- 1Anesthesia and Intensive Care Unit, Department of Biomedical Sciences and Public Health, Università Politecnica delle Marche, Ancona, Italy
- 2Experimental Animal Models for Aging Units, Scientific Technological Area, Istituto di Ricovero e Cura a Carattere Scientifico – Istituto Nazionale Ricovero e Cura Anziani, Ancona, Italy
- 3Anesthesia and Intensive Care Unit, Azienda Ospedaliera Universitaria “Ospedali Riuniti Umberto I – Lancisi – Salesi” of Ancona, Ancona, Italy
Objectives: Excessive oxygen (O2) administration may have a negative impact on tissue perfusion by inducing vasoconstriction and oxidative stress. We aimed to evaluate the effects of different inhaled oxygen fractions (FiO2) on macro-hemodynamics and microvascular perfusion in a rat model.
Methods: Isoflurane-anesthetised spontaneously breathing male Wistar rats were equipped with arterial (carotid artery) and venous (jugular vein) catheters and tracheotomy, and randomized into three groups: normoxia (FiO2 21%, n = 6), hyperoxia (FiO2 100%, n = 6) and mild hypoxia (FiO2 15%, n = 6). Euvolemia was maintained by infusing Lactate Ringer solution at 10 ml/kg/h. At hourly intervals for 4 h we collected measurements of: mean arterial pressure (MAP); stroke volume index (SVI), heart rate (HR), respiratory rate (by means of echocardiography); arterial and venous blood gases; microvascular density, and flow quality (by means of sidestream dark field videomicroscopy on the hindlimb skeletal muscle).
Results: MAP and systemic vascular resistance index increased with hyperoxia and decreased with mild hypoxia (p < 0.001 in both cases, two-way analysis of variance). Hyperoxia induced a reduction in SVI, while this was increased in mild hypoxia (p = 0.002). The HR increased under hyperoxia (p < 0.05 vs. normoxia at 3 h). Cardiax index, as well as systemic O2 delivery, did not significantly vary in the three groups (p = 0.546 and p = 0.691, respectively). At 4 h, microvascular vessel surface (i.e., the percentage of tissue surface occupied by vessels) decreased by 29 ± 4% in the hyperoxia group and increased by 19 ± 7 % in mild hypoxia group (p < 0.001). Total vessel density and perfused vessel density showed similar tendencies (p = 0.003 and p = 0.005, respectively). Parameters of flow quality (microvascular flow index, percentage of perfused vessels, and flow heterogeneity index) remained stable and similar in the three groups.
Conclusions: Hyperoxia induces vasoconstriction and reduction in skeletal muscle microvascular density, while mild hypoxia has an opposite effect.
Introduction
Supplemental oxygen (O2) is one of the most frequently applied therapies in clinical medicine and usually represents a life-saving intervention in patients with hypoxemia due to respiratory failure. Since clinicians often tolerate supranormal PaO2 values as perceived as a safety buffer against hypoxemia, many critically ill patients in Intensive Care Units (ICUs) are at risk of being exposed to excessive O2 administration (1). Nonetheless, increasing evidence shows the potentially deleterious effects of hyperoxia, which enhances oxidative stress and inflammation in the lungs and other organs, causes vasoconstriction, reduces coronary blood flow and cardiac output and may alter microvascular perfusion (2). In mechanically ventilated patients, short term hyperoxia induced a reduction in sublingual microvascular density and flow (3): this may lead to a paradoxical net reduction in regional O2 delivery to the cells. The exposure to hyperoxemia in the ICU was associated with adverse outcome (4–6). On the contrary, the use of a more restrictive O2 therapy, with precise control of arterial oxygenation, was able to improve survival in critically ill patients (7).
The concept of permissive hypoxemia has been postulated based on the evidence that the body can mount a complex process of adaption to a condition of reduced O2 availability (8). At the tissue level, this adaptation to hypoxia includes a vasodilation response with a rise in microvascular vessel density and a concomitant decrease in blood flow (9). According to the theory of permissive hypoxemia, in selected ICU patients, tolerating levels of arterial oxygenation lower than those that are currently accepted may be advantageous as compared to the exposure to hyperoxia (8).
In this context, it is of utmost importance to evaluate the physiological responses to different levels of inspired O2 fractions, in order to understand what may be the benefits and harms of hyperoxia exposure as opposed to accepting a mild hypoxemia status. In this preclinical study we evaluated the effects of normoxia, hyperoxia, or mild hypoxia on macro-hemodynamics and the skeletal muscle microcirculation in a model of anesthetized spontaneously breathing rats.
Materials and Methods
The study protocol was approved and authorized by the Italian “Ministero della Salute – Direzione Generale della Sanità Animale e dei Farmaci Veterinari,” authorization number 865/2016-PR, protocol number 8502E.7 of September 14th, 2016.
Adult (9–12 month old) male specific-pathogen free Wistar rats (500 ± 75 g body weight) were used. Surgical instrumentation was performed under 2–5% isoflurane anesthesia with maintenance of spontaneous breathing in a 21% O2 gas mixture (10). The rectal temperature was monitored and maintained at 37°C throughout the experiment by means of a heated mat. The left common carotid artery and right internal jugular vein were cannulated for arterial pressure monitoring and fluid infusion, respectively. The arterial line was connected to a pressure transducer (TruWave, Edwards Lifesciences Corp., Irvine, CA) for continuous monitoring of mean arterial pressure (MAP). A tracheostomy was performed using a 14-gauge cannula and connected to a T-piece to maintain anesthesia and vary the fraction of inspired O2 (FiO2). The bladder was exposed through a small laparotomy and cannulated for drainage and quantification of urine output. For microcirculatory assessment, a 2-cm skin incision was performed on the medial side of the right hindlimb, the perimysium was separated from the muscle by blunt dissection in order to minimize tissue damage and bleeding (10). Euvolaemia was obtained by intravenous administration of 4 ml*kg−1 Ringer's Lactate followed by a continuous infusion of 10 ml*kg−1*h−1. The animals were allowed to stabilize for 60 min.
After baseline measurements, rats were randomized to the following groups: normoxia (FiO2 = 21%, n = 6); hyperoxia (FiO2 = 100%, n = 6); mild hypoxia (FiO2 = 15%, n = 6). The FiO2 was changed accordingly and maintained stable for 4 h.
Measurements
At baseline and at hourly intervals, MAP, and urine output were recorded. Transthoracic echocardiography (Philips Sonos, with a 6–12 MHz frequency transducer) was performed as described elsewhere (11). In brief, the stroke volume (SV) was estimated from the velocity-time integral of the ascending aortic flow (suprasternal view) using pulsed-wave Doppler. The heart rate (HR) was calculated by measuring the time between six consecutive cycles from the start of each Doppler trace to account for variation with respiration. The cardiac output (CO) was calculated by multiplying SV and HR. The ejection fraction (EF) and fractional shortening (FS) were calculated by measuring internal left ventricular end-diastolic and end-systolic diameters from the long-axis and short-axis parasternal views. The respiratory rate (RR) was measured by echocardiography from the frequency of diaphragmatic excursions as visualized in M-mode. Arterial and venous blood samples (0.2 ml) were collected in heparinized syringes for blood gas analysis (EDAN i15 blood gas analyzer, GEPA Srl, Bollate MI, Italy), which included measurements of haemoglobin (Hb), lactate and glucose. The arterial O2 content (CaO2), systemic O2 delivery (DO2), and O2 consumption (VO2) were calculated using standard formulae. Global O2 extraction ratio (O2ER) was calculated as (CaO2-CvO2)/CaO2. The skeletal muscle microcirculation was evaluated on the vastus medialis of the left quadriceps femoris muscle with sidestream dark field videomicroscopy (Microscan, Microvision Medical, Amsterdam, NL), which enables the real-time in vivo visualization of blood flow in microvascular beds (12). A supportive device was used to enhance stability during image acquisition. Videos from 5 adjacent sites were recorded with adequate contrast, focus and stability; absence of pressure artifacts was defined by preservation of venular perfusion. Parameters of vessel density and microvascular flow quality were calculated offline for small vessels (diameter <20 microns) using the Automated Vascular Analysis 3.0 software (Microvision Medical, Amsterdam, NL), as described elsewhere (13). These parameters included the De Backer score, total vessel density (TVD), perfused vessel density (PVD), microvascular flow index (MFI), percentage of perfused vessels (PPV), flow heterogeneity index (FHI) (13). In addition, we calculated the Vessel Surface (VS) as the percentage of the image surface occupied by vessels. At end-experiment, rats were euthanized with an intravenous overdose of pentobarbital.
Statistical Analysis
This was performed using GraphPad version 5 (GraphPad Software, La Jolla, CA, USA). Normality of distribution was assessed using the Kolmogorov-Smirnov test. Data are expressed as mean ± standard deviation (SD) or standard error (SE) or median [25th to 75th percentile], as appropriate. Parametric data were analyzed using repeated measures two-way analysis of variance followed by Dunnet's (between groups) and Tukey's (between time points) multiple comparisons tests. Non-parametric data were analyzed using the Friedman test with Dunn's post-hoc test for multiple comparisons and the Mann-Whitney U-test. A p-value < 0.05 was used to indicate statistical significance.
Results
All rats survived until the end of the experiment. Changes in blood gases are reported in Table 1. Under hyperoxia, the RR tended to be lower and the PaCO2 significantly increased over time, while the pH remained substantially stable and similar between the three groups due to metabolic compensation. The PaO2/FiO2 ratio decreased under hyperoxia (p < 0.05 vs. normoxia at all time-points).
Macro-hemodynamic parameters are shown in Table 2. MAP transiently increased after 1 h of hyperoxia (by 15 ± 6% at 1 h, p < 0.05 vs. normoxia, Figure 1) and was stably reduced under mild hypoxia (Table 2) with a maximum decrease of 35 ± 6% at 3 h (p < 0.01 vs. normoxia, Figure 1). The SVRI showed a similar tendency (Table 2). The SVI decreased with hyperoxia as compared to baseline (by a maximum of 14 ± 5 % after 2 h, p < 0.05 vs. normoxia, Figure 1), while it tended to increase under mild hypoxia (Figure 1 and Table 2). The HR increased with hyperoxia, so that the CI remained substantially unchanged over time. No variations were observed in EF or FS with different FiO2 values.
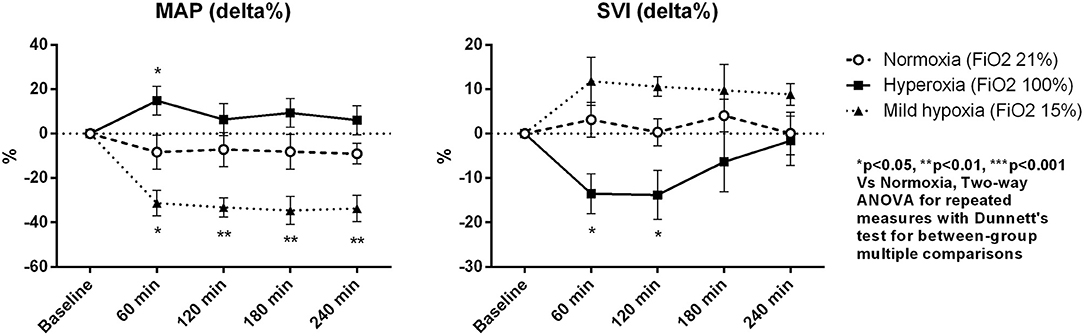
Figure 1. Percentage variations of mean arterial pressure (MAP) and stroke volume index (SVI). Data are expressed as mean ± standard error.
Although the DO2 tended to be higher in the hyperoxia group, the difference with the other two groups did not reach statistical significance. We did not observe significant variations in VO2. The O2ER increased in mild hypoxia and normoxia, while it tended to be lower under hyperoxia at all time-points. Lactate levels were lower under hyperoxia, while they slightly increased in mild hypoxia, despite remaining <2 mmol/L in all cases.
Microvascular density decreased under hyperoxia and tended to increase with mild hypoxia (Table 3 and Figure 2). At 4 h, microvascular VS decreased by 29 ± 4% in the hyperoxia group and increased by 19 ± 7 % in mild hypoxia group (p < 0.001, Figure 3). Total vessel density and perfused vessel density showed similar tendencies (p = 0.003 and p = 0.005, respectively, Figure 3). Parameters of flow quality (MFI, PPV, and FHI) remained stable and similar in the three groups.
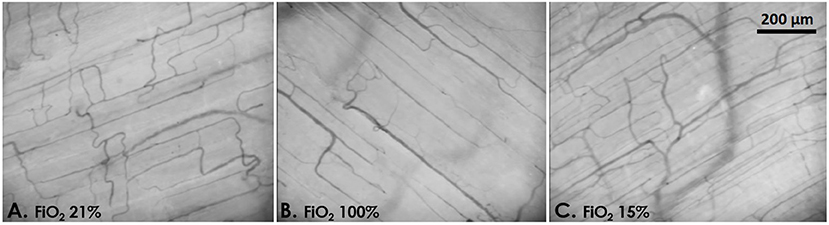
Figure 2. Example images of the rat skeletal muscle microcirculation under normoxia [(A) FiO2 21%, PaO2 92 mmHg], hyperoxia [(B) FiO2 100%, PaO2 305 mmHg], and mild hypoxia [(C) FiO2 15%, PaO2 58 mmHg].
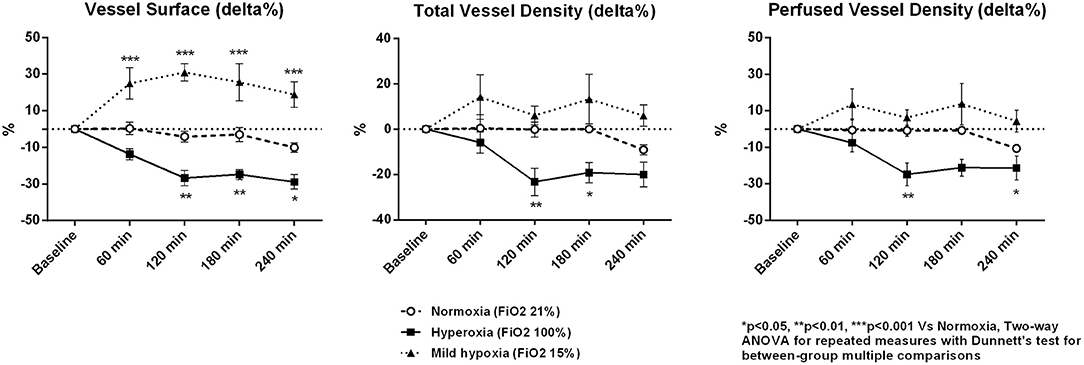
Figure 3. Percentage variations of microcirculatory vessel surface, total vessel density, and perfused vessel density. Data are expressed as mean ± standard error.
No difference was observed in the cumulative urine output between the three groups (normoxia: 4.9 [3.1–7.8] ml; hyperoxia: 5 [4–6.3] ml; mild hypoxia: 5 [3.6–9.2] ml; p = 0.921).
Discussion
In this preclinical study we evaluated the physiological macro-hemodynamic and microcirculatory responses to a 4-h exposure to different FiO2 levels in a model of anesthetised spontaneously breathing rats. Our study shows that hyperoxia leads to a vasoconstriction response with a transient increase in MAP and SVR. Breathing 100% FiO2 induced a reduction in SVI with no significant variation in CI due to a parallel increase in HR. At the tissue level, hyperoxia induced a reduction in microvascular vessel density in the skeletal muscle, while indices of microvascular flow quality were unaltered. On the contrary, mild hypoxia (FiO2 15%) led to a reduction in MAP and SVRI without inducing any significant variation in SVI and CI, and induced a vasodilatory response in the microcirculation, with an increase in microvascular vessel density and no change in flow quality.
The human body has the ability to mount an impressive adaptive response to a condition of even extreme and prolonged hypoxia (14). Several studies on high-altitude climbers and highlanders suggest that microvascular regulation plays an important role in this process (9, 15–18). The cardiovascular effects of hypoxia depend on a complex interaction between local tissue responses to reduced O2 availability and the activation of chemoreceptors and baroreceptors as part of autonomic nervous system reflexes (19). Hypoxic vasodilation is a well-known adaptive response to an acute O2 supply-demand mismatch, aimed to restore tissue O2 delivery. Several mechanisms are implicated in this vasodilation, although, a crucial role is played by the NO pathway: hypoxemia induces increased production of NO by nitric oxide synthetase isoforms, reduces NO release by haemoglobin and NO deactivation by the mitochondrial cytochrome C oxidase (20). Chemoreceptor stimulation during systemic hypoxia also induces the activation of the sympathetic nervous system, however its vasoconstrictor effect on the skeletal muscle vasculature is blunted by the locally-released vasodilator autacoids (21). The vasodilation of resistance arteries leads to a reduction in peripheral vascular resistance and arterial pressure, with a compensatory increase in heart rate and cardiac output (22). In our short-term model, rats subjected to mild hypoxia showed an increase in the skeletal muscle microvascular density with no impairment in capillary blood flow. This response was not secondary to an increase in convective flow or perfusion pressure (CI was globally unchanged and MAP decreased under hypoxia), but appeared more as an intrinsic mechanism of the microcirculation to optimize tissue O2 extraction capacity. In fact, we did not observe a clinically significant activation of anaerobic metabolism (blood lactate levels remained low). Contrary to previous reports (22), in our experiments the HR tended to decrease under mild hypoxia, while the SVI increased thus allowing the maintenance of a stable cardiac output. Cardio-inhibitory reflexes (i.e. the Bezold-Jarisch reflex) induced by cardiac receptor stimulation may have played a role in inhibiting a rise in HR in our anesthetised rat model (23).
Unlike hypoxia, the exposure to high concentrations of inspired O2 can only be traced back to the relatively modern era. Therefore, the responses to hyperoxia are more likely the results of a iatrogenic insult rather than part of evolutionary innate mechanisms of adaptation to high O2 concentrations. Hyperoxia induces oxidative stress and potential damage to various organs (2). In the lung, exposure to 100% O2 induces inflammation, impaired production of surfactant and resorption atelectasis (2). In our study, rats subjected to hyperoxia showed a significant reduction in the PaO2/FiO2 ratio, which is consistent with the existing evidence. The hemodynamic response to arterial hyperoxia seems mainly driven by enhanced production of peroxynitrates and reduced NO bioavailability, and is characterized by vasoconstriction and increased systemic vascular resistance (24), which also involves the coronary vascular bed and provokes a reduction in cardiac output and myocardial O2 consumption (2, 25). In a study in rabbits, normobaric hyperoxia induced a reversible decrease in sublingual microvascular density and vessel diameters (26). Pre-clinical studies using different techniques for microcirculatory evaluation confirmed these results (27) and also showed different response in vessels of varying diameter in response to hyperoxia, with constriction of the smallest order 1 and 2 vessels with no change in the larger order 3 arterioles, allowing blood to by-pass the tissue (28). Similar hyperoxia-induced microvascular alterations were found in healthy volunteers (29) and ICU patients (3). In the present study, we found a similar reduction in vessel density in the skeletal muscle microcirculation, but no alteration in blood flow quality: several factors may explain this discrepancy, including the use of different sedative agents, and the fact that the CI remained globally unaltered in our rats. This hyperoxia-induced vasoconstriction was not described during sepsis (30, 31). Vasoplegia due to an underlying microvascular dysfunction in this particular condition could be responsible for the lack of a vasoconstrictive response to hyperoxia.
Exposure to hyperoxia was associated with increased mortality in several categories of ICU patients (4–7, 32). In patients with septic shock, hyperoxia was associated with higher risk of mortality and serious adverse events, including ICU-acquired weakness and atelectasis (33). In recent years, several clinical trials showed that more conservative oxygenation strategies could be beneficial in critically ill patients (7). The concept of permissive hypoxemia has been formulated with the rationale of reducing mortality and morbidity in selected hypoxemic patients by targeting levels of PaO2 lower than those that are currently accepted, thereby limiting excessive O2 exposure (8). Recently, two randomized controlled trials in patients with acute hypoxemic respiratory failure failed to show an improvement in survival with the use of a lower oxygenation target as compared to a more liberal strategy and the use of a higher PaO2 target (34, 35). Nonetheless, the fact remains that no evidence exists to support an unnecessary exposure to hyperoxia in non-hypoxemic patients. If hemoglobin is fully saturated, excess of O2 will only marginally increase arterial O2 content; on the other hand, vasoconstriction and a reduction in microvascular vessel density could lead to a paradoxical decrease in regional O2 delivery (6). In fact, we did not find a significant increase in systemic DO2 in rats subjected to hyperoxia. This is consistent with data on healthy volunteers, in whom supraphysiological arterial oxygen tensions had no effect on systemic DO2, whereas sublingual microcirculatory PVD decreased in a dose-dependent fashion (36).
Our study has several limitations. First, we evaluated the microcirculatory response in the resting skeletal muscle: we cannot exclude that hyperoxia and mild hypoxia could elicit different responses in the splanchnic microcirculation. Second, mild hypoxemia was artificially induced by administering a hypoxic gas mixture: this model cannot closely reproduce the clinical scenario of an acute respiratory failure, in which the pulmonary inflammatory process could influence the systemic vascular response to varying O2 levels. Third, we observed a similar reduction in Hb levels in all groups, which was probably due to hemodilution and repeated blood sampling: this may have influenced the macro-hemodynamic and microvascular responses observed by contributing to changes in CaO2 and blood viscosity. Fourth, we did not measure markers of oxidative stress (37) or inflammation (38): this may have helped to understand the pathophysiological basis of cardiovascular changes induced by hyperoxia. Moreover, we did not measure variations in NO levels and could not demonstrate the role of NO in microvascular perturbations induced by hypoxia/hyperoxia. Fifth, we evaluated the physiological response to different FiO2 in a model of anesthetised, otherwise healthy, rats. It will be important to evaluate the ability of the body to respond to hyperoxia or mild hypoxia in models of critical illness, such as sepsis. Moreover, it would be interesting to explore the cardiovascular changes induced by varying O2 levels in aged rats, since aging or age-related chronic diseases (such as arterial hypertension) may influence microvascular reactivity (39) and elderly patients represent a large part of the critical care population. Lastly, our microcirculatory analysis was focused on small vessels (diameter <20 microns) as being the main site of O2 delivery to the cells, while other authors using different techniques have also explored the response of arterioles of different orders (28): evaluating the response of larger resistance arterioles may be important to clarify the role of microcirculation in determining macro-hemodynamic changes induced by varying O2 levels.
Conclusions
In a condition of mild hypoxemia, the peripheral (skeletal muscle) microcirculation has the ability to adapt perfusion to maintain tissue O2 availability and meet cellular metabolic demand, whereas, hyperoxia elicits a vasoconstrictive response with a potential paradoxical decrease in peripheral tissue perfusion.
Data Availability Statement
The raw data supporting the conclusions of this article will be made available by the authors, without undue reservation.
Ethics Statement
The animal study was reviewed and approved by Ministero della Salute – Direzione Generale della Sanità Animale e dei Farmaci Veterinari.
Author Contributions
ED designed the study, performed the experiments and statistical analysis, interpreted the data, and drafted the manuscript. EC, FO, CS, and SC performed the experiments, interpreted the data, and drafted the manuscript. AC, RD, and EA contributed to the data analysis and interpretation and revised the manuscript. MP and AD designed the study, interpreted the data, and revised the manuscript. All authors read and approved the submitted version of the manuscript and agreed both to be personally accountable for the author's own contributions and to ensure that questions related to the accuracy or integrity of any part of the work, even ones in which the author was not personally involved, are appropriately investigated, resolved, and the resolution documented in the literature.
Conflict of Interest
The authors declare that the research was conducted in the absence of any commercial or financial relationships that could be construed as a potential conflict of interest.
Acknowledgments
The authors wish to thank the staff of the Servizio di Allevamento e Sperimentazione Animale, Polo Scientifico Tecnologico Masera-IRCCS INRCA of Ancona (Italy) for the valuable contribution.
References
1. Kraft F, Andel H, Gamper J, Markstaller K, Ullrich R, Klein KU. Incidence of hyperoxia and related in-hospital mortality in critically ill patients: a retrospective data analysis. Acta Anaesthesiol Scand. (2018) 62:347–56. doi: 10.1111/aas.13047
2. Damiani E, Donati A, Girardis M. Oxygen in the critically ill: friend or foe? Curr Opin Anaesthesiol. (2018) 31:129–35. doi: 10.1097/ACO.0000000000000559
3. Donati A, Damiani E, Zuccari S, Domizi R, Scorcella C, Girardis M, et al. Effects of short-term hyperoxia on erythropoietin levels and microcirculation in critically Ill patients: a prospective observational pilot study. BMC Anesthesiol. (2017) 17:49. doi: 10.1186/s12871-017-0342-2
4. Ni YN, Wang YM, Liang BM, Liang ZA. The effect of hyperoxia on mortality in critically ill patients: a systematic review and meta analysis. BMC Pulm Med. (2019) 19:53. doi: 10.1186/s12890-019-0810-1
5. You J, Fan X, Bi X, Xian Y, Xie D, Fan M, et al. Association between arterial hyperoxia and mortality in critically ill patients: a systematic review and meta-analysis. J Crit Care. (2018) 47:260–8. doi: 10.1016/j.jcrc.2018.07.014
6. Damiani E, Adrario E, Girardis M, Romano R, Pelaia P, Singer M, et al. Arterial hyperoxia and mortality in critically ill patients: a systematic review and meta-analysis. Crit Care. (2014) 18:711. doi: 10.1186/s13054-014-0711-x
7. Chu DK, Kim LH, Young PJ, Zamiri N, Almenawer SA, Jaeschke R, et al. Mortality and morbidity in acutely ill adults treated with liberal versus conservative oxygen therapy (IOTA): a systematic review and meta-analysis. Lancet. (2018) 391:1693–705. doi: 10.1016/S0140-6736(18)30479-3
8. Martin DS, Grocott MPW. Oxygen therapy in critical illness: precise control of arterial oxygenation and permissive hypoxemia. Crit Care Med. (2013) 41:423–32. doi: 10.1097/CCM.0b013e31826a44f6
9. Martin DS, Goedhart P, Vercueil A, Ince C, Levett DZ, Grocott MP, et al. Changes in sublingual microcirculatory flow index and vessel density on ascent to altitude. Exp Physiol. (2010) 95:880–91. doi: 10.1113/expphysiol.2009.051656
10. Damiani E, Dyson A, Zacchetti L, Donati A, Singer M. Exploring alternative routes for oxygen administration. Intensive Care Med Exp. (2016) 4:34. doi: 10.1186/s40635-016-0108-z
11. Slama M, Susic D, Varagic J, Ahn J, Frohlich ED. Echocardiographic measurement of cardiac output in rats. Am J Physiol Heart Circ Physiol. (2003) 284:H691–7. doi: 10.1152/ajpheart.00653.2002
12. Goedhart PT, Khalilzada M, Bezemer R, Merza J, Ince C. Sidestream Dark Field (SDF) imaging: a novel stroboscopic LED ring-based imaging modality for clinical assessment of the microcirculation. Opt Express. (2007) 15:15101–14. doi: 10.1364/OE.15.015101
13. Damiani E, Ince C, Orlando F, Pierpaoli E, Cirioni O, Giacometti A, et al. Effects of the infusion of 4% or 20% human serum albumin on the skeletal muscle microcirculation in endotoxemic rats. PLoS ONE. (2016) 11:e0151005. doi: 10.1371/journal.pone.0151005
14. Grocott MP, Martin DS, Levett DZ, McMorrow R, Windsor J, Montgomery HE, et al. Arterial blood gases and oxygen content in climbers on Mount Everest. N Engl J Med. (2009) 360:140–9. doi: 10.1056/NEJMoa0801581
15. Hilty MP, Merz TM, Hefti U, Ince C, Maggiorini M, Pichler Hefti J. Recruitment of non-perfused sublingual capillaries increases microcirculatory oxygen extraction capacity throughout ascent to 7126 m. J Physiol. (2019) 597:2623–38. doi: 10.1113/JP277590
16. Gilbert-Kawai E, Coppel J, Court J, van der Kaaij J, Vercueil A, Feelisch M, et al. Sublingual microcirculatory blood flow and vessel density in Sherpas at high altitude. J Appl Physiol (1985). (2017) 122:1011–8. doi: 10.1152/japplphysiol.00970.2016
17. Dinenno FA. Skeletal muscle vasodilation during systemic hypoxia in humans. J Appl Physiol. (2016) 120:216–25. doi: 10.1152/japplphysiol.00256.2015
18. Martin DS, Khosravi M, Grocott MP, Mythen MG. Concepts in hypoxia reborn. Crit Care. (2010) 14:315. doi: 10.1186/cc9078
19. Cowburn AS, Macias D, Summers C, Chilvers ER, Johnson RS. Cardiovascular adaptation to hypoxia and the role of peripheral resistance. Elife. (2017) 6:e28755. doi: 10.7554/eLife.28755
20. Umbrello M, Dyson A, Feelisch M, Singer M. The key role of nitric oxide in hypoxia: hypoxic vasodilation and energy supply-demand matching. Antioxid Redox Signal. (2013) 19:1690–710. doi: 10.1089/ars.2012.4979
21. Hudson S, Johnson CD, Marshall JM. Changes in muscle sympathetic nerve activity and vascular responses evoked in the spinotrapezius muscle of the rat by systemic hypoxia. J Physiol. (2011) 589(Pt. 9):2401–14. doi: 10.1113/jphysiol.2010.201814
22. Mirna M, Bimpong-Buta NY, Hoffmann F, Abusamrah T, Knost T, Sander O, et al. Exposure to acute normobaric hypoxia results in adaptions of both the macro- and microcirculatory system. Sci Rep. (2020) 10:20938. doi: 10.1038/s41598-020-77724-5
23. Campagna JA, Carter C. Clinical relevance of the Bezold-Jarisch reflex. Anesthesiology. (2003) 98:1250–60. doi: 10.1097/00000542-200305000-00030
24. Smit B, Smulders YM, Eringa EC, Oudemans-van Straaten HM, Girbes ARJ, Wever KE, et al. Effects of hyperoxia on vascular tone in animal models: systematic review and meta-analysis. Crit Care. (2018) 22:189. doi: 10.1186/s13054-018-2123-9
25. Smit B, Smulders YM, van der Wouden JC, Oudemans-van Straaten HM, Spoelstra-de Man AME. Hemodynamic effects of acute hyperoxia: systematic review and meta-analysis. Crit Care. (2018) 22:45. doi: 10.1186/s13054-018-1968-2
26. Milstein DM, Helmers R, Hackmann S, Belterman CN, van Hulst RA, de Lange J. Sublingual microvascular perfusion is altered during normobaric and hyperbaric hyperoxia. Microvasc Res. (2016) 105:93–102. doi: 10.1016/j.mvr.2016.02.001
27. Jia Y, Li P, Dziennis S, Wang RK. Responses of peripheral blood flow to acute hypoxia and hyperoxia as measured by optical microangiography. PLoS ONE. (2011) 6:e26802. doi: 10.1371/journal.pone.0026802
28. Bertuglia S, Colantuoni A, Coppini G, Intaglietta M. Hypoxia- or hyperoxia-induced changes in arteriolar vasomotion in skeletal muscle microcirculation. Am J Physiol. (1991) 260(2 Pt. 2):H362–72. doi: 10.1152/ajpheart.1991.260.2.H362
29. Orbegozo Cortés D, Puflea F, Donadello K, Taccone FS, Gottin L, Creteur J, et al. Normobaric hyperoxia alters the microcirculation in healthy volunteers. Microvasc Res. (2015) 98:23–8. doi: 10.1016/j.mvr.2014.11.006
30. He X, Su F, Xie K, Taccone FS, Donadello K, Vincent JL. Should hyperoxia be avoided during sepsis? An Experimental Study in Ovine Peritonitis. Crit Care Med. (2017) 45:e1060–7. doi: 10.1097/CCM.0000000000002524
31. Valenzuela Espinoza ED, Pozo MO, Kanoore Edul VS, Furche M, Motta MF, Risso Vazquez A, et al. Effects of short-term hyperoxia on systemic hemodynamics, oxygen transport, and microcirculation: an observational study in patients with septic shock and healthy volunteers. J Crit Care. (2019) 53:62–8. doi: 10.1016/j.jcrc.2019.05.021
32. Aggarwal NR, Brower RG, Hager DN, Thompson BT, Netzer G, Shanholtz C, et al. Oxygen exposure resulting in arterial oxygen tensions above the protocol goal was associated with worse clinical outcomes in acute respiratory distress syndrome. Crit Care Med. (2018) 46:517–24. doi: 10.1097/CCM.0000000000002886
33. Asfar P, Schortgen F, Boisrame'-Helms J, Charpentier J, Guérot E, Megarbane B, et al. Hyperoxia and hypertonic saline in patients with septic shock (HYPERS2S): a two-by-two factorial, multicentre, randomised, clinical trial. Lancet Respir Med. (2017) 5:180–90. doi: 10.1016/S2213-2600(17)30046-2
34. Barrot L, Asfar P, Mauny F, Winiszewski H, Montini F, Badie J, et al. Liberal or conservative oxygen therapy for acute respiratory distress syndrome. N Engl J Med. (2020) 382:999–1008. doi: 10.1056/NEJMoa1916431
35. Schjørring OL, Klitgaard TL, Perner A, Wetterslev J, Lange T, Siegemund M, et al. Lower or higher oxygenation targets for acute hypoxemic respiratory failure. N Engl J Med. (2021) 384:1301–11. doi: 10.1056/NEJMoa2032510
36. Smit B, Smulders YM, Eringa EC, Gelissen HPMM, Girbes ARJ, de Grooth HJS, et al. Hyperoxia does not affect oxygen delivery in healthy volunteers while causing a decrease in sublingual perfusion. Microcirculation. (2018) 25:e12433. doi: 10.1111/micc.12433
37. Ottolenghi S, Sabbatini G, Brizzolari A, Samaja M, Chiumello D. Hyperoxia and oxidative stress in anesthesia and critical care medicine. Minerva Anestesiol. (2020) 86:64–75. doi: 10.23736/S0375-9393.19.13906-5
38. García-Laorden MI, Rodríguez-González R, Martín-Barrasa JL, García-Hernández S, Ramos-Nuez Á, González-García HC, et al. Systemic effects induced by hyperoxia in a preclinical model of intra-abdominal sepsis. Mediators Inflamm. (2020) 2020:5101834. doi: 10.1155/2020/5101834
Keywords: hyperoxia, hypoxia, microcirculation, oxygen, hemodymamics
Citation: Damiani E, Casarotta E, Orlando F, Carsetti A, Scorcella C, Domizi R, Adrario E, Ciucani S, Provinciali M and Donati A (2021) Effects of Normoxia, Hyperoxia, and Mild Hypoxia on Macro-Hemodynamics and the Skeletal Muscle Microcirculation in Anesthetised Rats. Front. Med. 8:672257. doi: 10.3389/fmed.2021.672257
Received: 25 February 2021; Accepted: 13 April 2021;
Published: 11 May 2021.
Edited by:
Inge Bauer, University Hospital of Düsseldorf, GermanyReviewed by:
Andrea Szabó, University of Szeged, HungaryFederico Aletti, Universidade Federal de São Paulo, Brazil
Copyright © 2021 Damiani, Casarotta, Orlando, Carsetti, Scorcella, Domizi, Adrario, Ciucani, Provinciali and Donati. This is an open-access article distributed under the terms of the Creative Commons Attribution License (CC BY). The use, distribution or reproduction in other forums is permitted, provided the original author(s) and the copyright owner(s) are credited and that the original publication in this journal is cited, in accordance with accepted academic practice. No use, distribution or reproduction is permitted which does not comply with these terms.
*Correspondence: Elisa Damiani, ZWxpLmRhbTk4NkBnbWFpbC5jb20=