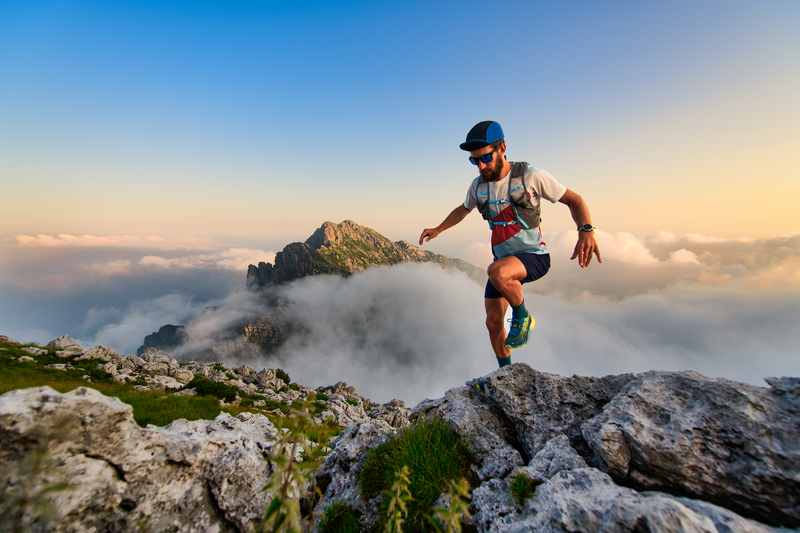
94% of researchers rate our articles as excellent or good
Learn more about the work of our research integrity team to safeguard the quality of each article we publish.
Find out more
REVIEW article
Front. Med. , 27 February 2020
Sec. Regulatory Science
Volume 7 - 2020 | https://doi.org/10.3389/fmed.2020.00050
In recent years inhaled systems have shown momentum as patient-personalized therapies emerge. A significant improvement in terms of therapeutic efficacy and/or reduction adverse systemic effects is anticipated from their use owing these systems regional accumulation. Nevertheless, whatever safety and efficacy evidence required for inhaled formulations regulatory approval, it still poses an additional hurdle to gaining market access. In contrast with the formal intravenous medicines approval, the narrower adoption of pulmonary administration might rely on discrepancies in pre-clinical and clinical data provided by the marketing authorization holder to the regulatory authorities. Evidences of a diverse and inconsistent regulatory framework led to concerns over toxicity issues and respiratory safety. However, an overall trend to support general concepts of good practices exists. Current regulatory guidelines1 that supports PK/PD (pharmacokinetics/pharmacodynamic) assessment seeks attention threatening those inhaled formulations set to be approved in the coming years. A more complex scenario arises from the attempt of implementing nanomedicines for pulmonary administration. Cutting-edge image techniques could play a key role in supporting diverse stages of clinical development facilitating this pharmaceutics take off and speed to patients. The ongoing challenge in adapting conventional regulatory frameworks has proven to be tremendously difficult in an environment where market entry relies on multiple collections of evidence. This paper intention is to remind us that an acceptable pre-clinical toxicological program could emerge from, but not only, an accurate and robust data imaging collection. It is our conviction that if implemented, inhaled nanomedicines might have impact in multiple severe conditions, such as lung cancer, by fulfilling the opportunity for developing tailored treatments while solving dose-related toxicity issues; the most limiting threat in conventional lung cancer clinical management.
Uncertainty over the conceivable safety issues over pulmonary administration has dramatically deemed its potential. Inhaled therapeutics or vaccines have the advantage of providing its accumulation in the target tissue avoiding its systemic spread and loss of pharmacological activity. Local drug accumulation with subsequent high local efficacy as it was demonstrated by the available data gathered from respiratory diseases, such as asthma, cystic fibrosis and chronic obstructive pulmonary disease (COPD) (1). Nanoparticle-based systems are cutting-edge feasible pulmonary nanomedicines, both for respiratory or non-respiratory diseases. Being developed as platforms they might represent for the pharmaceutical industry the much-needed flexibility to reach a pipeline of several rationales to provide inhaled formulations as a high-quality strategy against uncured diseases, such as, lung cancer.
Different indications of approved medicines reflect the need to fight multiple targets over cancer tumorigenesis, e.g., the complex microenvironment, growth rate, angiogenesis, dissemination and immunosuppressive properties. Concerning the clinical implications of innovative tools, in which drug “cocktails” and “sandwich-based” treatments have become more used, one challenge for the pharmaceutical technology is to fulfill unmet medical needs using more precise medicines.
Regulatory approval of breakthrough molecules, such as, immunotherapies, nivolumab (Opdivo®, Bristol-Myers Squibb), and pembrolizumab (Keytruda®, Merck) highlighted that pharmaceutical companies are still pursuing truly innovative solutions for lung cancer management. This trend can been also seen in the recent approved personalized therapies, such as osimertinib to be used in Epidermal Growth Factor Receptor (EGFR) exon 20 mutated lung carcinomas after failure of first line Tyrosine kinase inhibitors (TKI), or crizotinib (Xalkori, Pfizer) for first line metastatic ROS1 positive lung carcinomas, or even alectinib for anaplastic lymphoma kinase (ALK) positive carcinomas progressing after crizotinib approved for ROS1-positive metastatic non-small cell lung cancer (NSCLC). Up until today, limitations based on systemic toxicity, efficacy and safety of the available therapies along with the lukewarm effectiveness in including more selective, targeted delivery strategies, such as nanomedicines: broadly defined as drug delivery systems (nanoDDS) (2, 3).
Nanomedicines, were thought as a first-of-a-kind strategy designed to improve patient outcomes trough, (1) promoting selective deliver of their cargo in the lesion; (2) avoidance of adverse effects in healthy cells, and (3) protecting the pharmacological molecule from earlier metabolization (4). Typically, pharmaceutical innovation and strategy focus their efforts in designing carriers, such as liposomes, polymeric nanoparticles and micelles, specifically devised to improve the efficacy of loaded therapeutic agents by enhancing their bioavailability, stability and body residency upon intravenous administration (5–7). By contrast, there has been little effort to fight diseases, either locally or systemically, using inhaled therapeutics as a delivering system (8). Underexploring pulmonary administration of inhaled nanomedicines, their capability to reduce dosing and to fine-tune known drugs pharmacokinetics (PK), constitutes an unprecedented waste of a medical and economic competitive turning point.
Ever-increasing attention in nanomedicines, either marketed or under clinical trials, have already stressed that it is advisable to encourage a better understanding of this systems features by the regulatory authorities. Efforts already underway include task forces to find a consensus on the requirements for registration and market approval accelerating patient access to those products. Regardless the existing favorable “regulatory environment”2 and further reflection papers on inhaled formulations, the approval process remains heavyweight, unnecessarily time and cost consuming. High quality unbiased evidence on inhaled nanomedicines absorption, distribution, metabolization and elimination (ADME) should be generated based on robust experimental protocols and scientific guidelines. Otherwise, the existent constrains, and regulatory gaps might result in a redundant loss of understanding on how lung mechanistic and physiologic properties do affect drug pharmacology upon pulmonary administration.
Patients and physicians are looking forward to have the augured advanced inhaled nanoDDS pharmaceuticals and its consequent add value. However, despite their promising capabilities, differences in distribution, pharmacology, immunology and toxicology raise numerous issues needing to be addressed to hardness pre-clinical validation of new targets supported by original data collections.
Obstacles in the drug development process toward a broad acceptance of inhaled systems opportunities prompted several initiatives (9), such as the SimInhale working group hosted by the MP1404 COST Action3 By starting to recognize that pulmonary delivery systems and particularly inhaled pharmaceuticals, do represent a shift in drug conventional pharmacokinetics/pharmacodynamics (PK/PD), the challenge was to reassess experimental frameworks enforcing its utility to strengthen regulatory decision-making offices toward inhaled nanoDDSs approval, without losing the ethical safety-centered archetype neither the Quality Target Product Profile (QTPP) [ICH Q8] indispensable to provide safe drugs to patients fostering therefore future regulatory approval and output applications.
Embracing innovative solutions supported by biomedical data gathered from clinical/translational research studies could support clinical decisions that might contribute to improve lung cancer modest outcomes: primary cause of cancer-related death, with more than 1.6 million yearly deaths worldwide (10, 11). Nowadays, lung cancer patients face intensive and invasive treatment protocols that comprise surgery, chemotherapy, radiation therapy, or combinations thereof depending on: (1) the cancer type, (2) the stage of the disease, and (3) individual factors, such as, general health condition and lung function (12, 13).
In 2011, the International Association for the Study of Lung Cancer (IASLC), the American Thoracic Society (ATS), and the European Respiratory Society (ERS) established significant changes to the prior pathologic classification of lung cancer (11), with emphasis on correlations between pathologic aspects of tumors with clinical, radiologic, and molecular characteristic (14).
Conventional histologic-based classification for diagnosis and treatment decision in lung cancer have shown a limited scenario based in the dichotomist classification of small cell lung carcinoma (SCLC) and non-small cell lung carcinoma (NSCLC) (14–17). It lacks important information in terms of tumor “omics”4 thus narrowing the therapeutic possibilities and weakening the already complex rationale that underlies disease prognosis5.
Molecular-driven understanding of tumor growth and progression might positively impact areas, such as diagnosis and patient sub-populations outcomes. As metastatic competencies increase, several intracellular events drive the primary tumor communication with the surrounding microenvironment as well as with distant organs. At that point, it is possible to observe the emergence of complex molecular cascades (18, 19).
Systematic disease mutations in oncogenes crucial for sustaining cells tumorigenic potential, are perfect examples of conditions that could dictate patients stratification making possible to achieve outcomes beyond our current therapeutic expectations (20–22). Key signaling events involved in NSCLC growth, proliferation and tumor suppressive properties are related to the activation of specific oncogenes including through RAS/RAF/MEK/ERK and PI3K/AKT/mTOR axis (23, 24).
Clinical evidence gathered in patients with EGFR mutations or ALK rearrangements drove recent progresses in precision medicine field (25–30). This integrative methodology was used to support the approval of EGFR-TKIs (gefitinib, Iressa, AstraZeneca; erlotinib, Tarceva, Genentech/Astellas) as first-line treatment for patients with advanced NSCLC harboring somatic mutations in EGFR exons 19 and 21. Higher efficacy (progression-free survival (PFS) and safety (lower toxicity when compared with conventional chemotherapy), was reported in a phase III randomized clinical trials (16, 31).
Nanomedicines, mainly as inhaled nanoDDS, can be foreseen as a key “tool” for the implementation of personalized medicine. The concept of delivering selectively a therapeutic strategy to the lung region increasingly drives clinicians and researchers to address the cancer physiopathology as a cell mutation-based injury providing substantial enrichment in clinical disease management.
Disrupting tumor immunosuppressive mechanisms is already used by exploring different concepts: (1) Non-specific immune stimulation (32); (2) Adoptive cell transfer (33); (3) Vaccination strategies (34); and (4) immune checkpoint blockade (35, 36). Unsurprisingly, enhanced immune responses, though associated with better therapeutic outcomes and higher survival rates, might lead to severe toxicities. Nevertheless, the impact of immunotherapies on the induction and re-establishment of the immune system have been shaded mainly by the occurrence of irAEs (immune-related adverse events).
Lung cancers are characterized by the constitutive activation of oncogenes that regulate the abrogation of the normal T-cell's activation, driving tumor escape from the immune surveillance (36). These tumors signatures also rely in disturbance of immune checkpoints, e.g., CTL-4 and PD-1 or PD-L1 (37–40). Interfering with immune checkpoints through monoclonal antibodies (mAb) has been used successfully to amplify dendritic cell (DC)-derived immune responses (41–43).
Differing from the healthy conservative feedback mechanism, tumor's up-regulation of activated T-cells led to the overexpression of CTLA-4, generating a competitive unregulated arrangement (36, 37). Already approved Ipilimumab, a fully humanized anti-CTLA-4 mAb that targets protein receptors at the DC surface (44) is in a phase II trial as a first-line treatment of metastatic NSCLC, in association with chemotherapy. This combination displayed an improvement of the immune related progression-free survival in comparison with chemotherapy alone, without additional toxicity. Tremelimumab is another mAb directed against CTLA-4 that has been developed for several solid tumors, namely NSCLC (45).
Additional strategies against molecular targets have already progressed to clinical use, the first resulting from the pro-inflammatory cytokines released in the tumor microenvironment as well as the initial T-cell activation both up-regulating the membrane receptor PD-1, while the second is the PD-1 ligand (PD-L1), up-regulated by cytokines, such as INF-γ and IL-4, which are produced after T-cell activation, establishing a feedback loop that attenuates tumors immune responses (37, 38, 46–48). Anti PD-1 and PD-L1 therapies, such as nivolumab (Opdivo®), pembrolizumab (Keytruda®), atezolizumab (Tecentriq®), avelumab (Bavencio®), and durvalumab (Imfinzi®) are in clinical use for the treatment or co-treatment of a broad range of malignant stages. FDA-approval of nivolumab (2014) has indications for patients with BRAF V600 wild type or mutation-positive unresectable metastatic melanoma. Lung cancer and specifically NSCLC patients already benefits from this therapy, as it becomes the first immunotherapy to be approved as second-line treatment (2015). Indications have now been extended to advanced renal, hepatocellular, and urothelial carcinomas, metastatic squamous cell carcinoma of the head and neck (SCCHN), classical Hodgkin's lymphoma (cHL) and microsatellite instability-high (MSI-H) or mismatch repair deficient (dMMR) metastatic colorectal cancer (49). Expectations in this molecular candidate have driven new clinical trials aiming: (1) investigate the efficacy of these mAb in combination with small targeted drugs/traditional chemotherapy; (2) to determine optimal administration patterns; and (3) to identify the subset of patients that may benefit the most from the new treatments (50, 51).
Recently, a combination of ipilimumab (anti-CTLA-4) and nivolumab (anti-PD-1) evaluated in a phase II trial against advanced melanoma, provided evidence of an improvement in patient response rate of 61%, compared to 11% for ipilimumab monotherapy, suggesting an exciting potential complementary role in regulating adaptive immunity (52, 53).
As broader utilization becomes a reality, more structured data identifies the strengths and weakness of its use. Unfortunately, from up to 90% of the patients treated with anti-CTLA-4, to 70% regarding to the anti-PD-1 or anti-PD-L1 therapy have experienced irAEs (54). Recent discouraging results have raised questions regarding the criteria to be followed for the selection of patients who may benefit from the use of anti-PD-1 monoclonal antibodies, e.g., patients with EGFR mutations or patients previously treated with TKIs (55, 56).
Lastly, despite some breakthrough innovations in the present clinical adopted protocols, the inclusion of conventional antitumor agents seems to remain mandatory. Together, the lack of cell selectivity and the known severe toxic side effects explain dose-limiting issues and consequent modest results in terms of survival rates. Adoption of precision medicines involves not only new molecules, supported by breakthrough knowledge in cell biology, but also their combination with novel administration strategies. Highly competitive therapeutic or diagnostic options could be foreseen by using pulmonary delivery of nanomedicines, either by addressing newly identified disease targets, thus solving unmet medical needs in lung cancer, or by decreasing conventional drugs administered doses (2, 3).
Though it is perhaps, unfair to claim that the actual classification of a tumor stage has been made over a conceptual weakness, the tremendously limited organ-based differentiation moves to a more precise mutation-driven disease classification.
Looking ahead, the sustainability of exploiting cell growth, signal transduction, angiogenesis, metastasis and cell cycle regulation as targets for new therapies regardless the cancer type may rely on nanoDDS transformative impact on the field. Bringing together new safety, quality and efficacy data, attracts the attention of regulatory authorities, and by doing so, genetically defined patients would greatly benefit from inhaled nanoDDS to delivery highly selective molecular-target agents or repurposing old, but indispensable, molecules.
On top of the opportunities offered by nanoDDS as carriers for a broad range of therapeutic entities, it is now possible to capture some of the nanoparticle's features to achieve optimal pulmonary administration and radically change “drugs” bioavailability. Nonetheless, for aerodynamic engineered nanoparticles, tracking their biodistribution from initial particle deposition region through the alveolar and interstitial space to the drainage routes—pulmonary arteries, veins and lymphatic's is mandatory, together with identification of possible toxicological effects. Though the acquired knowledge regarding lung dynamics, namely the complex network responsible for the drainage of intrapulmonary fluids, also involved in cancer progression and metastases dissemination, emphasizes that no “simple approach” can be devised. Nevertheless, nanoDDS PK/PD, based upon the nanoparticle properties rather than the molecule's biochemical classification (BCC) can be design to improve lung distribution and deposition providing higher local pharmacological doses with attenuated systemic adverse effects (57, 58).
Recent breakthroughs in the field of molecular biology and developments in RNA interference (RNAi) technology have promoted the therapeutic use of RNAi-mediated strategies in the oncologic field. Particularly, the use of short interfering RNA (siRNA) arose as a reliable therapeutic approach by which harmful genes can be “silenced” by delivering complementary and rationally designed sequences (59–62). Unlike conventional anti-cancer drugs (e.g., small molecules and antibody-based drugs), siRNA therapeutic potential is enormous due to unlimited possibilities in terms of targets and specificity, which are determined by the principle of complementary base pairing (63). Compare to other anti-sense alternatives, such as, DNA oligonucleotides, this approach is known to be safer, as it interferes at the post-translational level, avoiding mutation and teratogenicity risks (62, 64).
One of the major challenges in the siRNA field is the dependence of therapeutic efficacy on effective cell targeting. It is accepted that both cell uptake and intracellular trafficking are critical to promote intracellular accumulation of these molecular-target agents. Furthermore, limitations are also found in systemic administration, owing its quick degradation by body nucleases and clearance from the bloodstream. Unlike other molecules, siRNA biological fate is totally determined by the existence and properties of a carrier-mediated delivery system and its ability to overcome both extracellular and intracellular barriers (62).
Nanomedicines might promptly change the limitations already faced with intracellular delivery of naked oligonucleotides. The protection and selective targeting can undoubtedly generate opportunities for molecular-target agent's translation from bench to clinical use can be anticipated.
siRNA-nanocarriers engineered to tune selectivity of advanced nanoDDS liposomes, polymeric nanoparticles, micelles, solid lipid nanoparticles and inorganic nanoparticles could be designed in order to promote higher bioavailability, stability and residency times at the lung (65–67). For this reason, inhaled siRNA-based therapy deserves to be explored. The direct and localized administration of inhaled nano-entrapped siRNA might allow higher accumulation of siRNA in the tumor vicinity, being potentially the most valuable tool for the biomedical RNAi application in the near future (68). Newly identified cell targets and preliminary clinical findings with siRNA-nanocarriers reveal broad and diverse opportunities to impair tumor growth and dissemination (62).
Shim et al. (69) described the pulmonary delivery of cationic lipoplexes against myeloid cell leukemia sequence (siRNA, siMcl1). Different nanoliposomes exhibited similar behavior in vitro (in B16F10 cell lines), but variations in vivo (mouse). The nanoliposomes prepared with cationic dioleoyl-sn-glycero-3-ethylphosphocholine and cholesterol (ECL) presented the highest pulmonary delivery in vivo (26.2-fold higher than naked siRNA) and also the lowest cytotoxicity in vitro among the formulations tested. According to this study, the intra-tracheal administration of siMcl1 in ECL lipoplexes was able to significantly silence Mcl1 mRNA and its protein levels in the lungs, while reducing the formation of melanoma tumor nodules. These findings support the use of ECL nanoliposomes for pulmonary delivery of therapeutic siRNA to address lung cancer and other respiratory pathologies (69).
Currently Alnilam's cholesterol-modified siRNA for RSV treatment which is currently in Phase IIb and ExcellairTM (ZaBeCor, Bala Cynwyd, PA, USA), an inhaled siRNA-based treatment of asthma are the most used inhaled systems undergoing clinical trials (70).
Whichever the transported pharmacological agent, innovation, drug discovery and subsequent clinical trials have been mostly focused on intravenous administration, a route closely related to drug's adverse effects (71).
Exploring alternative administration routes, e.g., lung administration, are urgently required to offer a selective and more effective therapeutic delivery while preventing drug accumulation at non-targeted tissues. This innovative strategy also aims to reduce clinical adverse effects avoiding dose-limiting toxicities. Advances emerging from the use of nanomedicines are a major source of improvements that can be applied to explore this regional route of administration to handle lung diseases with emphasis in lung carcinomas (72, 73).
In line with this observation, data from our research group shown activity against breast metastasis in lung, when paclitaxel entrapped in a lipid carrier system was administered in vivo by inhalation (74)6 Other experiments performed within our research team reinforced this inhaled nanoDDS efficacy against lung regional and distant metastasis in vivo upon transplantation of a spontaneous metastases model of the malignant breast cancer sub-population (MXT C1.1) (Figure 1). The entrapped drug (paclitaxel) proved to be more efficient when compared with the free drug administered I.V (74)6.
Figure 1. MXT C1.1 was selected due to its mesenchymal phenotype and its ability to produce lung metastases in vivo. Orthotopic implantation of cells (1 × 106 cells) in the mammary fat pad of female BALB/c mice (Harlan Iberica, Barcelona, SP) was used to develop the primary tumor. Dissemination in distant organs, such as the pulmonary region was confirmed by histomorphology of the animal's lungs at the study endpoint (42 days). Animals were divided according to the inhaled formulation (randomized groups, n = 10): untreated control (group 1), plain SLN (group 2), paclitaxel-lipid nanoparticles (group 3). Each group received 6 administrations as depicted at the photo A mean tumor volume of 0.5 mm2 in the control group was considered the study “end point.” The primary tumors volumes were measured using a Vernier caliper. The results were expressed as the following: the prevalence of detected lung metastasis, as well as number and volume. The correlation between lung metastasis invasion with the paclitaxel-lipid nanoparticles inhalation was evaluated by regression analysis. P-values <0.05 were considered statistically significant.
Tomoda et al. (75) reported a TAS-103 loaded PLGA-based nanomedicines against lung cancer, formulated in the form of a nanocomposite and administered by inhalation to rats. The authors found that the drug concentration in the lungs, following pulmonary administration, was significantly higher when compared to that obtained by I.V. of the free drug (75). Therapeutic evidences prove the potential of pulmonary administration of nanomedicines to the management of lung cancer or other cancer lung invasion (76).
The effective transition of novel nanomedicines from experimental into the clinical arena demands a proper assessment of the properties forming the basis of their use (proof of concept plus kinetics plus toxicity). The relationship between the administered dose and the amount of drug deposited in the lung, the regional distribution, the residence time and/or clearance to the lung region or circulatory system need to be assessed for proper therapy planning (77).
For conventional drugs, this information is initially obtained in pre-clinical studies in animals and other test systems, using classical approaches, e.g., by analysis of biological samples (tissues, fluids) using a combination of techniques, such as high-performance liquid chromatography (HPLC) and mass spectrometry (MS) (78). In the case of nanomedicines, one of the main challenges arises from the fact that nanoparticles are extremely difficult to detect and quantify once distributed in an organism. Additionally, degradation or aggregation of the nanocarriers, interaction with biomolecules leading to alteration of the biological fate, and the process of drug release are extremely difficult to investigate using classical approaches (75, 79).
One strategy complementary to classical approaches for the tracking and quantification of nanoparticle-based complexes consists of using labeled particles, which implies the creation and use of dedicated technology for, (1) the labeling, (2) the visualization at organ, tissue, cellular and/or subcellular level of particles and drug-nanoparticle complexes, and (3) the quantification of nanoparticle-drug complexes, empty nanoparticles, non-complexed therapeutic molecule and degradation products (79, 80).
In vivo, ex vivo, and in vitro test systems are available or under development for the purposes above described, such as Positron Emission Tomography (PET) and Single Photon Emission Computerized Tomography (SPECT) (81). An excellent alternative to optical imaging techniques is the use of positron or gamma emitters. The disintegration of positron and gamma emitters leads ultimately to the generation of gamma rays (in the case of positron emitters, as the result of the annihilation of the emitted positron with an electron of a surrounding atom) which have virtually no penetration limits. Hence, if positron- or gamma emitter-radiolabeled nanomedicines are administered to experimental animals or eventually humans, the gamma rays resulting from the disintegration process can be externally detected and processed to generate 3D-images, which provide quantitative information about the spatiotemporal distribution of the labeled specie This is the basis of nuclear imaging techniques, i.e., PET and SPECT. When applying nuclear imaging techniques, appropriate selection of the labeling strategy is paramount to guarantee reliable results. The labeling strategy will ultimately depend on the physicochemical properties of the nanocarrier/drug, the selected radionuclide and the duration of the biological process to be investigated (81).
Critical success factors such, as the accurate quantification in vivo owing the breathing-related motion of the subject during image acquisition. Of note, gated imaging can be applied to correct for this; alternatively, in the preclinical setting excision of the organs of interest and ex vivo imaging can solve this problem, at the cost of animal sacrifice. Additionally, resolution of nuclear imaging is usually poor (around 1 mm in the center of the field of view for preclinical systems, close to 5 mm for clinical systems). If higher resolution images are required, tissue dissection, slicing and evaluation using autoradiography can provide accurate information about regional distribution of the administered drug with a resolution close to 50 μm (Figure 2) (81).
Figure 2. Representative workflow of the use of nuclear imaging to gain information related to the distribution of NCs after intratracheal insufflation. Gallium-67 labeled LPs (A) are administered to healthy rats. (B) In vivo (C) or Ex vivo (E) images can be obtained using SPECT. In vivo imaging enables quantification of the regional distribution. (D) Ex vivo autoradiography studies provide information about distribution at a higher spatial resolution (F) bottom. A photograph of the tissue slice is also shown (F) top. In the case shown, liposomes (LPs, as a model of nanocarrier) were labeled with Gallium-67 (gamma emitter, T1/2 = 3.3 days, Emax = 93, 184, and 296 keV) using a trans chelation method. The LPs were administered to healthy rats using the Penn-Century insufflators, and In vivo images were acquired using SPECT, enabling the determination of the regional distribution of the NCs within the lungs by drawing volumes of interest in the different lung lobes. In parallel studies, the lungs were harvested after appropriate tissue fixation and imaged using SPECT, resulting in more detailed images due to suppression of lung motion. Finally, autoradiography studies on tissue slices provided information about distribution at a higher spatial resolution.
Incorporation of labels both at the nanocarrier and the drug and subsequent tracking of the location of the different components integrating the nanomedicine can be envisaged, and information regarding biodistribution, residence time in the target organ or tissue and drug delivery kinetics in vivo might be achieved. This strategy, to the best of our knowledge, has so far not been applied to the investigation of drug loaded nanocarriers. However, recent works demonstrate the feasibility of the approach and hence energy-discriminant SPECT might become a powerful tool to gain information about the in vivo behavior of nanomedicines in the near future (80, 82).
For inhaled drugs, bioavailability after pulmonary administration is a function not only of its characteristics, but also of the pharmaceutical formula and the physiological barriers that it must overcome. It has been postulated that the physiological deposition and adsorption mechanisms could be estimated based on the aerodynamic diameter of the inhaled agents. But this is a limited and controversy way to solve the problem and for the scientific way to solve the problem and for the scientific community does not take in consideration the lung histopathological state (83).
Few harmonized recommendations, such as the EMA/CHMP/QWP/49313/2005corr: Guideline on the Pharmaceutical Quality for Inhalation and Nasal Products; 3/26, have contributed for a harmonized environment mostly relative to chronic, combined chronic and carcinogenicity and carcinogenicity testing (84, 85).
The existing requirements for safety and efficacy evidence may not be adequate to describe nanoDDS' lung deposition and clearance. More important, conventional measure of AUC and Cmax do not accurately predict the real clinical response upon pulmonary delivery, both at local and systemic level.
Given the different aspects, mainly the size and morphology, the evaluation of the nanosized-formulation impact on the safety attributes of the active agent shouldn't be focused on conventional pre-clinical tests alone. Up to now, functional pulmonary imaging technologies represent an important tool for the biodistribution assessment of nebulized nanoparticles.
Regarding existing ICH guidance on the non-clinical requirements for support of clinical development and marketing authorization of medicinal products, mainly related to safety pharmacology the already existing guidelines (ICH 7a and I7b) and ICH topic M3 (2) lack aspects related to the lung specificity. This underlines the question: if the pre-clinical existing package seems to be insufficient for fostering the approval of the advanced inhaled pharmaceuticals what should be done to avoid this gap? This means a review on many aspects related to the value of non-clinical conventional safety evaluation (ICH Topic M3 (R2), biological issues associated with the active pharmaceutical ingredient bioavailability upon lung deposition but also considering critical the role of excipients, known and new, that are being used for preparing pulmonary delivery formulations.
Massive information has been gathered from research over the pulmonary administration route mechanisms and toxicological aspects. Not considering this fragment of evidence on emerging inhaled nanomedicines and their particularities represents a disadvantage for both industry and police makers.
Different situations may emerge in this exercise, for the development of medicinal products to administer by the inhalation route, (1) the repurposing of an existing nanomedicine, (2) the repurposing of an approved molecule using an innovative nanomedicine, (3) innovative molecule and formulation with well-characterized nanoparticles, and (4) innovative molecule and formulation with newly developed nanoparticles.
Given the relatively large spectrum of proven or potentially useful molecular entities currently available, which show activity in lung cancer, or in non-lung tumors, but with plausible mode of action justifying their study in lung cancer, the hypothesis (1) and (2) might be the most straightforward for improving the tumor access of drugs through their local administration. For both concepts to be applied into nanomedicines development, multiple requests need to be fulfilled in what concerns the characterization of the biological effects and of the methodological tools used, e.g., the inhaled route and the delivery systems (Figure 3).
Figure 3. Different paths of regulatory requirements are summarized in the schematic diagram. Investigational new drug applications (IND), using existing or innovative nanoparticle formulations, will benefit from images supporting safety and efficacy evidence to complete “non-image” conventional end-points, pre-clinically and clinically, including quality and efficacy proof of concept, PK/PD, sub-acute to chronic toxicity, repeated dose toxicity, genotoxicity, carcinogenicity, reproductive toxicity, etc. Functional medical images of non-invasive lung administration either for inhaled active molecules or image agents can strengths the approval decision-make process. Bearing this in mind, regulatory acceptance of imaging-based evidence might have a time reduction impact in the benefit-risk assessment whenever image-guided “drug” delivery provides clinically significant data. Owing its potential to cover “drug” biodistribution cycle including body response and tissue alterations, and knowing that most of the new targetable cell events are common in cancer growth and survival, it is expectable that innovative molecules, targeting newly identified cell function alterations/abnormal signaling cascades, might have the decision-make process abbreviated even for broader indications.
The clinical testing of such medicines demands a previous non-clinical estimation of the benefits (through proof of concepts) and of the risks posed by the new (pulmonary/inhaled) route of administration. Relevant methodologies are available, as described above, to address the distribution of the nanoDDS across the respiratory tract, their potential accumulation in the tumor vs. healthy cells, the intracellular distribution in relevant T-cells, and the biodistribution from the sites of administration and accumulation into the systemic circulation, with subsequent clearance. The comparison of these aspects within the delivery routes, e.g., the inhaled vs. the approved non-inhalation route (like I.V., or oral) will inform on the type and extension of studies that will be needed to support the entry into human trials.
Existing modeling and simulation approaches, together with tests in artificial respiratory tract/lung equipment's are becoming important tools for planning the particle characteristics vs. the intended deposition across the respiratory tract. If the systemic exposure, including tissue distribution of the inhaled formulation will be, as expected, lower than the observed with the approved molecule and formulation, the preclinical requirements will be mostly circumscribed to the local effects in the respiratory tract, meaning that a short-term repeated dose studies in one or two species might suffice to support the entry into human use.
On the contrary, depending on the comparative exposures reached systemically with the approved molecule vs. its innovative inhaled nanomedicines, a more extensive program will be needed, fully characterizing the (nano) particles, pre-clinical profile (e.g., ADME, long term toxicity, cellular fate) as well as the local toxicity.
This opens up countless opportunities for using the accumulated knowledge on existing molecules developed for non-lung tumors to support and abbreviate their development under inhaled formulations for lung cancer. The simplification of the development programs will allow faster patient access to innovation, but also will allow a faster success or failure together with increased knowledge on the relevance of the targeted cascades on the newly tested lung tumor(s), which are the remit of this manuscript. For now, it is important to focus our attention in toxicological matters rather than pharmacology and pharmacokinetics.
The pulmonary route of administration embodies local/regional drug delivery against multiple diseases of the lung and the respiratory tract, e.g., asthma or cystic fibrosis. The systemic access of drugs through the lung has been attempted with limited success, as has been the case on inhaled insulin or calcitonin. Though this exploratory systemic administration shows great potential, information regarding possible advantages and challenges are out of this work scope.
The enormously potential value of the development of inhaled nanomedicines has been illustrated in this manuscript specifically for regional management of lung cancer or other cancers invasion in the lung compartment. This incurable disease may embody situations where a cost-benefit could be achieved by the local delivery of anticancer products. Furthermore, pulmonary administration followed by regional nanoDDS deposition would be expected to promote faster and more direct access of drugs to the tumor microenvironment, higher pharmacological concentrations even though with decreasing administered dosing and at the same time, reducing the systemic exposure and associated toxicities.
The increasing knowledge on the mechanisms behind tumors environment and the associated cellular/functional cascades, provides evidences on shared mechanisms not exclusive of one tumor type, making possible to approve therapeutic strategies with indications for multiple tumors in different organs. In another words, tumor characterization based on identified mutations rather than lesions in specific tissues/organs open up the rationale for shared therapies among multiple cancer situations independent of their location.
Numerous challenges need to be overcome for a successful and efficient pulmonary delivery of antitumor products, including the “construction” of appropriate formulations based on nanoparticles having appropriated quality attributes for reaching and targeting the intended cell, within the “geography” of the respiratory system, taking into consideration the physiopathological changes inherent to the disease itself.
Furthermore, any innovative formulation aiming to deliver new or existing therapeutic molecules will need to undergo a pre-clinical and clinical development program for supporting the marketing authorization stage and further human administration. Pre-clinical programs will include proof of concept, PK/PD and toxicology studies, being the extension of the studies determined by the innovative components of the developing medicines.
Medicines repurposing will be a consequence of these new approaches, which mat allow a faster application of innovation to multiple situations. The preclinical programs may therefore be reduced and adjusted when the repurposing of a drug is attempted for changing the target organ of efficacy. A faster and better patient access to innovative treatments will be a direct beneficial consequence. These considerations apply to pulmonary delivery of existing medicines approved for use through other administration routes or intended for different organ targeting.
Tracking nanoDDS across the pulmonary tract, different lung cells and eventually subcellular systems, demands technical solutions that are emerging, such as imaging technologies. Nevertheless, it is expectable that one of the higher advantages of local pulmonary delivery of antitumor drugs, particularly for lung cancer will be the reduction of the doses needed and reduction of systemic toxicity.
The early and continuous dialogue between the scientists involved in the development process and regulators will be fundamental for the design of well-structured, potentially more simplified development programs, pre-clinical and/or clinical, to allow an earlier access and benefit of patients to those innovations.
MV, JL, and CS managed the writing/manuscript preparation and data presentation. MV and JL were also responsible for the funding acquisition. BK, IV, BF, and BS-L conducted and prepared the manuscript section about toxicity and regulatory issues. NG provided a critical review on the clinical status and UC conducted the experimental work.
The authors declare that the research was conducted in the absence of any commercial or financial relationships that could be construed as a potential conflict of interest.
MV acknowledges the Portuguese Science and Technology Foundation (PTDC/SAU-FAR/120453/2010) and COST Action MP1404, SimInahle. The work was partially funded by iMed.ULisboa (UID/DTP/04138/2013) from Fundação para a Ciência e a Tecnologia (FCT), Portugal. JL acknowledges European Commission, FP7-NMP-2013-LARGE-7, project reference 604434 and the Ministerio de Economía y Competitividad (MINECO, project MAT2013-48169-R) for financial support, and Prof. Dr. Gert Storm for kindly providing the liposomes.
1. ^EMEA/CHMP/QWP/49313/2005: Guideline on the Pharmaceutical Quality of Inhalation and Nasal Products; OECD/ 1085/2003: Guideline for Testing of Chemicals No. 413. “Subchronic Inhalation Toxicity: 90-Day Study.
2. ^The European Medicines Agency's scientific guidelines on nanomedicines help medicine developers prepare marketing authorization applications for human medicines.
3. ^COST (CO-operation in Science and Technology). Pan-European intergovernmental framework dedicated to European-based S&T networking activities under the EU Framework Program Horizon 2020. https://www.cost.eu/who-we-are/about-cost/.
4. ^Genomics, proteomics, transcriptomics, etc.
5. ^NSCLCs (80–85% of lung cancer) were grouped together disregarding more specific histologic subtypes (i.e., adenocarcinoma, squamous cell carcinoma, and large cell carcinoma), though no differences in the therapeutic systemic approach where considered for each subtype.
6. ^Dissertation thesis is available in Repositório da Universidade de Lisboa in the following URL: http://hdl.handle.net/10451/271.
1. Cosio MG, Saetta M, Agusti A. Immunologic aspects of chronic obstructive pulmonary disease. N Engl J Med. (2009) 360:2445. doi: 10.1056/NEJMra0804752
2. Burris HA. Shortcomings of current therapies for non-small-cell lung cancer: unmet medical needs. Oncogene. (2009) 28:S4–13. doi: 10.1038/onc.2009.196
3. Yun YH, Shon E-J, Yang A-J, Kim SH, Kim YA, Chang YJ, et al. Needs regarding care and factors associated with unmet needs in disease-free survivors of surgically treated lung cancer. Ann Oncol. (2013) 24:1552–9. doi: 10.1093/annonc/mdt032
4. Wolfram J, Ferrari M. Clinical cancer nanomedicine. Nano Today. (2019) 25:85–98. doi: 10.1016/j.nantod.2019.02.005
5. Plattt VM, Szoka FC. Anticancer therapeutics: targeting macromolecules and nanocarriers to hyaluronan or CD44, a hyaluronan receptor. Mol Pharm. (2008) 5:474–86. doi: 10.1021/mp800024g
6. Kumari A, Yadav SK, Yadav SC. Biodegradable polymeric nanoparticles based drug delivery systems. Colloids Surf B Biointerf. (2010) 75:1–18. doi: 10.1016/j.colsurfb.2009.09.001
7. Biswas S, Torchilin VP. Nanopreparations for organelle-specific delivery in cancer. Adv Drug Deliv Rev. (2014) 66:26–41. doi: 10.1016/j.addr.2013.11.004
8. Wilhelm S, Tavares AJ, Dai Q, Ohta S, Audet J, Dvorak HF, et al. Analysis of nanoparticle delivery to tumours. Nat Rev Mater. (2016) 1:16014. doi: 10.1038/natrevmats.2016.14
9. Hochhaus G, Davis-Cutting C, Oliver M, Lee SL, Lyapustina S. Current scientific and regulatory approaches for development of orally inhaled and nasal drug products: overview of the IPAC-RS/university of florida orlando inhalation conference. AAPS J. (2015) 17:1305–11. doi: 10.1208/s12248-015-9791-z
10. Mariotto AB, Yabroff KR, Shao Y, Feuer EJ, Brown ML. Projections of the cost of cancer care in the Unites States: 2010-2020. J Natl Cancer Inst. (2010) 103:117–28. doi: 10.1093/jnci/djq495
11. Stewart BW, Wild C, International Agency for Research on Cancer, World Health Organization. World Cancer Report. (2014). Available online at: http://publications.iarc.fr/Non-Series-Publications/World-Cancer-Reports/World-Cancer-Report-2014 (accessed August 23, 2018).
12. Novello S, Barlesi F, Califano R, Cufer T, Ekman S, Levra MG, et al. Metastatic non-small-cell lung cancer: ESMO Clinical Practice Guidelines for diagnosis, treatment and follow-up. Ann Oncol. (2016) 27:v1–27. doi: 10.1093/annonc/mdw326
13. Postmus PE, Kerr KM, Oudkerk M, Senan S, Waller DA, Vansteenkiste J, et al. Early and locally advanced non-small-cell lung cancer (NSCLC): ESMO Clinical Practice Guidelines for diagnosis, treatment and follow-up. Ann Oncol. (2017) 28:iv1–21. doi: 10.1093/annonc/mdx222
14. Travis WD. Pathology of lung cancer. Clin. Chest Med. (2002) 23:65–81. doi: 10.1016/S0272-5231(03)00061-3
15. Travis WD, Brambilla E, Noguchi M, Nicholson AG, Geisinger KR, Yatabe Y, et al. International association for the study of lung cancer/american thoracic society/European respiratory society international multidisciplinary classification of lung adenocarcinoma. J Thorac Oncol. (2011) 6:244–85. doi: 10.1097/JTO.0b013e318206a221
16. Travis WD, Brambilla E, Riely GJ. New pathologic classification of lung cancer: relevance for clinical practice and clinical trials. J Clin Oncol. (2013) 31:992–1001. doi: 10.1200/JCO.2012.46.9270
17. Travis WD, Brambilla E, Nicholson AG, Yatabe Y, Austin JHM, Beasley MB, et al. The 2015 world health organization classification of lung tumors. J Thorac Oncol. (2015) 10, 1243–60. doi: 10.1097/JTO.0000000000000630
18. Ding L, Getz G, Wheeler DA, Mardis ER, Mclellan MD, Cibulskis K, et al. Somatic mutations affect key pathways in lung adenocarcinoma. Nature. (2008) 455:1069–75. doi: 10.1038/nature07423
19. MacKinnon AC, Kopatz J, Sethi T. The molecular and cellular biology of lung cancer: identifying novel therapeutic strategies. Br Med Bull. (2010) 95:47–61. doi: 10.1093/bmb/ldq023
20. Mascaux C, Iannino N, Martin B, Paesmans M, Berghmans T, Dusart M, et al. The role of RAS oncogene in survival of patients with lung cancer: a systematic review of the literature with meta-analysis. Br J Cancer. (2005) 92:131–9. doi: 10.1038/sj.bjc.6602258
21. Karachaliou N, Mayo C, Costa C, Magrí I, Gimenez-Capitan A, Molina-Vila MA, et al. KRAS mutations in lung cancer. Clin Lung Cancer. (2013) 14:205–14. doi: 10.1016/j.cllc.2012.09.007
22. Chen F, Zhang Y, Parra E, Rodriguez J, Behrens C, Akbani R, et al. Multiplatform-based molecular subtypes of non-small cell lung cancer. Oncogene. (2017) 36:1384–93. doi: 10.1038/onc.2016.303
23. Choi YL, Takeuchi K, Soda M, Inamura K, Togashi Y, Hatano S, et al. Identification of novel isoforms of the EML4-ALK transforming gene in non-small cell lung cancer. Cancer Res. (2008) 68:4971–6. doi: 10.1158/0008-5472.CAN-07-6158
24. Koivunen JP, Mermel C, Zejnullahu K, Murphy C, Lifshits E, Holmes AJ, et al. EML4-ALK fusion gene and efficacy of an ALK kinase inhibitor in lung cancer. Clin Cancer Res. (2008) 14:4275–83. doi: 10.1158/1078-0432.CCR-08-0168
25. Lynch TJ, Bell DW, Sordella R, Gurubhagavatula S, Okimoto RA, Brannigan BW, et al. Activating mutations in the epidermal growth factor receptor underlying responsiveness of non–small-cell lung cancer to gefitinib. N Engl J Med. (2004) 350:2129–39. doi: 10.1056/NEJMoa040938
26. Pao W, Miller V, Zakowski M, Doherty J, Politi K, Sarkaria I, et al. EGF receptor gene mutations are common in lung cancers from “never smokers” and are associated with sensitivity of tumors to gefitinib and erlotinib. Proc Natl Acad Sci USA. (2004) 101:13306–11. doi: 10.1073/pnas.0405220101
27. Takeuchi K, Lim Choi Y, Soda M, Inamura K, Togashi Y, Hatano S, et al. Multiplex reverse transcription-PCR screening for EML4-ALK fusion transcripts. Clin Cancer Res. (2008) 14:6618–24. doi: 10.1158/1078-0432.CCR-08-1018
28. Shaw AT, Kim D-W, Mehra R, Tan DSW, Felip E, Chow LQM, et al. Ceritinib in ALK-rearranged non–small-cell lung cancer. N Engl J Med. (2014) 370:1189–97. doi: 10.1056/NEJMoa1311107
29. Zhang Z, Stiegler AL, Boggon TJ, Kobayashi S, Halmos B. EGFR-mutated lung cancer: a paradigm of molecular oncology. Oncotarget. (2010) 1:497–514. doi: 10.18632/oncotarget.186
30. da Cunha Santos G, Shepherd FA, Tsao MS. EGFR mutations and lung cancer. Annu Rev Pathol Mech Dis. (2011) 6:49–69. doi: 10.1146/annurev-pathol-011110-130206
31. Sgambato A, Casaluce F, Maione P, Rossi A, Rossi E, Napolitano A, et al. The role of EGFR tyrosine kinase inhibitors in the first-line treatment of advanced non small cell lung cancer patients harboring EGFR mutation. Curr Med Chem. (2012) 19:3337–52. doi: 10.2174/092986712801215973
32. Monjazeb AM, Hsiao H-H, Sckisel GD, Murphy WJ. The role of antigen-specific and non-specific immunotherapy in the treatment of cancer. J Immunotoxicol. (2012) 9:248–58. doi: 10.3109/1547691X.2012.685527
33. Rosenberg SA, Restifo NP, Yang JC, Morgan RA, Dudley ME. Adoptive cell transfer: a clinical path to effective cancer immunotherapy. Nat Rev Cancer. (2008) 8:299–308. doi: 10.1038/nrc2355
34. Guo C, Manjili MH, Subjeck JR, Sarkar D, Fisher PB, Wang X-Y. Therapeutic cancer vaccines: past, present and future. Adv Cancer Res. (2013) 119:421–75. doi: 10.1016/B978-0-12-407190-2.00007-1
35. Drake CG, Jaffee E, Pardoll DM. Mechanisms of immune evasion by tumors. Adv Immunol. (2006) 90:51–81. doi: 10.1016/S0065-2776(06)90002-9
36. Mellman I, Coukos G, Dranoff G. Cancer immunotherapy comes of age. Nature. (2011) 480:480–9. doi: 10.1038/nature10673
37. Sharpe AH, Abbas AK. T-cell costimulation—biology, therapeutic potential, and challenges. N Engl J Med. (2006) 355:973–5. doi: 10.1056/NEJMp068087
38. Butte MJ, Keir ME, Phamduy TB, Sharpe AH, Freeman GJ. Programmed death-1 ligand 1 interacts specifically with the B7-1 costimulatory molecule to inhibit T cell responses. Immunity. (2007) 27:111–22. doi: 10.1016/j.immuni.2007.05.016
39. Fife BT, Pauken KE, Eagar TN, Obu T, Wu J, Tang Q, et al. Interactions between PD-1 and PD-L1 promote tolerance by blocking the TCR-induced stop signal. Nat Immunol. (2009) 10:1185–92. doi: 10.1038/ni.1790
40. Homet Moreno B, Ribas A. Anti-programmed cell death protein-1/ligand-1 therapy in different cancers. Br J Cancer. (2015) 112:1421–7. doi: 10.1038/bjc.2015.124
41. Creelan BC. Update on immune checkpoint inhibitors in lung cancer. Cancer Control. (2014) 21:80–9. doi: 10.1177/107327481402100112
42. Anagnostou VK, Brahmer JR. Cancer immunotherapy: a future paradigm shift in the treatment of non-small cell lung cancer. Clin Cancer Res. (2015) 21:976–84. doi: 10.1158/1078-0432.CCR-14-1187
43. Iwai Y, Hamanishi J, Chamoto K, Honjo T. Cancer immunotherapies targeting the PD-1 signaling pathway. J Biomed Sci. (2017) 24:26. doi: 10.1186/s12929-017-0329-9
44. Tomasini P, Khobta N, Greillier L, Barlesi F. Ipilimumab: its potential in non-small cell lung cancer. Ther Adv Med Oncol. (2012) 4:43–50. doi: 10.1177/1758834011431718
45. Tarhini AA. Tremelimumab: a review of development to date in solid tumors. Immunotherapy. (2013) 5:215–29. doi: 10.2217/imt.13.9
46. Agata Y, Kawasaki A, Nishimura H, Ishida Y, Tsubat T, Yagita H, et al. Expression of the PD-1 antigen on the surface of stimulated mouse T and B lymphocytes. Int Immunol. (1996) 8:765–72. doi: 10.1093/intimm/8.5.765
47. Sauce D, Almeida JR, Larsen M, Haro L, Autran B, Freeman GJ, et al. PD-1 expression on human CD8 T cells depends on both state of differentiation and activation status. AIDS. (2007) 21:2005–13. doi: 10.1097/QAD.0b013e3282eee548
48. Ostrand-Rosenberg S, Horn LA, Haile ST. The programmed death-1 immune-suppressive pathway: barrier to antitumor immunity. J Immunol. (2014) 193:3835–41. doi: 10.4049/jimmunol.1401572
49. Raedler LA. Opdivo (nivolumab): second PD-1 inhibitor receives FDA approval for unresectable or metastatic melanoma. Am Heal Drug Benefits. (2015) 8:180–3. Available online at: http://www.ncbi.nlm.nih.gov/pubmed/26629287 (accessed November 25, 2019).
50. Topalian SL, Hodi FS, Brahmer JR, Gettinger SN, Smith DC, McDermott DF, et al. Safety, activity, and immune correlates of anti–PD-1 antibody in cancer. N Engl J Med. (2012) 366:2443–54. doi: 10.1056/NEJMoa1200690
51. Shih K, Arkenau HT, Infante JR. Clinical impact of checkpoint inhibitors as novel cancer therapies. Drugs. (2014) 74:1993–2013. doi: 10.1007/s40265-014-0305-6
52. Larkin J, Chiarion-Sileni V, Gonzalez R, Grob JJ, Cowey CL, Lao CD, et al. Combined nivolumab and ipilimumab or monotherapy in untreated melanoma. N Engl J Med. (2015) 373:23–34. doi: 10.1056/NEJMoa1504030
53. Postow MA, Chesney J, Pavlick AC, Robert C, Grossmann K, McDermott D, et al. Nivolumab and ipilimumab versus ipilimumab in untreated melanoma. N Engl J Med. (2015) 372:2006–17. doi: 10.1056/NEJMoa1414428
54. Diesendruck Y, Benhar I. Novel immune check point inhibiting antibodies in cancer therapy—opportunities and challenges. Drug Resist Updat. (2017) 30:39–47. doi: 10.1016/j.drup.2017.02.001
55. Goswami T, Canelos P, Parikh R. A phase Ib/II open-label study to evaluate the safety and efficacy of MEDI-551 in combination with immunomodulating therapy in patients with relapsed or refractory aggressive B cell lymphomas. J Immunother Cancer. 2(Suppl. 3):P73. doi: 10.1186/2051-1426-2-S3-P73
56. Westin JR, Chu F, Zhang M, Fayad LE, Kwak LW, Fowler N, et al. Safety and activity of PD1 blockade by pidilizumab in combination with rituximab in patients with relapsed follicular lymphoma: a single group, open-label, phase 2 trial. Lancet Oncol. (2014) 15:69–77. doi: 10.1016/S1470-2045(13)70551-5
57. Videira MA, Botelho MF, Santos AC, Gouveia LF, Pedroso de Lima JJ, Almeida AJ. Lymphatic uptake of pulmonary delivered radiolabelled solid lipid nanoparticles. J Drug Target. (2002) 10:607–13. doi: 10.1080/1061186021000054933
58. Zhang X-Y, Lu W-Y. Recent advances in lymphatic targeted drug delivery system for tumor metastasis. Cancer Biol Med. (2014) 11:247–54. doi: 10.7497/j.issn.2095-3941.2014.04.003
59. Fire A, Xu S, Montgomery MK, Kostas SA, Driver SE, Mello CC. Potent and specific genetic interference by double-stranded RNA in caenorhabditis elegans. Nature. (1998) 391:806–11. doi: 10.1038/35888
60. Almeida R, Allshire RC. RNA silencing and genome regulation. Trends Cell Biol. (2005) 15:251–8. doi: 10.1016/j.tcb.2005.03.006
61. Tiemann K, Rossi JJ. RNAi-based therapeutics-current status, challenges and prospects. EMBO Mol Med. (2009) 1:142–51. doi: 10.1002/emmm.200900023
62. Singh A, Trivedi P, Jain NK. Advances in siRNA delivery in cancer therapy. Artif Cells Nanomed Biotechnol. (2018) 46:274–83. doi: 10.1080/21691401.2017.1307210
63. Xu C, Wang J. Delivery systems for siRNA drug development in cancer therapy. Asian J Pharm Sci. (2015) 10:1–12. doi: 10.1016/j.ajps.2014.08.011
64. Bertrand J-R, Pottier M, Vekris A, Opolon P, Maksimenko A, Malvy C. Comparison of antisense oligonucleotides and siRNAs in cell culture and in vivo. Biochem Biophys Res Commun. (2002) 296:1000–4. doi: 10.1016/S0006-291X(02)02013-2
65. Videira M, Arranja A, Rafael D, Gaspar R. Preclinical development of siRNA therapeutics: towards the match between fundamental science and engineered systems. Nanomed Nanotechnol Biol Med. (2014) 10:689–702. doi: 10.1016/j.nano.2013.11.018
66. Kim Y-D, Park T-E, Singh B, Maharjan S, Choi Y-J, Choung P-H, et al. Nanoparticle-mediated delivery of siRNA for effective lung cancer therapy. Nanomedicine. (2015) 10:1165–88. doi: 10.2217/nnm.14.214
67. Kotelianski V, Zatsepin T, Kotelevtsev Y. Lipid nanoparticles for targeted siRNA delivery—going from bench to bedside. Int J Nanomed. (2016) 11:3077–86. doi: 10.2147/IJN.S106625
68. Merkel OM, Kissel T. Nonviral pulmonary delivery of siRNA. Acc Chem Res. (2012) 45:961–70. doi: 10.1021/ar200110p
69. Shim G, Choi H, Lee S, Choi J, Yu YH, Park D-E, et al. Enhanced intrapulmonary delivery of anticancer siRNA for lung cancer therapy using cationic ethylphosphocholine-based nanolipoplexes. Mol Ther. (2013) 21:816–24. doi: 10.1038/mt.2013.10
70. Merkel OM, Rubinstein I, Kissel T. siRNA Delivery to the lung: what's new? Adv Drug Deliv Rev. (2014) 75:112–28. doi: 10.1016/j.addr.2014.05.018
71. Bahrami B, Hojjat-Farsangi M, Mohammadi H, Anvari E, Ghalamfarsa G, Yousefi M, et al. Nanoparticles and targeted drug delivery in cancer therapy. Immunol Lett. (2017) 190:64–83. doi: 10.1016/j.imlet.2017.07.015
72. Azarmi S, Tao X, Chen H, Wang Z, Finlay W, Lobenberg R, et al. Formulation and cytotoxicity of doxorubicin nanoparticles carried by dry powder aerosol particles. Int J Pharm. (2006) 319:155–61. doi: 10.1016/j.ijpharm.2006.03.052
73. Roa WH, Azarmi S, Al-Hallak MHDK, Finlay WH, Magliocco AM, Löbenberg R. Inhalable nanoparticles, a non-invasive approach to treat lung cancer in a mouse model. J Control Release. (2011) 150:49–55. doi: 10.1016/j.jconrel.2010.10.035
74. Videira MA. Nanopartículas Lipídicas Como Sistemas Transportadores de Fármacos Para Administração Pulmonar. Lisboa: Faculdade de Farmácia da Universidade de Lisboa (2008).
75. Tomoda K, Ohkoshi T, Hirota K, Sonavane GS, Nakajima T, Terada H, et al. Preparation and properties of inhalable nanocomposite particles for treatment of lung cancer. Colloids Surf B Biointerf. (2009) 71:177–82. doi: 10.1016/j.colsurfb.2009.02.001
76. Polach KJ, Matar M, Rice J, Slobodkin G, Sparks J, Congo R, et al. Delivery of siRNA to the mouse lung via a functionalized lipopolyamine. Mol Ther. (2012) 20:91–100. doi: 10.1038/mt.2011.210
77. Forbes B, O'Lone R, Allen PP, Cahn A, Clarke C, Collinge M, et al. Challenges for inhaled drug discovery and development: induced alveolar macrophage responses. Adv Drug Deliv Rev. (2014) 71:15–33. doi: 10.1016/j.addr.2014.02.001
78. Lewis DJ, Williams TC, Beck SL. Foamy macrophage responses in the rat lung following exposure to inhaled pharmaceuticals: a simple, pragmatic approach for inhaled drug development. J Appl Toxicol. (2014) 34:319–31. doi: 10.1002/jat.2950
79. McElroy MC, Kirton C, Gliddon D, Wolff RK. Inhaled biopharmaceutical drug development: nonclinical considerations and case studies. Inhal Toxicol. (2013) 25:219–32. doi: 10.3109/08958378.2013.769037
80. Owen K. Regulatory toxicology considerations for the development of inhaled pharmaceuticals. Drug Chem Toxicol. (2013) 36:109–18. doi: 10.3109/01480545.2011.648327
81. Roig JL, Gómez-Vallejo V, Gibson PN. Isotopes in Nanoparticles: Fundamentals and Applications. 1st ed. Boca Raton, FL: Pan Standford (2016).
82. Wolff RK. Toxicology studies for inhaled and nasal delivery. Mol Pharm. (2015) 12:2688–96. doi: 10.1021/acs.molpharmaceut.5b00146
83. Fröhlich E, Mercuri A, Wu S, Salar-Behzadi S. Measurements of deposition, lung surface area and lung fluid for simulation of inhaled compounds. Front Pharmacol. (2016) 7:181. doi: 10.3389/fphar.2016.00181
84. Report of the Questionnaire on Regulatory Regimes for Manufactured Nanomaterials 2010–2011. 42nd ed. Paris: OECD Environment, Health and Safety Publications (2014).
85. Shaw BW, Toby B, Zak C. TCEQ Guidelines to Develop Toxicity Factors. Austin, TX: Texas Commission on Environmental Quality (2015). Available online at: https://www.tceq.texas.gov/toxicology/esl/guidelines/about (accessed November 24, 2019).
Keywords: pulmonary administration, pre-clinical and clinical studies, inhaled nanomedicines, selective targeting, lung cancer, imaging, toxicity
Citation: Videira MA, Llop J, Sousa C, Kreutzer B, Cossío U, Forbes B, Vieira I, Gil N and Silva-Lima B (2020) Pulmonary Administration: Strengthening the Value of Therapeutic Proximity. Front. Med. 7:50. doi: 10.3389/fmed.2020.00050
Received: 04 February 2019; Accepted: 03 February 2020;
Published: 27 February 2020.
Edited by:
Hilde Stevens, Université Libre de Bruxelles, BelgiumReviewed by:
Rolf Bass, Retired, Berlin, GermanyCopyright © 2020 Videira, Llop, Sousa, Kreutzer, Cossío, Forbes, Vieira, Gil and Silva-Lima. This is an open-access article distributed under the terms of the Creative Commons Attribution License (CC BY). The use, distribution or reproduction in other forums is permitted, provided the original author(s) and the copyright owner(s) are credited and that the original publication in this journal is cited, in accordance with accepted academic practice. No use, distribution or reproduction is permitted which does not comply with these terms.
*Correspondence: Mafalda A. Videira, bWFmYWxkYXZpZGVpcmFAY2FtcHVzLnVsLnB0
†These authors have contributed equally to this work
Disclaimer: All claims expressed in this article are solely those of the authors and do not necessarily represent those of their affiliated organizations, or those of the publisher, the editors and the reviewers. Any product that may be evaluated in this article or claim that may be made by its manufacturer is not guaranteed or endorsed by the publisher.
Research integrity at Frontiers
Learn more about the work of our research integrity team to safeguard the quality of each article we publish.