- 1Department of Precision and Translational Medicine, Sapienza University of Rome, Rome, Italy
- 2Department of Movement, Human and Health Sciences, Division of Health Sciences, University of Rome “Foro Italico,” Rome, Italy
- 3Department of Anatomical, Histological, Forensic Medicine and Orthopedic Sciences, Sapienza University of Rome, Rome, Italy
- 4Department of Medico-Surgical Sciences and Biotechnologies, Sapienza University of Rome, Latina, Italy
In normal human livers, EpCAMpos cells are mostly restricted in two distinct niches, which are (i) the bile ductules and (ii) the mucous glands present inside the wall of large intrahepatic bile ducts (the so-called peribiliary glands). These EpCAMpos cell niches have been proven to harbor stem/progenitor cells with great importance in liver and biliary tree regeneration and in the pathophysiology of human diseases. The EpCAMpos progenitor cells within bile ductules are engaged in driving regenerative processes in chronic diseases affecting hepatocytes or interlobular bile ducts. The EpCAMpos population within peribiliary glands is activated when regenerative needs are finalized to repair large intra- or extra-hepatic bile ducts affected by chronic pathologies, including primary sclerosing cholangitis and ischemia-induced cholangiopathies after orthotopic liver transplantation. Finally, the presence of distinct EpCAMpos cell populations may explain the histological and molecular heterogeneity characterizing cholangiocarcinoma, based on the concept of multiple candidate cells of origin. This review aimed to describe the precise anatomical distribution of EpCAMpos populations within the liver and the biliary tree and to discuss their contribution in the pathophysiology of human liver diseases, as well as their potential role in regenerative medicine of the liver.
Introduction
In the adult liver and biliary tree, mature parenchymal cells (i.e., hepatocytes and cholangiocytes) are characterized by remarkable proliferative capabilities, which support the regenerative needs of these organs in physiological conditions (1). According to that, lineage tracing studies indicated that hepatocytes are able to restore hepatic parenchyma after subcritical liver resection and regenerate cell loss after acute and chronic damage; furthermore, studies on cell plasticity in rodents indicated the potential of hepatocytes to differentiate toward biliary cells after injury (1–5). Therefore, conflicting evidence is present in scientific literature regarding the existence and function of a distinct stem/progenitor cell population capable of participating in liver regeneration (6). Nonetheless, experimental models characterized by an extensive (or prolonged over time) liver injury, which mimic the natural history of human liver diseases, demonstrated the contribution of stem/progenitor cells in the regenerative response (7, 8).
Recent single cell transcriptomic studies turned the spotlight on the EpCAMpos cell fraction within the liver as a candidate progenitor cell population (9, 10). The epithelial cell adhesion molecule (EpCAM) is a surface epithelial marker selectively expressed in the biliary tree, where it individuates the stem/progenitor cell fraction from mature cholangiocytes (1, 11); differently, mature hepatocytes within the hepatic lobule do not express EpCAM (11). The elegant single-cell transcriptomic atlas compiled by Aizarani et al. produced a comprehensive depiction of liver cell populations, including hepatocytes, biliary epithelial cells, endothelial cells, macrophages, and inflammatory cells (9). The authors revealed the presence of transcriptomic heterogeneity in the EpCAMpos population and, within this population, identified the cell fraction with the highest potential to form liver organoids and to putatively serve as a stem cell compartment. In parallel, the study by Segal et al. individuated, by using a similar approach, a unique gene expression profile within the EpCAMpos cell population in human fetal livers: in particular, they individuated a distinct EpCAMpos cell fraction which has bi-potential capability, is distinguishable from hepatocytes and cholangiocytes, and persists in the adult organ (10). Besides some discrepancies in cell phenotype, both studies individuated the EpCAMpos population within the intrahepatic biliary tree as a strong candidate stem/progenitor cell compartment with regenerative potential for the liver and biliary tree.
The present review aimed to discuss the phenotype and the precise anatomical distribution of EpCAMpos cell populations within the liver and the biliary tree, to describe their changes induced by human liver diseases, and to highlight their role as a potential source for regenerative medicine.
The Heterogeneity of EpCAMpos Cell Populations in Human Liver
In human livers, EpCAMpos cells are localized and organized in two distinct anatomical niches (Figure 1), namely, bile ductules and peribiliary glands (PBGs).
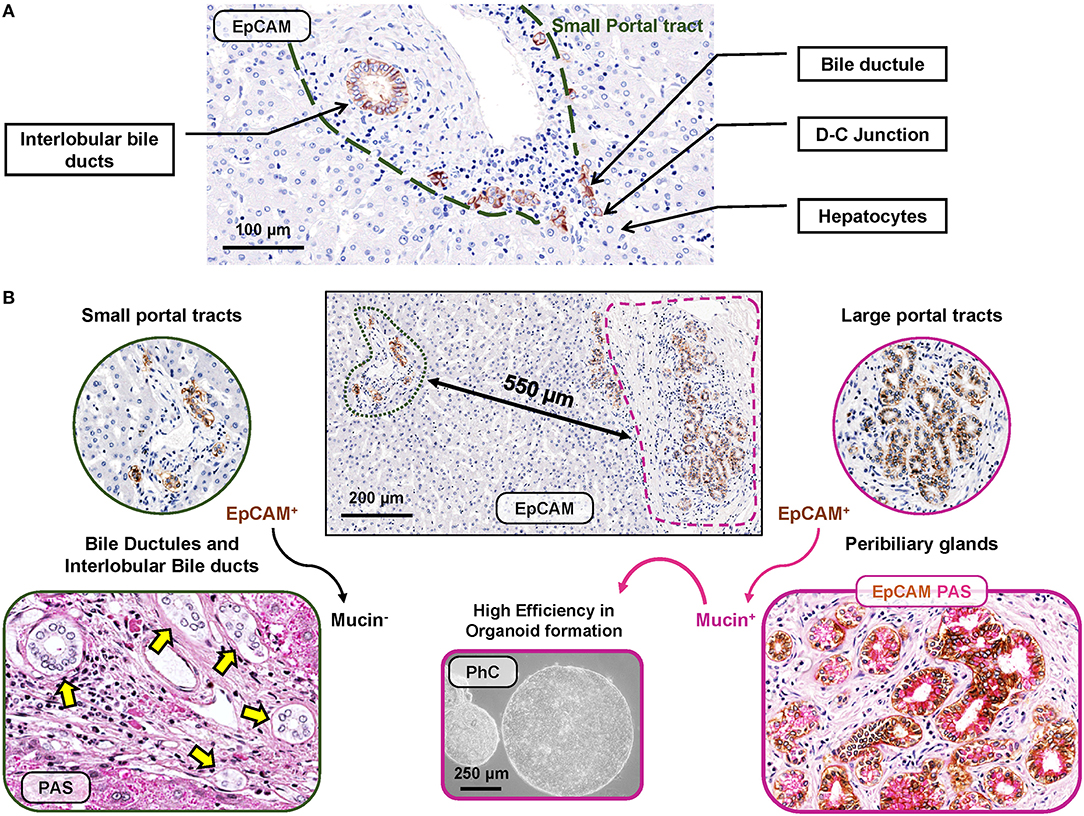
Figure 1. EpCAMpos cell niches in human liver fragments obtained from normal subjects. (A) EpCAMpos cells are present in small portal tracts and are endowed in interlobular bile ducts and bile ductules up to the ductular-canalicular (D-C) junction. Hepatocytes are constantly negative for EpCAM. (B) Large portal tracts can be present together with small ones in the same liver fragment, given their close proximity in sectional histology. Large intrahepatic bile ducts (i.e., segmental and area bile ducts) contain an EpCAMpos cell niche endowed in the mucous glands present inside their walls (so-called peribiliary glands). Mucin positivity is typically present in EpCAMpos peribiliary gland cells (on the right) but not in bile ductules and interlobular bile ducts at small portal tracts (on the left). Once isolates from peribiliary glands, EpCAMpos cells showed progenitor cell features, including high efficiency in organoid formation in vitro. Histologic images are representatives of human liver fragments obtained from liver donors (N = 5). Specimens were stained by immunohistochemistry for EpCAM and periodic acid-Schiff (PAS) for mucins. EpCAM immunohistochemistry is counterstained with hematoxylin or with PAS. Organoids were generated by EpCAMpos peribiliary gland cells isolated from the human common hepatic duct obtained from organ donors (routinely discarded in orthotopic liver transplantation procedures); the phase contrast (PhC) microscopic image is representative of at least N = 3 biological replicates.
The intrahepatic biliary tree begins with the canals of Hering, which represent the point of junction between the hepatocyte canalicular system and the biliary tree (12, 13). The canals of Hering are located at the interface between the portal tract and the hepatic parenchyma and continue into the bile ductules, with tortuous conduits draining into the interlobular bile ducts inside the portal space. A population of EpCAMpos cells has been identified within the canals of Hering and the bile ductules, serving as facultative bipotent progenitors (Hepatic Stem/progenitor Cells: HpSCs) capable to differentiate into hepatocytes and cholangiocytes (Figure 2, panel A) (9, 14, 15), and represents the remnant of the ductal plate in the adult liver (10, 16, 17). Morphologically, HpSCs are small cells characterized by a high nucleus-to-cytoplasm ratio and expressing a large variety of markers, which include stem cell markers [e.g., EpCAM, neural cell adhesion molecule (NCAM), transcription factor Sox9, CD44, and CD133], biliary cytokeratins (CK7/19), and hepatocellular traits (e.g., albumin, CK18, hepatocyte nuclear factor 4 alpha) (10, 18). While differentiating toward a mature fate, the progeny of HpSCs is characterized by the progressive loss of EpCAM and NCAM expression and the acquirement of mature hepatocyte or cholangiocyte traits (10, 14, 19, 20). Recently, this EpCAMpos cellular population has been further characterized by single-cell transcriptomic, with the identification of an EpCAMpos/NCAMpos fraction which also displays prominent stem cell features in vitro (10).
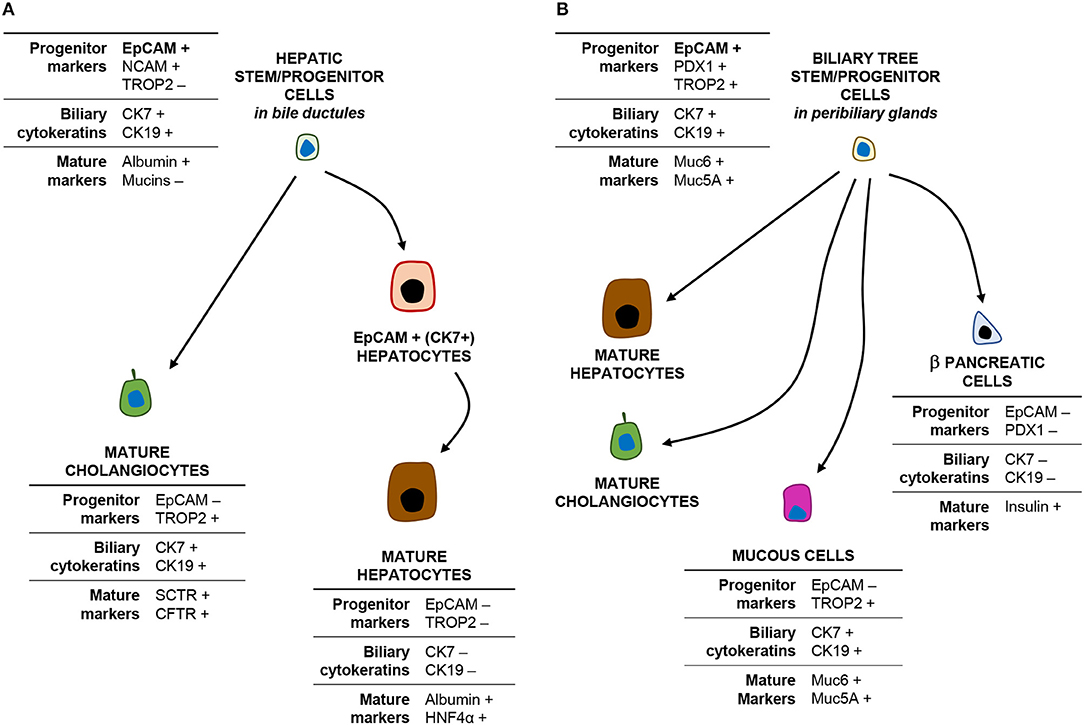
Figure 2. EpCAMpos cells within the liver and biliary tree and their progeny. The cartoon shows the phenotype (main markers) of EpCAMpos stem/progenitor cells within the bile ductules (A) and the peribiliary glands (B) and their differentiative capabilities.
Notably, a second EpCAMpos cell niche is endowed in PBGs located inside the walls of large (i.e., segmental and area) intrahepatic bile ducts and along the entire extrahepatic biliary tree (21). PBGs are tubulo-alveolar mucous glands, in continuity with the surface epithelium of the bile duct (22, 23). Intriguingly, the EpCAMpos cell population within PBGs showed stem/progenitor properties, including organoid formation and plasticity to differentiate into hepatocytes, cholangiocytes, and endocrine pancreatic cells (Figure 2, panel B) (21, 24, 25). PBG cells have been collectively named biliary tree stem/progenitor cells (BTSC) and, embryologically, they represent the remnant of the common bilio-pancreatic progenitors of the ventral endoderm (23, 24). Phenotypically, mucin family genes (e.g., MUC5A and MUC6) are largely expressed in this anatomical niche (23); moreover, the EpCAMpos cell pool within PBGs shows a heterogeneous profile and a radial axis (depth-to-surface) organization: EpCAMpos cells are mostly found at the bottom of PBGs, where a subpopulation of them co-expresses markers of pluripotency (i.e., Oct4, Sox2, and Nanog) (23); a transit-amplifying (i.e., proliferating) population is located in the middle portion of the glands; finally, cells with a more mature phenotype [e.g., expressing secretin receptor, cystic fibrosis transmembrane conductance receptor (CFTR), mucins, and Trop2] are located in direct continuity with the surface epithelium (23, 26, 27). Altogether, these findings are confirmed by single-cell transcriptomic analysis in human, with the identification of an EpCAMpos/TROP2int/MUC6high progenitor compartment with prominent stem cell features (9). Moreover, the contribution of PBG cells in the regeneration of mature biliary epithelium has been demonstrated in experimental conditions and by an ex vivo human model of biliary regeneration, which have disclosed that PBG cells can repopulate the surface epithelium of bile ducts by proliferation and differentiation into mature cholangiocytes (25, 28, 29).
In the light of these findings, one of the main aspects to be considered when interpreting transcriptomic analysis of liver samples resides in the fact that PBGs and bile ductules are two anatomically distinct compartments which, however, can be found in strict spatial proximity within the same liver fragment (Figure 1, panel B). Thus, the transcriptomic heterogeneity in the EpCAMpos population revealed by single cell approaches could be due to the collection of one or both of these distinct progenitor cell compartments from the same specimen. EpCAMpos progenitor cells within bile ductules are characterized by a distinct signature identifying them from mature cholangiocytes, immature hepatocytes, and mature hepatocytes, based on NCAMpos/TROP2neg expression (Figure 2). Therefore, these cells could be distinguished from cholangiocytes that populate interlobular bile ducts, the latter expressing TROP2 and mucin family genes. However, a TROPint cell fraction among the EpCAMpos compartment was proven to have high stemness properties; thus, this fraction resides more likely into peribiliary glands than in bile ductules, given also its high mucin family gene expression (e.g., MUC5A and MUC6) and low CK19 and CFTR levels (25, 30).
Understanding the phenotypes of distinct biliary populations and their anatomical niches is crucial when considering that the pathophysiology of hepatic and biliary diseases is often hindered by difficulties in identifying regenerative pathways. Interestingly, the EpCAMpos population exhibited only a stochastic expression of proliferation markers (9). This finding strengthens the concept of a facultative progenitor compartment that is only engaged by the regenerative needs and trajectories required after prolonged chronic or acute massive damage (8).
EpCAMpos Cell Populations in Chronic Human Diseases of the Liver and Biliary Tree
In human liver diseases, the phenotypic and transcriptomic profiles of liver cells change noticeably. In this context, taking into account the heterogeneity within the EpCAMpos progenitor population is a crucial prerequisite to understand the specific contribution of different hepatic regenerative pathways in human disease progression. Chronic prolonged diseases affecting hepatocytes or interlobular bile ducts could engage HpSCs within bile ductules (Figure 3, panel A) (19); differently, PBGs and their EpCAMpos cell fraction (i.e., BTSCs) could be activated when the disease leads to the damage of large intrahepatic (or extra-hepatic) bile ducts (Figure 3, panel B) (30, 31).
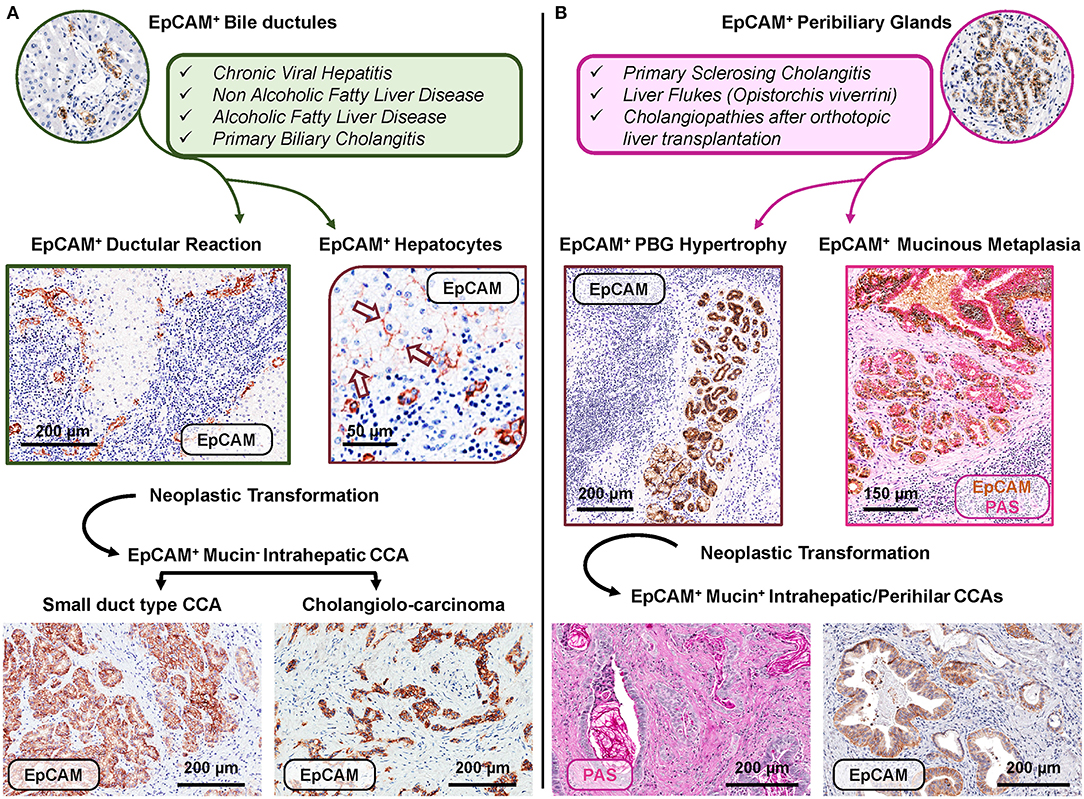
Figure 3. Distinct EpCAMpos cell niches are engaged by specific human diseases based on regenerative needs and may represent the cell of origin of specific subtypes of cholangiocarcinoma. (A) Regenerative pathways engage EpCAMpos/MUC6neg progenitors within bile ductules in chronic human diseases affecting hepatocytes and/or interlobular bile ducts. In these diseases, an expansion of the EpCAMpos cell population is present and includes the emergence of ductular reaction and the appearance of EpCAMpos hepatocytes (arrows). Two subtypes of EpCAMpos cholangiocarcinoma (CCA) may derive from the neoplastic transformation of EpCAMpos interlobular cholangiocytes (small duct type CCA) and bile ductules (cholangiolo-carcinoma). (B) The EpCAMpos/MUC6pos population within peribiliary glands (PBGs) could be engaged when regenerative needs are finalized to repair large intra- or extrahepatic bile ducts affected by chronic pathologies, including primary sclerosing cholangitis. The EpCAMpos PBG cell population response is characterized by hypertrophy and mucinous metaplasia of this compartment. The onset of mucinous CCAs could be due to the neoplastic transformation of the EpCAMpos PBG niche, through the sequence hypertrophy-metaplasia-dysplasia-cancer. Histologic images in (A) are representative of human liver fragments obtained from patients affected by chronic viral hepatitis, primary biliary cholangitis, and CCA. In (B), specimens were obtained from patients affected by primary sclerosing cholangitis and CCA. Specimens were stained by immunohistochemistry for EpCAM and periodic acid-Schiff (PAS) for mucins. EpCAM immunohistochemistry is counterstained with hematoxylin or with PAS.
EpCAMpos Cell Fraction in Bile Ductules: Ductular Reaction and Chronic Liver Diseases
In a number of different chronic liver diseases, EpCAMpos HpSCs within the canals of Hering and the bile ductules represent the source of a prominent ductular reaction (DR) (Figure 3, panel A) (19, 32). The phenotype and the behavior of EpCAMpos DR have been extensively characterized in liver diseases affecting hepatocytes (19). In these conditions, DR appearance is associated with the emergence of a unique EpCAMpos hepatocyte population, which localizes periportally and is characterized by the co-expression of biliary cytokeratins (11, 19, 33). Detailed studies of liver samples obtained from cirrhotic patients allowed accurately describing the expansion of DR and the emergence of an EpCAMpos hepatocyte population, able to regenerate large portions of liver parenchyma (34, 35).
In chronic liver diseases, the expansion of DR is secondary to the prolonged exposure of hepatocytes to pathogenetic insults, which leads to the progressive loss of hepatocyte replicative capability and induces hepatocellular senescence. For example, in non-alcoholic fatty liver disease (NAFLD), hepatocyte regenerative capabilities are highly impaired due to progressive lipotoxicity and lobular inflammation (36, 37); this induces a prominent EpCAMpos DR, followed by the appearance of EpCAMpos hepatocytes at the periportal zone (37–42). In NAFLD, DR correlates with disease activity and NASH onset; interestingly, DR extent also correlates with periportal fibrogenesis and is predictive of fibrosis stage (43, 44). Parallel studies confirmed this role of DR also in pediatric subjects affected by NASH (39, 45). Moreover, DR is associated with systemic oxidative stress levels (38) and extra-hepatic clinical manifestations such as obstructive sleep apnea syndrome (46).
Extensive EpCAMpos DR can also be triggered by a severe hepatocyte loss as a consequence of acute liver injury. In alcoholic hepatitis, a relevant cause of morbidity and mortality in heavy drinkers with alcoholic liver disease (47), DR extension is correlated with the severity of the damage and can predict short-term mortality (47, 48). In this setting, patients responding to first-line steroid therapy were characterized by higher DR (49); differently, in non-responders, cells within DR do not show signs of differentiation into hepatocytes, which correlates with a less favorable outcome (19, 50–53).
A prominent EpCAMpos DR also appears in chronic diseases affecting the biliary tree (i.e., cholangiopathies) and acquires a peculiar phenotype, which reflects the pathophysiology of the disease. Primary biliary cholangitis (PBC) is a chronic, autoimmune cholangiopathy, characterized by the damage of interlobular bile ducts, leading to impaired bile duct flow into the biliary tree (54). In patients affected by PBC, DR is composed of cells expressing stem cell markers (EpCAM, NCAM, Sox9, and CD133) and displaying a variable degree of cholangiocyte differentiation signs, together with Notch pathway activation (19, 33, 55, 56). Disease severity and stage are correlated with the extent of DR, which might serve as prognostic marker for the development of clinical symptoms, patient prognosis, and response to therapy (55, 57). Differently from PBC, patients affected by primary sclerosing cholangitis (PSC) show a unique DR phenotype. In PSC, chronic inflammatory damage targets large intrahepatic bile ducts and mostly spares interlobular bile ducts; the development of fibrotic strictures and consequent cholestatic injury of the liver parenchyma trigger DR which, however, is less prominent compared to PBC and is characterized by the expression of hepatocellular traits, with the appearance of numerous EpCAMpos hepatocytes (55).
In summary, liver diseases induce a substantial modification of the EpCAMpos fraction within the liver; these changes are strongly influenced by the etiology of liver diseases and the specific regenerative needs. Remarkably, liver diseases further re-shape the non-parenchymal cell fractions (i.e., macrophages and hepatic stellate cells), inducing an inflammatory and fibrogenetic response (Table 1) (37, 40). In turn, these changes influence the EpCAMpos cell compartment and support the vicious cycle which promotes and sustains liver fibrosis (58, 59). This represents the rationale for the strong correlation between EpCAMpos fraction activation and clinical outcomes in human diseases (40, 57).
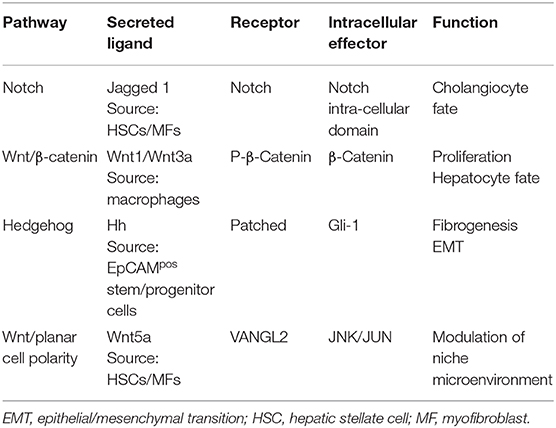
Table 1. Summary of the main signaling pathways involved in EpCAMpos niches of the liver and biliary tree.
EpCAMpos Cells Within PBGs in Human Cholangiopathies
The EpCAMpos cell population in PBGs is implicated in human diseases affecting large intrahepatic and extrahepatic bile ducts (Figure 3, panel B) (1). Indeed, in PSC samples, progressive hyperplasia, and mucinous metaplasia of PBGs characterize fibrotic large bile ducts. Hyperplasia of PBGs is determined by the expansion of BTSCs, which also contributes to biliary fibrosis trough epithelial-to-mesenchymal transition and is sustained by the signaling pathway mediated by hedgehog ligands (Table 1) (30, 55). Interestingly, in PSC patients, the expansion of PBG mass correlated with the severity of the histological stage of liver cirrhosis and with the clinical stages according the Mayo score (30). It is worth noting that the histopathology of large bile duct fibrosis (which is at the basis of biliary strictures development in PSC) was understudied for years, although a consensus on PSC animal models highlighted the need to study large bile ducts and associated PBGs (60). In PSC and in pathologies of large bile ducts and in extrahepatic cholestasis, indeed, inflammation mostly spares the interlobular bile ducts within the liver parenchyma, whereas ductular reaction and fibrosis are present (55). These observations indicate that parenchymal injury in PSC is the consequence of ascending cholestasis. Differently, in PBC patients, the histomorphological study of bile ducts and livers demonstrated no activation of the PBG niche, thus indicating that the EpCAMpos BTSCs endowed in large intrahepatic and extrahepatic bile ducts are elicited by the injury of this portion of the biliary tree (as in PSC) but not in diseases affecting occurring interlobular bile ducts (i.e., PBC) (30).
Other than PSC, PBGs are involved in the turnover and regeneration of biliary epithelia in sclerosing reactions in secondary sclerosing cholangitis and hepatolithiasis (22, 61). Cystic changes in PBGs can occur de novo, as part of a congenital syndrome, or secondarily to insults such as alcoholic cirrhosis (61). Interestingly, the systematic examination of bile ducts of liver grafts revealed the relationship between the degree of ischemic-based PBG injuries and the future development of non-anastomotic strictures (28, 62). Moreover, using an ex vivo model based on precision-cut slices of extrahepatic human bile ducts obtained from discarded donor livers, the spatiotemporal differentiation and migration of PBG cells after severe biliary injury was recently studied; this approach revealed that human PBGs contain biliary progenitor cells and are able to respond to bile duct epithelial loss with proliferation, differentiation, and maturation to restore epithelial integrity, providing evidence for a pivotal role of PBGs in biliary regeneration after severe injury (28).
Although normal PBGs themselves are particularly small structures that cannot be recognized using any of the currently available imaging modalities, these glands are closely associated with several diseases which have typical imaging features. The knowledge of the basic pathophysiology of PBGs could be helpful for depicting innovative diagnostic modalities and endpoints in biliary diseases associated with PBG injury (61). Since human BTSC activation in fibrotic large duct pathologies is associated with PBGs hyperplasia/metaplasia and dysplasia, in the future, histomorphology and radiology correlation studies are needed in order to envision innovative PBG imaging tools.
Mechanisms underlying the repair of extrahepatic biliary tree after injury have been scarcely explored. We have recently shown that the Wnt signaling pathway triggers human BTSC proliferation in vitro and influences PBG hyperplasia in vivo in a mouse biliary injury model (25). In particular, Notch signaling pathway activation induces BTSC differentiation in vitro toward mature cholangiocytes, in parallel with the observation of Notch pathway activation within PBGs in a mouse model; moreover, in human PSC, inflammatory and stromal cells trigger PBG activation through the upregulation of the Wnt and Notch signaling pathways (25). Considering these findings, the demonstration of the involvement of PBG cells in regenerating the injured biliary epithelium and identifying the signaling pathways driving BTSC activation could have relevant implications on the treatment of cholangiopathies.
The EpCAMpos (MUC6high) cell population may even respond to systemic stimuli, like hyperglycemia. In experimental and human diabetes, EpCAMpos/MUC6high cell population in PBGs respond to diabetes with proliferation and differentiation toward insulin-producing cells, indicating that PBG niches may rescue pancreatic islet impairment in diabetes (63). These findings offer important implications for the pathophysiology and complications of this disease, comprising the understanding of the paramount role of type II diabetes in cholangiocarcinoma risk nowadays (see the next paragraph) (63).
EpCAMpos Cell Populations in Cholangiocarcinoma
The recent focus on the EpCAMpos/MUC6high cell population residing in large intrahepatic and extrahepatic bile ducts sheds light on the clinical management of malignancies arising in this organ, which are collectively termed cholangiocarcinoma (30, 31). From an anatomical point of view, cholangiocarcinoma can be classified as intrahepatic, perihilar, or distal (64); however, growing evidence suggests that distinct cells of origin within an organ, particularly tissue-specific stem cells, may give rise to different cancer subtypes (Figure 3) (65). In this context, certain subtypes of intrahepatic cholangiocarcinoma (small bile duct type and cholangiolo-carcinoma) are constituted by mucinneg cells and could originate from small cholangiocytes or from the EpCAMpos/MUC6neg population located in ductules and/or interlobular bile ducts. In keeping, chronic liver diseases and cirrhosis represent specific risk factors for these tumor subtypes, and the surrounding parenchyma is generally characterized by a marked EpCAMpos/mucinneg DR (40, 57). The division rate of stem cells is linearly correlated with the lifetime risk of developing primary liver cancer (66).
Intriguingly, mucinous intrahepatic (large bile duct type) and perihilar cholangiocarcinoma show a similar phenotype, with mucinpos cells occupying the entire neoplastic mass, and are characterized by a similar clinical course (67); such tumors could arise from PBGs and, likely, from the EpCAMpos/TROP2int/MUC6high compartment (31). PSC and liver flukes represent well-recognized risk factors for these tumor subtypes (67); these diseases chronically affect large intrahepatic and extrahepatic bile ducts and determine hyperplasia, mucinous metaplasia, and dysplasia in PBGs (30). The expansion of the EpCAMpos/MUC6high compartment within PBGs represents an attempt to restore epithelial integrity as a response to chronic bile duct damage (25). However, the continuous exposure to harmful stimuli leads to a progressively uncontrolled response, and dysplasia-to-cancer progression takes place diffusely within bile ducts with multiple neoplastic foci, mimicking field cancerization (31).
The inter-tumor heterogeneity of cholangiocarcinoma might be due to the interplay of distinct tissues/cells of origin, the underlying disease, and the associated molecular clustering based on driver mutations which shape the pathobiological features of the different cholangiocarcinoma subtypes. In keeping with that, intrahepatic cholangiocarcinoma can be distinguished into two main subtypes (small and large bile duct type) based on its origin and histomorphologic features. Remarkably, these tumor subtypes have distinct cancer stem cell profiles (68), thus suggesting a putative different cell of origin (HpSCs vs. BTSCs). These subtypes also differ in terms of gross growth pattern, genetic signatures, and driver mutations (e.g., IDH1/2 in small bile duct type and KRAS in large bile duct type), and prognosis. With this latter regard, the large bile duct cholangiocarcinoma subtype is a highly aggressive cancer, characterized by a low disease-free and overall survival. This tumor typically arises in patients with PSC and has been proved to derive from dysplastic EpCAMpos PBGs both in humans (31) and in experimental models (69).
Emphasizing the role of the different tissues/cells of origin on the complex pathogenesis of cholangiocarcinoma, also in the picture of EpCAMpos cell niches, could have implications on preventive strategies and early diagnosis in patients with underlying clinical or subclinical hepatobiliary disease, shedding new light on dissecting cholangiocarcinoma heterogeneity and allowing a rational approach to personalized medicine for this devastating cancer (67, 70).
Perspectives and Applications of EpCAMpos Cells in Regenerative Medicine
Orthotopic liver transplantation represents the main curative option for the majority of chronic liver diseases. In this context, the shortage of organ donors represents a severe limitation to patients' treatment, which results in many patients dying while waiting for transplantation. For these patients, the development of effective strategies for cell therapy could represent a promising approach to the treatment of liver diseases, also as a bridge to liver transplantation.
Therefore, different strategies of cell therapy have been attempted (71, 72). Hepatocyte transplantation represents the proof of concept of liver cell therapy but it is limited by the scarcity of donor organs, the low cell engraftment, difficulties in cryopreservation, and the necessity of long-term immunosuppression (72–74). Mesenchymal-derived stem cells, comprising hematopoietic stem cells and mesenchymal stem cells, have been largely applied for liver regenerative medicine purposes in a number of clinical trials throughout the world (71, 75–79). Although autologous transplantation of hematopoietic and mesenchymal stem cells is clinically safe, the recent negative results of randomized clinical trials limit the future application of this strategy (75–79). Clinical applications of pluripotent stem cells in hepatology are actually null; this is due to the teratocarcinogenic risk and the ethical concerns for the use of embryonic stem cells and to the possibility of tumorigenic expansion of reprogrammed induced pluripotent stem cells (72).
In this scenario, the hepatic EpCAMpos cell fractions could represent an important alternative source, currently under investigation worldwide. Other than case reports concerning the use of EpCAMpos HpSCs in biliary atresia and inborn errors of metabolism (80, 81), a non-randomized clinical trial of 25 subjects and 25 controls with decompensated liver cirrhosis due to various causes undergoing human fetal EpCAMpos HpSCs infusion into the liver via the hepatic artery has been reported (82). At 6-months follow-up, multiple diagnostic and biochemical parameters showed improvement, with a significant decrease in the patients' MELD (model for end-stage liver disease) scores (82). In western countries, Pietrosi et al. performed an intrasplenic infusion of a total cell population obtained from the fetal liver in nine patients affected by end-stage liver disease, demonstrating that the procedure was safe and well-tolerated in all patients (83). Similar to HpSCs, EpCAMpos BTSCs could represent a possible source for stem/progenitor cells for liver regenerative medicine, as they are readily available from the biliary tree of adult or fetal organ donors and from cholecystectomized patients (84–87). In the past, we reported a preliminary experience in treating patients with advanced cirrhosis by the infusion via hepatic artery of human EpCAMpos BTSCs (84). This report represents the proof of concept that EpCAMpos BTSCs are a suitable source for the cell therapy of liver cirrhosis and represents the basis for an ongoing controlled clinical trial (84). Moreover, cells are routinely isolated in good manufacturing practices (GMP) conditions, and all required media are available in GMP-grade (84). Finally, the EpCAM-based sorting procedure is highly standardized and has been already used in clinical program (11, 84, 88, 89).
3D organoids represent an advanced culture technology in the field of stem cells and regenerative medicine, recapitulating embryonic development and the physiology of the tissue of origin. So far, organoid cultures have been developed for the adult intestine (90), stomach (91), and pancreas (92) of different species, like mouse and human. When cultured as organoids, cells can expand long term in culture, maintain the genetic stability, and spontaneously self-organize into structures that resemble the in vivo tissue in terms of cellular composition and function.
Organoid techniques have been largely applied to human liver, in particular to its EpCAMpos stem/progenitor compartments. Under defined culture conditions, EpCAMpos HpSCs from adult liver can be expanded for months (>1 year) in culture, showing an exponential rate of proliferation and genetic stability (92). HpSCs can readily be expanded as bipotent stem cells into 3D organoids and differentiate into functional hepatocyte cells both in vitro and in vivo upon transplantation (93, 94). Noteworthily, single isolated EpCAMpos cells develop into organoid with high colony forming efficiency, while EpCAMneg cells failed to create organoids (93). Moreover, the engraftment and differentiation of organoids was demonstrated in mouse models of acute liver injury; in these mice, a stable expression of human hepatocyte markers was observed in recipient serum for more than 4 months, and cell engraftment was confirmed by immunohistochemistry for human albumin (93).
The application of the organoid technique is feasible to obtain long-term expansion of BTSCs, maintaining stem cell markers and genetic stability over months (24, 87, 95). Interestingly, Sampaziotis et al. have demonstrated that cells isolated from the extrahepatic biliary tree can be expanded in vitro in long-term 3D organoid culture, while maintaining the biliary transcriptional signature and functional characteristics; this culture can be propagated as a potential system for the application of regenerative medicine in the field of cholangiopathies and in vitro common bile duct disease modeling (96).
Overall, organoid cultures derived from patients' liver could represent a relevant system to study human biology, physiology, and the regeneration and development of the liver and biliary tree (97). In addition, 3D organoid cultures enable the study of molecular mechanism driving liver diseases, comprising cholangiopathies (98) and primary liver cancers including cholangiocarcinoma (99); at the same time, this technique can provide potential tools to manipulating genomes and facilitate gene correction for regenerative medicine and autologous therapy (97). Recently, a specific interest has increased for the genetic modification of liver organoids to create disease-specific models in settings where providing a patient's material is challenging (100).
Liver organoids facilitate the study of human liver diseases and regeneration and open the way to expand human liver cells in vitro in the field of personalized medicine or drug testing for biliary and liver disease. The effective application of organoid-derived hepatocytes and cholangiocytes to humans needs to face important technical issues related to the in vitro manipulation and the large use of non-clinical-grade GMP substances necessary to promote and sustain long-term 3D growth.
Conclusion
The liver and the biliary tree harbor two EpCAMpos stem/progenitor cell niches, both yielding high regenerative capabilities and the potential to differentiate into mature hepatocytes and cholangiocytes, but displaying distinct localization and phenotype and peculiar behavior and role in the physio-pathogenesis of liver and biliary diseases.
EpCAMpos HpSCs are located at the canals of Hering and in the bile ductules; these cells are recruited in human acute and chronic liver diseases affecting hepatocytes, contributing to liver regeneration and being implicated in the fibrogenetic processes. HpSCs are also elicited in cholangiopathies affecting interlobular bile ducts (i.e., primary biliary cholangitis). EpCAMpos BTSCs can be found in the PBGs of large intrahepatic or extrahepatic bile ducts and participate in regenerative responses following cholangiopathies affecting large ducts. Their activation is key in the pathogenesis of biliary strictures in primary sclerosing cholangitis, and their injury is implicated in the onset of non-anastomotic strictures following orthotropic liver transplantation.
Both niches could contribute to the development of cholangiocarcinoma due to their prominent activation occurring in pre-neoplastic conditions, and their distinct phenotype could explain, at least in part, the heterogeneity observed in cholangiocarcinoma subtypes.
Finally, due to their stem cell properties and safety of use, they represent a valid cell source for regenerative medicine of the liver.
Author Contributions
SS: study concept, design, and drafting of the manuscript. GC: study concept, design, drafting of the manuscript, figure preparation, and study supervision. DO, AF, and VC acquisition of images, figure preparation, drafting of the manuscript, and critical revision of the manuscript for important intellectual content. PO, DA, and EG critical revision of the manuscript for important intellectual content, obtained funding, and study supervision. All authors contributed to the article and approved the submitted version.
Funding
This study was supported by research project grant from Sapienza University of Rome (EG, PO) and by a sponsored research agreement (SRAs) from Vesta Therapeutics (Bethesda, MD).
Conflict of Interest
The authors declare that the research was conducted in the absence of any commercial or financial relationships that could be construed as a potential conflict of interest.
Abbreviations
BTSC, biliary tree stem/progenitor cell; CD, cluster differentiation; CFTR, cystic fibrosis transmembrane conductance receptor; CK, cytokeratin; DR, ductular reaction; EpCAM, epithelial cell adhesion molecule; GMP, good manufacturing practice; HpSC, hepatic stem/progenitor cell; MUC, mucin; NAFLD, non-alcoholic fatty liver disease; NCAM, neural cell adhesion molecule; PBC, primary biliary cholangitis; PBG, peribiliary gland; PSC, primary sclerosing cholangitis.
References
1. Lanzoni G, Cardinale V, Carpino G. The hepatic, biliary, and pancreatic network of stem/progenitor cell niches in humans: a new reference frame for disease and regeneration. Hepatology. (2016) 64:277–86. doi: 10.1002/hep.28326
2. Malato Y, Naqvi S, Schurmann N, Ng R, Wang B, Zape J, et al. Fate tracing of mature hepatocytes in mouse liver homeostasis and regeneration. J Clin Invest. (2011) 121:4850–60. doi: 10.1172/JCI59261
3. Yanger K, Knigin D, Zong Y, Maggs L, Gu G, Akiyama H, et al. Adult hepatocytes are generated by self-duplication rather than stem cell differentiation. Cell Stem Cell. (2014) 15:340–9. doi: 10.1016/j.stem.2014.06.003
4. Chen F, Jimenez RJ, Sharma K, Luu HY, Hsu BY, Ravindranathan A, et al. Broad distribution of hepatocyte proliferation in liver homeostasis and regeneration. Cell Stem Cell. (2020) 26:27–33 e4. doi: 10.1016/j.stem.2019.11.001
5. Matsumoto T, Wakefield L, Tarlow BD, Grompe M. In vivo lineage tracing of polyploid hepatocytes reveals extensive proliferation during liver regeneration. Cell Stem Cell. (2020) 26:34–47 e3. doi: 10.1016/j.stem.2019.11.014
6. Aleksieva N, Forbes SJ. Biliary-derived hepatocytes in chronic liver injury: bringing new troops to the battlefield? J Hepatol. (2019) 70:1051–3. doi: 10.1016/j.jhep.2019.03.015
7. Choi TY, Ninov N, Stainier DY, Shin D. Extensive conversion of hepatic biliary epithelial cells to hepatocytes after near total loss of hepatocytes in zebrafish. Gastroenterology. (2014) 146:776–88. doi: 10.1053/j.gastro.2013.10.019
8. Russell JO, Lu WY, Okabe H, Abrams M, Oertel M, Poddar M, et al. Hepatocyte-specific beta-catenin deletion during severe liver injury provokes cholangiocytes to differentiate into hepatocytes. Hepatology. (2019) 69:742–59. doi: 10.1002/hep.30270
9. Aizarani N, Saviano A, Sagar, Mailly L, Durand S, Herman JS, et al. A human liver cell atlas reveals heterogeneity and epithelial progenitors. Nature. (2019) 572:199–204. doi: 10.1038/s41586-019-1373-2
10. Segal JM, Kent D, Wesche DJ, Ng SS, Serra M, Oules B, et al. Single cell analysis of human foetal liver captures the transcriptional profile of hepatobiliary hybrid progenitors. Nat Commun. (2019) 10:3350. doi: 10.1038/s41467-019-11266-x
11. Yoon SM, Gerasimidou D, Kuwahara R, Hytiroglou P, Yoo JE, Park YN, et al. Epithelial cell adhesion molecule (EpCAM) marks hepatocytes newly derived from stem/progenitor cells in humans. Hepatology. (2011) 53:964–73. doi: 10.1002/hep.24122
12. Gaudio E, Carpino G, Cardinale V, Franchitto A, Onori P, Alvaro D. New insights into liver stem cells. Dig Liver Dis. (2009) 41:455–62. doi: 10.1016/j.dld.2009.03.009
13. Alvaro D, Gaudio E. Liver capsule: biliary tree stem cell subpopulations. Hepatology. (2016) 64:644. doi: 10.1002/hep.28546
14. Roskams TA, Theise ND, Balabaud C, Bhagat G, Bhathal PS, Bioulac-Sage P, et al. Nomenclature of the finer branches of the biliary tree: canals, ductules, and ductular reactions in human livers. Hepatology. (2004) 39:1739–45. doi: 10.1002/hep.20130
15. Schmelzer E, Zhang L, Bruce A, Wauthier E, Ludlow J, Yao HL, et al. Human hepatic stem cells from fetal and postnatal donors. J Exp Med. (2007) 204:1973–87. doi: 10.1084/jem.20061603
16. Carpentier R, Suner RE, Van Hul N, Kopp JL, Beaudry JB, Cordi S, et al. Embryonic ductal plate cells give rise to cholangiocytes, periportal hepatocytes, and adult liver progenitor cells. Gastroenterology. (2011) 141:1432–8. doi: 10.1053/j.gastro.2011.06.049
17. Turner R, Lozoya O, Wang Y, Cardinale V, Gaudio E, Alpini G, et al. Human hepatic stem cell and maturational liver lineage biology. Hepatology. (2011) 53:1035–45. doi: 10.1002/hep.24157
18. Libbrecht L, Desmet V, Van Damme B, Roskams T. The immunohistochemical phenotype of dysplastic foci in human liver: correlation with putative progenitor cells. J Hepatol. (2000) 33:76–84. doi: 10.1016/S0168-8278(00)80162-2
19. Spee B, Carpino G, Schotanus BA, Katoonizadeh A, Vander Borght S, Gaudio E, et al. Characterisation of the liver progenitor cell niche in liver diseases: potential involvement of Wnt and Notch signalling. Gut. (2010) 59:247–57. doi: 10.1136/gut.2009.188367
20. Deng X, Zhang X, Li W, Feng RX, Li L, Yi GR, et al. Chronic liver injury induces conversion of biliary epithelial cells into hepatocytes. Cell Stem Cell. (2018) 23:114–22 e3. doi: 10.1016/j.stem.2018.05.022
21. Cardinale V, Wang Y, Carpino G, Cui CB, Gatto M, Rossi M, et al. Multipotent stem/progenitor cells in human biliary tree give rise to hepatocytes, cholangiocytes, and pancreatic islets. Hepatology. (2011) 54:2159–72. doi: 10.1002/hep.24590
22. Igarashi S, Sato Y, Ren XS, Harada K, Sasaki M, Nakanuma Y. Participation of peribiliary glands in biliary tract pathophysiologies. World J Hepatol. (2013) 5:425–32. doi: 10.4254/wjh.v5.i8.425
23. Carpino G, Cardinale V, Onori P, Franchitto A, Berloco PB, Rossi M, et al. Biliary tree stem/progenitor cells in glands of extrahepatic and intraheptic bile ducts: an anatomical in situ study yielding evidence of maturational lineages. J Anatomy. (2012) 220:186–99. doi: 10.1111/j.1469-7580.2011.01462.x
24. Carpino G, Cardinale V, Gentile R, Onori P, Semeraro R, Franchitto A, et al. Evidence for multipotent endodermal stem/progenitor cell populations in human gallbladder. J Hepatol. (2014) 60:1194–202. doi: 10.1016/j.jhep.2014.01.026
25. Carpino G, Nevi L, Overi D, Cardinale V, Lu WY, Di Matteo S, et al. Peribiliary gland niche participates in biliary tree regeneration in mouse and in human primary sclerosing cholangitis. Hepatology. (2020) 71:972–89. doi: 10.1002/hep.30871
26. Cardinale V, Wang Y, Carpino G, Mendel G, Alpini G, Gaudio E, et al. The biliary tree–a reservoir of multipotent stem cells. Nat Rev Gastroenterol Hepatol. (2012) 9:231–40. doi: 10.1038/nrgastro.2012.23
27. Matsui S, Harada K, Miyata N, Okochi H, Miyajima A, Tanaka M. Characterization of peribiliary gland-constituting cells based on differential expression of trophoblast cell surface protein 2 in biliary tract. Am J Pathol. (2018) 188:2059–73. doi: 10.1016/j.ajpath.2018.05.016
28. de Jong IEM, Matton APM, van Praagh JB, van Haaften WT, Wiersema-Buist J, van Wijk LA, et al. Peribiliary glands are key in regeneration of the human biliary epithelium after severe bile duct injury. Hepatology. (2019) 69:1719–34. doi: 10.1002/hep.30365
29. DiPaola F, Shivakumar P, Pfister J, Walters S, Sabla G, Bezerra JA. Identification of intramural epithelial networks linked to peribiliary glands that express progenitor cell markers and proliferate after injury in mice. Hepatology. (2013) 58:1486–96. doi: 10.1002/hep.26485
30. Carpino G, Cardinale V, Renzi A, Hov JR, Berloco PB, Rossi M, et al. Activation of biliary tree stem cells within peribiliary glands in primary sclerosing cholangitis. J Hepatol. (2015) 63:1220–8. doi: 10.1016/j.jhep.2015.06.018
31. Carpino G, Cardinale V, Folseraas T, Overi D, Grzyb K, Costantini D, et al. Neoplastic transformation of the peribiliary stem cell niche in cholangiocarcinoma arisen in primary sclerosing cholangitis. Hepatology. (2019) 69:622–38. doi: 10.1002/hep.30210
32. Pepe-Mooney BJ, Dill MT, Alemany A, Ordovas-Montanes J, Matsushita Y, Rao A, et al. Single-cell analysis of the liver epithelium reveals dynamic heterogeneity and an essential role for YAP in homeostasis and regeneration. Cell Stem Cell. (2019) 25:23–38 e8. doi: 10.1016/j.stem.2019.04.004
33. Gouw AS, Clouston AD, Theise ND. Ductular reactions in human liver: diversity at the interface. Hepatology. (2011) 54:1853–63. doi: 10.1002/hep.24613
34. Stueck AE, Wanless IR. Hepatocyte buds derived from progenitor cells repopulate regions of parenchymal extinction in human cirrhosis. Hepatology. (2015) 61:1696–707. doi: 10.1002/hep.27706
35. Dezso K, Rokusz A, Bugyik E, Szucs A, Szuak A, Dorogi B, et al. Human liver regeneration in advanced cirrhosis is organized by the portal tree. J Hepatol. (2017) 66:778–86. doi: 10.1016/j.jhep.2016.11.014
36. Schuster S, Cabrera D, Arrese M, Feldstein AE. Triggering and resolution of inflammation in NASH. Nat Rev Gastroenterol Hepatol. (2018) 15:349–64. doi: 10.1038/s41575-018-0009-6
37. Overi D, Carpino G, Franchitto A, Onori P, Gaudio E. Hepatocyte injury and hepatic stem cell niche in the progression of non-alcoholic steatohepatitis. Cells. (2020) 9:30590. doi: 10.3390/cells9030590
38. Carpino G, Pastori D, Baratta F, Overi D, Labbadia G, Polimeni L, et al. PNPLA3 variant and portal/periportal histological pattern in patients with biopsy-proven non-alcoholic fatty liver disease: a possible role for oxidative stress. Sci Rep. (2017) 7:15756. doi: 10.1038/s41598-017-15943-z
39. Nobili V, Carpino G, De Peppo F, Caccamo R, Mosca A, Romito I, et al. Laparoscopic sleeve gastrectomy improves nonalcoholic fatty liver disease-related liver damage in adolescents by reshaping cellular interactions and hepatic adipocytokine production. J Pediatr. (2018) 194:100–8 e3. doi: 10.1016/j.jpeds.2017.10.036
40. Gadd VL, Skoien R, Powell EE, Fagan KJ, Winterford C, Horsfall L, et al. The portal inflammatory infiltrate and ductular reaction in human nonalcoholic fatty liver disease. Hepatology. (2014) 59:1393–405. doi: 10.1002/hep.26937
41. Guldiken N, Kobazi Ensari G, Lahiri P, Couchy G, Preisinger C, Liedtke C, et al. Keratin 23 is a stress-inducible marker of mouse and human ductular reaction in liver disease. J Hepatol. (2016) 65:552–9. doi: 10.1016/j.jhep.2016.04.024
42. Franchitto A, Carpino G, Alisi A, De Peppo F, Overi D, De Stefanis C, et al. The contribution of the adipose tissue-liver axis in pediatric patients with nonalcoholic fatty liver disease after laparoscopic sleeve gastrectomy. J Pediatr. (2020) 216:117–27 e2. doi: 10.1016/j.jpeds.2019.07.037
43. Carpino G, Del Ben M, Pastori D, Carnevale R, Baratta F, Overi D, et al. Increased liver localization of lipopolysaccharides in human and experimental non-alcoholic fatty liver disease. Hepatology. (2019). doi: 10.1002/hep.31056. [Epub ahead of print].
44. Richardson MM, Jonsson JR, Powell EE, Brunt EM, Neuschwander-Tetri BA, Bhathal PS, et al. Progressive fibrosis in nonalcoholic steatohepatitis: association with altered regeneration and a ductular reaction. Gastroenterology. (2007) 133:80–90. doi: 10.1053/j.gastro.2007.05.012
45. Nobili V, Carpino G, Alisi A, Franchitto A, Alpini G, De Vito R, et al. Hepatic progenitor cells activation, fibrosis, and adipokines production in pediatric nonalcoholic fatty liver disease. Hepatology. (2012) 56:2142–53. doi: 10.1002/hep.25742
46. Nobili V, Alisi A, Cutrera R, Carpino G, De Stefanis C, D'Oria V, et al. Altered gut-liver axis and hepatic adiponectin expression in OSAS: novel mediators of liver injury in paediatric non-alcoholic fatty liver. Thorax. (2015) 70:769–81. doi: 10.1136/thoraxjnl-2015-206782
47. Sancho-Bru P, Altamirano J, Rodrigo-Torres D, Coll M, Millan C, Jose Lozano J, et al. Liver progenitor cell markers correlate with liver damage and predict short-term mortality in patients with alcoholic hepatitis. Hepatology. (2012) 55:1931–41. doi: 10.1002/hep.25614
48. Aguilar-Bravo B, Rodrigo-Torres D, Arino S, Coll M, Pose E, Blaya D, et al. Ductular reaction cells display an inflammatory profile and recruit neutrophils in alcoholic hepatitis. Hepatology. (2019) 69:2180–95. doi: 10.1002/hep.30472
49. Lanthier N, Rubbia-Brandt L, Lin-Marq N, Clement S, Frossard JL, Goossens N, et al. Hepatic cell proliferation plays a pivotal role in the prognosis of alcoholic hepatitis. J Hepatol. (2015) 63:609–21. doi: 10.1016/j.jhep.2015.04.003
50. Dubuquoy L, Louvet A, Lassailly G, Truant S, Boleslawski E, Artru F, et al. Progenitor cell expansion and impaired hepatocyte regeneration in explanted livers from alcoholic hepatitis. Gut. (2015) 64:1949–60. doi: 10.1136/gutjnl-2014-308410
51. Odena G, Chen J, Lozano JJ, Altamirano J, Rodrigo-Torres D, Affo S, et al. LPS-TLR4 pathway mediates ductular cell expansion in alcoholic hepatitis. Sci Rep. (2016) 6:35610. doi: 10.1038/srep35610
52. Rastogi A, Maiwall R, Bihari C, Trehanpati N, Pamecha V, Sarin SK. Two-tier regenerative response in liver failure in humans. Virchows Arch. (2014) 464:565–73. doi: 10.1007/s00428-014-1547-0
53. Dezso K, Nagy P, Paku S. Human liver regeneration following massive hepatic necrosis: two distinct patterns. J Gastroenterol Hepatol. (2020) 35:124–34. doi: 10.1111/jgh.14721
54. Lindor KD, Bowlus CL, Boyer J, Levy C, Mayo M. Primary biliary cholangitis: 2018 practice guidance from the American Association for the Study of Liver Diseases. Hepatology. (2019) 69:394–419. doi: 10.1002/hep.30145
55. Carpino G, Cardinale V, Folseraas T, Overi D, Floreani A, Franchitto A, et al. Hepatic stem/progenitor cell activation differs between primary sclerosing and primary biliary cholangitis. Am J Pathol. (2018) 188:627–39. doi: 10.1016/j.ajpath.2017.11.010
56. Kennedy L, Francis H, Invernizzi P, Venter J, Wu N, Carbone M, et al. Secretin/secretin receptor signaling mediates biliary damage and liver fibrosis in early-stage primary biliary cholangitis. FASEB J. (2019) 33:10269–79. doi: 10.1096/fj.201802606R
57. Carbone M, Nardi A, Flack S, Carpino G, Varvaropoulou N, Gavrila C, et al. Pretreatment prediction of response to ursodeoxycholic acid in primary biliary cholangitis: development and validation of the UDCA Response Score. Lancet Gastroenterol Hepatol. (2018) 3:626–34. doi: 10.1016/S2468-1253(18)30163-8
58. Wilson DH, Jarman EJ, Mellin RP, Wilson ML, Waddell SH, Tsokkou P, et al. Non-canonical Wnt signalling regulates scarring in biliary disease via the planar cell polarity receptors. Nat Commun. (2020) 11:445. doi: 10.1038/s41467-020-14283-3
59. Planas-Paz L, Sun T, Pikiolek M, Cochran NR, Bergling S, Orsini V, et al. YAP, but not RSPO-LGR4/5, signaling in biliary epithelial cells promotes a ductular reaction in response to liver injury. Cell Stem Cell. (2019) 25:39–53 e10. doi: 10.1016/j.stem.2019.04.005
60. Fickert P, Pollheimer MJ, Beuers U, Lackner C, Hirschfield G, Housset C, et al. Characterization of animal models for primary sclerosing cholangitis (PSC). J Hepatol. (2014) 60:1290–303. doi: 10.1016/j.jhep.2014.02.006
61. Matsubara T, Kozaka K, Matsui O, Nakanuma Y, Uesaka K, Inoue D, et al. Peribiliary glands: development, dysfunction, related conditions and imaging findings. Abdom Radiol. (2020) 45:416–36. doi: 10.1007/s00261-019-02298-4
62. Franchitto A, Overi D, Mancinelli R, Mitterhofer AP, Muiesan P, Tinti F, et al. Peribiliary gland damage due to liver transplantation involves peribiliary vascular plexus and vascular endothelial growth factor. Eur J Histochem. (2019) 63:3022. doi: 10.4081/ejh.2019.3022
63. Carpino G, Puca R, Cardinale V, Renzi A, Scafetta G, Nevi L, et al. Peribiliary glands as a niche of extrapancreatic precursors yielding insulin-producing cells in experimental and human diabetes. Stem Cells. (2016) 34:1332–42. doi: 10.1002/stem.2311
64. Kendall T, Verheij J, Gaudio E, Evert M, Guido M, Goeppert B, et al. Anatomical, histomorphological and molecular classification of cholangiocarcinoma. Liver Int. (2019) 39(Suppl.1):7–18. doi: 10.1111/liv.14093
65. Hoadley KA, Yau C, Hinoue T, Wolf DM, Lazar AJ, Drill E, et al. Cell-of-origin patterns dominate the molecular classification of 10,000 tumors from 33 types of cancer. Cell. (2018) 173:291–304 e6. doi: 10.1016/j.cell.2018.03.022
66. Tomasetti C, Vogelstein B. Cancer etiology. Variation in cancer risk among tissues can be explained by the number of stem cell divisions. Science. (2015) 347:78–81. doi: 10.1126/science.1260825
67. Banales JM, Cardinale V, Carpino G, Marzioni M, Andersen JB, Invernizzi P, et al. Expert consensus document: cholangiocarcinoma: current knowledge and future perspectives consensus statement from the European Network for the Study of Cholangiocarcinoma (ENS-CCA). Nat Rev Gastroenterol Hepatol. (2016) 13:261–80. doi: 10.1038/nrgastro.2016.51
68. Cardinale V, Renzi A, Carpino G, Torrice A, Bragazzi MC, Giuliante F, et al. Profiles of cancer stem cell subpopulations in cholangiocarcinomas. Am J Pathol. (2015) 185:1724–39. doi: 10.1016/j.ajpath.2015.02.010
69. Nakagawa H, Suzuki N, Hirata Y, Hikiba Y, Hayakawa Y, Kinoshita H, et al. Biliary epithelial injury-induced regenerative response by IL-33 promotes cholangiocarcinogenesis from peribiliary glands. Proc Natl Acad Sci USA. (2017) 114:E3806–15. doi: 10.1073/pnas.1619416114
70. Carnevale G, Carpino G, Cardinale V, Pisciotta A, Riccio M, Bertoni L, et al. Activation of Fas/FasL pathway and the role of c-FLIP in primary culture of human cholangiocarcinoma cells. Sci Rep. (2017) 7:14419. doi: 10.1038/s41598-017-14838-3
71. Lanzoni G, Oikawa T, Wang Y, Cui CB, Carpino G, Cardinale V, et al. Concise review: clinical programs of stem cell therapies for liver and pancreas. Stem Cells. (2013) 31:2047–60. doi: 10.1002/stem.1457
72. Qi X, Guo X, Su C. Clinical outcomes of the transplantation of stem cells from various human tissue sources in the management of liver cirrhosis: a systematic review and meta-analysis. Curr Stem Cell Res Ther. (2015) 10:166–80. doi: 10.2174/1574888X09666141112114011
73. Lee CA, Sinha S, Fitzpatrick E, Dhawan A. Hepatocyte transplantation and advancements in alternative cell sources for liver-based regenerative medicine. J Mol Med. (2018) 96:469–81. doi: 10.1007/s00109-018-1638-5
74. Fox IJ, Chowdhury JR, Kaufman SS, Goertzen TC, Chowdhury NR, Warkentin PI, et al. Treatment of the Crigler-Najjar syndrome type I with hepatocyte transplantation. N Engl J Med. (1998) 338:1422–6. doi: 10.1056/NEJM199805143382004
75. An SY, Jang YJ, Lim HJ, Han J, Lee J, Lee G, et al. Milk fat globule-EGF factor 8, secreted by mesenchymal stem cells, protects against liver fibrosis in mice. Gastroenterology. (2017) 152:1174–86. doi: 10.1053/j.gastro.2016.12.003
76. Lanthier N, Lin-Marq N, Rubbia-Brandt L, Clement S, Goossens N, Spahr L. Autologous bone marrow-derived cell transplantation in decompensated alcoholic liver disease: what is the impact on liver histology and gene expression patterns? Stem Cell Res Ther. (2017) 8:88. doi: 10.1186/s13287-017-0541-2
77. Newsome PN, Fox R, King AL, Barton D, Than NN, Moore J, et al. Granulocyte colony-stimulating factor and autologous CD133-positive stem-cell therapy in liver cirrhosis (REALISTIC): an open-label, randomised, controlled phase 2 trial. Lancet Gastroenterol Hepatol. (2018) 3:25–36. doi: 10.1016/S2468-1253(17)30326-6
78. Peng L, Xie DY, Lin BL, Liu J, Zhu HP, Xie C, et al. Autologous bone marrow mesenchymal stem cell transplantation in liver failure patients caused by hepatitis B: short-term and long-term outcomes. Hepatology. (2011) 54:820–8. doi: 10.1002/hep.24434
79. Spahr L, Chalandon Y, Terraz S, Kindler V, Rubbia-Brandt L, Frossard JL, et al. Autologous bone marrow mononuclear cell transplantation in patients with decompensated alcoholic liver disease: a randomized controlled trial. PLoS ONE. (2013) 8:e53719. doi: 10.1371/journal.pone.0053719
80. Khan AA, Parveen N, Mahaboob VS, Rajendraprasad A, Ravindraprakash HR, Venkateswarlu J, et al. Treatment of Crigler-Najjar Syndrome type 1 by hepatic progenitor cell transplantation: a simple procedure for management of hyperbilirubinemia. Transplant Proc. (2008) 40:1148–50. doi: 10.1016/j.transproceed.2008.03.022
81. Lysy PA, Najimi M, Stephenne X, Bourgois A, Smets F, Sokal EM. Liver cell transplantation for Crigler-Najjar syndrome type I: update and perspectives. World J Gastroenterol. (2008) 14:3464–70. doi: 10.3748/wjg.14.3464
82. Khan AA, Shaik MV, Parveen N, Rajendraprasad A, Aleem MA, Habeeb MA, et al. Human fetal liver-derived stem cell transplantation as supportive modality in the management of end-stage decompensated liver cirrhosis. Cell Transplant. (2010) 19:409–18. doi: 10.3727/096368909X484707a
83. Pietrosi G, Vizzini G, Gerlach J, Chinnici C, Luca A, Amico G, et al. Phases I-II matched case-control study of human fetal liver cell transplantation for treatment of chronic liver disease. Cell Transplant. (2015) 24:1627–38. doi: 10.3727/096368914X682422
84. Cardinale V, Carpino G, Gentile R, Napoletano C, Rahimi H, Franchitto A, et al. Transplantation of human fetal biliary tree stem/progenitor cells into two patients with advanced liver cirrhosis. BMC Gastroenterol. (2014) 14:204. doi: 10.1186/s12876-014-0204-z
85. Nevi L, Cardinale V, Carpino G, Costantini D, Di Matteo S, Cantafora A, et al. Cryopreservation protocol for human biliary tree stem/progenitors, hepatic and pancreatic precursors. Sci Rep. (2017) 7:6080. doi: 10.1038/s41598-017-05858-0
86. Nevi L, Carpino G, Costantini D, Cardinale V, Riccioni O, Di Matteo S, et al. Hyaluronan coating improves liver engraftment of transplanted human biliary tree stem/progenitor cells. Stem Cell Res Ther. (2017) 8:68. doi: 10.1186/s13287-017-0492-7
87. Costantini D, Overi D, Casadei L, Cardinale V, Nevi L, Carpino G, et al. Simulated microgravity promotes the formation of tridimensional cultures and stimulates pluripotency and a glycolytic metabolism in human hepatic and biliary tree stem/progenitor cells. Sci Rep. (2019) 9:5559. doi: 10.1038/s41598-019-41908-5
88. Carpino G, Renzi A, Franchitto A, Cardinale V, Onori P, Reid L, et al. Stem/progenitor cell niches involved in hepatic and biliary regeneration. Stem Cells Int. (2016) 2016:3658013. doi: 10.1155/2016/3658013
89. Semeraro R, Carpino G, Cardinale V, Onori P, Gentile R, Cantafora A, et al. Multipotent stem/progenitor cells in the human foetal biliary tree. J Hepatol. (2012) 57:987–94. doi: 10.1016/j.jhep.2012.07.013
90. Sato T, Vries RG, Snippert HJ, van de Wetering M, Barker N, Stange DE, et al. Single Lgr5 stem cells build crypt-villus structures in vitro without a mesenchymal niche. Nature. (2009) 459:262–5. doi: 10.1038/nature07935
91. Barker N, Huch M, Kujala P, van de Wetering M, Snippert HJ, van Es JH, et al. Lgr5(+ve) stem cells drive self-renewal in the stomach and build long-lived gastric units in vitro. Cell Stem Cell. (2010) 6:25–36. doi: 10.1016/j.stem.2009.11.013
92. Huch M, Dorrell C, Boj SF, van Es JH, Li VS, van de Wetering M, et al. In vitro expansion of single Lgr5+ liver stem cells induced by Wnt-driven regeneration. Nature. (2013) 494:247–50. doi: 10.1038/nature11826
93. Huch M, Gehart H, van Boxtel R, Hamer K, Blokzijl F, Verstegen MM, et al. Long-term culture of genome-stable bipotent stem cells from adult human liver. Cell. (2015) 160:299–312. doi: 10.1016/j.cell.2014.11.050
94. Aloia L, McKie MA, Vernaz G, Cordero-Espinoza L, Aleksieva N, van den Ameele J, et al. Epigenetic remodelling licences adult cholangiocytes for organoid formation and liver regeneration. Nat Cell Biol. (2019) 21:1321–33. doi: 10.1038/s41556-019-0402-6
95. Rimland CA, Tilson SG, Morell CM, Tomaz RA, Lu WY, Adams SE, et al. Regional differences in human biliary tissues and corresponding in vitro derived organoids. Hepatology. (2020). doi: 10.1002/hep.31252. [Epub ahead of print].
96. Sampaziotis F, Justin AW, Tysoe OC, Sawiak S, Godfrey EM, Upponi SS, et al. Reconstruction of the mouse extrahepatic biliary tree using primary human extrahepatic cholangiocyte organoids. Nat Med. (2017) 23:954–63. doi: 10.1038/nm.4360
97. Kruitwagen HS, Oosterhoff LA, Vernooij I, Schrall IM, van Wolferen ME, Bannink F, et al. Long-term adult feline liver organoid cultures for disease modeling of hepatic steatosis. Stem Cell Rep. (2017) 8:822–30. doi: 10.1016/j.stemcr.2017.02.015
98. Soroka CJ, Assis DN, Boyer JL. Patient-derived organoids from human bile: an in vitro method to study cholangiopathies. Methods Mol Biol. (2019) 1981:363–72. doi: 10.1007/978-1-4939-9420-5_24
99. Broutier L, Mastrogiovanni G, Verstegen MM, Francies HE, Gavarro LM, Bradshaw CR, et al. Human primary liver cancer-derived organoid cultures for disease modeling and drug screening. Nat Med. (2017) 23:1424–35. doi: 10.1038/nm.4438
Keywords: progenitor cells, liver, biliary tree, cholangiopathy, cholangiocarcinoma
Citation: Safarikia S, Carpino G, Overi D, Cardinale V, Venere R, Franchitto A, Onori P, Alvaro D and Gaudio E (2020) Distinct EpCAM-Positive Stem Cell Niches Are Engaged in Chronic and Neoplastic Liver Diseases. Front. Med. 7:479. doi: 10.3389/fmed.2020.00479
Received: 01 April 2020; Accepted: 15 July 2020;
Published: 02 September 2020.
Edited by:
Gianfranco Danilo Alpini, Indiana University, United StatesReviewed by:
Lindsey Kennedy, Texas A&M University, United StatesUlrich Beuers, Amsterdam University Medical Center, Netherlands
Copyright © 2020 Safarikia, Carpino, Overi, Cardinale, Venere, Franchitto, Onori, Alvaro and Gaudio. This is an open-access article distributed under the terms of the Creative Commons Attribution License (CC BY). The use, distribution or reproduction in other forums is permitted, provided the original author(s) and the copyright owner(s) are credited and that the original publication in this journal is cited, in accordance with accepted academic practice. No use, distribution or reproduction is permitted which does not comply with these terms.
*Correspondence: Guido Carpino, Z3VpZG8uY2FycGlub0B1bmlyb21hMS5pdA==
†These authors have contributed equally to this work