- 1Laboratório de Biologia das Interações Celulares, Departamento de Morfologia, Instituto de Ciências Biológicas, Universidade Federal de Minas Gerais, Belo Horizonte, Brazil
- 2Departamento de Clínica e Cirurgia Veterinárias, Escola de Veterinária, Universidade Federal de Minas Gerais, Belo Horizonte, Brazil
- 3Departamento de Medicina, Universidade José Do Rosário Vellano, UNIFENAS, Belo Horizonte, Brazil
- 4Laboratório de Fisiologia de Insetos Hematófagos, Departamento de Parasitologia, Instituto de Ciências Biológicas, Universidade Federal de Minas Gerais, Belo Horizonte, Brazil
- 5Laboratório de Virologia Básica e Aplicada, Departamento de Microbiologia, Instituto de Ciências Biológicas, Universidade Federal de Minas Gerais, Belo Horizonte, Brazil
- 6Laboratório de Mosquitos Vetores: Endossimbiontes e Interação Patógeno-Vetor, Instituto René Rachou, Fiocruz, Belo Horizonte, Brazil
Continuous climate changes associated with the disorderly occupation of urban areas have exposed Latin American populations to the emergence and reemergence of arboviruses transmitted by Aedes aegypti. The magnitude of the financial and political problems these epidemics may bring to the future of developing countries is still ignored. Due to the lack of effective antiviral drugs and vaccines against arboviruses, the primary measure for preventing or reducing the transmission of diseases depends entirely on the control of vectors or the interruption of human-vector contact. In Brazil the first attempt to control A. aegypti took place in 1902 by eliminating artificial sites of eproduction. Other strategies, such as the use of oviposition traps and chemical control with dichlorodiphenyltrichlorethane and pyrethroids, were successful, but only for a limited time. More recently, biotechnical approaches, such as the release of transgenics or sterile mosquitoes and the, development of transmission blocking vaccines, are being applied to try to control the A. aegypti population and/or arbovirus transmission. Endemic countries spend about twice as much to treat patients as they do on the prevention of mosquito-transmitted diseases. The result of this strategy is an explosive outbreak of arboviruses cases. This review summarizes the social impacts caused by A. aegypti-transmitted diseases, mainly from a biotechnological perspective in vector control aimed at protecting Latin American populations against arboviruses.
Introduction
Mosquitoes of the Culicidae family are considered the most dangerous animals on earth due to their capacity to transmit diseases and their medical importance regarding viruses, protozoa, and nematode transmission (1). According to the Pan American Health Organization (PAHO) vector-borne diseases are considered public health problems and have a major social and economic impact causing high morbidity and mortality, especially in developing countries (2). Approximately 75% of the Latin American population lives in cities, which makes the most urbanized continent in the world (3). The magnitude of climate and ecosystem changes and disorderly occupation of urban areas may increase the vulnerability of human populations to infectious disease transmission (2). Moreover, these changes have led several vectors to adapt to the urban environment (4, 5). We list the top 15 pathogens transmitted by Culicidae in the Americas and the prevalence of viruses is evident (80%), all considered as arboviruses (Table 1). Aedes genus has drawn the attention of the world health authorities due to the severity of diseases transmitted in the last 10 years, especially Dengue, Chikungunya, and Zika fevers.
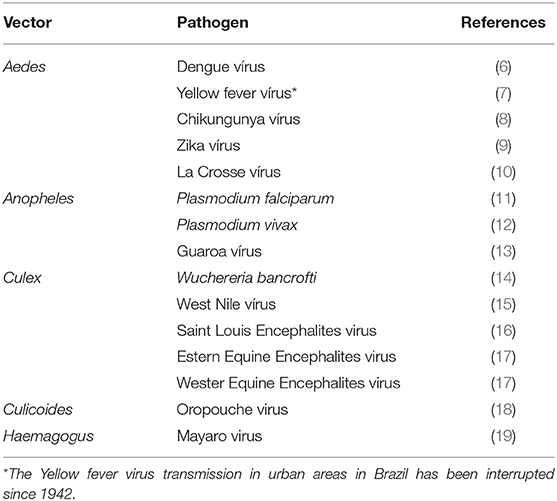
Table 1. Culicidae family includes vectors of major medical importance and the main pathogens transmitted in the Americas.
The Aedes genus has more than 950 species of mosquitoes, many of which transmit potentially deadly pathogens to humans and other animals. Among the species, Aedes aegypti is currently the main global arbovirus vector (20). Carvalho and Moreira (21) reviewed the main reasons for A. aegypti having both reproductive success and being so well-adapted to the urban environment. This mosquito lives in urban habitats and reproduces primarily in artificial containers (5, 6, 21, 22). A single female makes several blood meals during each feeding period and hematophagy occurs preferentially in humans (21). The blood meal is essential for egg maturation. During oviposition, the female spreads the eggs in many breeding sites to ensure reproductive success and can remain viable for over 1 year (20, 21). Beyond the vertical transmission, an infected female is able to transmit the arbovirus for the rest of its life after virus incubation (21). In this review, we demonstrate the impact caused by Aedes aegypti-borne diseases based on current literature, highlighting the history of vector control based on the major biotechnological approaches for preventing new arboviral epidemics.
Origin of the Main Arboviruses That Cause Human Diseases and the Role of Aedes aegypti in Their Transmission
Arbovirus (arthropod-borne virus) is the non-taxonomic term used to define a group of viruses transmitted by hematophagous arthropods (23). According to the International Catalog of Arbovirus, there are 545 species and most of the viruses registered have non-human vertebrates as the main reservoir (6, 22, 24). It is believed that approximately 150 arboviruses cause diseases in humans, most of them zoonotic, and belonging to five viral families, namely Bunyaviridae, Flaviviridae, Reoviridae, Rhabdoviridae, and Togaviridae (6, 24). In humans, clinical manifestations of arboviruses include moderate or severe febrile illness with or without hemorrhage, headache, retro-orbital pain, rash, myalgia, arthralgia, and several neurological syndromes (24). Currently, international travel and the global distribution of vectors have made arboviruses a constant threat to the human population (2, 24). The reasons for the emergence or reemergence of some diseases are not fully known, but the following mechanisms have been identified as causes of change or increases in the incidence of many diseases: altered habitat, loss of biodiversity, niche invasion or host–shifting by pathogens, human–induced genetic changes in disease vectors or pathogens, environmental contamination, and diminishing global travel times, when infected people carry pathogens before symptoms occur (2, 25, 26).
A. aegypti was the main vector of some of the most important arboviral epidemics of all time such as Yellow fever virus (YFV) and dengue virus (DENV), which resulted in millions of fatalities worldwide (6, 22, 27). In addition to the extensive history of epidemics, chikungunya virus (CHIKV) and Zika virus (ZIKV) caused serious outbreaks in the Americas continent in recent years (9, 28–30). The major arboviruses causing human diseases transmitted by Aedes mosquitoes in the Americas and their possible places of origin are described in Figure 1.
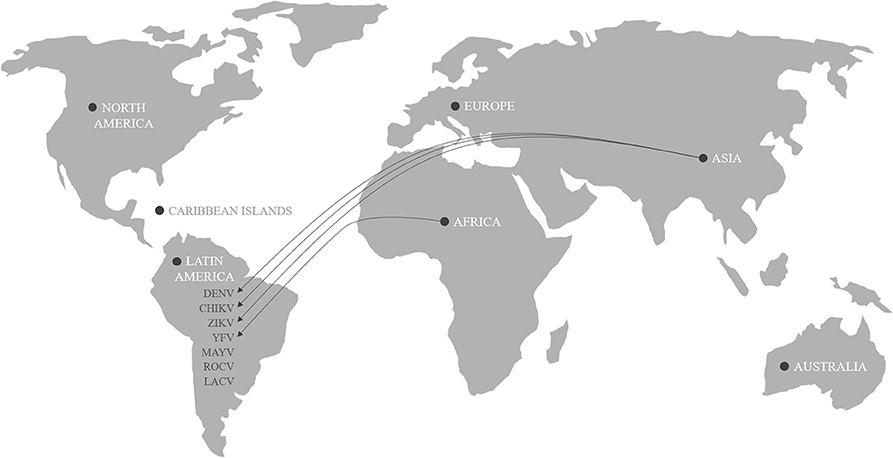
Figure 1. Origin of arboviral genotype of Dengue (DENV), Chikungunya (CHIKV), Zika (ZIKV), Yellow Fever (YFV), Mayaro (MAYV), Rocio (ROCV), and La Crosse virus (LACV) causing human diseases transmitted by Aedes aegypti.
The YFV came from West Africa and arrived in the Americas during the slave trade in the sixteenth and seventeenth centuries (30). It is believed that the DENV spread throughout the world through transport routes in the eighteenth and nineteenth centuries and that, after establishing itself, decreased between the 1950s and 1970s due to the aggressive vector control method of the time (6, 29, 31). In the 1990s, DENV reemerged following a decline in vector control efforts and increased insecticide resistance, culminating in one of the worst global epidemics with 2,326,000 reported cases in the Americas in 2015 (24, 28). The first case of CHIKV on the Caribbean island of Saint Martin was confirmed in October 2013. Subsequently, CHIKV spread to the Caribbean, Central America, and some regions of South America, totaling about 1.6 million cases (28). Chikungunya fever is a debilitating disease characterized by high fever associated with chronic polyarthralgia and generalized rash (23, 28, 32). Some complications have been reported, including hemorrhaging and cardiac, neurological, and gastrointestinal injury (22, 32). The social damage caused by CHIKV infection is inestimable because the virus can remain in the tissues of the joints, causing persistent arthralgia for months or even years (23). Kantor (23) suggested the virus was imported from Asia since it was shown that the isolates had the Asian genotype. Oliveira Melo et al. (33) suggested that ZIKV introduced in the Americas also came from Asia. This study evaluated two pregnant women from the state of Pernambuco, in northeast region of Brazil, who were diagnosed with fetal microcephaly and had symptoms of ZIKV infection. The patients were submitted to an amniocentesis test, blood test, and qPCR to detect the presence of the virus. Although the blood test was negative, amniocenteses and qPCR showed a positive result for ZIKV. The virus was isolated and sequenced, and the result showed Asian genotype in both cases (33). Studies based on the sequence of a large number of virus isolates indicated that a cryptic ZIKV circulation occurred in the Northeast of Brazil as early as February 2014, and from the region the virus disseminated nationally and internationally (34). In February 2016, the World Health Organization declared the situation “a public health emergency of international importance” due to the increasing number of cases of ZIKV infection in Brazil, and its possible association with neurological disorders and congenital anomalies, such as microcephaly (22, 29, 33). In 2016, the situation worsened and at the end of the year, the World Health Organization estimated that ~4 million people were infected with ZIKV. The impact of this number of cases in Brazil had deeper social and emotional consequences, since 1,434 cases of microcephaly were confirmed (2). The extent of the financial and political problems that the epidemic may cause in the future is still unknown, but efforts need to be concentrated on supporting and monitoring affected families. Lately, the first clinical case of infection with Mayaro (MAYV), a virus of the Togaviridae family recently arrived in Haiti, has been described. This virus was discovered in 1954 in Trinidad and Tobago, but so far only isolated cases are known in the Amazon and other regions of South America. According to epidemiological data available to the Ministry of Health, there were 197 notifications distributed in nine Brazilian states between December 2014 and June 2015, mainly in the north and center-west regions. There are still no records of deaths from the disease, but as is the case with CHIKV, those infected can continue to experience joint pain for weeks or months. Although Haemagogus sp. is the main vector of MAYV, A. aegypti is also capable of transmitting this arbovirus (35). The constant transit of arboviruses represents a challenge in the diagnosis and treatment of patients because, in addition to causing similar clinical manifestations (36), they can be transmitted simultaneously.
Along with the five arboviruses listed above, it is worth highlighting the presence of La Crosse and Rocio viruses, which can be transmitted by mosquitoes of the genus Aedes. The first cases of Rocio virus (ROCV) were recorded in the southeastern region of Brazil during a viral encephalitis epidemic that lasted for about 2 years in the 1970s. Although subsequent outbreaks did not occur, there was serological evidence of ROCV circulation, which serves as a warning signal for possible new epidemics (37). La crosse virus (LACV) is present in Latin America and in some regions of North America and is transmitted by mosquitoes of the genus Aedes. Although A. aegypti is not a very efficient vector, A. albopictus is able to transmit this virus. The cases of encephalitis due to LACV infection have low mortality, but there are reports of patients with neurological complications, which may make the differential diagnosis difficult with other arboviruses (10).
Importance of Aedes aegypti and Biotechnological Approaches Applied for Mosquito Control as a Strategy to Prevent the Emergence of Arboviruses
Physical and Chemical Control Approaches
The physical and chemical control methods are based on the elimination of breeding sites and in the use of insecticides to reduce population density, thus decreasing the transmission of pathogens and, consequently, preventing epidemics. In 1902, Oswaldo Cruz led the first Brazilian public campaign to control A. aegypti, which was based on the elimination of artificial breeding sites. At the time, this campaign aimed to reduce the transmission of yellow fever. However, the vector was eradicated only in the middle of 1955. Afterwards, the mosquito was reintroduced over time and became endemic in much of the country. In the following years, control strategies were guided by the “Programa Nacional de Controle da Dengue” (PNCD), which recommended using physical strategies based on the identification of regions according to infestation rates. To date, WHO has promoted integrated vector control, in addition to conventional tools such as mosquito nets treated with materials, nets, oviposition traps (ovitraps), among others. Numerous studies have shown that ovitraps are an effective strategy that contributes to the monitoring of A. aegypti populations hence the areas at risk of transmission of arboviruses (38–43). Mackay et al. (44) developed the autocidal gravid ovitrap (AGO-A), a lethal ovitrap based on the gravid ovitrap (GO). It has already been shown that the use of AGO has significantly reduced the prevalence of mosquitoes infected with CHIKV (39). The main limitation to using an ovitrap is that it contains insecticides, which may not be effective in regions where the mosquito population is resistant.
The larval period is the only immature stage during which feeding and growth occur in the life cycle of A. aegypti. The chemical control measures employed preferentially focus on this stage due to three striking attributes. First, the larvae are aquatic and feed on suspended organic matter adhering to the walls or sediment at the bottom of the reservoirs. As larval eating habits are not selective, they can easily ingest chemical or biological insecticides (45). Second, the larvae breathe atmospheric air through the siphon, located in the eighth abdominal segment. The moment they rise to the surface to breathe, they become more susceptible to control agents (21). Third, they are restricted to the oviposition site, which restricts their ability to migrate (21, 45). The main insecticides used act on the insects'central nervous system and belong to the groups of organochlorines, carbamates, organophosphates, and pyrethroids (46, 47). The indiscriminate use of Dichloro-diphenyltrichlorethane (DDT) and pyrethroids induced the development of resistance, which negatively impacted the effectiveness of vector control interventions in endemic countries (48–52). Macoris et al. (53) described that, after 10 years without usage of pyrethroids in São Paulo state, Brazil, A. aegypti showed persistent resistance. A bioassay with papers impregnated with a deltamethrin diagnostic dose (DD) was conducted yearly, from 2004 to 2015, with A. aegypti adults from 7 different sites, showing resistance in most cases. These results were a direct effect of pyrethroids being extensively used by São Paulo state governments between 1989 and 2000, and being the preferred insecticide for domestic use (53). This scenario is not limited only to Brazil or South America. Moyes et al. (54) described that resistance to pyrethroids are widespread throughout the world. Temephos was the preferred larvicide used in Brazil from 2003 to 2014. As a result of continuous usage, cases of resistance were detected in most of studies in all Brazilian geographic regions as compiled by Valle et al. (55). Corte et al. (56) conducted a study from October 2010 to August 2011 to evaluate A. aegypti resistance to Temephos from 7 municipalities in Sergipe state, Brazil. All mosquitoes were found to be resistant, varying between cities, even with an increased dose of Temephos. In recent years, interest has grown in the identification of plants with insecticidal properties (57). The use of plant extracts in the control of A. aegypti demonstrated low production cost, high biodegradability, and different active elements that delay the development of resistance in insects (57–62). However, phytochemicals can also have toxic effects that vary according to the species of the plant, with the part used, age of the plant, and extraction with selected solvent (57, 58, 63).
Immunoprophylactic and Biological Control Approaches
Several research groups around the world have focused on the search for arbovirus vaccines, primarily against DENV (64–69). Generally, this type of approach is species-specific and considering the huge variety of known arboviruses, vaccine strategies that seek to prevent transmission seem difficult and remote. This is a long process that requires active participation of public policies and a significant financial investment, which does not occur in most endemic regions (70–72). Several DENV vaccine candidates have been developed over the years, some of which are in the testing phase. The composition of the formulations varies between DNA (monovalent or tetravalent), recombinant adenoviruses, Alfavirus replicons, and chimeric E protein subunits, among others (64–69, 73, 74). However, only the CYD-TDV vaccine has reached the final stages of testing in humans in different parts of the world (75). CYD-TDV (Sanofi-Pasteur) is an attenuated chimeric vaccine containing recombinant fractions from the four serotypes of DENV (tetravalent). The tetravalent attenuated component (TDV) was developed by the Reed Army Research Institute (WRAIR), in collaboration with GlaxoSmithKline Vaccines (68). Sanofi Pasteur then developed the vaccine including the viral strains PUO-359 (DENV-1), PUO-218 (DENV-2), PaH881/88 (DENV-3), and 1228 (DENV-4) (75). After going through the clinical study phases, the vaccine was made commercially available under the name DengVaxia® (CYD-TDV, Sanofi-Pasteur). According to da Costa et al. (76), the effectiveness of this vaccine varies around 59%, but more studies are needed to confirm its long-term effectiveness. However, on December 13, 2017, WHO issued a statement warning that DengVaxia® should not be administered to people who have not been previously exposed to DENV due to an increased incidence of childhood cases of severe dengue in vaccinated individuals who had never been infected with the virus. Still, it remains the only arbovirus vaccine available at the moment.
While efforts in the search for vaccine targets are being made, vector control strategies need to be developed that can prevent the emergence of new epidemics and the simultaneously control circulating arboviruses. Due to the lack of antiviral drugs against arboviruses, the main measure for preventing or reducing the transmission of diseases depends entirely on the control of vectors or the interruption of human-vector contact (27). In this context, the use of sterile insect technique (SIT) and Release of Insects carrying a Dominant Lethal (RIDL) developed by genetic engineering was proposed as a control strategy (77). Alphey and Andreasen (78) reviewed these techniques, which are largely based on the RIDL. After copulation, this dominant gene causes the death of its progeny. These approaches show a great potential for disease control (79, 80) and have been tested in different endemic countries for A. aegypti control (81–84). In Brazil, the use of OX513A self-limiting strain reduced the local A. aegypti population by up to 95% in a suburb of Juazeiro, Bahia (85). This transgenically modified A. aegypti mosquito containing a dominant lethal gene transgenic strain (OX513A), was incorporated into the target field population (86). Furthermore, the impacts of introgression from a A. aegypti transgenic strain remain unclear for arbovirus control and transmission purposes (86).
A number of natural predators (fish and crustaceans) and pathogenic organisms (fungi and bacteria) have already been employed in an attempt to control A. aegypti. The entomopathogenic bacteria Bacillus thuringiensis var. Israelensis (Bti) is commonly found in nature and carries toxins with strong insecticidal activity through the formation of spores and crystals. The Bti has high specificity for A. aegypti and is practically innocuous to humans (21, 87). In addition, the combined action of different toxins reduces the risk of resistance (88). The use of this bacteria enabled the development of larvicides such as DengTech® (Fiocruz/BR3) and VectoBac WG. However, it has been reported that environmental factors such as high temperatures and a high annual rainfall interfere with VectoBac's efficacy (89).
An interesting approach is the use of Wolbachia gram-negative bacteria (Order Rickettisiales) discovered by Hertig and Wolbach (90) in the reproductive tissues of Culex pipiens. It is known that the different strains establish symbiotic relationships with more than 60% of the world's insect species and that they have a great potential to reduce the life span of mosquitoes and block infection by pathogens (27, 91–96). This strategy can be used as a population suppression or as a population replacement approach. The latter uses strains of Wolbachia that can block arboviruses, especially Flaviviruses (27, 97) and Alphaviruses (98). Several studies on this approach have shown promising results, especially in Australia (27, 92, 94–100). McMeniman et al. (101) showed that the introduction of the wMelPop-CLA, a Wolbachia strain, reduced a population of A. aegypti kept in the laboratory by up to 50%. Moreira et al. (27) demonstrated that the introduction of this strain inhibits the infection of DENV-2 and CHIKV in A. aegypti and suggested that the blocking strategy, in synergy with the reduction in life span, could be a promising approach to controlling the transmission of arbovirus. Walker et al. (96) transfected mosquitoes with the avirulent wMel, another Wolbachia strain, and showed a more accelerated invasion of A. aegypti populations when compared to the wMelPop-CLA strain in semi-field conditions. The authors also showed a blockage of DENV-2 transmission by both strains. The use of Wolbachia as a vector control method provided subsidies for the creation of the “Eliminate Dengue: Our challenge” Project (World Mosquito Program—WMP). The release of mosquitoes in northern Australia (2011) showed that Wolbachia had already spread throughout the population of A. aegypti 10 weeks later. The WMP originally aimed to control the transmission of DENV, but today it contributes significantly to the control of other arboviruses in 12 countries, including Brazil. Currently, it is being used in Brazil by the World Mosquito Program (formerly “Eliminate Dengue: Brazil Challenge”) and has shown results worth of attention (102). Moreover, the use of this bacteria has also proved to be a promising method of controlling other viruses. Ekwudu et al. (103) evaluated the ability of the wAlbB strain to reduce or block the replication of Flavivirus and Alphavirus species in cell cultures and showed that this control strategy is effective against a wide variety of RNA viruses. The cultures in the presence of WNV, ZIKV, Ross River (RRV), Barmah Forest (BFV), and Sindbis virus (SINV) infected with wAlbB showed significantly reduced in viruse load compared to Wolbachia-free controls.
Despite abundant evidence of success, the use of Wolbachia presents limitations in countries with wide temperature fluctuations. Ross et al. (104) showed a drastic reduction in the frequency of wMel infection after a heatwave of a 43.6°C, an effect that is stage specific. Another limitation of this strategy is that the establishment of Wolbachia in the mosquito population depends on resistance to insecticides. Garcia et al. (105) released A. aegypti strains infected with Wolbachia using susceptible (wMelBr) and resistant (wMelRio) mosquito-infected Wolbachia strains to pyrethroids in an isolated region of Rio de Janeiro, Brazil. Only the A. aegypti strain infected with the resistant strain (wMelRio) allowed the establishment of Wolbachia. The use of Wolbachia, when combined with other strategies such as a transmission blocking vaccine (TBVs), could help reduce arbovirus transmission; yet endemic countries spend about twice as much on treating patients as they do on preventing mosquito-transmitted diseases (106). The cost of dengue in the Americas is already billions of dollars a year (106). The strategy of investing in patient treatment and disregard vector control has already proven to be a complete failure, as arbovirus-related diseases have shown no clear sign of reduction over the past years.
The TBVs are strategies that consist of using essential vector proteins capable of inducing specific antibody production after host immunization. Therefore, after blood meal on an immunized host, the antibody triggered by vaccination could induce interference in the cell cycle on the vector or/and block virus infection to the vector, thus preventing disease transmission to human hosts (107). Some successful examples against transmission of Plasmodium berghei by Anopheles stephensi (108), P. vivax (109) by A. dirus (110), P. falsiparum by A. gambiae (111), and Leishmania infantum by Lutzomyia longipalpis (112) have been reported in the literature, showing this strategy as promising and possibly helpful in interrupting distinct pathogen transmission cycle. Notwithstanding the potential of TBVs, they do not directly protect humans, so there is resistance to their use in public health programs (113). In 1980, there were about 95 species of arboviruses cataloged in Brazil alone, but this number has doubled in the last three decades and today 210 arboviruses circulate in the country. It is known that at least 37 of them are capable of causing disease in humans (2). The recent epidemics in South America warn of the possibility of the emergence of new arboviruses and the resurgence of diseases transmitted by previously controlled vectors (114). In addition to preventing disease transmission, new strategies for sustainable control of vector populations are highly recommended (27). As viruses first infect and multiply in the midgut, molecules overexpressed after blood meal have been suggested as possible TBVs. The challenge with this strategy is the fact that some viruses have more than one serotype (114). Our research group has focused on the development of TBVs against some vectors, including A. aegypti. In a pre-clinical trial, we analyzed distinct vector antigen targets. The data are in the final phase of analysis, but we have already identified promising targets that interfered in all A. aegypti developmental stages and reduced the number of mosquitoes generated at the end of the first generation by up to 90% (unpublished data). TBVs that target specific mosquito molecules can help to reduce the circulation of pathogens transmitted by the same vector simultaneously. Taking into account the challenges of controlling vector-borne diseases, the future of vaccines against pathogens transmitted by vector will most likely incorporate the TBV rationale. One example is the use of subunit vaccines against Dengue in which nanocarrier platforms are added to recombinant immunogenic DENV proteins. Such nanocarriers are able to increase the immunogenicity of rather poor immunogens in the presence of adjuvants, potentiating their use. Carbon nanotubes are a good example of carriers that could be used for this purpose (Figure 2), being also associated to the TBV antigen in a single formulation. This approach could permit improved vaccinal efficacy toward a new generation of high-performance vaccines against vector-borne diseases.
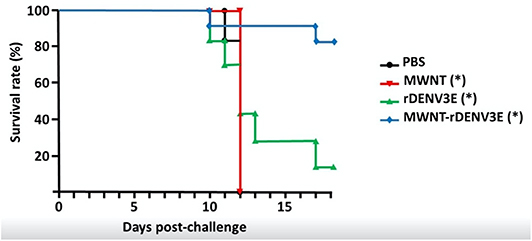
Figure 2. Survival rates in mice immunized or not with a subunit anti-Dengue vaccine and challenged with a virulent DENV3 strain. Briefly, the recombinant Dengue serotype 3 Envelope protein was produced in E. coli, purified by affinity chromatography, and quantified. Purified recombinant proteins were covalently linked to multi-walled carbon nanotubes though a diimide-activated amidation protocol (115). Evaluation of the nanotubes functionalization was verified by transmission electronic microscopy and Raman spectroscopy. Ten-weeks old BALB/c mice were divided into 4 groups: non-immunized control (PBS), vaccine-immunized group (MWNT-rDENV3E), animals immunized with the recombinant protein alone (rDENV3E), and animals inoculated only with pristine nanotubes (MWNT). All groups were either immunized or mock-immunized three times every 7 days, through the intramuscular route. After 7 days of the last inoculation, animals were challenged with 103 PFUs of DENV3. Animals were evaluated daily until the appearance of neuropathology and were then humanely euthanized when a 25% weight loss was confirmed. In total, surviving mice were evaluated for 21 days. The study was approved by the Committee on the Ethics of Animal Experiments from the Universidade Federal de Minas Gerais (CETEA/UFMG 270/2010). The symbol “*” in the groups (MWNT, rDENV3E, MWNT-rDENV3E) demonstrates statistical differences (p < 0.05) compared to PBS group, according One-way ANOVA test and Tukey's post-test analysis.
The medical importance of A. aegypti is clear, such as the failure to control it, the large number of transmitted pathogens, and the persistently high number of cases, as observed in dengue. In this scenario, biotechnological proposals, such as TBV development, could result in an important reduction in number of vectors and prevent the transmission of pathogens.
Author Contributions
MR-A, OM-J, PS, RM, JL, TS, IS, DL, MM, LR, and RG wrote the manuscript. DS-L, WD, NG, RA, MS, LA, FF, LM, and RG reviewed the manuscript. MR-A, OM-J, NG, RA, MS, LA, FF, LM, and RG drafted and critically evaluated the manuscript. All authors contributed to the article and approved the submitted version.
Funding
This work was supported by Fundação de Amparo à Pesquisa do Estado de Minas Gerais (grant PPM-00637-17 to RG), Conselho Nacional de Desenvolvimento Científico e Tecnológico (grant 309327/2017-2 to RG), Ministério da Saúde/DECIT, CAPES and Instituto Nacional de Ciência e Tecnologia em Doenças Tropicais (INCT-DT).
Conflict of Interest
The authors declare that the research was conducted in the absence of any commercial or financial relationships that could be construed as a potential conflict of interest.
References
1. Hubálek Z. Mosquito-borne viruses in Europe. Parasitol Res. (2008) 1:S29–43. doi: 10.1007/s00436-008-1064-7
2. Pan American Health Organization. Neglected, Tropical and Vector-Borne Diseases. (2017) Available online at: http://www.paho.org/hq/index.php?option=com_topicsandview=articleandid=37andItemid=40760
3. United Nation. World Urbanization Progress. (2014) Available online at: https://esa.un.org/unpd/wup/publications/files/wup2014-highlights.pdf
4. Sutherst RW. Global change and human vulnerability to vector-borne diseases. Clin Microbiol Rev. (2004) 17:136–73. doi: 10.1128/CMR.17.1.136-173.2004
5. Kraemer MUG, Sinka ME, Duda K, Mylne A, Shearer FM, Brady OJ, et al. The global compendium of Aedes aegypti and Ae. albopictus occurrence. Sci Data. (2015) 2:150035. doi: 10.1038/sdata.2015.35
6. Gubler DJ. Epidemic dengue/dengue hemorrhagic fever as a public health, social and economic problem in the 21st century. Trends Microbiol. (2002) 10:100–3. doi: 10.1016/S0966-842X(01)02288-0
8. Jupp PG, McIntosh BM, Dos Santos I, DeMoor P. Laboratory vector studies on six mosquito and one tick species with chikungunya virus. Trans R Soc Trop Med Hyg. (1981) 75:15–9. doi: 10.1016/0035-9203(81)90005-5
9. Zanluca C, Melo VC, Mosimann AL, Santos GI, Santos CN, Luz K. First report of autochthonous transmission of Zika virus in Brazil. Mem Inst Oswaldo Cruz. (2015) 110:569–72. doi: 10.1590/0074-02760150192
10. Gerhardt RR, Gottfried KL, Apperson CS, Davis BS, Erwin PC, Smith AB, et al. First isolation of La Crosse virus from naturally infected Aedes albopictus. Emerg Infect Dis. (2001) 7:807–11. doi: 10.3201/eid0705.017506
11. De Arruda M, Carvalho MB, Nussenzweig RS, Maracic M, Ferreira AW, Cochrane AH. Potential vectors of malaria and their different susceptibility to Plasmodium falciparum and Plasmodium vivax in northern Brazil identified by immunoassay. Am J Trop Med Hyg. (1986) 35:873–81. doi: 10.4269/ajtmh.1986.35.873
12. De Oliveira-Ferreira J, Lourenço-de-Oliveira R, Teva A, Deane LM, Daniel-Ribeiro CT. Natural malaria infections in anophelines in Rondonia State, Brazilian Amazon. Am J Trop Med Hyg. (1990) 43:6–10. doi: 10.4269/ajtmh.1990.43.1.TM0430010006
13. Groot H, Oya A, Bernal C, Barreto-Reyes P. Guaroa virus, a new agent isolated in Colombia, South America. Am J Trop Med Hyg. (1959) 8:604–9. doi: 10.4269/ajtmh.1959.8.604
14. Michael E, Bundy DA, Grenfell BT. Re-assessing the global prevalence and distribution of lymphatic filariasis. Parasitology. (1996) 112:409–28. doi: 10.1017/S0031182000066646
15. Komar N, Clark GG. West Nile virus activity in Latin America and the Caribbean. Rev Panam Salud Publica. (2006) 19:112–7. doi: 10.1590/S1020-49892006000200006
16. Monath TP, Sabattini MS, Pauli R, Daffner JF, Mitchell CJ, Bowen GS, et al. Arbovirus investigations in Argentina, 1977-1980. Serologic surveys and sentinel equine program. Am J Trop Med Hyg. (1985) 34:966–75. doi: 10.4269/ajtmh.1985.34.966
17. Alice FJ. Encefalomielite equina na Bahia - estudo de três amostras isoladas. Rev Bras Biol. (1951) 11:125–44.
18. Pinheiro FP, Travassos da Rosa AP, Travassos da Rosa JF, Bensabath G. An outbreak of Oropouche virus disease in the Vicinity of Santarém, Pará, Brazil. Trop Med Parasitol. (1976) 27:213–23.
19. Causey OR, Maroja OM. Mayaro virus: a new human disease agent. Investigation of an epidemic of acute febrile illness on the river Guama in Pará, Brazil, and isolation of Mayaro virus as causative agent. Am J Trop Med Hyg. (1957) 6:1017–23. doi: 10.4269/ajtmh.1957.6.1017
20. Powell JR, Tabachnick WJ. History of domestication and spread of Aedes aegypti - a review. Mem Inst Oswaldo Cruz Rio de Janeiro. (2013) 108:11–7. doi: 10.1590/0074-0276130395
21. Carvalho FD, Moreira LA. Why is Aedes aegypti Linnaeus so successful as a species? Neotrop Entomol. (2017) 46:243–55. doi: 10.1007/s13744-017-0520-4
22. World Health Organization. Zika virus outbreaks in the Americas. Wkly Epidemiol Rec. (2017) 90:609–16.
24. Cleton N, Koopmans M, Reimerink J, Godeke GJ, Reusken C. Come fly with me: review of clinically important arboviruses for global travelers. J Clin Virol. (2012) 55:191–203. doi: 10.1016/j.jcv.2012.07.004
25. Martens P. How will climate change affect human health? Am Sci. (1999) 87:534–41. doi: 10.1511/1999.42.839
26. Tatem AJ. Rogers DJ, Hay SI. Global transport networks and infectious disease spread. Adv Parasitol. (2006) 62:293–343. doi: 10.1016/S0065-308X(05)62009-X
27. Moreira LA, Iturbe-Ormaetxe I, Jeffery JA, Lu G, Pyke AT, Hedges LM, et al. A Wolbachia symbiont in Aedes aegypti limits infection with dengue, Chikungunya, and Plasmodium. Cell. (2009) 139:1268–78. doi: 10.1016/j.cell.2009.11.042
28. Diaz-González EE, Kautz TF, Dorantes-Delgado A, Malo-García IR, Laguna-Aguilar M, Langsjoen RM, et al. First report of Aedes aegypti transmission of chikungunya virus in the Americas. Am J Trop Med Hyg. (2015) 93:1325–9. doi: 10.4269/ajtmh.15-0450
29. Schuler-Faccini L, Ribeiro EM, Feitosa IML, Horovitz DD, Cavalcanti DP, Pessoa A, et al. Brazilian Medical Genetics Society-Zika embryopathy task force. Possible association between Zika virus infection and microcephaly - Brazil, 2015. Morb Mortal Wkly Rep. (2016) 65:59–62. doi: 10.15585/mmwr.mm6503e2
30. Ali S, Gugliemini O, Harber S, Harrison A, Houle L, Ivory J, et al. Environmental and social change drive the explosive emergence of Zika virus in the Americas. PLoS Negl Trop Dis. (2017) 11:e0005135. doi: 10.1371/journal.pntd.0005135
31. Gubler DJ. Climate change: implications for human health. Health Environ Digest. (1998) 12:54–6.
32. Caglioti C, Lalle E, Castilletti C, Carletti F, Capobianchi MR, Bordi L. Chikungunya virus infection: an overview. New Microbiol. (2013) 36:211–27.
33. Oliveira Melo AS, Malinger G, Ximenes R, Szejnfeld PO, Alves Sampaio S, Bispo de Filippis AM. Zika virus intrauterine infection causes fetal brain abnormality and microcephaly: tip of the iceberg? Ultrasound Obstet Gynecol. (2016) 47:6–7. doi: 10.1002/uog.15831
34. Faria NR, Quick J, Claro IM, Thézé J, de Jesus JG, Giovanetti M, et al. Establishment and cryptic transmission of Zika virus in Brazil and the Americas. Nature. (2017) 546:406–10. doi: 10.1038/nature22401
35. Long KC, Ziegler SA, Thangamani S, Hausser NL, Kochel TJ, Higgs S, et al. Experimental transmission of Mayaro virus by Aedes aegypti. Am J Trop Med Hyg. (2011) 85:750–7. doi: 10.4269/ajtmh.2011.11-0359
36. Moulin K, Selby K, Cherpillod P, Kaiser L, Boillat-Blanco N. Simultaneous outbreaks of Dengue, Chikungunya and Zika virus infections: diagnosis challenge in a returning traveller with nonspecific febrile illness. New Microbes New Infect. (2016) 11:6–7. doi: 10.1016/j.nmni.2016.02.003
37. Chávez JH, França RF, Oliveira CJ, Aquino MT, Farias KJ, Machado PR, et al. Influence of the CCR-5/MIP-1 α axis in the pathogenesis of Rocio virus encephalitis in a mouse model. Am J Trop Med Hyg. (2013) 89:1013–8. doi: 10.4269/ajtmh.12-0591
38. Wermelinger ED, Ferreira AP, Carvalho RW, Silva AA, Benigno CV. Aedes aegypti eggs oviposited on water surface collected from field ovitraps in Nova Iguaçu City, Brazil. Rev Soc Bras Med Trop. (2015) 48:770–2. doi: 10.1590/0037-8682-0087-2015
39. Barrera R, Acevedo V, Felix GE, Hemme RR, Vazquez J, Munoz JL, et al. Impact of autocidal gravid ovitraps on Chikungunya virus incidence in Aedes aegypti (Diptera: Culicidae) in areas with and without traps. J Med Entomol. (2017) 54:387–95. doi: 10.1093/jme/tjw187
40. Piovezan R, Visockas A, de Azevedo TS, Von Zuben CJ, Sallum MAM. Spatial-temporal distribution of Aedes (Stegomyia) aegypti and locations of recycling units in southeastern Brazil. Parasit Vectors. (2019) 12:541. doi: 10.1186/s13071-019-3794-z
41. Sharp TM, Lorenzi O, Torres-Velásquez B, Acevedo V, Pérez-Padilla J, Rivera A, et al. Autocidal gravid ovitraps protect humans from chikungunya virus infection by reducing Aedes aegypti mosquito populations. PLoS Negl Trop Dis. (2019) 13:e0007538. doi: 10.1371/journal.pntd.0007538
42. Soares ENL, Santos MAB, Macedo LO, Santos CVB, Agra MCR, Alves LC, et al. Spatial distribution of Aedes aegypti (Diptera: Culicidae) in vulnerable areas for the transmission of arboviruses. Rev Soc Bras Med Trop. (2019) 52:e20180341. doi: 10.1590/0037-8682-0341-2018
43. Nascimento KLC, da Silva JFM, Zequi JAC, Lopes J. Comparison between larval survey index and positive ovitrap index in the evaluation of populations of Aedes (Stegomyia) aegypti (Linnaeus, 1762) North of Paraná, Brazil. Environ Health Insights. (2020) 14:1178630219886570. doi: 10.1177/1178630219886570
44. Mackay AJ, Amador M, Barrera R. An improved autocidal gravid ovitrap for the control and surveillance of Aedes aegypti. Parasit Vectors. (2013) 6:225. doi: 10.1186/1756-3305-6-225
45. Lourenço de Oliveira R. Biologia e comportamento do vetor. In: Valle D, editors. Dengue: Teorias e Práticas. Rio de Janeiro: Fiocruz (2015) p. 75–92.
47. Palchick S. Chemical control of vectors. In: Marquardt W, editor. The Biology of Disease Vectors. University Press of Colorado (1996) 816.
48. Ponlawat A, Scott JG, Harrington LC. Insecticide susceptibility of Aedes aegypti and Aedes albopictus across Thailand. J Med Entomol. (2005) 42:821–5. doi: 10.1603/0022-2585(2005)042[0821:ISOAAA]2.0.CO;2
49. Da-Cunha Pereira M, Pereira Lima JB, Brogdon WG, Moya GE, Valle D, et al. Monitoring of resistance to the pyrethroid cypermethrin in Brazilian Aedes aegypti (Díptera: Culicidae) populations collected between 2001 and 2003. Mem Inst Oswaldo Cruz. (2005) 100:441–4. doi: 10.1590/S0074-02762005000400017
50. Santacoloma Veron L, Chaves Cordoba B, Brochero HL. Susceptibilidad de Aedes aegypti a DDT, deltametrina y lamdacialotrina en Colombia. Rev Panam Salud Publica. (2012) 27:66–73. doi: 10.1590/S1020-49892010000100010
51. McAllister JC, Godsey MS, Scott ML. Pyrethroid resistance in Aedes aegypti and Aedes albopictus from Port-au-Prince, Haiti. J Vector Ecol. (2012) 37:325–32. doi: 10.1111/j.1948-7134.2012.00234.x
52. Seccacini E, Juan L, Zerba E, Licastro S. Aedes aegypti (Diptera: Culicidae): evaluation of natural long-lasting materials containing pyriproxyfen to improve control strategies. Parasitol Res. (2014) 113:3355–60. doi: 10.1007/s00436-014-3998-2
53. Macoris ML, Martins AJ, Andrighetti MTM, Lima JBP, Valle D. Pyrethroid resistance persists after ten years without usage against Aedes aegypti in governmental campaigns: lessons from São Paulo State, Brazil. PLoS Negl Trop Dis. (2018) 12:e0006390. doi: 10.1371/journal.pntd.0006390
54. Moyes CL, Vontas J, Martins AJ, Ng LC, Koou SY, Dusfour I, et al. Contemporary status of insecticide resistance in the major Aedes vectors of arboviruses infecting humans. PLoS Negl Trop Dis. (2017) 11:e0005625. doi: 10.1371/journal.pntd.0005625
55. Valle D, Bellinato DF, Viana-Medeiros PF, Lima JBP, Martins Junior AJ. Resistance to temephos and deltamethrin in Aedes aegypti from Brazil between 1985 and 2017. Mem Inst Oswaldo Cruz. (2019) 114:e180544. doi: 10.1590/0074-02760180544
56. Corte R, Melo VAD, Dolabella SS, Marteis LS. Variation in temephos resistance in field populations of Aedes aegypti (Diptera: Culicidae) in the State of Sergipe, Northeast Brazil. Rev Soc Bras Med Trop. (2018) 51:284–90. doi: 10.1590/0037-8682-0449-2017
57. Barbosa PBBM, De Oliveira JM, Chagas JM, Rabelo LM, de Medeiros GF, Giodani R, et al. Evaluation of seed extracts from plants found in the Caatinga biome for the control of Aedes aegypti. Parasitol Res. (2014) 113:3565–80. doi: 10.1007/s00436-014-4022-6
58. Luna JS, Santos AF, Lima MRF, de Omena MC, de Mendonça FA, Bieber LW, et al. A study of the larvicidal and molluscicidal activities of some medicinal plants from northeast Brazil. J Ethnopharmacol. (2005) 97:199–206. doi: 10.1016/j.jep.2004.10.004
59. Omena MC, Navarro DMAF, De Paula JE, Luna JS, Ferreira de Lima MR, Sant'Ana AE. Larvicidal activities against Aedes aegypti of some Brazilian medicinal plants. Bioresour Technol. (2007) 98:2549–56. doi: 10.1016/j.biortech.2006.09.040
60. Govindarajan M, Mathivanant EK, Krishnappa K, Anandan A. Ovicidal and repellent activities of botanical extracts against Culex quinquefasciatus, Aedes aegypti and Anopheles stephensi (Diptera: Culicidae). Asian Pac J Trop Biomed. (2011) 1:43–8. doi: 10.1016/S2221-1691(11)60066-X
61. Pontual EV, Napoleão TH, Assis CRD, de Souza Bezerra R, Xavier HS, Navarro DM, et al. Effect of Moringa oleifera flower extract on larval trypsin and acethylcholinesterase activities in Aedes aegypti. Arch Insect Biochem Physiol. (2012) 79:135–52. doi: 10.1002/arch.21012
62. Valle D, Belinato TA, Martins AJ. Controle químico de Aedes aegypti, resistência a inseticidas e alternativas. In: Valle D, editor. Dengue: Teorias e Práticas. Fiocruz (2015). p. 93–126.
63. Patil CD, Patil SV, Salunke BK, Salunkhe RB. Bioefficacy of Plumbago zeylanica (Plumbaginaceae) and Cestrum nocturnum (Solanaceae) plant extracts against Aedes aegypti (Diptera: Culicide) and nontarget fish Poecilia reticulate. Parasitol Res. (2011) 108:1253–63. doi: 10.1007/s00436-010-2174-6
64. Zellweger RM, Miller R, Eddy WE, White LJ, Johnston RJ, Shresta S, et al. Role of humoral versus cellular responses induced by a protective dengue vaccine candidate. PLoS Pathog. (2013) 9:e1003723. doi: 10.1371/journal.ppat.1003723
65. Torresi J, Tapia-Conyer R, Margolis H. Preparing for dengue vaccine introduction: recommendations from the 1st dengue v2V international meeting. PLoS Negl Trop Dis. (2013) 7:e2261. doi: 10.1371/journal.pntd.0002261
66. Züst R, Dong H, Li XF, Chang DC, Zhang B, Balakrishnan T, et al. Rational design of a live attenuated dengue vaccine: 2-o-methyltransferase mutants are highly attenuated and immunogenic in mice and macaques. PLoS Pathog. (2013) 9:e1003521. doi: 10.1371/journal.ppat.1003521
67. Huang CY, Kinney RM, Livengood JA, Bolling B, Arguello JJ, Luy BE, et al. Genetic and phenotypic characterization of manufacturing seeds for a tetravalent dengue vaccine (DENVax). PLoS Negl Trop Dis. (2013) 7:e2243. doi: 10.1371/journal.pntd.0002243
68. Thomas SJ, Eckels KH, Carletti I, De La Barrera R, Dessy F, Fernandez S, et al. A phase II, randomized, safety and immunogenicity study of a re-derived, live-attenuated dengue virus vaccine in healthy adults. Am J Trop Med Hyg. (2013) 88:73–88. doi: 10.4269/ajtmh.2012.12-0361
69. Zhao H, Jiang T, Zhou XZ, Deng YQ, Li XF, Chen SP, et al. Induction of neutralizing antibodies against four serotypes of dengue viruses by MixBiEDIII, a tetravalent dengue vaccine. PLoS ONE. (2014) 9:e86573. doi: 10.1371/journal.pone.0086573
70. Durbin AP, Whitehead SS. Next-generation dengue vaccines: novel strategies currently under development. Viruses. (2011) 3:800–14. doi: 10.3390/v3101800
71. del Angel RM, Reyes-Del J. Dengue vaccines: strongly sought but not a reality just yet. PLoS Pathog. (2013) 9:e1003551. doi: 10.1371/journal.ppat.1003551
72. McArthur MA, Sztein MB, Edelman R. Dengue vaccines: recent developments, ongoing challenges and current candidates. Expert Rev Vaccines. (2013) 12:933–53. doi: 10.1586/14760584.2013.815412
73. Azevedo AS, Yamamura AM, Freire MS, Trindade GF, Bonaldo M, Galler R, et al. DNA vaccines against dengue vírus type 2 based on truncate envelope protein or its domain III. PLoS ONE. (2011) 6:e20528. doi: 10.1371/journal.pone.0020528
74. Azevedo AS, Gonçalves AJ, Archer M, Freire MS, Galler R, Alves AM. The synergistic effect of combined immunization with a DNA vaccine and chimeric yellow fever/dengue virus leads to strong protection against dengue. PLoS ONE. (2013) 8:e58357. doi: 10.1371/journal.pone.0058357
75. Guy B, Barrere B, Malinowski C, Saville M, Teyssou R, Lang J. From research to phase III: preclinical, industrial and clinical development of the Sanofi Pasteur tetravalent dengue vaccine. Vaccine. (2011) 29:7229–41. doi: 10.1016/j.vaccine.2011.06.094
76. da Costa VG, Marques-Silva AC, Floriano VG, Moreli ML. Safety, immunogenicity and efficacy of a recombinant tetravalentdengue vaccine: a meta-analysis of randomized trials. Vaccine. (2014) 32:4885–92 doi: 10.1016/j.vaccine.2014.07.008
77. Alphey L. Re-engineering the insect sterile technique. Ins Bioch Mol Biol. (2002) 32:1243–7. doi: 10.1016/S0965-1748(02)00087-5
78. Alphey L, Andreasen M. Dominant lethality and insect population control. Mol Bioch Parasitol. (2002) 121:173–8. doi: 10.1016/S0166-6851(02)00040-3
79. Alphey L, Benedict M, Bellini R, Clark GG, Dame DA, Service MW, et al. Sterile-insect methods for control of mosquito-borne diseases: an analysis. Vector Borne Zoonotic Dis. (2010) 10:295–311. doi: 10.1089/vbz.2009.0014
80. Zheng X, Zhang D, Li Y, Yang C, Wu Y, Liang X, et al. Incompatible and sterile insect techniques combined eliminate mosquitoes. Nature. (2019) 572:56–61. doi: 10.1038/s41586-019-1407-9
81. Harris AF, Nimmo D, McKemey AR, Kelly N, Scaife S, Donnelly CA, et al. Field performance of engineered male mosquitoes. Nat Biotechnol. (2011) 29:1034–7. doi: 10.1038/nbt.2019
82. Harris AF, McKemey AR, Nimmo D, Curtis Z, Black I, Morgan SA, et al. Successful suppression of a field mosquito population by sustained release of engineered male mosquitoes. Nat Biotechnol. (2012) 30:828–30. doi: 10.1038/nbt.2350
83. Kittayapong P, Kaeothaisong NO, Ninphanomchai S, Limohpasmanee W. Combined sterile insect technique and incompatible insect technique: sex separation and quality of sterile Aedes aegypti male mosquitoes released in a pilot population suppression trial in Thailand. Parasit. Vectors. (2018) 11(Suppl. 2):657. doi: 10.1186/s13071-018-3214-9
84. Kittayapong P, Ninphanomchai S, Limohpasmanee W, Chansang C, Chansang U, Mongkalangoon P. Combined sterile insect technique and incompatible insect technique: The first proof-of-concept to suppress Aedes aegypti vector populations in semi-rural settings in Thailand. PLoS Negl Trop Dis. (2019) 13:e0007771. doi: 10.1371/journal.pntd.0007771
85. Carvalho DO, McKemey AR, Garziera L, Lacroix R, Donnelly CA, Alphey L, et al. Suppression of a field population of Aedes aegypti in Brazil by sustained release of transgenic male mosquitoes. PLoS Negl Trop Dis. (2015) 9:e0003864. doi: 10.1371/journal.pntd.0003864
86. Evans BR, Kotsakiozi P, Costa-da-Silva AL, Ioshino RS, Garziera L, Pedrosa MC, et al. Transgenic Aedes aegypti mosquitoes transfer genes into a natural population. Sci Rep. (2019) 9:13047. doi: 10.1038/s41598-019-49660-6
87. Boyce R, Lenhart A, Kroeger A, Velayudhan R, Roberts B, Horstick O. Bacillus thuringiensis israelensis (Bti) for the control of dengue vectors: systematic literature review. Trop Med Int Heal. (2013) 18:564–77. doi: 10.1111/tmi.12087
88. Andrade CFS, Cabrini I. Controle de pernilongos e borrachudos em áreas urbanas. In: Pinto AS, Rossi MM, editors. Salmeron E Manejo de Pragas Urbanas ESALQ. Piracicaba: ESALQ (2007). p. 55–66.
89. Silva AS, Lobo KS, Da Silva JS, Vale CFS, Wanderli PT, Soares Pinheiro VC. Influência dos fatores abióticos na efetividade de Bacillus thuringiensis var. israelensis (Berliner, 1911) para larvas de Aedes aegypti (Linnaeus, 1762). Rev Cubana Med Trop. (2014) 66:174–90.
90. Hertig M, Wolbach SB. Studies on Rickettsia-like micro-organisms in insects. J Med Res. (1924) 44:329–74.
91. Sinkins SP, O'Neill SL. Wolbachia as a vehicle to modify insect populations. In: A James, editors. Insect Transgenesis: Methods and Applications A, Boca Raton, FL: CRC Press (2000). p. 271–87.
92. Brownstein JS, Hett E, O'Neill SL. The potential of virulent Wolbachia to modulate disease transmission by insects. J Invertebr Pathol. (2003) 84:24–9. doi: 10.1016/S0022-2011(03)00082-X
93. Rasgon JL, Styer LM, Scott TW. Wolbachia-induced mortality as a mechanism to modulate pathogen transmission by vector arthropods. J Med Entomol. (2003) 40:125–32. doi: 10.1603/0022-2585-40.2.125
94. Cook PE, McMeniman CJ, O'Neill SL. Modifying insect population age structure to control vector-borne disease. Adv Exp Med Biol. (2008) 627:126–40. doi: 10.1007/978-0-387-78225-6_11
95. Hoffmann AA, Montgomery BL, Popovici J, Iturbe-Ormaetxe I, Johnson PH, Muzzi F, et al. Successful establishment of Wolbachia in Aedes populations to suppress dengue transmission. Nature. (2011) 476:454–7. doi: 10.1038/nature10356
96. Walker T, Johnson PH, Moreira LA, Iturbe-Ormaetxe I, Frentiu FD, et al. The wMel Wolbachia strain blocks dengue and invades caged Aedes aegypti populations. Nature. (2011) 476:450–3. doi: 10.1038/nature10355
97. Dutra HL, Rocha MN, Dias FB, Mansur SB, Caragata EP, Moreira LA. Wolbachia blocks currently circulating Zika virus isolates in Brazilian Aedes aegypti mosquitoes. Cell Host Microbe. (2016) 19:771–4. doi: 10.1016/j.chom.2016.04.021
98. Aliota MT, Walker EC, Uribe Yepes A, Velez ID, Christensen BM, Osorio JE. The wMel strain of Wolbachia reduces transmission of Chikungunya virus in Aedes aegypti. PLoS Negl Trop Dis. (2016) 10:e0004677. doi: 10.1371/journal.pntd.0004677
99. O'Neill SL, Ryan PA, Turley AP, Wilson G, Retzki K, Iturbe-Ormaetxe I, et al. Scaled deployment of Wolbachia to protect the community from dengue and other Aedes transmitted arboviruses. Version 3. Gates Open Res. (2019) 2:36. doi: 10.12688/gatesopenres.12844.3
100. Ryan PA, Turley AP, Wilson G, Hurst TP, Retzki K, Brown-Kenyon J, et al. Establishment of wMel Wolbachia in Aedes aegypti mosquitoes and reduction of local dengue transmission in Cairns and surrounding locations in northern Queensland, Australia. Gates Open Res. (2019) 3:1547. doi: 10.12688/gatesopenres.13061.1
101. McMeniman CJ, Lane RV, Cass BN, Fong AW, Sidhu M, Wang YF, et al. Stable introduction of a life-shortening Wolbachia infection into the mosquito Aedes aegypti. Science. (2009) 323:141–4. doi: 10.1126/science.1165326
102. Dutra HL, Dos Santos LM, Caragata EP, Silva JB, Villela DA, Maciel-de-Freitas R, et al. From lab to field: the influence of urban landscapes on the invasive potential of Wolbachia in Brazilian Aedes aegypti mosquitoes. PLoS Negl Trop Dis. (2015) 9:e0003689. doi: 10.1371/journal.pntd.0003689
103. Ekwudu O, Devine GJ, Aaskov JG, Frentiu FD. Wolbachia strain wAlbB blocks replication of flaviviruses and alphaviruses in mosquito cell culture. Parasit Vectors. (2020) 13:54. doi: 10.1186/s13071-020-3936-3
104. Ross PA, Axford JK, Yang Q, Staunton KM, Ritchie SA, Richardson KM, et al. Heatwaves cause fluctuations in wMel Wolbachia densities and frequencies in Aedes aegypti. PLoS Negl Trop Dis. (2020) 14:e0007958. doi: 10.1371/journal.pntd.0007958
105. Garcia GA, Hoffmann AA, Maciel-de-Freitas R, Villela DAM. Aedes aegypti insecticide resistance underlies the success (and failure) of Wolbachia population replacement. Sci Rep. (2020) 10:63. doi: 10.1038/s41598-019-56766-4
106. Fitzpatrick C, Haines A, Bangert M, Farlow A, Hemingway J, Velayudhan R. An economic evaluation of vector control in the age of a dengue vaccine. PLoS Negl Trop Dis. (2017) 11:e0005785. doi: 10.1371/journal.pntd.0005785
107. Conway MJ, Colpitts TM, Fikrig E. Role of the vector in arbovirus transmission. Ann Rev Virol. (2014) 1:71–88. doi: 10.1146/annurev-virology-031413-085513
108. Mathias DK, Plieskatt JL, Armistead JS, Bethony JM, Abdul-Majid KB, McMillan A, et al. Expression, immunogenicity, histopathology, potency of a mosquito-based malaria transmission-blocking recombinant vaccine. Infect Immun. (2012) 80:1606–14. doi: 10.1128/IAI.06212-11
109. Qiu Y, Zhao Y, Liu F, Ye B, Zhao Z, Thongpoon S, et al. Evaluation of Plasmodium vivax HAP2 as a transmission-blocking vaccine candidate. Vaccine. (2020) 38:2841–8. doi: 10.1016/j.vaccine.2020.02.011
110. Miyata T, Harakuni T, Sugawa H, Sattabongkot J, Kato A, Tachibana M, et al. Adenovirus-vectored Plasmodium vivax ookinete surface protein, Pvs25, as a potential transmission-blocking vaccine. Vaccine. (2011) 29:2720–6. doi: 10.1016/j.vaccine.2011.01.083
111. Yenkoidiok-Douti L, Williams AE, Canepa GE, Molina-Cruz A, Barillas-Mury C. Engineering a virus-like particle as an antigenic platform for a Pfs47-targeted malaria transmission-blocking vaccine. Sci Rep. (2019) 9:16833. doi: 10.1038/s41598-019-53208-z
112. Gomes R, Teixeira C, Teixeira MJ, Oliveira F, Menezes MJ, Silva C, et al. Immunity to a salivary protein of a sand fly vector protects against the fatal outcome of visceral leishmaniasis in a hamster model. Proc Natl Acad Sci USA. (2008) 105:7845–50. doi: 10.1073/pnas.0712153105
113. Zumaya-Estrada FA, Rodríguez MC, Rodríguez MH. Pathogen-insect interaction candidate molecules for transmission-blocking control strategies of vector borne diseases. Salud Publica Mex. (2018) 60:77–85. doi: 10.21149/8140
114. Londono-Renteria B, Troupin A, Colpitts TM. Arbovirosis and potential transmission blocking vaccines. Parasit Vectors. (2016) 9:516. doi: 10.1186/s13071-016-1802-0
Keywords: Aedes aegypti, arboviruses, vector control, Latin America, vaccines
Citation: Rodrigues-Alves ML, Melo-Júnior OAdO, Silveira P, Mariano RMdS, Leite JC, Santos TAP, Soares IS, Lair DF, Melo MM, Resende LA, da Silveira-Lemos D, Dutra WO, Gontijo NdF, Araujo RN, Sant'Anna MRV, Andrade LAF, da Fonseca FG, Moreira LA and Giunchetti RC (2020) Historical Perspective and Biotechnological Trends to Block Arboviruses Transmission by Controlling Aedes aegypti Mosquitos Using Different Approaches. Front. Med. 7:275. doi: 10.3389/fmed.2020.00275
Received: 19 February 2020; Accepted: 18 May 2020;
Published: 23 June 2020.
Edited by:
Herbert Leonel de Matos Guedes, Federal University of Rio de Janeiro, BrazilReviewed by:
Ajay Kumar Sharma, Institute of Nuclear Medicine & Allied Sciences (DRDO), IndiaDevanathan Sukumaran, Defence Research & Development Establishment (DRDE), India
Copyright © 2020 Rodrigues-Alves, Melo-Júnior, Silveira, Mariano, Leite, Santos, Soares, Lair, Melo, Resende, da Silveira-Lemos, Dutra, Gontijo, Araujo, Sant'Anna, Andrade, da Fonseca, Moreira and Giunchetti. This is an open-access article distributed under the terms of the Creative Commons Attribution License (CC BY). The use, distribution or reproduction in other forums is permitted, provided the original author(s) and the copyright owner(s) are credited and that the original publication in this journal is cited, in accordance with accepted academic practice. No use, distribution or reproduction is permitted which does not comply with these terms.
*Correspondence: Rodolfo Cordeiro Giunchetti, Z2l1bmNoZXR0aUBpY2IudWZtZy5icg==; Z2l1bmNoZXR0aUBnbWFpbC5jb20=