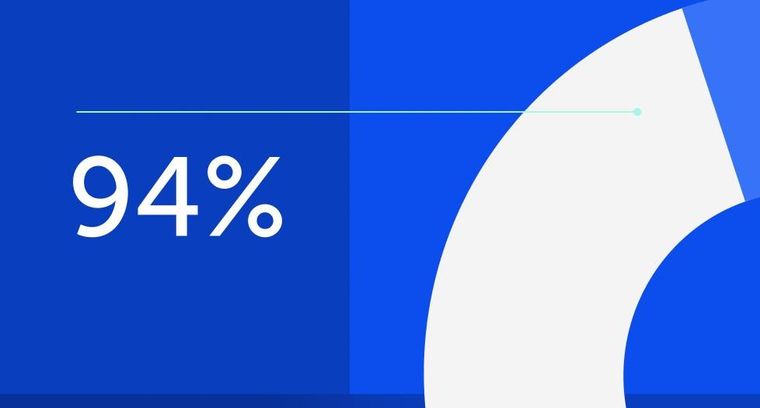
94% of researchers rate our articles as excellent or good
Learn more about the work of our research integrity team to safeguard the quality of each article we publish.
Find out more
PERSPECTIVE article
Front. Med., 05 August 2020
Sec. Intensive Care Medicine and Anesthesiology
Volume 7 - 2020 | https://doi.org/10.3389/fmed.2020.00416
This article is part of the Research TopicMicrocirculation Guided/Targeted ResuscitationView all 12 articles
Circulatory shock is associated with marked disturbances of the macro- and microcirculation and flow heterogeneities. Furthermore, a lack of tissue adenosine trisphosphate (ATP) and mitochondrial dysfunction are directly associated with organ failure and poor patient outcome. While it remains unclear if microcirculation-targeted resuscitation strategies can even abolish shock-induced flow heterogeneity, mitochondrial dysfunction and subsequently diminished ATP production could still lead to organ dysfunction and failure even if microcirculatory function is restored or maintained. Preserved mitochondrial function is clearly associated with better patient outcome. This review elucidates the role of the microcirculation and mitochondria during circulatory shock and patient management and will give a viewpoint on the advantages and disadvantages of tailoring resuscitation to microvascular or mitochondrial targets.
Shock can be defined as the “imbalance between oxygen supply and requirements” (1). This imbalance can be due to “inadequate O2 transport” resulting from “hypovolemia,” “cardiogenic factors” (e.g., myocardial infarction), “obstruction” (e.g., pulmonary embolism), and/or “distributive shock” (e.g., septic shock), which is characterized by “decreased systemic vascular resistance and altered oxygen extraction” (1). Hence, cellular hypoxia is central to shock pathophysiology. Current resuscitation strategies mainly address macrocirculatory targets to restore appropriate tissue perfusion, but achieving these targets does not necessarily result in improved microcirculatory perfusion, since there may be a marked dissociation between the former and the latter (2–4).
It is beyond any doubt that circulatory shock, no matter whether it is septic (5) or traumatic-hemorrhagic (i.e., hypovolemic) (6), is associated with marked disturbances of the microcirculation. Moreover, it is well-established that survivors present with improved markers of microcirculatory perfusion (7, 8). Nevertheless, it remains a matter of debate as to whether this is an epiphenomenon or a causal relationship, in other words, whether “recruiting the microcirculation” (9) or “microvascular resuscitation” (10) is the “magic bullet” that will improve survival after circulatory shock. This question still raises fairly equivocal viewpoints (9, 11, 12). Trezciak et al. (13) failed to demonstrate any relationship between changes in microcirculatory markers and the severity of organ failure. Furthermore, in resuscitated patients with septic shock, a direct relationship was noted between eventual outcome and skeletal muscle adenosine trisphosphate (ATP) (14), suggesting a perhaps more important role for cellular metabolic capacity compared to microcirculatory O2 supply, at least in sepsis. This review covers the respective roles of the microcirculation and mitochondria in circulatory shock (summarized in Figure 1), with a particular focus on traumatic-hemorrhagic vs. septic shock. It addresses the question as to whether altered mitochondrial respiration and subsequently diminished ATP production could still lead to organ dysfunction and failure, despite adequate tissue oxygenation, even if microcirculatory function is restored or maintained (15, 16).
Figure 1. The microvasculature and mitochondria in circulatory shock. Illustrations of the microcirculation and the mitochondrium are taken from the Library of Science and Medical Illustrations (somersault18:24, https://creativecommons.org/licenses/by-nc-sa/4.0/).
There is numerous experimental and clinical evidence for impaired microcirculatory perfusion during shock, no matter whether the origin is sepsis or trauma-hemorrhage (i.e., hypovolemic). In experimental animal models, impaired microcirculatory perfusion has been demonstrated using various techniques in the heart, kidney, liver, gut, and brain, even after resuscitation had restored the macrocirculatory hemodynamics (17–22).
The disturbance of the microcirculation is characterized by a markedly enhanced heterogeneity of blood flow comprising obstructed vessels, vessels with stagnant or intermittently on/off flow related to vasoconstriction, and vessels with an increased blood flow velocity (2). All these effects, together with an increased O2 diffusion distance (e.g., due to tissue edema) and/or reduced systemic O2 transport capacity (e.g., due to hemodilution) will result in impaired tissue O2 availability (4). In fact, in the intestinal mucosa, increased O2 extraction was directly related to the degree of these microcirculatory disturbances (23). Finally, the increased heterogeneity of the intestinal mucosal microcirculation, characterized by a substantial proportion of non-perfused capillaries, coincided with increased regional venous lactate/pyruvate ratios, a well-established marker for cellular dysoxia, and tissue acidosis (17).
This experimental evidence is supported by clinical observations. In a seminal study in stabilized septic patients, De Backer et al. (5) demonstrated the presence of marked microcirculatory disturbances characterized by a decreased density of perfused small vessels, and a large number of either non-perfused or only intermittently perfused vessels. While the proportion of perfused capillaries was not related to systemic macro-hemodynamics, it was significantly lower in non-survivors. This group (7, 24) and others have subsequently confirmed these findings in patients with sepsis (8, 25, 26) and following trauma-hemorrhage (6).
Given the importance of an impaired microcirculation, “microvascular resuscitation” (10) or “recruiting the microcirculation” (9) has been advocated. Strikingly, few clinical studies have been published to date that integrate a microcirculation-targeted resuscitation into the study protocol. One, by Boerma et al. (27), found no outcome benefit from targeting the sublingual microcirculation with nitroglycerin. Whether such an approach may provide a “magic bullet” to improve survival after circulatory shock has generated conflicting views (9, 11, 12). This is in part due to the ongoing lack of readily accessible data from bedside techniques that assess the microcirculation, notwithstanding the conclusions of a recent consensus conference (28). Moreover, despite the undoubtedly existing impairment of microcirculatory perfusion in shock, the subsequent conclusion that tissue O2 availability is reduced to a meaningful degree has been questioned, at least in resuscitated septic shock: in one study, not only was skeletal tissue PO2 not reduced in such patients, but—in sharp contrast to patients with cardiac pump failure—was even higher than a healthy control group (29).
Any recruitment maneuver targeting the microcirculation will comprise two aspects, i.e., the “(re-)opening” of the capillary network (e.g., using fluid resuscitation, ino-dilation and restriction of vasoconstriction) and the subsequent attenuation of flow heterogeneity (summarized in Figure 2A) (2). Based on this rationale, fluid resuscitation (30–34), dobutamine (35), levosimendan (36), milrinone (37), nitric oxide (NO) donors (38–40), and prostacyclin (PGI2) (40–42) have all been tested. In experimental settings, this approach was often successful but the majority of animal studies were only of short duration and/or did not include standard intensive care measures, which may limit transferability into clinical practice (43). Given the potential NO- or NO-derivatives induced uncoupling of mitochondrial respiration and inhibition of complex I and complex IV (14, 44–46), caution must be taken with NO-donors as a therapeutic strategy to avoid detrimental effects on the mitochondria. In longer-term, fluid-resuscitated large animal models, therapeutic interventions that attenuated shock-related cellular dysoxia (e.g., selective inhibition of the inducible NO synthase (iNOS), antioxidant infusion, therapeutic hyperoxia) revealed that any beneficial effect on the microcirculation coincided with improved parameters of inflammation, oxidative and nitrosative stress, and/or cellular metabolism (21, 47, 48). It thus remains unclear whether the beneficial effect on the microcirculation is the cause or the result of these responses. Indeed, neither selective iNOS inhibition nor infusion of the PGI2 analog iloprost had any effect on the measured parameters of microcirculation at all, but still resulted in improved markers of cellular dysoxia (49, 50).
Figure 2. Experimental therapeutic strategies. (A) Microvascular recruitment. (B) Mitochondrial protection and stimulation. CIV, cytochrome c oxidase; ROS, reactive oxygen species. Illustrations of the microcirculation and the mitochondrium are taken from the Library of Science and Medical Illustrations (somersault18:24, https://creativecommons.org/licenses/by-nc-sa/4.0/) .
To date, the results of clinical interventional studies that have integrated monitoring of microcirculatory perfusion and oxygenation have failed to show a direct relationship between the effects of the respective intervention on the measured markers of microcirculation and either mortality and/or morbidity. For example, Ospina-Tascon et al. (51) found that fluid resuscitation (infusion of at least 1,000 ml Ringer's lactate or 400 ml 4% albumin solution) within the first 24 h of diagnosis of sepsis increased the fraction of perfused small vessels, and this effect was directly related to a decrease in lactate concentration. Beyond 48 h of diagnosis, no effect was observed (51).
Dobutamine can recruit the microcirculation in septic patients but, again, its effects were directly related to a decrease in lactate levels, rather than the individual macrocirculatory response (52). More recently, Hernandez et al. (53) failed to demonstrate any beneficial effect of dobutamine neither on the sublingual microcirculation nor on metabolic, hepatosplanchnic or peripheral perfusion parameters. Based on a theoretical benefit of vasodilating drugs (54–56) the use of NO releasing compounds has been investigated. Administration of nitroglycerin increased the perfusion of sublingual microvessels (57), but, as mentioned previously, continuous infusion over 24 h trended to a worse outcome in a study including 70 patients (p = 0.08) (27). Inhaling 40 ppm of NO over 6 h after initial resuscitation neither improved microcirculatory flow, lactate clearance, nor organ dysfunction, and no association was found between parameters of microcirculatory perfusion and organ dysfunction after initial resuscitation (13). Based on promising results with infusion of PGI2 (or its analogs) (58, 59), a multicenter randomized controlled trial is currently under way in patients with septic shock and “persistent microperfusion defects” (I-MICRO, NCT03788837). However, it must be underscored that PGI2 and its analogs have effects beyond those on the microcirculation (60), in particular with respect to cellular energy metabolism (61–65). Whether or not vasoconstrictors have deleterious effects on the microcirculation has also been recently questioned. In healthy volunteers, neither norepinephrine, phenylephrine nor vasopressin affected microcirculatory parameters prior to or after bolus injection of endotoxin, despite an immediate rise in blood pressure (66). Overall, a recent meta-analysis on the impact of vasoactive drugs on microcirculatory blood flow concluded that there is “no robust evidence that any agent can lead to improved microvascular flow” and that “no study demonstrated outcome benefit” (67).
Some two decades ago, the late Mitchell Fink created the term “cytopathic hypoxia” (68, 69). The above-detailed disturbances of the microcirculation could result in inadequate cellular O2 supply as a reason for decreased ATP production and subsequent hyperlactatemia. Clearly, aggravation of microvascular heterogeneity with mismatch of the local O2-transport/ uptake relationship could explain at least in part, why the threshold of O2 supply, below which hyperlactatemia occurs, is much higher during sepsis than under normal conditions (70, 71). In this context, the concept of cytopathic hypoxia (or, perhaps, more accurately, cytopathic dysoxia) attempts to reconcile the phenomenon that organ failure coincides with hardly any cell death, availability of oxygen at the cellular level, relatively minor inflammatory cell migration, and capacity of these failed organs to recover (72, 73). Cytopathic dysoxia refers to impaired ATP formation despite normal [or even supra-normal (20)] tissue PO2 levels. It can result from several factors, for instance, diminished delivery of key substrates (e.g., pyruvate) into the tricarboxylic acid (TCA) cycle, inhibition of various TCA cycle or (in particular) electron transport chain enzymes such as Complexes I and IV (74, 75), and uncoupling [“slipping” (76)] of the electron transport chain with a decrease in the proton gradient across the inner mitochondrial membrane resulting in production of heat rather than generation of ATP.
To date, the assessment of mitochondrial function is mostly limited to ex vivo methods, which might misrepresent the in vivo situation. A detailed discussion of available methods for ex vivo and in vivo mitochondrial measurements are beyond the scope of this paper, but have been recently compared by Bettink et al. (77), with a particular focus on the protoporphyrin IX-triplet state lifetime technique, which is applicable in vivo (78). However, in the light of the absolute values reported for the latter in vivo measurement technique, which range between 30 and 110 mmHg (77, 79), the results obtained with this method have to be interpreted with care. In contrast to these “mitochondrial” PO2 levels, reports of tissue PO2 measured with the Pd-phosphorescence quenching method typically range between 20 and 25 mmHg, with maximally 52 mmHg and even levels as low as 5 mmHg detected, which is the threshold for tissue to be considered anoxic (80). A steep O2 pressure gradient is needed to facilitate O2 diffusion from capillaries through the interstitium to cells/mitochondria. Thus, it is not surprising to see reports of intracellular PO2 of no more than 1–10 mmHg (81). Mitochondrial ATP supply is stable over a wide range of intracellular PO2, measured ex vivo by oxygraphy, and will only suffer if the mitochondrial PO2 is lower than 0.1–0.5 mmHg (81). Thus, the values reported for mitoPO2 of 30–110 mmHg may represent a mixture of PO2 values from different compartments rather than solely mitoPO2.
Despite these limitations in determining mitochondrial function, there is ample experimental evidence that reduced (disturbed) mitochondrial respiratory activity assumes crucial importance for shock-related organ dysfunction or failure, similar to the potential role of impaired microcirculatory perfusion and oxygenation. Several rodent studies that included resuscitation demonstrated hyperlactatemia despite unchanged or even increased tissue PO2, and this coincided with both reduced function and structural damage to mitochondria (74, 82–85). Resuscitated models investigating higher species (cats, swine, baboons) and characterized by a normotensive and a normo- or even hyperdynamic circulation, confirmed these findings (20, 86–89). In this context, studies simultaneously recording parameters of both microcirculation and cellular O2 utilization assume particular importance: LPS-challenged, fluid-resuscitated swine showed a reduced efficiency of hepatic mitochondrial respiration despite maintained liver surface laser Doppler blood flow (20). The same group however reported in a longer-term model, unchanged liver tissue mitochondrial resuscitation with a tendency toward enhanced microcirculatory blood flow (88). They undertook a meta-analysis reviewing both experimental models and clinical studies and reported variable results on mitochondrial function depending on the species, organ, and time point investigated (90). However, the available experimental literature mainly originates from young and otherwise healthy animals, which does not represent the more frequent clinical scenario of elderly patients with comorbidities, a common pitfall of experimental studies in shock research in general (43). Nevertheless, there is elegant clinical evidence that despite adequate resuscitation, reduced mitochondrial respiration is (i) present in patients after the initial management of circulatory shock, and (ii) associated with worse outcomes. In a landmark study in patients with septic shock, complex I activity was lower in non-survivors than in survivors; and corresponding tissue ATP content values mirrored this result (14). Moreover, complex I activity was inversely related to the mitochondrial antioxidant, glutathione, and directly related to nitrate/nitrite concentrations, suggesting a crucial role of oxidative and nitrosative stress in shock-related mitochondrial (dys)function. The same group subsequently demonstrated that impaired mitochondrial function coincided with a decrease in mitochondrial respiratory protein content in non-survivors. In contrast, survivors showed an early activation of mitochondrial biogenesis (91). A post-hoc analysis of the HYPER2S trial (92) in patients with sepsis-induced hypotension found that hyperoxia during the first 24 h of treatment increased mortality rate at day 28 (p = 0.054) in hyperlactatemic patients, whereas in those with lactate levels ≤ 2 mmol/L there was no effect on mortality or morbidity. Since hyperoxia increases tissue O2 partial pressure, even under conditions of profound reduction of O2 supply (93), this finding implicitly suggests that outcome of septic shock is associated with impaired cellular O2 utilization rather than microcirculatory O2 availability, possibly due to aggravated oxidative and nitrosative stress (94).
Multiple mitochondria-targeted therapeutic strategies have been proposed (summarized in Figure 2B) (95–98). Despite several promising experimental therapies, there are currently no clinically-approved strategies for mitochondrial protection. Metformin has been shown to be beneficial for the attenuation of mitochondrial transition pore opening (99), stimulation of mitochondrial biogenesis and reduction of mitochondrial ROS production, but has to be used with caution in shock patients due to its potentially severe side effects, i.e., renal impairment, and lactic acidosis (100).
In contrast, the glucose-lowering compound Imeglimine, though to date only tested experimentally, has none of the side effects as metformin, but inhibited mitochondrial permeability transition, improved mitochondrial function, and was associated with less acidosis (100, 101). The inhibition of the mitochondrial permeability transition pore with cyclosporine A showed promise in a pre-clinical sepsis model and a large-animal model of traumatic brain injury (102, 103). In humans, cyclosporine A did not show a benefit in cardiac arrest and acute myocardial infarct (104, 105), and it was never clinically approved for the treatment of any type of circulatory shock.
Given the above-mentioned role of oxidative and nitrosative stress, mitochondria-targeted antioxidant strategies and manipulation of shock-related excess formation of nitric oxide (NO) and/or peroxynitrite (ONOO−) have been suggested but so far, despite promising results even in clinically relevant, resuscitated large animal models (47, 48, 106–110), none have made their way into clinical practice. Other strategies have targeted restoration of cytochrome-c-oxidase (Complex IV) activity (111, 112), maintenance of mitochondrial inner membrane integrity (113), activation of autophagy to clear damaged mitochondria to promote biogenesis (114), direct activation of mitochondrial biogenesis (95), and modulation of mitochondrial respiration using gaseous mediators (115, 116). To date, only experimental evidence is available for all of the latter strategies. In contrast, tight blood glucose control preserved both mitochondrial function and ultrastructure, and, thereby, attenuated organ dysfunction independently of organ perfusion in a rabbit model of prolonged critical illness (85). The protective effect of this strategy was associated with better maintenance of mitochondrial activity and morphological integrity in patients (117).
In the context of mitochondrial dysfunction-induced organ failure, the “decatecholaminization” paradigm (118, 119) may assume particular importance. It is well-established that catecholamines, beyond their effects of hemodynamics, have profound immune- and metabolism-modulating properties (120, 121), ultimately resulting in “metabolic stress” (122), the degree of which is directly related to their β-adrenergic activity (123). Catecholamines inhibit cellular respiration in vitro in a dose-dependent manner (124, 125). In clinically relevant, resuscitated, large animal models, the degree of impaired mitochondrial respiration and, ultimately, organ dysfunction was directly related to the norepinephrine infusion rates required to achieve hemodynamic targets (55, 89). It is tempting to speculate that this finding is related to the well-known norepinephrine-related aggravation of oxidative (126, 127) and nitrosative stress: in patients, nitrite/nitrate concentrations were inversely related to both tissue complex I activity and glutathione concentrations, but directly related to norepinephrine requirements which, in turn, coincided with low complex I activities (14).
Both microvascular and mitochondrial alterations are consequences of circulatory shock and associated with worse outcomes. However, none of the promising microvasculature- or mitochondrial-targeted pre-clinical therapeutic approaches have yet translated to clinical practice. The challenges of assessing microvascular and/or mitochondrial function in patients limit the current understanding in this field. Simultaneous measurements of both parameters, such as recently performed by Rutai et al. (128) in a pre-clinical study, might help to alleviate this issue. To date, it remains unclear whether any of the microcirculation therapies currently under investigation will be successful in abolishing shock-induced flow heterogeneity, rather than having beneficial effects through their anti-inflammatory and/or anti-oxidant properties. Similarly, mitochondrial dysfunction may result in organ failure despite adequate tissue perfusion and oxygenation. Therefore, a crucial question to address in the future is: how, when and in whom should we protect/support the mitochondria?
The original contributions presented in the study are included in the article/supplementary material, further inquiries can be directed to the corresponding author/s.
PR envisioned, drafted, and corrected the final version of the manuscript. TM drafted, created the figures, and edited the final version of the manuscript. MS contributed to the draft and edited the final version of the manuscript. ND, OM, and MH-L edited the manuscript. All authors read and approved the final version.
TM, PR, and MH-L received funding of the Deutsche Forschungsgemeinschaft (DFG, German Research Foundation)—Project-ID 251293561—Collaborative Research Center (CRC) 1149. PR received funding of the Deutsche Forschungsgemeinschaft (DFG, German Research Foundation) and GRK 2203 (PulmoSens).
The authors declare that the research was conducted in the absence of any commercial or financial relationships that could be construed as a potential conflict of interest.
1. Vincent JL, De Backer D. Circulatory shock. N Engl J Med. (2013) 369:1726–34. doi: 10.1056/NEJMra1208943
2. Elbers PW, Ince C. Mechanisms of critical illness–classifying microcirculatory flow abnormalities in distributive shock. Crit Care. (2006) 10:221. doi: 10.1186/cc4969
3. De Backer D, Ortiz JA, Salgado D. Coupling microcirculation to systemic hemodynamics. Curr Opin Crit Care. (2010) 16:250–4. doi: 10.1097/MCC.0b013e3283383621
4. Ince C, Guerci P. Why and when the microcirculation becomes disassociated from the macrocirculation. Intensive Care Med. (2016) 42:1645–6. doi: 10.1007/s00134-016-4494-1
5. De Backer D, Creteur J, Preiser JC, Dubois MJ, Vincent JL. Microvascular blood flow is altered in patients with sepsis. Am J Respir Crit Care Med. (2002) 166:98–104. doi: 10.1164/rccm.200109-016OC
6. Hutchings SD, Naumann DN, Hopkins P, Mellis C, Riozzi P, Sartini S, et al. Microcirculatory impairment is associated with multiple organ dysfunction following traumatic hemorrhagic shock: The MICROSHOCK Study. Crit Care Med. (2018) 46:e889–96. doi: 10.1097/CCM.0000000000003275
7. De Backer D, Donadello K, Sakr Y, Ospina-Tascon G, Salgado D, Scolletta S, et al. Microcirculatory alterations in patients with severe sepsis: impact of time of assessment and relationship with outcome. Crit Care Med. (2013) 41:791–9. doi: 10.1097/CCM.0b013e3182742e8b
8. Massey MJ, Hou PC, Filbin M, Wang H, Ngo L, Huang DT, et al. Microcirculatory perfusion disturbances in septic shock: results from the ProCESS trial. Crit Care. (2018). 22:308. doi: 10.1186/s13054-018-2240-5
9. Legrand M, Ait-Oufella H, Ince C. Could resuscitation be based on microcirculation data? Yes. Intensive Care Med. (2018) 44:944–6. doi: 10.1007/s00134-018-5121-0
10. Bateman RM, Walley KR. Microvascular resuscitation as a therapeutic goal in severe sepsis. Crit Care. (2005) 9(Suppl. 4):S27–32. doi: 10.1186/cc3756
11. Monnet X, Saugel B. Could resuscitation be based on microcirculation data? We are not sure. Intensive Care Med. (2018) 44:950–3. doi: 10.1007/s00134-018-5180-2
12. Naumann DN, Lima A. Could resuscitation be based on microcirculation data? No. Intensive Care Med. (2018) 44:947–9. doi: 10.1007/s00134-018-5095-y
13. Trzeciak S, Glaspey LJ, Dellinger RP, Durflinger P, Anderson K, Dezfulian C, et al. Randomized controlled trial of inhaled nitric oxide for the treatment of microcirculatory dysfunction in patients with sepsis. Crit Care Med. (2014) 42:2482–92. doi: 10.1097/CCM.0000000000000549
14. Brealey D, Brand M, Hargreaves I, Heales S, Land J, Smolenski R, et al. Association between mitochondrial dysfunction and severity and outcome of septic shock. Lancet. (2002) 360:219–23. doi: 10.1016/S0140-6736(02)09459-X
15. Bar-Or D, Carrick MM, Mains CW, Rael LT, Slone D, Brody EN. Sepsis, oxidative stress, and hypoxia: are there clues to better treatment? Redox Rep. (2015) 20:193–7. doi: 10.1179/1351000215Y.0000000005
16. Østergaard L, Granfeldt A, Secher N, Tietze A, Iversen NK, Jensen MS, et al. Microcirculatory dysfunction and tissue oxygenation in critical illness. Acta Anaesthesiol Scand. (2015) 59:1246–59. doi: 10.1111/aas.12581
17. Tugtekin IF, Radermacher P, Theisen M, Matejovic M, Stehr A, Ploner F, et al. Increased ileal-mucosal-arterial PCO2 gap is associated with impaired villus microcirculation in endotoxic pigs. Intensive Care Med. (2001) 27:757–66. doi: 10.1007/s001340100871
18. Benes J, Chvojka J, Sykora R, Radej J, Krouzecky A, Novak I, et al. Searching for mechanisms that matter in early septic acute kidney injury: an experimental study. Crit Care. (2011) 15:R256. doi: 10.1186/cc10517
19. Chvojka J, Sykora R, Krouzecky A, Radej J, Varnerova V, Karvunidis T, et al. Renal haemodynamic, microcirculatory, metabolic and histopathological responses to peritonitis-induced septic shock in pigs. Crit Care. (2008) 12:R164. doi: 10.1186/cc7164
20. Porta F, Takala J, Weikert C, Bracht H, Kolarova A, Lauterburg BH, et al. Effects of prolonged endotoxemia on liver, skeletal muscle and kidney mitochondrial function. Crit Care. (2006) 10:R118. doi: 10.1186/cc5013
21. He X, Su F, Xie K, Taccone FS, Donadello K, Vincent JL. Should hyperoxia be avoided during sepsis? An experimental study in ovine peritonitis. Crit Care Med. (2017) 45:e1060–7. doi: 10.1097/CCM.0000000000002524
22. Taccone FS, Su F, De Deyne C, Abdellhai A, Pierrakos C, He X, et al. Sepsis is associated with altered cerebral microcirculation and tissue hypoxia in experimental peritonitis. Crit Care Med. (2014) 42:e114–22. doi: 10.1097/CCM.0b013e3182a641b8
23. Ellis CG, Bateman RM, Sharpe MD, Sibbald WJ, Gill R. Effect of a maldistribution of microvascular blood flow on capillary O(2) extraction in sepsis. Am J Physiol Heart Circ Physiol. (2002) 282:H156–64. doi: 10.1152/ajpheart.2002.282.1.H156
24. Sakr Y, Dubois MJ, De Backer D, Creteur J, Vincent JL. Persistent microcirculatory alterations are associated with organ failure and death in patients with septic shock. Crit Care Med. (2004) 32:1825–31. doi: 10.1097/01.CCM.0000138558.16257.3F
25. Trzeciak S, Dellinger RP, Parrillo JE, Guglielmi M, Bajaj J, Abate NL, et al. Microcirculatory alterations in resuscitation and shock investigators. early microcirculatory perfusion derangements in patients with severe sepsis and septic shock: relationship to hemodynamics, oxygen transport, and survival. Ann Emerg Med. (2007). 49:88–98, 98.e1–2. doi: 10.1016/j.annemergmed.2006.08.021
26. Trzeciak S, McCoy JV, Phillip Dellinger R, Arnold RC, Rizzuto M, Abate NL, et al. Microcirculatory alterations in resuscitation and Shock (MARS) investigators. Early increases in microcirculatory perfusion during protocol-directed resuscitation are associated with reduced multi-organ failure at 24 h in patients with sepsis. Intensive Care Med. (2008) 34:2210–7. doi: 10.1007/s00134-008-1193-6
27. Boerma EC, Koopmans M, Konijn A, Kaiferova K, Bakker AJ, van Roon EN, et al. Effects of nitroglycerin on sublingual microcirculatory blood flow in patients with severe sepsis/septic shock after a strict resuscitation protocol: a double-blind randomized placebo controlled trial. Crit Care Med. (2010) 38:93–100. doi: 10.1097/CCM.0b013e3181b02fc1
28. Ince C, Boerma EC, Cecconi M, De Backer D, Shapiro NI, Duranteau J, et al. Second consensus on the assessment of sublingual microcirculation in critically ill patients: results from a task force of the european society of intensive care medicine. Intensive Care Med. (2018) 44:281–99. doi: 10.1007/s00134-018-5070-7
29. Boekstegers P1, Weidenhöfer S, Kapsner T, Werdan K. Skeletal muscle partial pressure of oxygen in patients with sepsis. Crit Care Med. (1994) 22:640–50. doi: 10.1097/00003246-199404000-00021
30. Obonyo NG, Fanning JP, Ng AS, Pimenta LP, Shekar K, Platts DG, et al. Effects of volume resuscitation on the microcirculation in animal models of lipopolysaccharide sepsis: a systematic review. Intensive Care Med Exp. (2016) 4:38. doi: 10.1186/s40635-016-0112-3
31. Hutchings S, Naumann DN, Harris T, Wendon J, Midwinter MJ. Observational study of the effects of traumatic injury, haemorrhagic shock and resuscitation on the microcirculation: a protocol for the MICROSHOCK study. BMJ Open. (2016) 6:e010893. doi: 10.1136/bmjopen-2015-010893
32. Orbegozo D, Su F, Santacruz C, He X, Hosokawa K, Creteur J, et al. Effects of different crystalloid solutions on hemodynamics, peripheral perfusion, and the microcirculation in experimental abdominal sepsis. Anesthesiology. (2016) 125:744–54. doi: 10.1097/ALN.0000000000001273
33. Ferrara G, Kanoore Edul VS, Caminos Eguillor JF, Buscetti MG, Canales HS, Lattanzio B, et al. Effects of fluid and norepinephrine resuscitation in a sheep model of endotoxin shock and acute kidney injury. J Appl Physiol. (2019). 127:788–97. doi: 10.1152/japplphysiol.00172.2019
34. Arnemann PH, Hessler M, Kampmeier T, Seidel L, Malek Y, Van Aken H, et al. Resuscitation with hydroxyethyl starch maintains hemodynamic coherence in ovine hemorrhagic shock. Anesthesiology. (2020) 132:131–9. doi: 10.1097/ALN.0000000000002998
35. Ospina-Tascón GA, García Marin AF, Echeverri GJ, Bermudez WF, Madriñán-Navia H, Valencia JD, et al. Effects of dobutamine on intestinal microvascular blood flow heterogeneity and O2 extraction during septic shock. J Appl Physiol. (2017). 122:1406–17. doi: 10.1152/japplphysiol.00886.2016
36. Fries M, Ince C, Rossaint R, Bleilevens C, Bickenbach J, Rex S, et al. Levosimendan but not norepinephrine improves microvascular oxygenation during experimental septic shock. Crit Care Med. (2008) 36:1886–91. doi: 10.1097/CCM.0b013e31817cede9
37. de Miranda ML, Pereira SJ, Santos AO, Villela NR, Kraemer-Aguiar LG, Bouskela E. Milrinone attenuates arteriolar vasoconstriction and capillary perfusion deficits on endotoxemic hamsters. PLoS ONE. (2015) 10:e0117004. doi: 10.1371/journal.pone.0117004
38. Johannes T, Mik EG, Klingel K, Goedhart PT, Zanke C, Nohé B, et al. Effects of 1400W and/or nitroglycerin on renal oxygenation and kidney function during endotoxaemia in anaesthetized rats. Clin Exp Pharmacol Physiol. (2009) 36:870–9. doi: 10.1111/j.1440-1681.2009.05204.x
39. Siegemund M, Van Bommel J, Sinaasappel M, Schwarte LA, Studer W, Girard T, et al. The NO donor SIN-1 improves intestinal-arterial P(CO(2)) gap in experimental endotoxemia: an animal study. Acta Anaesthesiol Scand. (2007) 51:693–700. doi: 10.1111/j.1399-6576.2007.01334.x
40. Johannes T, Ince C, Klingel K, Unertl KE, Mik EG. Iloprost preserves renal oxygenation and restores kidney function in endotoxemia-related acute renal failure in the rat. Crit Care Med. (2009) 37:1423–32. doi: 10.1097/CCM.0b013e31819b5f4e
41. Truse R, Hinterberg J, Schulz J, Herminghaus A, Weber A, Mettler-Altmann T, et al. Effect of topical iloprost and nitroglycerin on gastric microcirculation and barrier function during hemorrhagic shock in dogs. J Vasc Res. (2017) 54:109–121. doi: 10.1159/000464262
42. Lehmann C, König JP, Dettmann J, Birnbaum J, Kox WJ. Effects of iloprost, a stable prostacyclin analog, on intestinal leukocyte adherence and microvascular blood flow in rat experimental endotoxemia. Crit Care Med. (2001) 29:1412–6. doi: 10.1097/00003246-200107000-00019
43. Guillon A, Preau S, Aboab J, Azabou E, Jung B, Silva S, et al. Preclinical septic shock research: why we need an animal ICU. Ann Intensive Care. (2019). 9:66. doi: 10.1186/s13613-019-0543-6
44. Brown GC. Regulation of mitochondrial respiration by nitric oxide inhibition of cytochrome c oxidase. Biochim Biophys Acta. (2001) 1504:46–57. doi: 10.1016/S0005-2728(00)00238-3
45. Sarti P, Forte E, Mastronicola D, Giuffrè A, Arese M. Cytochrome C oxidase and nitric oxide in action: molecular mechanisms and pathophysiological implications. Biochim Biophys Acta. (2012) 1817:610–9. doi: 10.1016/j.bbabio.2011.09.002
46. Poderoso JJ, Helfenberger K, Poderoso C. The effect of nitric oxide on mitochondrial respiration. Nitric Oxide. (2019) 88:61–72. doi: 10.1016/j.niox.2019.04.005
47. Matejovic M, Krouzecky A, Martinkova V, Rokyta R Jr, Kralova H, Treska V, et al. Selective inducible nitric oxide synthase inhibition during long-term hyperdynamic porcine bacteremia. Shock. (2004) 21:458–65. doi: 10.1097/00024382-200405000-00010
48. Matejovic M, Krouzecky A, Martinkova V, Rokyta R Jr, Radej J, Kralova H, et al. Effects of tempol, a free radical scavenger, on long-term hyperdynamic porcine bacteremia. Crit Care Med. (2005) 33:1057–63. doi: 10.1097/01.CCM.0000162927.94753.63
49. Pittner A, Nalos M, Asfar P, Yang Y, Ince C, Georgieff M, et al. Mechanisms of inducible nitric oxide synthase (iNOS) inhibition-related improvement of gut mucosal acidosis during hyperdynamic porcine endotoxemia. Intensive Care Med. (2003) 29:312–6. doi: 10.1007/s00134-002-1577-y
50. Träger K, Matejovic M, Zülke C, Vlatten A, Vogt J, Wachter U, et al. Hepatic O2 exchange and liver energy metabolism in hyperdynamic porcine endotoxemia: effects of iloprost. Intensive Care Med. (2000) 26:1531–9. doi: 10.1007/s001340000645
51. Ospina-Tascon G, Neves AP, Occhipinti G, Donadello K, Büchele G, Simion D, et al. Effects of fluids on microvascular perfusion in patients with severe sepsis. Intensive Care Med. (2010) 36:949–55. doi: 10.1007/s00134-010-1843-3
52. De Backer D, Creteur J, Dubois MJ, Sakr Y, Koch M, Verdant C, et al. The effects of dobutamine on microcirculatory alterations in patients with septic shock are independent of its systemic effects. Crit Care Med. (2006) 34:403–8. doi: 10.1097/01.CCM.0000198107.61493.5A
53. Hernandez G, Bruhn A, Luengo C, Regueira T, Kattan E, Fuentealba A, et al. Effects of dobutamine on systemic, regional and microcirculatory perfusion parameters in septic shock: a randomized, placebo-controlled, double-blind, crossover study. Intensive Care Med. (2013) 39:1435–43. doi: 10.1007/s00134-013-2982-0
54. Buwalda M, Ince C. Opening the microcirculation: can vasodilators be useful in sepsis? Intensive Care Med. (2002) 28:1208–17. doi: 10.1007/s00134-002-1407-2
55. Corrêa TD, Pereira AJ, Brandt S, Vuda M, Djafarzadeh S, Takala J, et al. Time course of blood lactate levels, inflammation, and mitochondrial function in experimental sepsis. Crit Care. (2017) 21:105. doi: 10.1186/s13054-017-1691-4
56. Legrand M, De Backer D, Dépret F, Ait-Oufella H. Recruiting the microcirculation in septic shock. Ann Intensive Care. (2019) 9:102. doi: 10.1186/s13613-019-0581-0
57. Spronk PE, Ince C, Gardien MJ, Mathura KR, Oudemans-van Straaten HM, Zandstra DF. Nitroglycerin in septic shock after intravascular volume resuscitation. Lancet. (2002) 360:1395–6. doi: 10.1016/S0140-6736(02)11393-6
58. Pittet JF, Lacroix JS, Gunning K, Laverriere MC, Morel DR, Suter PM. Prostacyclin but not phentolamine increases oxygen consumption and skin microvascular blood flow in patients with sepsis and respiratory failure. Chest. (1990) 98:1467–72. doi: 10.1378/chest.98.6.1467
59. Dépret F1, Sitbon A, Soussi S, De Tymowski C, Blet A, Fratani A, et al. Intravenous iloprost to recruit the microcirculation in septic shock patients? Intensive Care Med. (2018) 44:121–2. doi: 10.1007/s00134-017-4935-5
60. De Backer D. Is there a place for prostacyclin in the treatment of septic shock? Intensive Care Med. (2001) 27:1110–2. doi: 10.1007/s001340100988
61. Bihari DJ. Indomethacin and arterial oxygenation in critically ill patients with severe bacterial pneumonia. Lancet. (1987) 1:755. doi: 10.1016/S0140-6736(87)90405-3
62. Scheeren T, Susanto F, Reinauer H, Tarnow J, Radermacher P. Prostacyclin improves glucose utilization in patients with sepsis. J Crit Care. (1994) 9:175–84. doi: 10.1016/0883-9441(94)90014-0
63. Radermacher P, Buhl R, Santak B, Klein M, Kniemeyer HW, Becker H, et al. The effects of prostacyclin on gastric intramucosal pH in patients with septic shock. Intensive Care Med. (1995) 21:414–21. doi: 10.1007/BF01707410
64. Eichelbrönner O, Reinelt H, Wiedeck H, Mezödy M, Rossaint R, Georgieff M, et al. Aerosolized prostacyclin and inhaled nitric oxide in septic shock–different effects on splanchnic oxygenation? Intensive Care Med. (1996) 22:880–7. doi: 10.1007/BF02044111
65. Kiefer P, Tugtekin I, Wiedeck H, Bracht H, Vogt J, Wachter U, et al. Hepato-splanchnic metabolic effects of the stable prostacyclin analogue iloprost in patients with septic shock. Intensive Care Med. (2001) 27:1179–86. doi: 10.1007/s001340100954
66. van Loon LM, Stolk RF, van der Hoeven JG, Veltink PH, Pickkers P, Lemson J, et al. Effect of vasopressors on the macro- and microcirculation during systemic inflammation in humans in vivo. Shock. (2020) 53:171–4. doi: 10.1097/SHK.0000000000001357
67. Potter EK, Hodgson L, Creagh-Brown B, Forni LG. Manipulating the microcirculation in sepsis - the impact of vasoactive medications on microcirculatory blood flow: a systematic review. Shock. (2019) 52:5–12. doi: 10.1097/SHK.0000000000001239
68. Fink M. Cytopathic hypoxia in sepsis. Acta Anaesthesiol Scand Suppl. (1997) 110:87–95. doi: 10.1111/j.1399-6576.1997.tb05514.x
69. Fink MP. Bench-to-bedside review: cytopathic hypoxia. Crit Care. (2002) 6:491–9. doi: 10.1186/cc1824
70. Curtis SE, Cain SM. Regional and systemic oxygen delivery/uptake relations and lactate flux in hyperdynamic, endotoxin-treated dogs. Am Rev Respir Dis. (1992) 145:348–54. doi: 10.1164/ajrccm/145.2_Pt_1.348
71. Suetrong B, Walley KR. Lactic acidosis in sepsis: it's not all anaerobic: implications for diagnosis and management. Chest. (2016) 149:252–61. doi: 10.1378/chest.15-1703
72. Hotchkiss RS, Swanson PE, Freeman BD, Tinsley KW, Cobb JP, Matuschak GM, et al. Apoptotic cell death in patients with sepsis, shock, and multiple organ dysfunction. Crit Care Med. (1999) 27:1230–51. doi: 10.1097/00003246-199907000-00002
73. Takasu O, Gaut JP, Watanabe E, To K, Fagley RE, Sato B, et al. Mechanisms of cardiac and renal dysfunction in patients dying of sepsis. Am J Respir Crit Care Med. (2013) 187:509–17. doi: 10.1164/rccm.201211-1983OC
74. Brealey D, Karyampudi S, Jacques TS, Novelli M, Stidwill R, Taylor V, et al. Mitochondrial dysfunction in a long-term rodent model of sepsis and organ failure. Am J Physiol Regul Integr Comp Physiol. (2004) 286:R491–7. doi: 10.1152/ajpregu.00432.2003
75. Levy RJ, Deutschman CS. Cytochrome c oxidase dysfunction in sepsis. Crit Care Med. (2007) 35(Suppl. 9):S468–75. doi: 10.1097/01.CCM.0000278604.93569.27
76. Leverve XM. Mitochondrial function and substrate availability. Crit Care Med. (2007) 35(Suppl. 9):S454–60. doi: 10.1097/01.CCM.0000278044.19217.73
77. Wefers Bettink MA, Harms FA, Dollee N, Specht PAC, Raat NJH, Schoonderwoerd GC, et al. Non-invasive versus ex vivo measurement of mitochondrial function in an endotoxemia model in rat: toward monitoring of mitochondrial therapy. Mitochondrion. (2020) 50:149–57. doi: 10.1016/j.mito.2019.11.003
78. Mik EG. Special article: measuring mitochondrial oxygen tension: from basic principles to application in humans. Anesth Analg. (2013) 117:834–46. doi: 10.1213/ANE.0b013e31828f29da
79. Neu C, Baumbach P, Plooij AK, Skitek K, Götze J, von Loeffelholz C, et al. Non-invasive assessment of mitochondrial oxygen metabolism in the critically ill patient using the protoporphyrin IX-triplet state lifetime technique-a feasibility study. Front Immunol. (2020) 11:757. doi: 10.3389/fimmu.2020.00757
80. Tsai AG, Johnson PC, Intaglietta M. Is the distribution of tissue pO(2) homogeneous? Antioxid Redox Signal. (2007) 9:979–84. doi: 10.1089/ars.2007.1633
81. Connett RJ, Honig CR, Gayeski TE, Brooks GA. Defining hypoxia: a systems view of VO2, glycolysis, energetics, and intracellular PO2. J Appl Physiol (1985). (1990) 68:833–42. doi: 10.1152/jappl.1990.68.3.833
82. Merz T, Vogt JA, Wachter U, Calzia E, Szabo C, Wang R, et al. Impact of hyperglycemia on cystathionine-γ-lyase expression during resuscitated murine septic shock. Intensive Care Med Exp. (2017) 5:30. doi: 10.1186/s40635-017-0140-7
83. Arulkumaran N, Pollen S, Greco E, Courtneidge H, Hall AM, Duchen MR, et al. Renal tubular cell mitochondrial dysfunction occurs despite preserved renal oxygen delivery in experimental septic acute kidney injury. Crit Care Med. (2018) 46:e318–25. doi: 10.1097/CCM.0000000000002937
84. Rosser BG, Gores GJ. Liver cell necrosis: cellular mechanisms and clinical implications. Gastroenterology. (1995) 108:252–75. doi: 10.1016/0016-5085(95)90032-2
85. Vanhorebeek I, Gunst J, Ellger B, Boussemaere M, Lerut E, Debaveye Y, et al. Hyperglycemic kidney damage in an animal model of prolonged critical illness. Kidney Int. (2009) 76:512–20. doi: 10.1038/ki.2009.217
86. Gellerich FN, Trumbeckaite S, Hertel K, Zierz S, Müller-Werdan U, Werdan K, et al. Impaired energy metabolism in hearts of septic baboons: diminished activities of complex i and complex II of the mitochondrial respiratory chain. Shock. (1999) 11:336–41. doi: 10.1097/00024382-199905000-00006
87. Crouser ED, Julian MW, Blaho DV, Pfeiffer DR. Endotoxin-induced mitochondrial damage correlates with impaired respiratory activity. Crit Care Med. (2002) 30:276–84. doi: 10.1097/00003246-200202000-00002
88. Regueira T, Djafarzadeh S, Brandt S, Gorrasi J, Borotto E, Porta F, et al. Oxygen transport and mitochondrial function in porcine septic shock, cardiogenic shock, and hypoxaemia. Acta Anaesthesiol Scand. (2012) 56:846–59. doi: 10.1111/j.1399-6576.2012.02706.x
89. Merz T, Wepler M, Nußbaum B, Vogt J, Calzia E, Wang R, et al. Cystathionine-γ-lyase expression is associated with mitochondrial respiration during sepsis-induced acute kidney injury in swine with atherosclerosis. Intensive Care Med Exp. (2018) 6:43. doi: 10.1186/s40635-018-0208-z
90. Jeger V, Djafarzadeh S, Jakob SM, Takala J. Mitochondrial function in sepsis. Eur J Clin Invest. (2013) 43:532–42. doi: 10.1111/eci.12069
91. Carré JE, Orban JC, Re L, Felsmann K, Iffert W, Bauer M, et al. Survival in critical illness is associated with early activation of mitochondrial biogenesis. Am J Respir Crit Care Med. (2010) 182:745–51. doi: 10.1164/rccm.201003-0326OC
92. Asfar P, Schortgen F, Boisramé-Helms J, Charpentier J, Guérot E, Megarbane B, et al. REVA research network. Hyperoxia and hypertonic saline in patients with septic shock (HYPERS2S): a two-by-two factorial, multicentre, randomised, clinical trial. Lancet Respir Med. (2017) 5:180–90. doi: 10.1016/S2213-2600(17)30046-2
93. Dyson A, Simon F, Seifritz A, Zimmerling O, Matallo J, Calzia E, et al. Bladder tissue oxygen tension monitoring in pigs subjected to a range of cardiorespiratory and pharmacological challenges. Intensive Care Med. (2012) 38:1868–76. doi: 10.1007/s00134-012-2712-z
94. Demiselle J, Wepler M, Hartmann C, Radermacher P, Schortgen F, Meziani F, et al. Hyperoxia toxicity in septic shock patients according to the Sepsis-3 criteria: a post hoc analysis of the HYPER2S trial. Ann Intensive Care. (2018). 8:90. doi: 10.1186/s13613-018-0435-1
95. Supinski GS, Schroder EA, Callahan LA. Mitochondria and critical illness. Chest. (2020) 157:310–22. doi: 10.1016/j.chest.2019.08.2182
96. Arulkumaran N, Deutschman CS, Pinsky MR, Zuckerbraun B, Schumacker PT, Gomez H, et al. ADQI XIV workgroup. mitochondrial function in sepsis. Shock. (2016) 45:271–81. doi: 10.1097/SHK.0000000000000463
97. Mantzarlis K, Tsolaki V, Zakynthinos E. Role of oxidative stress and mitochondrial dysfunction in sepsis and potential therapies. Oxid Med Cell Longev. (2017) 2017:5985209. doi: 10.1155/2017/5985209
98. Protti A, Singer M. Bench-to-bedside review: potential strategies to protect or reverse mitochondrial dysfunction in sepsis-induced organ failure. Crit Care. (2006) 10:228. doi: 10.1186/cc5014
99. Detaille D, Guigas B, Chauvin C, Batandier C, Fontaine E, Wiernsperger N, et al. Metformin prevents high-glucose-induced endothelial cell death through a mitochondrial permeability transition-dependent process. Diabetes. (2005) 54:2179–87. doi: 10.2337/diabetes.54.7.2179
100. Vogt JA, Wachter U, Wagner K, Calzia E, Gröger M, Weber S, et al. Effects of glycemic control on glucose utilization and mitochondrial respiration during resuscitated murine septic shock. Intensive Care Med Exp. (2014) 2:19. doi: 10.1186/2197-425X-2-19
101. Detaille D, Vial G, Borel AL, Cottet-Rouselle C, Hallakou-Bozec S, Bolze S, et al. Imeglimin prevents human endothelial cell death by inhibiting mitochondrial permeability transition without inhibiting mitochondrial respiration. Cell Death Discov. (2016) 2:15072. doi: 10.1038/cddiscovery.2015.72
102. Larche J, Lancel S, Hassoun SM, Favory R, Decoster B, Marchetti P, et al. Inhibition of mitochondrial permeability transition prevents sepsis-induced myocardial dysfunction and mortality. J Am Coll Cardiol. (2006) 48:377–85. doi: 10.1016/j.jacc.2006.02.069
103. Karlsson M, Pukenas B, Chawla S, Ehinger JK, Plyler R, Stolow M, et al. Neuroprotective effects of cyclosporine in a porcine pre-clinical trial of focal traumatic brain injury. J Neurotrauma. (2018) 36:14–24. doi: 10.1089/neu.2018.5706
104. Argaud L, Cour M, Dubien PY, Giraud F, Jossan C, Riche B, et al. Effect of cyclosporine in nonshockable out-of-hospital cardiac arrest: the CYRUS randomized clinical trial. JAMA Cardiol. (2016). 1:557–65. doi: 10.1001/jamacardio.2016.1701
105. Cung TT, Morel O, Cayla G, Rioufol G, Garcia-Dorado D, Angoulvant D, et al. Cyclosporine before PCI in patients with acute myocardial infarction. N Engl J Med. (2015) 373:1021–31. doi: 10.1056/NEJMoa1505489
106. Matejovic M, Krouzecky A, Rokyta R Jr, Radej J, Kralova H, Treska V, et al. Effects of combining inducible nitric oxide synthase inhibitor and radical scavenger during porcine bacteremia. Shock. (2007) 27:61–8. doi: 10.1097/01.shk.0000235088.53421.6f
107. Soejima K, McGuire R, Snyder N IV, Uchida T, Szabó C, Salzman A, et al. The effect of inducible nitric oxide synthase (iNOS) inhibition on smoke inhalation injury in sheep. Shock. (2000) 13:261–6. doi: 10.1097/00024382-200004000-00002
108. Yamamoto Y, Sousse LE, Enkhbaatar P, Kraft ER, Deyo DJ, Wright CL, et al. γ-tocopherol nebulization decreases oxidative stress, arginase activity, and collagen deposition after burn and smoke inhalation in the ovine model. Shock. (2012). 38:671–6. doi: 10.1097/SHK.0b013e3182758759
109. Enkhbaatar P, Murakami K, Shimoda K, Mizutani A, Traber L, Phillips GB, et al. The inducible nitric oxide synthase inhibitor BBS-2 prevents acute lung injury in sheep after burn and smoke inhalation injury. Am J Respir Crit Care Med. (2003) 167:1021–6. doi: 10.1164/rccm.200209-1031PP
110. Su F, Huang H, Akieda K, Occhipinti G, Donadello K, Piagnerelli M, et al. Effects of a selective iNOS inhibitor versus norepinephrine in the treatment of septic shock. Shock. (2010) 34:243–9. doi: 10.1097/SHK.0b013e3181d75967
111. Piel DA, Gruber PJ, Weinheimer CJ, Courtois MR, Robertson CM, Coopersmith CM, et al. Mitochondrial resuscitation with exogenous cytochrome c in the septic heart. Crit Care Med. (2007) 35:2120–7. doi: 10.1097/01.CCM.0000278914.85340.FE
112. Verma R, Huang Z, Deutschman CS, Levy RJ. Caffeine restores myocardial cytochrome oxidase activity and improves cardiac function during sepsis. Crit Care Med. (2009) 37:1397–402. doi: 10.1097/CCM.0b013e31819cecd6
113. Crouser ED. Respiratory failure during critical illness: are mitochondria to blame? Am J Respir Crit Care Med. (2005) 172:793–4. doi: 10.1164/rccm.2507005
114. Sun Y, Yao X, Zhang QJ, Zhu M, Liu ZP, Ci B, et al. Beclin-1-Dependent autophagy protects the heart during sepsis. Circulation. (2018) 138:2247–62. doi: 10.1161/CIRCULATIONAHA.117.032821
115. Hartmann C, Nussbaum B, Calzia E, Radermacher P, Wepler M. Gaseous mediators and mitochondrial function: the future of pharmacologically induced suspended animation? Front Physiol. (2017) 8:691. doi: 10.3389/fphys.2017.00691
116. Dyson A, Dal-Pizzol F, Sabbatini G, Lach AB, Galfo F, Dos Santos Cardoso J, et al. Ammonium tetrathiomolybdate following ischemia/reperfusion injury: chemistry, pharmacology, and impact of a new class of sulfide donor in preclinical injury models. PLoS Med. (2017) 14:e1002310. doi: 10.1371/journal.pmed.1002310
117. Vanhorebeek I, De Vos R, Mesotten D, Wouters PJ, De Wolf-Peeters C, Van den Berghe G. Protection of hepatocyte mitochondrial ultrastructure and function by strict blood glucose control with insulin in critically ill patients. Lancet. (2005) 365:53–9. doi: 10.1016/S0140-6736(04)17665-4
118. Singer M, Matthay MA. Clinical review: thinking outside the box–an iconoclastic view of current practice. Crit Care. (2011) 15:225. doi: 10.1186/cc10245
119. Rudiger A, Singer M. Decatecholaminisation during sepsis. Crit Care. (2016) 20:309. doi: 10.1186/s13054-016-1488-x
120. Andreis DT, Singer M3. Catecholamines for inflammatory shock: a Jekyll-and-Hyde conundrum. Intensive Care Med. (2016) 42:1387–97. doi: 10.1007/s00134-016-4249-z
121. Hartmann C, Radermacher P, Wepler M, Nußbaum B. Non-Hemodynamic effects of catecholamines. Shock. (2017) 48:390–400. doi: 10.1097/SHK.0000000000000879
122. Mizock BA. Alterations in carbohydrate metabolism during stress: a review of the literature. Am J Med. (1995) 98:75–84. doi: 10.1016/S0002-9343(99)80083-7
123. De Backer D, Creteur J, Silva E, Vincent JL. Effects of dopamine, norepinephrine, and epinephrine on the splanchnic circulation in septic shock: which is best? Crit Care Med. (2003) 31:1659–67. doi: 10.1097/01.CCM.0000063045.77339.B6
124. Lünemann JD, Buttgereit F, Tripmacher R, Baerwald CG, Burmester GR, Krause A. Effects of norepinephrine on oxygen consumption of quiescent and activated human peripheral blood mononuclear cells. Ann N Y Acad Sci. (2002) 966:365–8. doi: 10.1111/j.1749-6632.2002.tb04236.x
125. Porta F, Bracht H, Weikert C, Beck M, Takala J, Brandt S, et al. Effects of endotoxin and catecholamines on hepatic mitochondrial respiration. Inflammation. (2009) 32:315–21. doi: 10.1007/s10753-009-9138-y
126. Rump AF, Klaus W. Evidence for norepinephrine cardiotoxicity mediated by superoxide anion radicals in isolated rabbit hearts. Naunyn Schmiedebergs Arch Pharmacol. (1994) 349:295–300. doi: 10.1007/BF00169296
127. Neri M, Cerretani D, Fiaschi AI, Laghi PF, Lazzerini PE, Maffione AB, et al. Correlation between cardiac oxidative stress and myocardial pathology due to acute and chronic norepinephrine administration in rats. J Cell Mol Med. (2007) 11:156–70. doi: 10.1111/j.1582-4934.2007.00009.x
Keywords: circulatory shock, oxidative stress, hypoxia, organ failure, inflammation
Citation: Merz T, Denoix N, Huber-Lang M, Singer M, Radermacher P and McCook O (2020) Microcirculation vs. Mitochondria—What to Target? Front. Med. 7:416. doi: 10.3389/fmed.2020.00416
Received: 19 May 2020; Accepted: 29 June 2020;
Published: 05 August 2020.
Edited by:
Mihaly Boros, University of Szeged, HungaryCopyright © 2020 Merz, Denoix, Huber-Lang, Singer, Radermacher and McCook. This is an open-access article distributed under the terms of the Creative Commons Attribution License (CC BY). The use, distribution or reproduction in other forums is permitted, provided the original author(s) and the copyright owner(s) are credited and that the original publication in this journal is cited, in accordance with accepted academic practice. No use, distribution or reproduction is permitted which does not comply with these terms.
*Correspondence: Tamara Merz, dGFtYXJhLm1lcnpAdW5pLXVsbS5kZQ==
Disclaimer: All claims expressed in this article are solely those of the authors and do not necessarily represent those of their affiliated organizations, or those of the publisher, the editors and the reviewers. Any product that may be evaluated in this article or claim that may be made by its manufacturer is not guaranteed or endorsed by the publisher.
Research integrity at Frontiers
Learn more about the work of our research integrity team to safeguard the quality of each article we publish.