- 1Department of Anesthesiology and Critical Care, Rouen University Hospital, Rouen, France
- 2Normandie Univ, UNIROUEN, INSERM U1096, FHU REMOD-VHF, Rouen, France
- 3Department of Medical Critical Care, Rouen University Hospital, Rouen, France
- 4Department of Biostatistics, Rouen University Hospital, Rouen, France
Introduction: Protein Tyrosine Phosphatase 1B (PTP1B) and endoplasmic reticulum stress (ERS) are involved in the septic inflammatory response. Their inhibition is associated with improved survival in murine models of sepsis. The objective was to describe PTP1B and ERS expression during septic shock in human.
Material and Methods: Prospective study including patients admitted to intensive care unit (ICU) for septic shock. Blood samples were collected on days 1 (D1), 3 and 5 (D5). Quantitative PCR (performed from whole blood) evaluated the expression of genes coding for PTP1B (PTPN1) and key elements of ERS (GRP78, ATF6, CHOP) or for endothelial dysfunction-related markers (ICAM1 and ET1). We analyzed gene variation between D5 and D1, collected glycemic parameters, insulin resistance and organ failure was evaluated by Sequential Organ Failure Assessment (SOFA) score.
Results: We included 44 patients with a mean SAPS II 50 ± 16 and a mortality rate of 13.6%. Between D1 and D5, there was a significant decrease of PTPN1 (p < 0.001) and ATF6 (p < 0.001) expressions. Their variations of expression were correlated with SOFA variation (PTPN1, r = 0.35, CI 95% [0.05; 0.54], p = 0.03 and ATF6, r = 0.45 CI 95% [0.20; 0.65], p < 0.001). We did not find any correlation between PTPN1 expression and insulin resistance or glycemic parameters. Between D1 and D5, ATF6 and PTPN1 expressions were correlated with that of ET1.
Conclusions: Our study has evaluated for the first time the expression of PTP1B and ERS in patients with septic shock, revealing that gene expression variation of PTPN1 and ATF6 are partly correlated with the evolution of septic organ failure and with endothelial dysfunction markers expression.
Introduction
Sepsis-caused multiple organ dysfunction syndrome (MODS) remains a major cause of morbidity and mortality in intensive care units (ICU) (1). Endothelial injury plays a major role in the pathogenesis of organ dysfunction during sepsis: endothelial dysfunction leads to the breakdown of the microvascular barrier resulting in increased extravascular fluid, tissue edema and organ failure (2, 3).
Protein Tyrosine Phosphatase 1B (PTP1B), a phosphatase localized on the cytoplasmic side of the endoplasmic reticulum (ER), is involved in the negative regulation of many cell pathways such as response to insulin or endothelial nitric oxide (NO) production (4). Our team and others recently showed that pharmacological or genetic inhibition of PTP1B restores vascular relaxation and endothelial NO synthase phosphorylation in septic models, protects against cardiac inflammation and dysfunction, and reduces mortality, suggesting its potential interest for the development of sepsis therapies that target endothelial injury (5, 6). The septic inflammatory process also causes serious disturbances in the insulin signaling pathway (7, 8). We previously demonstrated that PTP1B gene (Protein Tyrosine Phosphatase Non-Receptor Type 1 (PTPN1)) deletion significantly limits cecal ligation and puncture (CLP) -induced insulin resistance, improves insulin receptor signal transduction and reduces sepsis-induced endothelial dysfunction/impaired NO production (9). Multiple disturbances observed during sepsis can result in a dysfunction of the ER, leading to the accumulation of unfolded proteins within the lumen of the ER, known as ER stress (ERS) (10). Recent studies indicated that PTP1B and ERS are closely interlinked: ERS induces and activates PTP1B in skeletal muscle via the NF-κB pathway and PTP1B inhibition protects against ERS-induced cardiac dysfunction (11, 12). PTP1B plays a major role in the regulation of ERS in endothelial cell, and genetic or pharmacological inhibition of PTP1B improves endothelial dysfunction induced by ERS (13).
The defense against ERS mainly involves the unfolded protein response (UPR). UPR is initiated by chaperone [such as the 78 kDa Glucose Related Protein (GRP78)] binding to unfolded peptides, which relieves the basal inhibitory signal of signaling pathways of UPR including Protein Kinase RNA-like ER kinase [PERK, involving CCAAT/enhancer binding protein homologous protein (CHOP)] and Activating Transcription Factor 6 (ATF6) pathways (10, 14). Systemic inflammation is associated with the activation of ERS pathways which are strongly activated in murine models of sepsis (15–17). Treatment with 4-phenylbutyric acid (4BPA; an ERS inhibitor) decreased the tissue expression level of inflammatory cytokines, NF-κB activation and reduced organ dysfunction induced by bacterial lipopolysaccharide (LPS) (16). 4BPA also improved the mortality rate in a murine CLP model (18). In human, ERS is activated in the mononuclear cells of patients with acute lung injury and is involved in acute kidney injury (16, 19). Finally, it has been shown that ERS is associated with endothelial dysfunction and its inhibition improves endothelium-dependent relaxing function (13). Thus, modulation of ER stress via PTP1B inhibitors may be a promising approach to protect the endothelium in sepsis but to our knowledge no study has evaluated their expression in severe infections in humans.
The objectives of our study were therefore to explore the relationship between PTP1B and ERS gene expression and organ failure during septic shock in humans, and to describe their kinetics.
Materials and Methods
Patient Population
This prospective pilot study was carried out in the medical ICU of a tertiary care University Hospital. The study, conducted between December 2015 and April 2016 (N°2014-A00959-38), was approved by the ethics committee of Rouen University Hospital (n° CPP 02/017/2014) in accordance with the ethical standards of the Declaration of Helsinki and its later amendments. Written consent was provided prospectively by the authorized representatives and/or retrospectively by the patient.
Patients admitted to medical ICU with septic shock as defined by the 2013 surviving sepsis campaign guidelines were included (20). Exclusion criteria were age under 18 years or patient under tutorship, pregnancy/ breastfeeding, obesity defined by a body mass index ≥30 kg/m2, diabetes mellitus treated by oral and/or insulin therapy, and patient refusal. Each patient included in the protocol had a specific follow-up for the study during the first 5 days of their ICU stay (D1 to D5). The following parameters were evaluated: demographic characteristics [sex, age, Simplified Acute Physiology Score II (SAPS II)], insulin resistance measurement using the Homeostasis Model Assessment of Insulin Resistance score (HOMA-IR) on the first day (with insulin dosage at D1), daily maximal or minimal capillary glycemia, daily physiologic parameters, sepsis origin, daily Sequential Organ Failure Assessment (SOFA) score, daily diuresis, cumulative daily dose of norepinephrine and biological parameters (lactate, procalcitonin (PCT), glycemia) at the time of each sample.
Objectives
The main objective of this study was to search for a relationship between whole blood PTPN1 gene (coding for PTP1B) expression and SOFA score, by comparing the variation in PTPN1 gene expression and the variation in SOFA score calculated between D1 and D5 (presented as a delta-SOFA score: SOFA D5-SOFA D1).
Secondary objectives were to study:
- The link between daily PTPN1 expression and insulin resistance assessment (HOMA score) at D1, and the link between daily PTPN1 expression and daily cumulative dose of insulin administered, daily blood glucose variability (evaluated via blood glucose meter), and venous glycemia at the time of sampling at D1, D3, and D5;
- The correlation between the expression of genes involved in UPR (ATF6, CHOP, GRP78, and the variation in SOFA score;
- The kinetics of PTPN1 and UPR gene expression in patients between D1 and D5;
- The correlation between PTPN1 and UPR gene expression and clinical or biological markers of sepsis severity: survival, norepinephrine infusion doses, daily diuresis, and SAPS II at D1;
- The correlation between PTPN1 and UPR gene expression and the expression of the genes involved in endothelial dysfunction ICAM1 (coding for Inter-Cellular Adhesion Molecule 1) and ET1 (coding for Endothelin 1).
Sample Collection and Analysis
In addition to the usual daily morning blood sample, a 2.5 ml sample was drawn using PAXgene tube (Quiagen, Hilden, Germany) in the morning at 8:00 a.m. at D1, D3, and D5. Blood samples were kept for a maximum of 72 h in the refrigerators of the ICU at −20°C and then were stored at −80°C until analysis. PAXgene sampling was stopped after ICU discharge (if occurring before D5).
Ribonucleic Acid Extraction, Reverse Transcription, and Quantitative Polymerase Chain Reaction
Briefly, ribonucleic acid (RNA) extraction was performed using the commercial kit PAXgene® Blood RNA System kit (Quiagen, Hilden, Germany) according to the manufacturer's protocol. Before RNA elution, residual genomic deoxyribonucleic acid (DNA) was digested using RNase-Free DNase set (Quiagen, Hilden, Germany). The integrity and quantity of the total RNA were assessed with a Nanodrop 2000 device (Thermo Fisher Scientific, Waltham, MS, USA). Total RNAs were reverse transcribed into cDNA using M-MLV Reverse Transcriptase (Invitrogen, Carlsbad, CA, USA) according to manufacturer's instructions.
A quantitative polymerase chain reaction (qPCR) was performed for:
- The mRNA of genes coding for PTP1B (PTPN1 gene) and for proteins involved in UPR: ATF6, CHOP, GRP78;
- The mRNA of genes coding for proteins associated with septic endothelial dysfunction: ICAM1 and ET1;
- The mRNA of the gene coding for succinate dehydrogenase complex flavoprotein subunit A (SDHA). As SDHA has been described as a pertinent housekeeping gene in humans with sepsis and as its cycle threshold (Ct) is close to the Ct of PTP1B and UPR genes in qPCR, it appeared as the best housekeeping gene for our work (21).
The genes that were amplified and the primers that were used are listed in Table 1. PCR reactions were performed on a LightCycler 480 instrument using the Fast-StartDNA Master SYBR Green I real-time PCR kit according to the manufacturer's instructions (Roche Molecular Biochemicals, Bâle, Switzerland). Thermocycling was performed in a final volume of 10 μl (including 2.5 μl of cDNA sample at 1.67.10−3 μg/μl diluted at 1:10) containing 0.5 μl of each required primers at a concentration of 10 μM. PCR was performed with an initial denaturation step of 10 min at 95°C, followed by 40 cycles of a touch-down PCR protocol (40 cycles at 95°C for 10 s, 60°C for 10 s, 72°C for 12 s and 1 melting cycle). Ct values were used for quantifying target gene expression relative to the housekeeping gene using the 2−ΔCt method. Variation in gene expression was defined as the ratio of gene expression between D5 and D1 (D5/D1), e.g., resulting in a ratio of 2 if the expression doubled or a ratio of 0.5 if the expression halved at D5.
Statistical Analysis
Quantitative values are expressed as mean with standard deviation (for demographic data) and confidence interval at 95% (for SOFA score and gene expression). Qualitative values are presented with absolute values and percentages. Gene expression comparisons between D1 and D5 and correlations were carried out after logarithmic transformation and are therefore described in this manner in the figures. The initial (D1) and the final (D5) levels of log transformed gene expression were compared with a Paired Student test. Among patients with a significant change in gene expression between D1 and D5, correlations between variations in log transformed gene expression were computed with the Pearson method, 95% confidence intervals were calculated with the Bootstrap method. Missing data were managed for the correlation analysis, the last-observation-carried-forward method was used when needed (when D5 values were missing, D3 values replaced it). The significance level was 0.05 for all the tests performed. All calculations and statistical analyses were conducted on R 3.4.2 statistical software.
Results
Clinical and Demographic Characteristics of Population
Forty-four patients with septic shock were included in this study. Diagnoses at admission were: pneumonia (n = 26, 59%), urinary-tract infection (n = 10, 23%), intra-abdominal infection (n = 4, 9%), other (n = 4, 9%). Due to technical problems (RNA extraction difficulties) or lack of genetic material after extraction, some samples could not be analyzed. Four patients had no sample measurement on D1 and therefore could not be analyzed in regard to the main objective. In addition, some patients were discharged from ICU before D3 or before D5. Thus, of the 40 patients remaining on whom the analysis was conducted, 30 had all measurements at D1, D3, and D5, 4 had a measurement at D1 and D5 only and 6 had a measurement at D1 and D3 only. The study flow chart is presented in Figure 1. Between D1 and D5, there was a significant decrease in SOFA score (cf. Figure 2). The main demographic and clinical characteristics of patients are summarized in Table 2, the leucocyte cell count at each sampling time are summarized in Table 3.
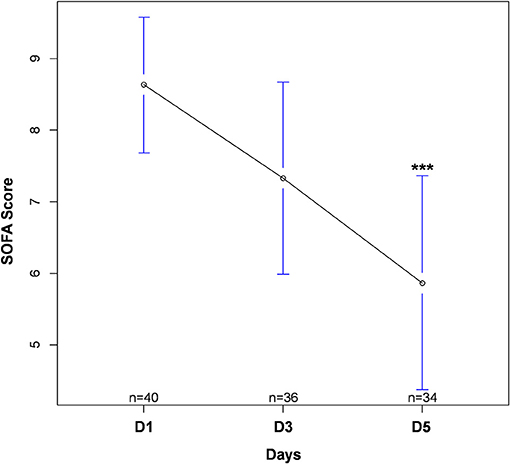
Figure 2. Evolution in SOFA score during intensive care unit stay. Values are expressed as mean with 95% confidence interval. Numbers (n) correspond to the number of patients still alive and in ICU at each time. ***p < 0.001 between D1 and D5. SOFA, Sequential Organ Failure Assessment.
PTP1B Gene Expression
There was a statistically significant decrease in PTPN1 expression between D1 and D5 (cf. Figure 3A). Concerning glycemic function, we did not find any statistically significant correlation between HOMA-IR and PTPN1 expression at D1, or any correlation between PTPN1 expression and daily dose of insulin, daily blood glucose variability and maximum daily blood glucose peak from D1 to D5 (cf. Table 4). No statistically significant correlation was observed between PTPN1 expression and diuresis or cumulative daily dose of norepinephrine. There was no significant difference in PTPN1 D1-D5 expression variations between deceased and surviving patients (cf. Table 5).
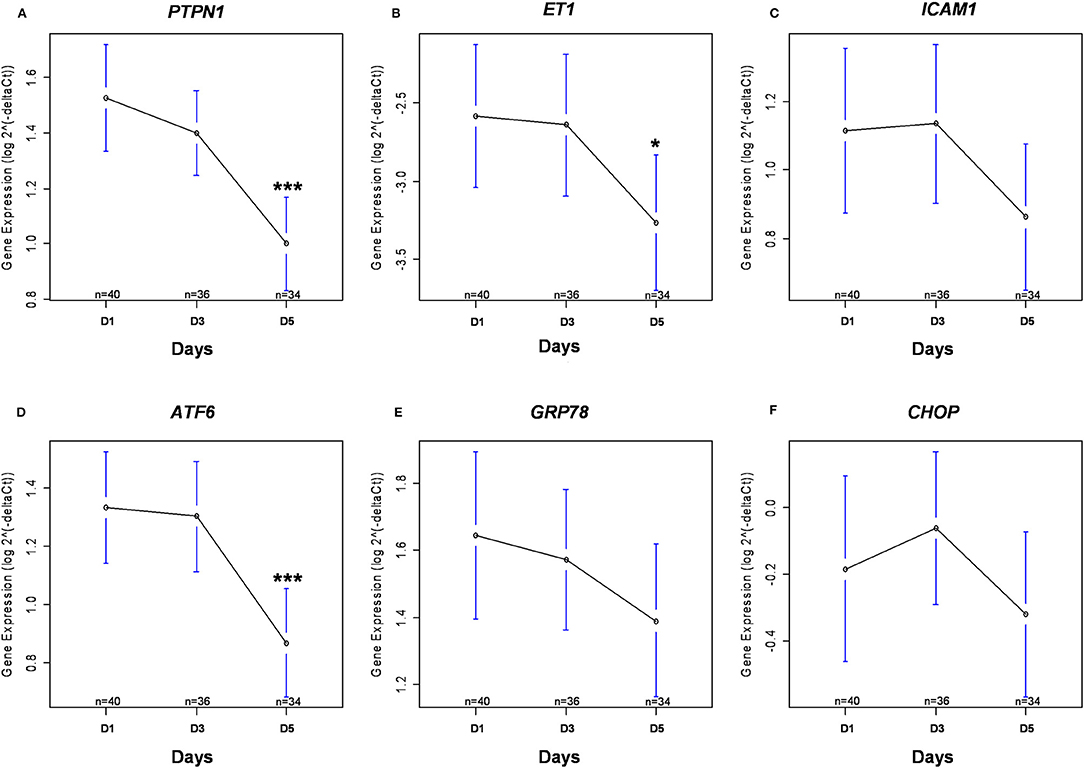
Figure 3. Kinetics of gene expression. Gene expression of PTPN1 (A), ET1 (B), ICAM1 (C), ATF6 (D), GRP78 (E), and CHOP (F) are presented after logarithmic transformation as mean with 95% confidence interval. The numbers (n) correspond to the number of patients still alive and in ICU at each time. *p < 0.05 between D1 and D5; ***p < 0.001 between D1 and D5. ATF6, Activating Transcription Factor 6; CHOP, DNA Damage Inducible Transcript 3; ET1, endothelin 1; GRP78, Heat Shock 70 kDa Protein 5; ICAM1, inter-cellular adhesion molecule 1; PTPN1, Protein Tyrosine Phosphatase Non-Receptor Type 1.
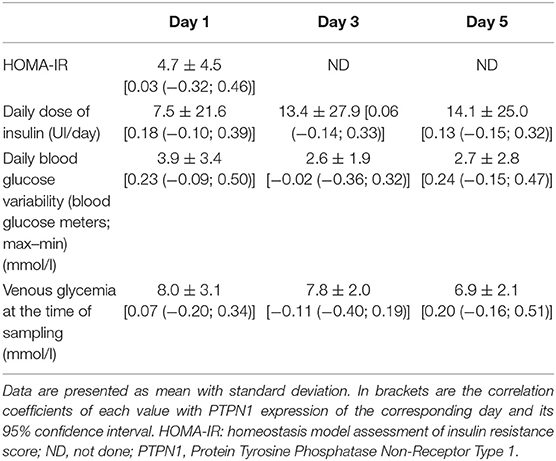
Table 4. Insulin resistance score, glycemic parameters, and their correlation with PTPN1 expression during ICU stay.
Endothelial Dysfunction Markers and UPR Gene Expression Kinetics
Between D1 and D5, there was a significant decrease in ET1 expression (cf. Figure 3B), and the expression of ICAM1 appeared to decrease gradually although no significant difference was found (cf. Figure 3C). Between D1 and D5, there was a significant decrease in ATF6 expression (cf. Figure 3D), GRP78 expression appeared to decrease gradually although no significant difference was found (cf. Figure 3E) and CHOP expression was stable over time (cf. Figure 3F).
No statistically significant correlation was observed between ATF6 expression and daily diuresis or cumulative daily dose of norepinephrine. There was no significant difference in ATF6 and ET1 D1-D5 expression variations between deceased and surviving patients (cf. Table 5).
Correlation Between PTPN1 and ATF6 Expressions and SOFA Score or Survival
Between D1 and D5, variations in PTPN1 expression and SOFA score were positively correlated (r = 0.35, CI 95% [0.05; 0.54]; p = 0.03; cf. Figure 4); PTPN1 expression increased by 7% (CI 95% [1%; 14%]) per point of SOFA. PTPN1 expression ratio was correlated with ET1 (r = 0.31 CI 95% [0.09; 0.52], p = 0.008; cf. Figure 5).
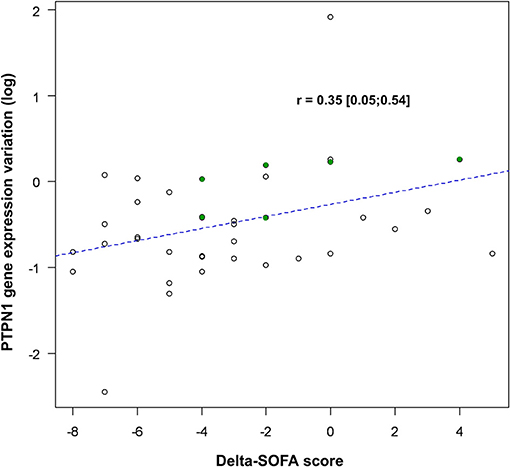
Figure 4. Correlation between variations in PTPN1 expression and SOFA score. PTPN1 expression variation is presented after logarithmic transformation [log(ratioD5/D1)] and variations in SOFA are presented as a delta-SOFA score (= SOFA D5–SOFA D1), showing a decrease of PTPN1 expression when the SOFA decrease between D1 and D5. The points highlighted in green correspond to the patients for whom the results of D3 have been taken into account. PTPN1, Protein Tyrosine Phosphatase Non-Receptor Type 1; SOFA, Sequential Organ Failure Assessment.
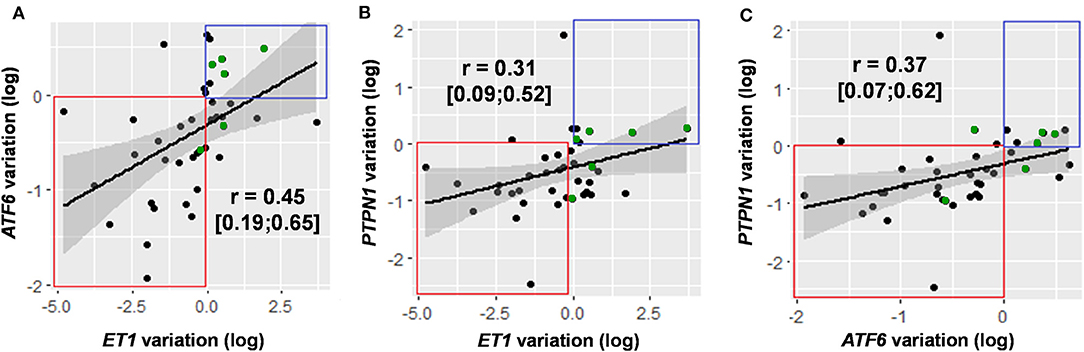
Figure 5. Correlation of variations in the expression of genes with significant variation in expression over time: ET1/ATF6 (A), ET1/PTPN1 (B), and ATF6/PTPN1 (C). Gene expression variations are presented after logarithmic transformation [log(ratioD5/D1)]. For each gene pair, data are presented in the form of a correlation coefficient with 95% confidence interval and in graphical form with regression line. Decreased expression of the two genes studied is framed in red, increased expression of the two genes studied is framed in blue. The points highlighted in green correspond to the patients for whom the results of D3 have been taken into account. ATF6, Activating Transcription Factor 6; ET1, endothelin 1; PTPN1, Protein Tyrosine Phosphatase Non-Receptor Type 1.
Between D1 and D5, variations in ATF6 expression and SOFA score were positively correlated (r = 0.45 CI 95% [0.20; 0.65], p < 0.001); ATF6 expression increased by 9% (CI 95% [3%; 15%]) per point of SOFA. Between D1 and D5, ATF6 expression was also positively correlated with ET1 (r = 0.45 CI 95% [0.19; 0.65], p < 0.001) and with PTPN1 (r = 0.37 CI 95% [0.07; 0.62], p = 0.02; cf. Figure 5).
Discussion
In regard to recent literature, our finding that PTPN1 and UPR genes are expressed in the plasma of patients with septic shock and that their expression shows significant variations suggests that these components may be involved in the pathophysiology of sepsis. To our knowledge, PTP1B and UPR have never been studied in patients with septic shock. We found that the variation in PTPN1 expression between D1 and D5 was significantly positively correlated with the variation in SOFA score (as was ATF6 expression). Additionally, PTPN1 and ATF6 expressions were correlated with ET1 which codes for a protein associated with septic endothelial dysfunction.
PTP1B Gene Expression
PTP1B is implicated in a wide range of cellular functions. PTP1B has a prominent role in the metabolism, through the regulation of insulin signaling, leading to the development of PTP1B inhibitors for the treatment of type 2 diabetes or obesity. However, the role of PTP1B in regulating immune signaling is also emerging in infectious diseases (22). Recently, it has been demonstrated that, in a P. aeruginosa infection model, PTP1B-deficient mice displayed enhanced bacterial clearance and reduced disease scores, suggesting that PTP1B might have a deleterious role in the host defense against bacterial infections (23). Moreover, it has been shown that, in a rat model of sepsis, PTP1B induced brain mitochondrial dysfunction associated with overproduction of reactive oxygen species (24). In animal models, genetic or pharmacological inhibition of PTP1B enhanced endothelial cell proliferation and NO production, and could also reverse septic endothelial dysfunction (5, 6, 9, 25). We have shown a correlation between variations in SOFA score and PTPN1 expression. However, the design of our work does not allow us to establish a causal link between organ failure assessed by SOFA score and PTPN1 expression. We did not find any link between markers of initial severity (SAPS II) and PTPN1 expression at D1. It is possible that, in view of the delay between gene expression and effective protein synthesis, organ failure at one time may be related to the expression of PTPN1 in the previous hours, which would explain the correlation found for the global variation in organ failure but not for failure at a given time.
Inflammation can directly contribute to insulin resistance by disrupting the insulin signaling pathway, in part via PTP1B activation. In a previous experimental study, we demonstrated that PTP1B gene deletion significantly limited CLP-induced insulin resistance, improved AMP-activated protein kinase signaling pathway and Glucose Transporter 4 translocation, and decreased inflammation (9). In the present work, we did not find any link between PTPN1 levels and HOMA-IR index, glycemia, glycemia variation or insulin consumption. A previous work described a strong correlation between insulin resistance evaluated through HOMA-IR index and plasma PTP1B level in a population of patients with polycystic ovarian syndrome (26). Moreover, PTP1B inhibition significantly decreased HOMA-IR index in obese and diabetic mice suggesting a direct link between PTP1B level and HOMA-IR (27). There are two reports on the use of HOMA-IR index in ICU patients but both studies have limitations with great variance and the use of HOMA-IR is controversial in ICU (28, 29). In our work, the conditions for measuring insulin levels using HOMA-IR were not standardized for insulin treatment at the time of sampling. In regard to this strong bias, to the results of previous study on the link between PTP1B and insulin resistance, and to controversies on the efficiency of HOMA-IR to assess insulin resistance in ICU patients, we should be cautious and not conclude the absence of a link between PTP1B levels and insulin resistance in septic patients.
UPR Gene Expression
We have shown a correlation between variations in SOFA score and ATF6 expression. ATF6 upregulates many protective genes and downregulates many potentially damaging genes (30, 31). Previous studies have shown that ATF6 activation in cardiac myocytes protects the heart from ischemic damage, while inhibiting ATF6 has the opposite effect (32). Since ATF6 is activated by ischemia but inactivated upon reperfusion, we could consider that organ ischemia related to the disequilibrium of the oxygen consumption/supply balance during sepsis is associated with the expression of ATF6 (32). The improvement in organ dysfunction could be associated with a decrease in ATF6 linked to organ reperfusion. We did not show any significant variation in the other two UPR genes studied. GRP78 seemed to decrease during the first 5 days and it is possible that a lack of power prevented us from showing a significant decrease. It is also possible that, among UPR pathways, ATF6 is the most intensely involved pathway during sepsis but additional experiments should be carried out to study this hypothesis before drawing any conclusion. Previous studies have shown a beneficial effect of ERS inhibition on survival and MODS, which suggest the involvement of ERS in critical illness-induced MODS (16, 18, 33). Finally, expression of ATF6, PTPN1, and ET1 tended to decrease more in surviving patients than in deceased patients (although this variation did not appear significant). However, this work was not designed to reveal a difference in mortality according to gene expression variations, but future work should analyze this point.
PTP1B/UPR Gene Expression and Endothelial Dysfunction
Endothelial dysfunction is considered as a precursor of tissue damage and end-organ dysfunction (34, 35). It has been shown that ET1 levels are significantly higher in non-survivors than in survivors and are correlated with organ dysfunction and mortality (2, 36, 37). We found that a variation in PTPN1 expression was correlated with a variation in ET1 expression. PTP1B is involved in endothelial dysfunction and it is known that PTP1B inhibition reduces sepsis-induced endothelial dysfunction and impaired NO production (9). There is no work studying the link between ET1 and PTP1B but, more than a direct link between these two markers, since ET1 is a marker of septic endothelial lesion, it is likely that the observed correlation could reflect the relationship between PTPN1 expression and septic endothelial dysfunction. As it has been demonstrated that LPS-induced ET1 expression occurs via an NF-κB-dependent pathway and, as ERS induces NF-κB, we can hypothesize that the correlation between ATF6 and ET1 expression involves, at least in part, the NF-κB pathway (16, 38). ERS induces endothelial dysfunction in vivo and we found that the variation in ATF6 expression was correlated with the variation in endothelial dysfunction marker. We can thus hypothesize that ERS may be one of the numerous triggering factors of endothelial dysfunction during sepsis in human (13, 39). Finally, we found no significant difference for ATF6, ET1, and PTPN1 variations between surviving and deceased groups. However, given the very small size of our cohort with only 6 deaths, it seems difficult to definitely conclude that there is no association between mortality and expression of these genes.
Limitations
Our work has several major limitations. It is a pilot physiologic study with a limited number of patients without a control group. We included patients with several infectious sources while related animal studies were conducted in standardized models. It is thus possible that PTPN1 and UPR genes have different expressions and kinetics depending on the kind of infection but, given the limited number of patients, it did not appear reasonable to compare patients according to their infectious source. We used the criteria of the 2013 surviving sepsis campaign to include patients. This work was designed before the change in sepsis diagnostic criteria, which explains why SEPSIS-3 criteria were not used here. Our mortality rate was quite low for a cohort of septic shock patients but it remained within the low mortality rates described in some recent works (15–20%) (40, 41). In addition, we have included many urinary sepsis whose prognosis is much better than sepsis from other sources (42). These combined factors probably explain the low mortality rate observed.
Our work focused on gene expression in the whole blood but we did not perform a direct protein assay. It is known that, in human muscle, PTPN1 expression is related to PTP1B protein level and that the increase in ATF6 mRNA in peripheral blood mononuclear cells is correlated with ATF6 protein synthesis (43, 44). These data suggest that, for PTP1B and ATF6, gene expression is a good indicator of protein synthesis in humans. This work was conducted in ICU patients with a limited access to tissue, and with the logistical necessity of taking samples during several days (including weekends and/or on-call periods). As most of the proteins studied in our work cannot be measured in blood without complex cell isolation techniques, RNA quantification appeared to be the best compromise. However, it seems important to consider a future work measuring ERS proteins and ERS gene expression at the same time points in septic patients to better specify their correlation in this specific population. As the RNA expression on whole blood measured using the Paxgen tubes is strongly correlated with the RNA expression in circulating leucocytes, we can assume that we detected variations in leucocyte gene expression (45). As it is known that UPR play a crucial role in immune cells, including differentiation, immune activation, antibody production and cytokine expression, it seemed relevant to study the leucocyte expression of UPR genes (46, 47). However, we may have missed a potentially greater variation in gene expression in tissues and organs, as observed in animal models (16, 18, 33). It is also important to note that several studies have focused on the interest of a genomic approach to classify sepsis according to its severity or prognosis (48–50). These genotypic studies have so far failed to adequately discriminate sepsis from non-septic inflammatory syndrome and have shown no link between organ failure or clinical evolution and genomic expression profile. Recent data suggest that, at the level of leucocyte transcriptome, alterations in the expression of classical inflammatory and anti-inflammatory as well as adaptive immunity genes occur simultaneously, not sequentially after severe injury (49). Thus, variations of many genes during septic shock shifts in a same manner over time (up- or down-regulation), and it appears difficult to analyze the impact of a specific gene expression variation independently of others. The genomic study during sepsis therefore remains an analysis with clear limitations, limiting the final interpretation of our results. Given the existence of several open genomic datasets performed in whole blood of septic patients which come from genome wide association studies, it also appears interesting to consider a future work on these database to analyze all the genes involved in endothelial dysfunction, PTP1B regulation and ERS (48).
Conclusion
We have described for the first time PTP1B and ERS expression in patients with septic shock. We have shown that PTP1B and ATF6 might be correlated with organ failure (SOFA score) and endothelial dysfunction (ET1 expression). The recent identification of PTP1B as a novel negative regulator of host defense against sepsis may have potential therapeutic implications. However, further studies are needed to better understand PTP1B and ERS implication during sepsis in humans.
Data Availability Statement
The raw data supporting the conclusions of this manuscript will be made available by the authors, without undue reservation, to any qualified researcher.
Ethics Statement
The studies involving human participants were reviewed and approved by ethics committee of Rouen University Hospital (n° CPP 02/017/2014). The patients/participants provided their written informed consent to participate in this study.
Author Contributions
TC was involved in acquisition of data, in analysis and interpretation of data, and in manuscript draft. SG was involved in acquisition of data, in study design, and in interpretation of data. TP-L was involved in statistical analysis, in interpretation of data, and in manuscript draft. EB was involved in the study conception and design, in acquisition of data, and in manuscript revision. SR was involved in acquisition of data and in analysis and interpretation of data. SF and P-AT were involved in analysis and interpretation of data and in manuscript revision. VR, BV, and FT were involved in the study conception and design, in analysis and interpretation of data, and in manuscript revision. All authors contributed to manuscript revision, read, and approved the submitted version.
Funding
This work was supported by Normandie Rouen University, Inserm, and Rouen University Hospital. The funding sources had no role in the design and conduct of the study; collection, management, analysis, or interpretation of the data; preparation, review, or approval of the manuscript; and decision to submit the manuscript for publication.
Conflict of Interest
The authors declare that the research was conducted in the absence of any commercial or financial relationships that could be construed as a potential conflict of interest.
Acknowledgments
The authors are grateful to Nikki Sabourin-Gibbs, Rouen University Hospital, for her help in editing the manuscript and for English proofreading. We thank Marion Beuzelin, Dorothée Carpentier, Gaëtan Beduneau, and Christophe Girault for their help in including patients.
Abbreviations
4BPA, 4-phenylbutyric acid; ATF6, Activating Transcription Factor 6; CHOP, CCAAT/enhancer binding protein homologous protein; CLP, Cecal ligation and puncture; Ct, Cycle threshold; CHOP, DNA Damage Inducible Transcript 3 (gene coding for CHOP); DNA, Deoxyribonucleic acid; ET1, Endothelin 1; ER, Endoplasmic reticulum; ERS, Endoplasmic reticulum stress; GRP78, 78 kDa Glucose Related Protein; HOMA-IR, Homeostasis Model Assessment of Insulin Resistance; GRP78, Heat Shock 70 kDa Protein 5; ICAM1, Inter-cellular adhesion molecule 1; ICU, Intensive care units; IRE1, Inositol-Requiring Enzyme 1 alpha; LPS, Lipopolysaccharide; MODS, Multiple organ failure; NF-κB, Nuclear Factor-Kappa B; NO, nitric oxide; PCR, Polymerase chain reaction; PCT, Procalcitonin; PERK, Protein Kinase RNA-like ER kinase; PTP1B, Protein Tyrosine Phosphatase 1B; PTPN1, Protein Tyrosine Phosphatase Non-Receptor Type 1 (gene coding for PTP1B); RNA, Ribonucleic acid; SAPS II, Simplified Acute Physiology Score II; SDHA, Succinate dehydrogenase complex flavoprotein subunit A; SOFA, Sequential Organ Failure Assessment; UPR, Unfolded protein response.
References
1. Angus DC, van der Poll T. Severe sepsis and septic shock. N Engl J Med. (2013) 369:840–51. doi: 10.1056/NEJMra1208623
2. Hein OV, Misterek K, Tessmann J-P, van Dossow V, Krimphove M, Spies C. Time course of endothelial damage in septic shock: prediction of outcome. Crit Care Lond Engl. (2005) 9:R323–30. doi: 10.1186/cc3532
3. Nedeva C, Menassa J, Puthalakath H. Sepsis: inflammation is a necessary evil. Front Cell Dev Biol. (2019) 7:108. doi: 10.3389/fcell.2019.00108
4. Thiebaut P-A, Besnier M, Gomez E, Richard V. Role of protein tyrosine phosphatase 1B in cardiovascular diseases. J Mol Cell Cardiol. (2016) 101:50–7. doi: 10.1016/j.yjmcc.2016.09.002
5. Zabolotny JM, Kim Y-B, Welsh LA, Kershaw EE, Neel BG, Kahn BB. Protein-tyrosine phosphatase 1B expression is induced by inflammation in vivo. J Biol Chem. (2008) 283:14230–41. doi: 10.1074/jbc.M800061200
6. Coquerel D, Neviere R, Delile E, Mulder P, Marechal X, Montaigne D, et al. Gene deletion of protein tyrosine phosphatase 1B protects against sepsis-induced cardiovascular dysfunction and mortality. Arterioscler Thromb Vasc Biol. (2014) 34:1032–44. doi: 10.1161/ATVBAHA.114.303450
7. Hagiwara S, Iwasaka H, Hasegawa A, Asai N, Noguchi T. Hyperglycemia contributes to cardiac dysfunction in a lipopolysaccharide-induced systemic inflammation model. Crit Care Med. (2009) 37:2223–7. doi: 10.1097/CCM.0b013e3181a007c6
8. Bar-Or D, Rael LT, Madayag RM, Banton KL, Tanner AI, Acuna DL, et al. Stress Hyperglycemia in critically ill patients: insight into possible molecular pathways. Front Med. (2019) 6:54. doi: 10.3389/fmed.2019.00054
9. Delile E, Nevière R, Thiébaut P-A, Maupoint J, Mulder P, Coquerel D, et al. Reduced insulin resistance contributes to the beneficial effect of protein tyrosine phosphatase-1B deletion in a mouse model of sepsis. Shock. (2017) 48:355–63. doi: 10.1097/SHK.0000000000000853
10. Khan MM, Yang W-L, Wang P. Endoplasmic reticulum stress in sepsis. Shock. (2015) 44:294–304. doi: 10.1097/SHK.0000000000000425
11. Panzhinskiy E, Hua Y, Culver B, Ren J, Nair S. Endoplasmic reticulum stress upregulates protein tyrosine phosphatase 1B and impairs glucose uptake in cultured myotubes. Diabetologia. (2013) 56:598–607. doi: 10.1007/s00125-012-2782-z
12. Wang S, Chen X, Nair S, Sun D, Wang X, Ren J. Deletion of protein tyrosine phosphatase 1B obliterates endoplasmic reticulum stress-induced myocardial dysfunction through regulation of autophagy. Biochim Biophys Acta Mol Basis Dis. (2017) 1863:3060–74. doi: 10.1016/j.bbadis.2017.09.015
13. Thiebaut P-A, Delile E, Coquerel D, Brunel J-M, Renet S, Tamion F, et al. Protein tyrosine phosphatase 1B regulates endothelial endoplasmic reticulum stress; role in endothelial dysfunction. Vascul Pharmacol. (2018) 109:36–44. doi: 10.1016/j.vph.2018.05.011
14. Hu H, Tian M, Ding C, Yu S. The C/EBP Homologous Protein (CHOP) transcription factor functions in endoplasmic reticulum stress-induced apoptosis and microbial infection. Front Immunol. (2019) 9:83. doi: 10.3389/fimmu.2018.03083
15. Ma T, Han L, Gao Y, Li L, Shang X, Hu W, et al. The endoplasmic reticulum stress-mediated apoptosis signal pathway is involved in sepsis-induced abnormal lymphocyte apoptosis. Eur Surg Res Eur Chir Forsch Rech Chir Eur. (2008) 41:219–25. doi: 10.1159/000135631
16. Kim HJ, Jeong JS, Kim SR, Park SY, Chae HJ, Lee YC. Inhibition of endoplasmic reticulum stress alleviates lipopolysaccharide-induced lung inflammation through modulation of NF-κB/HIF-1α signaling pathway. Sci Rep. (2013) 3:1142. doi: 10.1038/srep01142
17. Grootjans J, Kaser A, Kaufman RJ, Blumberg RS. The unfolded protein response in immunity and inflammation. Nat Rev Immunol. (2016) 16:469–84. doi: 10.1038/nri.2016.62
18. Liu L, Wu H, Zang J, Yang G, Zhu Y, Wu Y, et al. 4-phenylbutyric acid reveals good beneficial effects on vital organ function via anti-endoplasmic reticulum stress in septic rats. Crit Care Med. (2016) 44:e689–701. doi: 10.1097/CCM.0000000000001662
19. Yan M, Shu S, Guo C, Tang C, Dong Z. Endoplasmic reticulum stress in ischemic and nephrotoxic acute kidney injury. Ann Med. (2018) 50:381–90. doi: 10.1080/07853890.2018.1489142
20. Dellinger RP, Levy MM, Rhodes A, Annane D, Gerlach H, Opal SM, et al. Surviving Sepsis Campaign: international guidelines for management of severe sepsis and septic shock, 2012. Intensive Care Med. (2013) 39:165–228. doi: 10.1007/s00134-012-2769-8
21. Cummings M, Sarveswaran J, Homer-Vanniasinkam S, Burke D, Orsi NM. Glyceraldehyde-3-phosphate dehydrogenase is an inappropriate housekeeping gene for normalising gene expression in sepsis. Inflammation. (2014) 37:1889–94. doi: 10.1007/s10753-014-9920-3
22. Feldhammer M, Uetani N, Miranda-Saavedra D, Tremblay ML. PTP1B: a simple enzyme for a complex world. Crit Rev Biochem Mol Biol. (2013) 48:430–45. doi: 10.3109/10409238.2013.819830
23. Yue L, Xie Z, Li H, Pang Z, Junkins RD, Tremblay ML, et al. Protein tyrosine phosphatase-1B negatively impacts host defense against Pseudomonas aeruginosa infection. Am J Pathol. (2016) 186:1234–44. doi: 10.1016/j.ajpath.2016.01.005
24. Lyu J, Zheng G, Chen Z, Wang B, Tao S, Xiang D, et al. Sepsis-induced brain mitochondrial dysfunction is associated with altered mitochondrial Src and PTP1B levels. Brain Res. (2015) 1620:130–8. doi: 10.1016/j.brainres.2015.04.062
25. Wang Y, Yan F, Zhang W, Pang S, Jiang F. Inhibiting protein tyrosine phosphatase 1B to improve regenerative functions of endothelial cells. J Cardiovasc Pharmacol. (2018) 71:59–64. doi: 10.1097/FJC.0000000000000530
26. Shao X, Li J, Wang Y, Liang Q, Song S. Expression and its correlation with metabolic syndrome of protein tyrosine phosphatase 1B in serum of polycystic ovary syndrome patients. Biomed Res. (2017) 28:6. Available online at: https://www.semanticscholar.org/paper/Expression-and-its-correlation-with-metabolic-of-1B-Shao-Li/39ad25bb06652bb4420340bd5ea0d694f401739e
27. Zhang X, Tian J, Li J, Huang L, Wu S, Liang W, et al. A novel protein tyrosine phosphatase 1B inhibitor with therapeutic potential for insulin resistance. Br J Pharmacol. (2016) 173:1939–49. doi: 10.1111/bph.13483
28. Koch A, Gressner OA, Sanson E, Tacke F, Trautwein C. Serum resistin levels in critically ill patients are associated with inflammation, organ dysfunction and metabolism and may predict survival of non-septic patients. Crit Care Lond Engl. (2009) 13:R95. doi: 10.1186/cc7925
29. von Loeffelholz C, Thomas-Rüddel D, Bauer M, Birkenfeld A. Effect of magnesium loading dose on insulin resistance in patients with stress-induced hyperglycemia: a randomized clinical trial. J Intensive Care Med. (2018). doi: 10.1177/0885066618803866. [Epub ahead of print].
30. Egawa N, Yamamoto K, Inoue H, Hikawa R, Nishi K, Mori K, et al. The endoplasmic reticulum stress sensor, ATF6α, protects against neurotoxin-induced dopaminergic neuronal death. J Biol Chem. (2011) 286:7947–57. doi: 10.1074/jbc.M110.156430
31. Wu J, Rutkowski DT, Dubois M, Swathirajan J, Saunders T, Wang J, et al. ATF6alpha optimizes long-term endoplasmic reticulum function to protect cells from chronic stress. Dev Cell. (2007) 13:351–64. doi: 10.1016/j.devcel.2007.07.005
32. Doroudgar S, Thuerauf DJ, Marcinko MC, Belmont PJ, Glembotski CC. Ischemia activates the ATF6 branch of the endoplasmic reticulum stress response. J Biol Chem. (2009) 284:29735–45. doi: 10.1074/jbc.M109.018036
33. Diao L, Marshall AH, Dai X, Bogdanovic E, Abdullahi A, Amini-Nik S, et al. Burn plus lipopolysaccharide augments endoplasmic reticulum stress and NLRP3 inflammasome activation and reduces PGC-1α in liver. Shock. (2014) 41:138–44. doi: 10.1097/SHK.0000000000000075
34. Shapiro NI, Schuetz P, Yano K, Sorasaki M, Parikh SM, Jones AE, et al. The association of endothelial cell signaling, severity of illness, and organ dysfunction in sepsis. Crit Care Lond Engl. (2010) 14:R182. doi: 10.1186/cc9290
35. Skibsted S, Jones AE, Puskarich MA, Arnold R, Sherwin R, Trzeciak S, et al. Biomarkers of endothelial cell activation in early sepsis. Shock. (2013) 39:427–32. doi: 10.1097/SHK.0b013e3182903f0d
36. Lundberg OHM, Bergenzaun L, Rydén J, Rosenqvist M, Melander O, Chew MS. Adrenomedullin and endothelin-1 are associated with myocardial injury and death in septic shock patients. Crit Care Lond Engl. (2016) 20:178. doi: 10.1186/s13054-016-1361-y
37. Rivers EP, Kruse JA, Jacobsen G, Shah K, Loomba M, Otero R, et al. The influence of early hemodynamic optimization on biomarker patterns of severe sepsis and septic shock. Crit Care Med. (2007) 35:2016–24. doi: 10.1097/01.CCM.0000281637.08984.6E
38. McKenna S, Gossling M, Bugarini A, Hill E, Anderson AL, Rancourt RC, et al. Endotoxemia induces IκBβ/NF-κB-dependent endothelin-1 expression in hepatic macrophages. J Immunol Baltim Md 1950. (2015) 195:3866–79. doi: 10.4049/jimmunol.1501017
39. Luo Y, Li S-J, Yang J, Qiu Y-Z, Chen F-P. HMGB1 induces an inflammatory response in endothelial cells via the RAGE-dependent endoplasmic reticulum stress pathway. Biochem Biophys Res Commun. (2013) 438:732–8. doi: 10.1016/j.bbrc.2013.07.098
40. Vincent J-L, Jones G, David S, Olariu E, Cadwell KK. Frequency and mortality of septic shock in Europe and North America: a systematic review and meta-analysis. Crit Care Lond Engl. (2019) 23:196. doi: 10.1186/s13054-019-2478-6
41. Stevenson EK, Rubenstein AR, Radin GT, Wiener RS, Walkey AJ. Two decades of mortality trends among patients with severe sepsis: a comparative meta-analysis*. Crit Care Med. (2014) 42:625–31. doi: 10.1097/CCM.0000000000000026
42. Qiang X-H, Yu T-O, Li Y-N, Zhou L-X. Prognosis risk of urosepsis in critical care medicine: a prospective observational study. BioMed Res Int. (2016) 2016:9028924. doi: 10.1155/2016/9028924
43. Tampakakis E, Tabit CE, Holbrook M, Linder EA, Berk BD, Frame AA, et al. Intravenous lipid infusion induces endoplasmic reticulum stress in endothelial cells and blood mononuclear cells of healthy adults. J Am Heart Assoc. (2016) 5:e002574. doi: 10.1161/JAHA.115.002574
44. Stull AJ, Wang ZQ, Zhang XH, Yu Y, Johnson WD, Cefalu WT. Skeletal muscle protein tyrosine phosphatase 1B regulates insulin sensitivity in African Americans. Diabetes. (2012) 61:1415–22. doi: 10.2337/db11-0744
45. Feezor RJ, Baker HV, Mindrinos M, Hayden D, Tannahill CL, Brownstein BH, et al. Whole blood and leucocyte RNA isolation for gene expression analyses. Physiol Genomics. (2004) 19:247–54. doi: 10.1152/physiolgenomics.00020.2004
46. So JS. Roles of endoplasmic reticulum stress in immune responses. Mol Cells. (2018) 41:705–16. doi: 10.14348/molcells.2018.0241
47. Ma Y, Shimizu Y, Mann MJ, Jin Y, Hendershot LM. Plasma cell differentiation initiates a limited ER stress response by specifically suppressing the PERK-dependent branch of the unfolded protein response. Cell Stress Chaperones. (2010) 15:281–93. doi: 10.1007/s12192-009-0142-9
48. Sweeney TE, Khatri P. Benchmarking sepsis gene expression diagnostics using public data*. Crit Care Med. (2017) 45:1–10. doi: 10.1097/CCM.0000000000002021
49. Xiao W, Mindrinos MN, Seok J, Cuschieri J, Cuenca AG, Gao H, et al. A genomic storm in critically injured humans. J Exp Med. (2011) 208:2581–90. doi: 10.1084/jem.20111354
Keywords: protein tyrosine phosphatase, non-receptor type 1/metabolism, endoplasmic reticulum stress, sepsis, shock, endothelium, inflammation
Citation: Clavier T, Grangé S, Pressat-Laffouilhere T, Besnier E, Renet S, Fraineau S, Thiebaut P-A, Richard V, Veber B and Tamion F (2019) Gene Expression of Protein Tyrosine Phosphatase 1B and Endoplasmic Reticulum Stress During Septic Shock. Front. Med. 6:240. doi: 10.3389/fmed.2019.00240
Received: 08 July 2019; Accepted: 14 October 2019;
Published: 01 November 2019.
Edited by:
Andrey V. Kozlov, Institute for Experimental and Clinical Traumatology (LBG), AustriaReviewed by:
Johanna Catharina Duvigneau, Veterinärmedizinische Universität Wien, AustriaLongxiang Su, Peking Union Medical College Hospital (CAMS), China
Eizo Watanabe, Chiba University Graduate School of Medicine, Japan
Copyright © 2019 Clavier, Grangé, Pressat-Laffouilhere, Besnier, Renet, Fraineau, Thiebaut, Richard, Veber and Tamion. This is an open-access article distributed under the terms of the Creative Commons Attribution License (CC BY). The use, distribution or reproduction in other forums is permitted, provided the original author(s) and the copyright owner(s) are credited and that the original publication in this journal is cited, in accordance with accepted academic practice. No use, distribution or reproduction is permitted which does not comply with these terms.
*Correspondence: Thomas Clavier, dGhvbWFzY2xhdmllcjc2QGdtYWlsLmNvbQ==
†ORCID: Thomas Clavier orcid.org/0000-0001-8630-7537