standard of treatment was systemic chemotherapy. Hellman and Weichselbaum suggested that a subset of patients with limited metastatic disease might benefit from aggressive local therapy (16, 17). These patients with a small number (five or fewer) of low volume metastatic lesions were classified as having oligometastatic disease. The management of oligometastatic disease has become a frequent question (18) because increasing evidence has shown that surgical resection or radiation therapy can lead to better outcomes (2). One of the main sites where radiotherapy is used in this setting is in the treatment of lung metastases. Stereotactic body radiation therapy (SBRT) is an excellent therapeutic modality, with control rates of >90% reported in prospective and retrospective series (19–21).
The 1980s saw the advent of three-dimensional conformal radiotherapy (3D-CRT) with the use of computed tomography (CT) for treatment planning and the replacement of Cerrobend blocking with multi-leaf collimators (MLC). These advances have allowed for the automation of radiation field formation and treatment planning that shapes the fields to the tumor volume. For the past decade, 3D-CRT has been the standard of care to treat unresectable local advanced lung cancer. In recent years there has been an increasing use of intensity-modulated radiation therapy (IMRT) (22). IMRT is a form of 3D-CRT where treatment planning system (TPS) determines non-uniform fluence to attain customized dose distribution, where dose is sculpted to target the tumor while sparing proximal organs at risk (OARs). IMRT is carried out by delivery of multiple beamlets of non-uniform fluence. The calculation of fluence is done by high performance computers using algorithms taking an iterative approach, called inverse planning. The inverse planning starts with desired result and works backwards to achieve best possible beam shape and fluence pattern.
IMRT itself has taken many forms including step-and-shoot that delivers discrete intensity levels and sliding window IMRT that delivers continuous intensity levels. Recently, there has been a growing interest in the rotating gantry IMRT techniques, tomotherapy and volumetric-modulated arc therapy (VMAT), that deliver radiation with MLCs and gantry both in motion. This type of radiation therapy allows the patient to be treated with the full 360° beam angle as the radiation source rotates around the patient during the irradiation. A higher modulation of the beam fluence in the whole arc is obtained thanks to the continuous movement of the leaf and the rotating gantry. The different IMRT techniques are equivalent except for a reduction in treatment time with VMAT (23–25).
However, to ensure full exploitation of this technique, it is necessary to monitor changes in tumor and OAR volume and/or position during treatment with image-guided radiation therapy (IGRT), such as cone-beam CT (CBCT) (26). Indeed, IGRT allows ensuring adequate target coverage while improving treatment outcome (27–30).
Since a secondary analysis of RTOG 0617, a randomized phase III trial comparing 60-74 Gy with concurrent chemotherapy in the treatment of inoperable stage III NSCLC in 544 patients, IMRT has become the gold standard technique (31). Indeed, IMRT was associated with similar survival and tumor control outcomes with a significant decrease in grade ≥ 3 radiation-induced lung fibrosis (RILF) compared with RTC3D (3.5% vs. 7.9) despite larger tumors and higher V5s (lung volume receiving 5 Gy or more) but similar V20s and mean lung doses (MLD) (31).
In the mid-1990s, stereotactic “body” radiotherapy (SBRT), also called stereotactic ablative radiotherapy (SABR), was first introduced by researchers at the Karolinska Institute in Stockholm by applying the principles of cranial stereotactic radiosurgery to extracranial tumor sites, particularly to the lung (32). Then, this technique was developed by several centers in the world (33–35). SBRT consists of delivering very high doses per fraction in a small number of sessions (usually between 3 and 8 fractions over 1-2 weeks) corresponding to a so-called hypofractionated scheme. Thus, radiation oncologists can perform an even more precise and tumor conformal radiation therapy with a rapid dose falloff in the lung parenchyma and adjacent structures leading to a higher biological effective dose (aiming at a BED10 of at least 100 Gy) directed to the tumors and to a more important destruction of the tumor cells (36–38).
A randomized trial comparing SBRT (54 Gy in 3 fractions or 48 Gy in 4 fractions) with conventional (66 Gy in 33 fractions) or moderate hypofractionation (50 Gy in 20 fractions) was conducted by the Trans-Tasmanian Radiation Oncology Group (TROG), known as the CHISEL trial. A total of 101 participants were included (2:1 with n = 66 SBRT and n = 35 conventional). Results showed superior local control (hazard ratio = 0.32, 95% CI 0.13-0.77, p = 0.0077) of the primary disease without an increase in major toxicity (3).
Multiple randomized controlled trials have sought to compare SBRT with surgery (lobar or sublobar resection) for stage I NSCLC, either in an unselected population or in a high-risk population (39–41). Unfortunately, all trials were stopped prematurely due to low inclusion rates, despite multiple adjustments of inclusion criteria to increase patient recruitment. However, a survival analysis including two phase III trials (STARS and ROSEL) by Chang et al. (39) demonstrated similar 3-year recurrence-free survival with SBRT or resection (86 and 80%, respectively, p = 0.54). OS was in favor of SBRT [95 vs. 79%, hazard ratio = 0.14, 95% confidence interval (CI) 0.017-1.190, p = 0.037]. However, these results should be interpreted with great caution because of the presence of numerous biases, including small patient numbers, unbalance cohorts in these two studies, the risk of type I inference error, and the relatively short follow-up. Based on these data, many international scientific societies and networks now consider SBRT as the best treatment strategy for medically inoperable patients with stage I NSCLC (40, 41).
In light of these results with lung SBRT in NSCLC, studies concerning the possible benefit of SBRT in patients with oligometastatic disease in the lung have been initiated (42–45). Recently, results of ongoing trials using SBRT in oligometastatic disease have been presented. They report improved overall survival and progression-free survival compared to standard therapy, confirming the benefit of local ablative therapy in limited systemic disease (46, 47). Thus, it is very likely that the treatment strategy and prognosis of these patients will change significantly. Indeed, more of them will be future candidates eligible for SBRT for lung metastases before and after multiple lines of immunotherapy and/or targeted agents.
Radiation exposure of the lungs is common during a course of therapeutic radiation for thoracic malignancies. Radiation-induced lung injury (RILI) encompasses radiation-induced pneumonitis (RIP), inflammation of the lung which may manifest as a dose-limiting acute or subacute toxicity, and radiation-induced lung fibrosis (RILF), a late effect of lung exposure to radiation. The diagnosis of RIP and RILF is based on clinical presentation and may be supported by associated imaging findings. Various grading scales are used (Tables 1, 2).
Despite the introduction of new radiotherapy techniques, RILI remains a significant treatment related morbidity of thoracic radiation therapy (3).
The occurrence of grade ≥ 2 RIP in the treatment of lung cancer has gradually decreased from 30–35% with 3D-radiotherapy (48) to 29–32% with IMRT (48). Grade ≥ 3 RIP was seen in about 10-15% with IMRT (49). Grade ≥ 2 and grade ≥3 RILF were seen in about 29 and 15% of patients with IMRT, respectively (50, 51).
Although quality of life after SBRT represents an important issue in assessing the effective impact of this radiation modality on patient management, it has been evaluated in few studies. Indeed, the majority of patients receiving SBRT have poor lung function at the time of diagnosis making them unfit for surgery, so it is crucial to know the effect of SBRT on lung function. The occurrence of RIP grade ≥ 2 and grade ≥ 3 are about 17-20% and 6-7%, respectively. The incidences of grade ≥ 2 and grade ≥ 3 RILF are about 15-20% and 5-6% with SBRT, respectively (3).
Thus, the thorax remains a challenging anatomical site for RT delivery, especially for patients with lung comorbidities, and the reduction of pulmonary side effects (and spare lung function) while achieving reasonable local control and sustaining its curative potential is an ongoing challenge faced by radiation oncologists and interdisciplinary treatment teams (3).
Currently, radiotherapy planning is anatomical for the treatment of lung tumors. Indeed, only CT images are used to define the lung volumes to which dose constraints are applied. To minimize toxicity to the OARs, in particular to the “healthy lung,” treatment planning system (TPS) are used to optimize the beam arrangements in order to respect the planning constraints. TPS allows to evaluate the dose delivered to each voxel in a volume of interest. Among the multiple plan options provided by TPS, the plan with the best therapeutic ratio (maximum tumor control with the least possible complications for normal tissues) is chosen. The dose-volume histogram (DVH) is used as a tool for comparison between plans.
In IMRT, the most important modifiable risk factors for RILF are the radiation dose and anatomical volume of lung irradiated, with lung V20 (volume of lung receiving 20 Gy or more) and mean lung dose (MLD) being validated in early studies. Consistency in lung volumes is important for reporting purposes, with the preferred method being total lung volume minus Gross Tumor Volume (GTV). In one of the most comprehensive analyses, a multi-institutional individual patient data meta-analysis investigated dosimetric predictors for RILF in patients treated with concurrent chemoradiation. This study reported symptomatic RILF in 30% of treated patients, with fatal RILF reported in 2% (52). The most important predictors for RILF included V20, older age, and carboplatin/paclitaxel chemotherapy. V20 as a continuous variable was also validated in a subgroup analysis of RTOG 0617 (31). In this analysis, treatment with IMRT was associated with a significantly lower risk of RILF despite having a higher V5, which had been associated with RILF in prior studies. Thus, the goal for radiation treatment planning is to achieve the lowest V20 and MLD possible, while V5 does not appear to be a critical variable. Commonly cited metrics include a V20 <35% and MLD <20 Gy, but lower doses are often achievable with modern treatment planning strategies. Patients with interstitial lung disease (ILD) should be treated with caution, as higher rates of symptomatic and fatal pneumonitis have been reported in this patient population (53).
In SBRT, there is few data about dose-effect relationship between lung anatomical volumes irradiated and the risk or the grade of toxicities. Saha et al. found that lower lobe tumor location, larger tumor size, PTV, mean lung dose, V20, and V12.5 were significant predictors of symptomatic RILF (54). Tumor size has been found to predict toxicity in previous studies (55–57). The only clinical factor associated with symptomatic RIP found was subclinical interstitial lung disease (45 vs. 1.6%) in the study of Okubo et al. (58). A recent review of 97 studies evaluated clinical and dosimetric predictors of RILI. Unfortunately, no threshold level of “tolerance dose volume” was found. However, the results seemed to show that the risk of symptomatic RILI was relatively low (<10-15%) with an MLD of 8 Gy and a V20 of 10-15% (59).
Current conventional anatomically-based planning simplistically assumes that the lungs are functionally homogeneous. However, it is well-known that the regional distribution of pulmonary function is heterogeneous, especially in patients with lung cancer, who frequently present with tobacco-related lung diseases such as chronic obstructive pulmonary disease (COPD) or emphysema, and treatment induced pulmonary disease from surgery, radiotherapy, or systemic therapies. In order to decrease pulmonary toxicities and preserve lung function, it has been proposed to personalize radiation therapy treatment planning to individual's lung functional distribution, i.e., to limit as far as possible the dose to the functional lung to the detriment of regions with already impaired lung function (4). Recent advances in radiotherapy techniques, with the use of inverse planning and TPS optimization algorithms, has made possible to add a constraint on a “functional lung” volume.
For that purpose, establishing a functional map of the regional function in the lungs is required. In that respect, the principle underlying Ventilation/Perfusion (V/Q) scintigraphy is very attractive. Indeed, lung scintigraphy allows to assess the regional distribution of ventilation and perfusion in the lungs. Ventilation is imaged after inhalation of inert gases or radiolabeled aerosols that reach terminal bronchioles and alveoli in proportion to regional distribution of ventilation. Perfusion is imaged after intravenous administration of macro-aggregated albumin (MAA) particles, which are trapped in the lung capillaries according to the regional blood flow.
Several studies showed that lung scintigraphy may provide additional information to assist in identifying patients at greater risk of radiation pneumonitis (60–62). Furthermore, studies have demonstrated the potential for lung SPECT imaging to be integrated into treatment planning to improve functional dose metrics (4). Several studies reported a reduction of mean functional volumes and mean lung dose when plans were optimized to spare functional lung (4). However, functional lung avoidance planning using SPECT imaging has not yet been adopted in daily clinical practice. Indeed, no clinical benefit has been clearly established so far (4). Furthermore, there are a wide variety of thresholds used for lung functional volumes delineation. This may be explained by the low spatial and temporal resolution of conventional V/Q scintigraphy, which prevents an accurate and reproducible mapping of lung functional volumes.
V/Q PET/CT is a novel imaging modality for regional lung function assessment. The same carrier molecules as conventional V/Q scintigraphy are used, but they are labeled with 68Gallium, a ß+ isotope, instead of 99mTc, allowing acquisition of images with PET technology (5, 63, 64). Similar physiological processes are therefore imaged using conventional V/Q scan or V/Q PET/CT, but PET is a vastly superior technology for image acquisition, with higher sensitivity, higher spatial and temporal resolution, superior quantitative capability, and a greater access to respiratory gated acquisition (5, 65). The test has already shown promising results in a variety of pulmonary conditions, such as pulmonary embolism diagnosis (63, 66) or pre-surgical assessment of lung cancer patients (67). Similarly, V/Q PET imaging offers an opportunity to improve the accuracy and utility of lung functional mapping for thoracic radiotherapy.
Besides improving the accuracy of lung functional volume delineation, pulmonary PET/CT imaging is also appealing for several reasons (5, 68). The acquisition time is much lower (5 min) than with conventional V/Q scan. V/Q PET imaging is a simple and non-invasive test, with no contraindication or side-effects, especially related to the injection of contrast media (allergy, renal dysfunction). No special procedure such as fasting or diet is required. The effective radiation dose of the scan is low, similar to the dose of conventional V/Q scan (~2-3 mSv for the PET acquisition), PET and CT respiratory-gated acquisition can be readily performed, which may further improve the accuracy of lung functional volumes delineation and improve the co-registration with the CT used for radiotherapy planning. In nuclear medicine facilities that routinely perform V/Q scans with Technegas, and equipped with a 68Ga generator, performing V/Q PET/CT does not require additional resources. 68Ga is an extremely convenient radiotracer for clinical use. 68Ga generators are increasingly available in nuclear medicine facilities owing to growing use for prostate cancer and neuroendocrine tumor imaging.
Le Roux et al. studied, in 30 lung cancer patients, the correlation between 68Ga V/Q PET/CT functional volumes and pulmonary function tests (PFTs) indices (69). The results showed that a percentage of lung volume with normal ventilation and perfusion >90% correctly identified impaired lung function in 93% of patients. A high degree of correlation between all functional lung volumes on V/Q PET/CT imaging and lung function as assessed by PFTs was also demonstrated. These results support the possible use of 68Ga V/Q PET/CT to predict potential consequences and side effects of treatments that may alter regional function, such as RT.
Although V/Q PET/CT is a very attractive test tool for functional lung avoidance planning, only few studies has been published on the topic so far. A first study simulated a 4D PET/CT perfusion-based radiotherapy plan in a cohort of NSCLC patients (70). These patients were planned to receive curative 3D-CRT at a dose of 60 Gy in 30 fractions. In this study, the definition of “perfused” or “well-perfused” lung on 68Ga-MAA PET/CT was based on an automated contour encompassing any 68Ga-MAA uptake or a maximum standardized uptake value (SUVmax) cutoff of 30%, respectively. To exclude any areas of clumping or other artifacts, automated contours were manually corrected. This PET/CT-guided planning improved the functional parameters V30, V40, V50, and V60 (all p-values < 0.05), and the mean functional dose to the lung was improved by a median value of 0.86 Gy (p < 0.01) for well-perfused lungs.
The same team evaluated the value of ventilation in addition to perfusion for functional lung volume sparing in IMRT. Perfusion-guided IMRT planning alone was able to decrease the functional lung dose while maintaining a consistent plan quality (71).
In the same population, changes in perfusion, ventilation, and CT lung density were assessed (72). The authors used deformable registration to register the functional images with RT planning (73). Then, they averaged the isodose volumes in 10 Gy bins intervals. Finally, the relative SUV loss was analyzed for ventilation and perfusion for each dose bin. They showed an almost perfectly linear negative dose-response relationship for perfusion (r2 = 0.99, P < 0.01) with a strongly negative correlation for ventilation (r2 = 0.95, P < 0.01). In some patients, peritumoral reperfusion/reventilation occurred. These results suggest that the effects of postradiotherapy may be closely correlated with impairments in perfusion, more so than ventilation. Thus, it would be necessary to preserve primarily the function of perfused lungs. In 68Ga V/Q PET/CT planning optimization, the aim would be to increase the weight of the radiation beams through the non-perfused lung regions and avoid the perfused lung regions without compromising the plan in terms of satisfying the established criteria for tumor coverage and preservation of other normal tissue (e.g., Figure 1).
Figure 1. Comparison between conventional (anatomically based) planning (A) and functional lung avoidance planning guided by 68Ga Q PET/CT (B) in a patient treated for a lung tumor by stereotactic body radiation therapy. Both planning show optimal coverage (red isodose line) of the tumor but the functional planning shows a decrease of the dose (20 Gy isodose in green and 16 Gy isodose in yellow) to the functional lung (in red the most functional areas and in blue the least functional areas). We can also see that on the corresponding anatomical images we cannot see any difference between the functional and non-functional areas.
For several years, radiotherapy in lung cancer has seen major technological advances, with the advent of motion management control (4D-CT, respiratory gating), image guidance, intensity modulated volumetric techniques or SBRT, which have led to an improvement in efficacy and tolerance. Nevertheless, further improvements in radiation therapy planning to spare lung function would be of interest for several reasons.
First, most of patients referred for thoracic radiation therapy have poor pulmonary function or have been heavily pretreated. Limiting the risk of high grade/fatal radiation pneumonitis remains therefore a major challenge. Second, multiple subsequent treatments are likely to be necessary for both primary NSCLC (second tumors) and lung metastases (oligo-progression). In these patients, toxicity should be kept as low as possible from the first treatment to preserve the possibility of re-irradiation, especially in SBRT. Third, patients with larger tumors or tumors close to critical structures would probably benefit the most from high-precision SBRT (74, 75), given the absence of other options and the higher risk of toxicity with current technology.
Moreover, V/Q PET/CT could be useful to better assess the relationship between radiation dose and lung toxicity. Indeed, in previous studies, the normal lung volume definitions for dose-volume histogram calculation are highly variable (76, 77). Usually, lung volume was defined as the bilateral lung excluding the planning target volume (Lung-PTV) (78–80) or excluding the gross tumor volume (Lung-GTV) (81–83). However, in RTOG 0617, lung volume was defined as the bilateral lung volume excluding the CTV (Lung-CTV). Currently, both RTOG and ESTRO-ACROP guidelines recommend using Lung-GTV delineation instead of Lung-PTV to standardize lung volume definition among different institutions (84, 85). However, there is only limited clinical evidence on which normal lung definition is better for symptomatic RILF prediction. The definition of a functional lung volume may be of interest to better predict toxicity.
Before implementation of functional lung avoidance planning using lung PET/CT imaging, a number of issues need to be resolved. Firstly, the definition of functional lung volumes was not consistent throughout publications. There is no consensus on the definition of the optimal functional region of the lung. The majority of current planning systems require the definition of a functional threshold, but continuum-based planning is possible (14, 32). Another possibility could be to define multiple levels of functional lung regions that do not overlap, with the most important dosimetric goals assigned to the most functional regions. Secondly, the dose constraints to the functional lung must be clarified. In the two studies that evaluated PET-guided functional lung avoidance planning, the functional lung dose constraints used were the same as those for anatomy-based planning. Most importantly, there is currently no publication that demonstrated the clinical benefit of functional lung imaging over anatomical lung imaging for radiotherapy planning. Indeed, no randomized interventional study has evaluated this question. Thus, it is necessary to test this hypothesis in a phase 3 randomized trial with appropriate statistical power to demonstrate a possible decrease in RILI using functional lung imaging.
Finally, there are currently different options for lung ventilation and perfusion imaging (SPECT, MR, ventilation CT and PET/CT) for the implementation of image-guided functional planning.
The availability and cost of the gas, the expertise required for gas imaging, including access to specialized equipment, and the need for image registration to the planning CT have been perceived as limitations to the clinical implementation of MR hyperpolarized gas imaging techniques in radiotherapy (86–88).
4D CT based methods have also shown promising results to estimate regional ventilation lung function (89, 90). However, high quality CT imaging is a key requirement given the potential for artifacts (90, 91). Furthermore, data in the literature seem to favor the use of perfusion data rather than ventilation for functional optimization of dose delivery to the lung. Indeed, perfusion defects are more frequent than ventilation defects, and both are more frequent than CT changes (92).
Thus, 68Ga Q PET/CT seems to be the imaging technique that can provide interesting functional data to guide radiotherapy with a fast and cost-effective imaging.
Two ongoing prospective trials should provide important data to support the initiation of such large scale randomized controlled trials.
Bucknell et al. are investigating a prospective dose escalation study with PET-guided radiotherapy planning that aims to show the value of PET-guided radiotherapy for IMRT stage III NSCLC (93). The HI-FIVE trial is a single arm interventional trial integrating 68Ga V/Q PET/CT respiratory-gated (four-dimensional) into radiation treatment planning to identify highly functioning lung volumes and avoidance of these using VMAT planning (NCT03569072). The aim is to evaluate the possibility for moderate dose escalation to the primary tumor only, while respecting conventional normal tissue toxicity constraints. For each patient, his radiation plan will be tailored to the location of his tumor and his lung functional mapping. Feasibility of this study is defined as meeting all dosimetric criteria for ≥15 of 20 patients. Thus, the purpose of this study is to provide valuable information on the feasibility of a larger-scale randomized trial and not to show a possible reduction in pulmonary toxicity or improvement in tumor control.
Our group has recently launched the PEGASUS trial, a single arm monocenter study in patients treated with SBRT for primary or secondary lung lesions (NCT04942275). Initially, patients will receive standard, “anatomical” planning, blinded to the results of the 68Ga Q PET/CT. In a second phase, patients will benefit from functional planning guided by 68Ga Q PET/CT. Each patient will be his own control. The doses to the functional lung will be calculated and compared for the two treatment planning. The primary aim is to estimate the percentage of patients for whom the dose to the functional lung can be reduced while respecting the standard constraints. Patients will have a clinical follow-up at 1 month and then every 3 months for 1 year. They will also undergo PFTs and repeated 68Ga Q PET/CT at 3 months and 1 year to assess the impact of SBRT on global and regional lung function.
Technological advances in radiation modalities have revolutionized the treatment of primary and secondary lung tumors. The advent of lung PET/CT imaging opens new perspectives for functional lung avoidance planning, in order to improve the therapeutic index, the technique may allow to decrease the dose to the functional lung and to reduce pulmonary toxicity, which may increase the possibility of dose escalation and re-irradiation. Results from ongoing clinical trials should help to guide future research and further push the boundaries of radiation therapy for years to come.
FL and MR: clinical experiment in lung cancer and radiation therapy and manuscript writing/editing. FB-B and P-YL: clinical experiment in PET/CT and manuscript writing/editing. All authors contributed to the article and approved the submitted version.
The authors declare that the research was conducted in the absence of any commercial or financial relationships that could be construed as a potential conflict of interest.
All claims expressed in this article are solely those of the authors and do not necessarily represent those of their affiliated organizations, or those of the publisher, the editors and the reviewers. Any product that may be evaluated in this article, or claim that may be made by its manufacturer, is not guaranteed or endorsed by the publisher.
1. Chansky K, Detterbeck FC, Nicholson AG, Rusch VW, Vallieres E, Groome P, et al. The IASLC lung cancer staging project: external validation of the revision of the TNM stage groupings in the eighth edition of the TNM classification of lung cancer. J Thorac Oncol. (2017) 12:1109–21. doi: 10.1016/j.jtho.2017.04.011
2. Timmerman RD, Bizekis CS, Pass HI, Fong Y, Dupuy DE, Dawson LA, et al. Local surgical, ablative, and radiation treatment of metastases. CA Cancer J Clin. (2009) 59:145–70. doi: 10.3322/caac.20013
3. Ball D, Mai GT, Vinod S, Babington S, Ruben J, Kron T, et al. Stereotactic ablative radiotherapy versus standard radiotherapy in stage 1 non-small-cell lung cancer (TROG 09.02 CHISEL): a phase 3, open-label, randomised controlled trial. Lancet Oncol. (2019) 20:494–503. doi: 10.1016/S1470-2045(18)30896-9
4. Bucknell NW, Hardcastle N, Bressel M, Hofman MS, Kron T, Ball D, et al. Functional lung imaging in radiation therapy for lung cancer: a systematic review and meta-analysis. Radiother Oncol. (2018) 129:196–208. doi: 10.1016/j.radonc.2018.07.014
5. Le Roux PY, Hicks RJ, Siva S, Hofman MS. PET/CT lung ventilation and perfusion scanning using Galligas and Gallium-68-MAA. Semin Nucl Med. (2019) 49:71–81. doi: 10.1053/j.semnuclmed.2018.10.013
6. Le Roux PY, Robin P, Salaun PY. New developments and future challenges of nuclear medicine and molecular imaging for pulmonary embolism. Thromb Res. (2018) 163:236–41. doi: 10.1016/j.thromres.2017.06.031
7. Bray F, Ferlay J, Soerjomataram I, Siegel RL, Torre LA, Jemal A. Global cancer statistics 2018: GLOBOCAN estimates of incidence and mortality worldwide for 36 cancers in 185 countries. CA Cancer J Clin. (2018) 68:394–424. doi: 10.3322/caac.21492
8. Postmus PE, Kerr KM, Oudkerk M, Senan S, Waller DA, Vansteenkiste J, et al. Early and locally advanced non-small-cell lung cancer (NSCLC): ESMO Clinical Practice Guidelines for diagnosis, treatment and follow-up. Ann Oncol. (2017) 28(suppl_4):iv1–iv21. doi: 10.1093/annonc/mdx222
9. Eberhardt WE, De Ruysscher D, Weder W, Le Pechoux C, De Leyn P, Hoffmann H, et al. 2nd ESMO Consensus Conference in Lung Cancer: locally advanced stage III non-small-cell lung cancer. Ann Oncol. (2015) 26:1573–88. doi: 10.1093/annonc/mdv187
10. Moyer VA, Force USPST. Screening for lung cancer: U.S. Preventive Services Task Force recommendation statement. Ann Inter Med. (2014) 160:330–8. doi: 10.7326/M13-2771
11. Marshall HM, Bowman RV, Yang IA, Fong KM, Berg CD. Screening for lung cancer with low-dose computed tomography: a review of current status. J Thorac Dis. (2013) 5(Suppl 5):S524–39. doi: 10.3978/j.issn.2072-1439.2013.09.06
12. Crabtree TD, Denlinger CE, Meyers BF, El Naqa I, Zoole J, Krupnick AS, et al. Stereotactic body radiation therapy versus surgical resection for stage I non-small cell lung cancer. J Thorac Cardiovasc Surg. (2010) 140:377–86. doi: 10.1016/j.jtcvs.2009.12.054
13. Altorki NK. Stereotactic body radiation therapy versus wedge resection for medically inoperable stage I lung cancer: tailored therapy or one size fits all? J Clin Oncol. (2010) 28:905–7. doi: 10.1200/JCO.2009.26.5157
14. Auperin A, Le Pechoux C, Rolland E, Curran WJ, Furuse K, Fournel P, et al. Meta-analysis of concomitant versus sequential radiochemotherapy in locally advanced non-small-cell lung cancer. J Clin Oncol. (2010) 28:2181–90. doi: 10.1200/JCO.2009.26.2543
15. Bezjak A, Temin S, Franklin G, Giaccone G, Govindan R, Johnson ML, et al. Definitive and adjuvant radiotherapy in locally advanced non-small-cell lung cancer: American Society of Clinical Oncology Clinical Practice Guideline Endorsement of the American Society for Radiation Oncology Evidence-Based Clinical Practice Guideline. J Clin Oncol. (2015) 33:2100–5. doi: 10.1200/JCO.2014.59.2360
16. Weichselbaum RR, Hellman S. Oligometastases revisited. Nat Rev Clin Oncol. (2011) 8:378–82. doi: 10.1038/nrclinonc.2011.44
17. Hellman S, Weichselbaum RR. Oligometastases. J Clin Oncol. (1995) 13:8–10. doi: 10.1200/JCO.1995.13.1.8
18. Ahmed KA, Torres-Roca JF. Stereotactic body radiotherapy in the management of oligometastatic disease. Cancer Control. (2016) 23:21–9. doi: 10.1177/107327481602300105
19. Milano MT, Katz AW, Zhang H, Okunieff P. Oligometastases treated with stereotactic body radiotherapy: long-term follow-up of prospective study. Int J Radiat Oncol Biol Phys. (2012) 83:878–86. doi: 10.1016/j.ijrobp.2011.08.036
20. Salama JK, Hasselle MD, Chmura SJ, Malik R, Mehta N, Yenice KM, et al. Stereotactic body radiotherapy for multisite extracranial oligometastases: final report of a dose escalation trial in patients with 1 to 5 sites of metastatic disease. Cancer. (2012) 118:2962–70. doi: 10.1002/cncr.26611
21. Binkley MS, Trakul N, Jacobs LR, von Eyben R, Le QT, Maxim PG, et al. Colorectal histology is associated with an increased risk of local failure in lung metastases treated with stereotactic ablative radiation therapy. Int J Radiat Oncol Biol Phys. (2015) 92:1044–52. doi: 10.1016/j.ijrobp.2015.04.004
22. Harris JP, Murphy JD, Hanlon AL, Le QT, Loo BW, Jr., Diehn M. A population-based comparative effectiveness study of radiation therapy techniques in stage III non-small cell lung cancer. Int J Radiat Oncol Biol Phys. (2014) 88:872-84. doi: 10.1016/j.ijrobp.2013.12.010
23. Chan C, Lang S, Rowbottom C, Guckenberger M, Faivre-Finn C, Committee IART. Intensity-modulated radiotherapy for lung cancer: current status and future developments. J Thorac Oncol. (2014) 9:1598–608. doi: 10.1097/JTO.0000000000000346
24. Jiang X, Li T, Liu Y, Zhou L, Xu Y, Zhou X, et al. Planning analysis for locally advanced lung cancer: dosimetric and efficiency comparisons between intensity-modulated radiotherapy (IMRT), single-arc/partial-arc volumetric modulated arc therapy (SA/PA-VMAT). Radiat Oncol. (2011) 6:140. doi: 10.1186/1748-717X-6-140
25. Yom SS, Liao Z, Liu HH, Tucker SL, Hu CS, Wei X, et al. Initial evaluation of treatment-related pneumonitis in advanced-stage non-small-cell lung cancer patients treated with concurrent chemotherapy and intensity-modulated radiotherapy. Int J Radiat Oncol Biol Phys. (2007) 68:94–102. doi: 10.1016/j.ijrobp.2006.12.031
26. Korreman S, Rasch C, McNair H, Verellen D, Oelfke U, Maingon P, et al. The European Society of Therapeutic Radiology and Oncology-European Institute of Radiotherapy (ESTRO-EIR) report on 3D CT-based in-room image guidance systems: a practical and technical review and guide. Radiother Oncol. (2010) 94:129–44. doi: 10.1016/j.radonc.2010.01.004
27. Kwint M, Conijn S, Schaake E, Knegjens J, Rossi M, Remeijer P, et al. Intra thoracic anatomical changes in lung cancer patients during the course of radiotherapy. Radiother Oncol. (2014) 113:392–7. doi: 10.1016/j.radonc.2014.10.009
28. Knap MM, Hoffmann L, Nordsmark M, Vestergaard A. Daily cone-beam computed tomography used to determine tumour shrinkage and localisation in lung cancer patients. Act Oncol. (2010) 49:1077–84. doi: 10.3109/0284186X.2010.498434
29. Persoon LC, Egelmeer AG, Ollers MC, Nijsten SM, Troost EG, Verhaegen F. First clinical results of adaptive radiotherapy based on 3D portal dosimetry for lung cancer patients with atelectasis treated with volumetric-modulated arc therapy (VMAT). Act Oncol. (2013)52:1484–9. doi: 10.3109/0284186X.2013.813642
30. Kilburn JM, Soike MH, Lucas JT, Ayala-Peacock D, Blackstock W, Isom S, et al. Image guided radiation therapy may result in improved local control in locally advanced lung cancer patients. Pract Radiat Oncol. (2016) 6:e73–80. doi: 10.1016/j.prro.2015.10.004
31. Chun SG, Hu C, Choy H, Komaki RU, Timmerman RD, Schild SE, et al. Impact of intensity-modulated radiation therapy technique for locally advanced non-small-cell lung cancer: a secondary analysis of the NRG oncology RTOG 0617 randomized clinical trial. J Clin Oncol. (2017) 35:56–62. doi: 10.1200/JCO.2016.69.1378
32. Blomgren H, Lax I, Naslund I, Svanstrom R. Stereotactic high dose fraction radiation therapy of extracranial tumors using an accelerator. Clinical experience of the first thirty-one patients. Acta Oncol. (1995) 34:861–70. doi: 10.3109/02841869509127197
33. Uematsu M, Shioda A, Tahara K, Fukui T, Yamamoto F, Tsumatori G, et al. Focal, high dose, and fractionated modified stereotactic radiation therapy for lung carcinoma patients: a preliminary experience. Cancer. (1998) 82:1062–70.
34. Wulf J, Hadinger U, Oppitz U, Olshausen B, Flentje M. Stereotactic radiotherapy of extracranial targets: CT-simulation and accuracy of treatment in the stereotactic body frame. Radiother Oncol. (2000) 57:225–36. doi: 10.1016/S0167-8140(00)00226-7
35. Timmerman R, Papiez L, McGarry R, Likes L, DesRosiers C, Frost S, et al. Extracranial stereotactic radioablation: results of a phase I study in medically inoperable stage I non-small cell lung cancer. Chest. (2003) 124:1946–55. doi: 10.1016/S0360-3016(03)01131-3
36. Videtic GM, Stephans KL. The role of stereotactic body radiotherapy in the management of non-small cell lung cancer: an emerging standard for the medically inoperable patient? Curr Oncol Rep. (2010) 12:235–41. doi: 10.1007/s11912-010-0108-1
37. Chua KLM, Sin I, Fong KW, Chua MLK, Onishi H. Stereotactic body radiotherapy for early stage lung cancer-historical developments and future strategies. Chin Clin Oncol. (2017) 6(Suppl 2):S20. doi: 10.21037/cco.2017.08.02
38. Nagata Y, Kimura T. Stereotactic body radiotherapy (SBRT) for Stage I lung cancer. Jpn J Clin Oncol. (2018) 48:405–9. doi: 10.1093/jjco/hyy034
39. Chang JY, Senan S, Paul MA, Mehran RJ, Louie AV, Balter P, et al. Stereotactic ablative radiotherapy versus lobectomy for operable stage I non-small-cell lung cancer: a pooled analysis of two randomised trials. Lancet Oncol. (2015) 16:630–7. doi: 10.1016/S1470-2045(15)70168-3
40. Ettinger DS, Wood DE, Aggarwal C, Aisner DL, Akerley W, Bauman JR, et al. NCCN guidelines insights: non-small cell lung cancer, Version 1.2020. J Natl Compr Cancer Netw. (2019) 17:1464–72. doi: 10.6004/jnccn.2019.0059
41. McGarry RC, Papiez L, Williams M, Whitford T, Timmerman RD. Stereotactic body radiation therapy of early-stage non-small-cell lung carcinoma: phase I study. Int J Radiat Oncol Biol Phys. (2005) 63:1010–5. doi: 10.1016/j.ijrobp.2005.03.073
42. Agolli L, Bracci S, Nicosia L, Valeriani M, De Sanctis V, Osti MF. Lung metastases treated with stereotactic ablative radiation therapy in oligometastatic colorectal cancer patients: outcomes and prognostic factors after long-term follow-up. Clin Colorectal Cancer. (2017) 16:58–64. doi: 10.1016/j.clcc.2016.07.004
43. Carvajal C, Navarro-Martin A, Cacicedo J, Ramos R, Guedea F. Stereotactic body radiotherapy for colorectal lung oligometastases: preliminary single-institution results. J BUON. (2015) 20:158–65.
44. Milano MT, Katz AW, Schell MC, Philip A, Okunieff P. Descriptive analysis of oligometastatic lesions treated with curative-intent stereotactic body radiotherapy. Int J Radiat Oncol Biol Phys. (2008) 72:1516–22. doi: 10.1016/j.ijrobp.2008.03.044
45. Rieber J, Streblow J, Uhlmann L, Flentje M, Duma M, Ernst I, et al. Stereotactic body radiotherapy (SBRT) for medically inoperable lung metastases-A pooled analysis of the German working group “stereotactic radiotherapy.” Lung Cancer. (2016) 97:51–8. doi: 10.1016/j.lungcan.2016.04.012
46. Gomez DR, Blumenschein GR, Jr., Lee JJ, Hernandez M, Ye R, Camidge DR, et al. Local consolidative therapy versus maintenance therapy or observation for patients with oligometastatic non-small-cell lung cancer without progression after first-line systemic therapy: a multicentre, randomised, controlled, phase 2 study. Lancet Oncol. (2016) 17:1672–82. doi: 10.1016/S1470-2045(16)30532-0
47. Palma DA, Olson R, Harrow S, Gaede S, Louie AV, Haasbeek C, et al. Stereotactic ablative radiotherapy verus standard of care palliative treatment in patients with oligometastatic cancers (SABR-COMET): a randomised, phase 2, open-label trial. Lancet. (2019) 393:2051–8. doi: 10.1016/S0140-6736(18)32487-5
48. Kasmann L, Dietrich A, Staab-Weijnitz CA, Manapov F, Behr J, Rimner A, et al. Radiation-induced lung toxicity - cellular and molecular mechanisms of pathogenesis, management, and literature review. Radiat Oncol. (2020) 15:214. doi: 10.1186/s13014-020-01654-9
49. Bradley JD, Paulus R, Komaki R, Masters G, Blumenschein G, Schild S, et al. Standard-dose versus high-dose conformal radiotherapy with concurrent and consolidation carboplatin plus paclitaxel with or without cetuximab for patients with stage IIIA or IIIB non-small-cell lung cancer (RTOG 0617): a randomised, two-by-two factorial phase 3 study. Lancet Oncol. (2015) 16:187–99. doi: 10.1016/S1470-2045(14)71207-0
50. Wijsman R, Dankers F, Troost EGC, Hoffmann AL, van der Heijden E, de Geus-Oei LF, et al. Comparison of toxicity and outcome in advanced stage non-small cell lung cancer patients treated with intensity-modulated (chemo-)radiotherapy using IMRT or VMAT. Radiother Oncol. (2017) 122:295–9. doi: 10.1016/j.radonc.2016.11.015
51. Jiang ZQ, Yang K, Komaki R, Wei X, Tucker SL, Zhuang Y, et al. Long-term clinical outcome of intensity-modulated radiotherapy for inoperable non-small cell lung cancer: the MD Anderson experience. Int J Radiat Oncol Biol Phys. (2012) 83:332–9. doi: 10.1016/j.ijrobp.2011.06.1963
52. Palma DA, Senan S, Tsujino K, Barriger RB, Rengan R, Moreno M, et al. Predicting radiation pneumonitis after chemoradiation therapy for lung cancer: an international individual patient data meta-analysis. Int J Radiat Oncol Biol Phys. (2013) 85:444–50. doi: 10.1016/j.ijrobp.2012.04.043
53. Bahig H, Filion E, Vu T, Chalaoui J, Lambert L, Roberge D, et al. Severe radiation pneumonitis after lung stereotactic ablative radiation therapy in patients with interstitial lung disease. Pract Radiat Oncol. (2016) 6:367–74. doi: 10.1016/j.prro.2016.01.009
54. Saha A, Beasley M, Hatton N, Dickinson P, Franks K, Clarke K, et al. Clinical and dosimetric predictors of radiation pneumonitis in early-stage lung cancer treated with Stereotactic Ablative radiotherapy (SABR) - an analysis of UK's largest cohort of lung SABR patients. Radiother Oncol. (2021) 156:153–9. doi: 10.1016/j.radonc.2020.12.015
55. Nakamura M, Nishimura H, Nakayama M, Mayahara H, Uezono H, Harada A, et al. Dosimetric factors predicting radiation pneumonitis after CyberKnife stereotactic body radiotherapy for peripheral lung cancer. Br J Radiol. (2016) 89:20160560. doi: 10.1259/bjr.20160560
56. Parker SM, Siochi RA, Wen S, Mattes MD. Impact of tumor size on local control and pneumonitis after stereotactic body radiation therapy for lung tumors. Pract Radiat Oncol. (2019) 9:e90–7. doi: 10.1016/j.prro.2018.09.003
57. Allibhai Z, Taremi M, Bezjak A, Brade A, Hope AJ, Sun A, et al. The impact of tumor size on outcomes after stereotactic body radiation therapy for medically inoperable early-stage non-small cell lung cancer. Int J Radiat Oncol Biol Phys. (2013) 87:1064–70. doi: 10.1016/j.ijrobp.2013.08.020
58. Okubo M, Itonaga T, Saito T, Shiraishi S, Mikami R, Nakayama H, et al. Predicting risk factors for radiation pneumonitis after stereotactic body radiation therapy for primary or metastatic lung tumours. Br J Radiol. (2017) 90:20160508. doi: 10.1259/bjr.20160508
59. Kong FS, Moiseenko V, Zhao J, Milano MT, Li L, Rimner A, et al. Organs at risk considerations for thoracic stereotactic body radiation therapy: what is safe for lung parenchyma? Int J Radiat Oncol Biol Phys. (2021) 110:172–87. doi: 10.1016/j.ijrobp.2018.11.028
60. Dhami G, Zeng J, Vesselle HJ, Kinahan PE, Miyaoka RS, Patel SA, et al. Framework for radiation pneumonitis risk stratification based on anatomic and perfused lung dosimetry. Strahlenther Onkol. (2017) 193:410–8. doi: 10.1007/s00066-017-1114-0
61. Farr KP, Kallehauge JF, Moller DS, Khalil AA, Kramer S, Bluhme H, et al. Inclusion of functional information from perfusion SPECT improves predictive value of dose-volume parameters in lung toxicity outcome after radiotherapy for non-small cell lung cancer: a prospective study. Radiother Oncol. (2015) 117:9–16. doi: 10.1016/j.radonc.2015.08.005
62. Wang D, Sun J, Zhu J, Li X, Zhen Y, Sui S. Functional dosimetric metrics for predicting radiation-induced lung injury in non-small cell lung cancer patients treated with chemoradiotherapy. Radiat Oncol. (2012) 7:69. doi: 10.1186/1748-717X-7-69
63. Hofman MS, Beauregard JM, Barber TW, Neels OC, Eu P, Hicks RJ. 68Ga PET/CT ventilation-perfusion imaging for pulmonary embolism: a pilot study with comparison to conventional scintigraphy. J Nucl Med. (2011) 52:1513–9. doi: 10.2967/jnumed.111.093344
64. Blanc-Beguin F, Elies P, Robin P, Tripier R, Kervarec N, Lemarie CA, et al. (68)Ga-Labelled carbon nanoparticles for ventilation PET/CT imaging: physical properties study and comparison with Technegas(R). Mol Imaging Biol. (2021) 23:62–9. doi: 10.1007/s11307-020-01532-6
65. Hicks RJ, Hofman MS. Is there still a role for SPECT-CT in oncology in the PET-CT era? Nat Rev Clin Oncol. (2012) 9:712–20. doi: 10.1038/nrclinonc.2012.188
66. Le Roux PY, Iravani A, Callahan J, Burbury K, Eu P, Steinfort DP, et al. Independent and incremental value of ventilation/perfusion PET/CT and CT pulmonary angiography for pulmonary embolism diagnosis: results of the PECAN pilot study. Eur J Nucl Med Mol Imaging. (2019) 46:1596–604. doi: 10.1007/s00259-019-04338-z
67. Le Roux PY, Leong TL, Barnett SA, Hicks RJ, Callahan J, Eu P, et al. Gallium-68 perfusion positron emission tomography/computed tomography to assess pulmonary function in lung cancer patients undergoing surgery. Cancer Imaging. (2016) 16:24. doi: 10.1186/s40644-016-0081-5
68. Bailey DL, Eslick EM, Schembri GP, Roach PJ. (68)Ga PET ventilation and perfusion lung imaging-current status and future challenges. Semin Nucl Med. (2016) 46:428–35. doi: 10.1053/j.semnuclmed.2016.04.007
69. Le Roux PY, Siva S, Steinfort DP, Callahan J, Eu P, Irving LB, et al. Correlation of 68Ga ventilation-perfusion PET/CT with pulmonary function test indices for assessing lung function. J Nucl Med. (2015) 56:1718–23. doi: 10.2967/jnumed.115.162586
70. Siva S, Devereux T, Ball DL, MacManus MP, Hardcastle N, Kron T, et al. Ga-68 MAA perfusion 4D-PET/CT scanning allows for functional lung avoidance using conformal radiation therapy planning. Technol Cancer Res Treat. (2016) 15:114–21. doi: 10.1177/1533034614565534
71. Siva S, Thomas R, Callahan J, Hardcastle N, Pham D, Kron T, et al. High-resolution pulmonary ventilation and perfusion PET/CT allows for functionally adapted intensity modulated radiotherapy in lung cancer. Radiother Oncol. (2015) 115:157–62. doi: 10.1016/j.radonc.2015.04.013
72. Siva S, Hardcastle N, Kron T, Bressel M, Callahan J, MacManus MP, et al. Ventilation/perfusion positron emission tomography–based assessment of radiation injury to lung. Int J Radiat Oncol Biol Phys. (2015) 93:408–17. doi: 10.1016/j.ijrobp.2015.06.005
73. Hardcastle N, Hofman MS, Hicks RJ, Callahan J, Kron T, MacManus MP, et al. Accuracy and utility of deformable image registration in (68)Ga 4D PET/CT assessment of pulmonary perfusion changes during and after lung radiation therapy. Int J Radiat Oncol Biol Phys. (2015) 93:196–204. doi: 10.1016/j.ijrobp.2015.05.011
74. Louie AV, Haasbeek CJ, Mokhles S, Rodrigues GB, Stephans KL, Lagerwaard FJ, et al. Predicting overall survival after stereotactic ablative radiation therapy in early-stage lung cancer: development and external validation of the amsterdam prognostic model. Int J Radiat Oncol Biol Phys. (2015) 93:82–90. doi: 10.1016/j.ijrobp.2015.07.241
75. Koshy M, Malik R, Weichselbaum RR, Sher DJ. Increasing radiation therapy dose is associated with improved survival in patients undergoing stereotactic body radiation therapy for stage I non-small-cell lung cancer. Int J Radiat Oncol Biol Phys. (2015) 91:344–50. doi: 10.1016/j.ijrobp.2014.10.002
76. Wang W, Xu Y, Schipper M, Matuszak MM, Ritter T, Cao Y, et al. Effect of normal lung definition on lung dosimetry and lung toxicity prediction in radiation therapy treatment planning. Int J Radiat Oncol Biol Phys. (2013) 86:956–63. doi: 10.1016/j.ijrobp.2013.05.003
77. Kabolizadeh P, Kalash R, Huq MS, Greenberger JS, Heron DE, Beriwal S. Dosimetric definitions of total lung volumes in calculating parameters predictive for radiation-induced pneumonitis. Am J Clin Oncol. (2015) 38:401–4. doi: 10.1097/COC.0b013e3182a2588f
78. Graham MV, Purdy JA, Emami B, Harms W, Bosch W, Lockett MA, et al. Clinical dose-volume histogram analysis for pneumonitis after 3D treatment for non-small cell lung cancer (NSCLC). Int J Radiat Oncol Biol Phys. (1999) 45:323–9. doi: 10.1016/S0360-3016(99)00183-2
79. Jenkins P, Watts J. An improved model for predicting radiation pneumonitis incorporating clinical and dosimetric variables. Int J Radiat Oncol Biol Phys. (2011) 80:1023–9. doi: 10.1016/j.ijrobp.2010.03.058
80. De Ruysscher D, Wanders S, Minken A, Lumens A, Schiffelers J, Stultiens C, et al. Effects of radiotherapy planning with a dedicated combined PET-CT-simulator of patients with non-small cell lung cancer on dose limiting normal tissues and radiation dose-escalation: a planning study. Radiother Oncol. (2005) 77:5–10. doi: 10.1016/j.radonc.2005.06.014
81. Hernando ML, Marks LB, Bentel GC, Zhou SM, Hollis D, Das SK, et al. Radiation-induced pulmonary toxicity: a dose-volume histogram analysis in 201 patients with lung cancer. Int J Radiat Oncol Biol Phys. (2001) 51:650–9. doi: 10.1016/S0360-3016(01)01685-6
82. Rodrigues G, Lock M, D'Souza D, Yu E, Van Dyk J. Prediction of radiation pneumonitis by dose - volume histogram parameters in lung cancer–a systematic review. Radiother Oncol. (2004) 71:127–38. doi: 10.1016/j.radonc.2004.02.015
83. Kong FM, Hayman JA, Griffith KA, Kalemkerian GP, Arenberg D, Lyons S, et al. Final toxicity results of a radiation-dose escalation study in patients with non-small-cell lung cancer (NSCLC): predictors for radiation pneumonitis and fibrosis. Int J Radiat Oncol Biol Phys. (2006) 65:1075–86. doi: 10.1016/j.ijrobp.2006.01.051
84. Kong FM, Ritter T, Quint DJ, Senan S, Gaspar LE, Komaki RU, et al. Consideration of dose limits for organs at risk of thoracic radiotherapy: atlas for lung, proximal bronchial tree, esophagus, spinal cord, ribs, and brachial plexus. Int J Radiat Oncol Biol Phys. (2011) 81:1442–57. doi: 10.1016/j.ijrobp.2010.07.1977
85. Nestle U, De Ruysscher D, Ricardi U, Geets X, Belderbos J, Pottgen C, et al. ESTRO ACROP guidelines for target volume definition in the treatment of locally advanced non-small cell lung cancer. Radiother Oncol. (2018) 127:1–5. doi: 10.1016/j.radonc.2018.02.023
86. Castillo R, Castillo E, Martinez J, Guerrero T. Ventilation from four-dimensional computed tomography: density versus Jacobian methods. Phys Med Biol. (2010) 55:4661–85. doi: 10.1088/0031-9155/55/16/004
87. Vinogradskiy YY, Castillo R, Castillo E, Chandler A, Martel MK, Guerrero T. Use of weekly 4DCT-based ventilation maps to quantify changes in lung function for patients undergoing radiation therapy. Med Phys. (2012) 39:289–98. doi: 10.1118/1.3668056
88. Yamamoto T, Kabus S, Bal M, Keall P, Benedict S, Daly M. The first patient treatment of computed tomography ventilation functional image-guided radiotherapy for lung cancer. Radiother Oncol. (2016) 118:227–31. doi: 10.1016/j.radonc.2015.11.006
89. Kipritidis J, Hofman MS, Siva S, Callahan J, Le Roux PY, Woodruff HC, et al. Estimating lung ventilation directly from 4D CT Hounsfield unit values. Med Phys. (2016) 43:33. doi: 10.1118/1.4937599
90. Eslick EM, Kipritidis J, Gradinscak D, Stevens MJ, Bailey DL, Harris B, et al. CT ventilation imaging derived from breath hold CT exhibits good regional accuracy with Galligas PET. Radiother Oncol. (2018) 127:267–73. doi: 10.1016/j.radonc.2017.12.010
91. Ireland RH, Tahir BA, Wild JM, Lee CE, Hatton MQ. Functional image-guided radiotherapy planning for normal lung avoidance. Clin Oncol. (2016) 28:695–707. doi: 10.1016/j.clon.2016.08.005
92. Evans ES, Hahn CA, Kocak Z, Zhou SM, Marks LB. The role of functional imaging in the diagnosis and management of late normal tissue injury. Semin Radiat Oncol. (2007) 17:72–80. doi: 10.1016/j.semradonc.2006.11.003
93. Bucknell N, Hardcastle N, Jackson P, Hofman M, Callahan J, Eu P, et al. Single-arm prospective interventional study assessing feasibility of using gallium-68 ventilation and perfusion PET/CT to avoid functional lung in patients with stage III non-small cell lung cancer. BMJ Open. (2020) 10:e042465. doi: 10.1136/bmjopen-2020-042465
Keywords: radiation—adverse effects, PET perfusion map, radiation planning, lung cancer, stereotactic body radiation therapy, intensity modulated radiation therapy, volumetric modulated arc therapy
Citation: Lucia F, Rehn M, Blanc-Béguin F and Le Roux P-Y (2021) Radiation Therapy Planning of Thoracic Tumors: A Review of Challenges Associated With Lung Toxicities and Potential Perspectives of Gallium-68 Lung PET/CT Imaging. Front. Med. 8:723748. doi: 10.3389/fmed.2021.723748
Received: 11 June 2021; Accepted: 09 August 2021;
Published: 27 August 2021.
Edited by:
Martin Huellner, University Hospital Zürich, SwitzerlandReviewed by:
Taiki Magome, Komazawa University, JapanCopyright © 2021 Lucia, Rehn, Blanc-Béguin and Le Roux. This is an open-access article distributed under the terms of the Creative Commons Attribution License (CC BY). The use, distribution or reproduction in other forums is permitted, provided the original author(s) and the copyright owner(s) are credited and that the original publication in this journal is cited, in accordance with accepted academic practice. No use, distribution or reproduction is permitted which does not comply with these terms.
*Correspondence: François Lucia, ZnJhbmNvaXMubHVjaWFAZ21haWwuY29t; orcid.org/0000-0001-7286-1350
Disclaimer: All claims expressed in this article are solely those of the authors and do not necessarily represent those of their affiliated organizations, or those of the publisher, the editors and the reviewers. Any product that may be evaluated in this article or claim that may be made by its manufacturer is not guaranteed or endorsed by the publisher.
Research integrity at Frontiers
Learn more about the work of our research integrity team to safeguard the quality of each article we publish.