- Division of Immunology, Alexander Fleming Biomedical Sciences Research Center, Athens, Greece
Lysophospholipid signaling is emerging as a druggable regulator of pathophysiological responses, and especially fibrosis, exemplified by the relative ongoing clinical trials in idiopathic pulmonary fibrosis (IPF) patients. In this review, we focus on ectonucleotide pyrophosphatase-phosphodiesterase 2 (ENPP2), or as more widely known Autotaxin (ATX), a secreted lysophospholipase D (lysoPLD) largely responsible for extracellular lysophosphatidic acid (LPA) production. In turn, LPA is a bioactive phospholipid autacoid, forming locally upon increased ATX levels and acting also locally through its receptors, likely guided by ATX's structural conformation and cell surface associations. Increased ATX activity levels have been detected in many inflammatory and fibroproliferative conditions, while genetic and pharmacologic studies have confirmed a pleiotropic participation of ATX/LPA in different processes and disorders. In pulmonary fibrosis, ATX levels rise in the broncheoalveolar fluid (BALF) and stimulate LPA production. LPA engagement of its receptors activate multiple G-protein mediated signal transduction pathways leading to different responses from pulmonary cells including the production of pro-inflammatory signals from stressed epithelial cells, the modulation of endothelial physiology, the activation of TGF signaling and the stimulation of fibroblast accumulation. Genetic or pharmacologic targeting of the ATX/LPA axis attenuated disease development in animal models, thus providing the proof of principle for therapeutic interventions.
Introduction
ATX was first identified as an autocrine motility-stimulating factor, isolated from the supernatant of highly metastatic melanoma cells (1). Its cDNA cloning revealed that ATX was homologous to ectonucleotide pyrophosphatase-phosphodiesterase 1 (ENPP1), possessing phosphodiesterase activity in vitro (2); ATX was thus classified as ENPP2 in the ENPP (1–7) protein family, being the only secreted and not transmembrane member (3). In addition, several years later it was discovered that ATX is identical to the long elusive plasma lysoPLD (4, 5), and is now considered responsible for the synthesis of the majority of extracellular LPA (Figure 1).
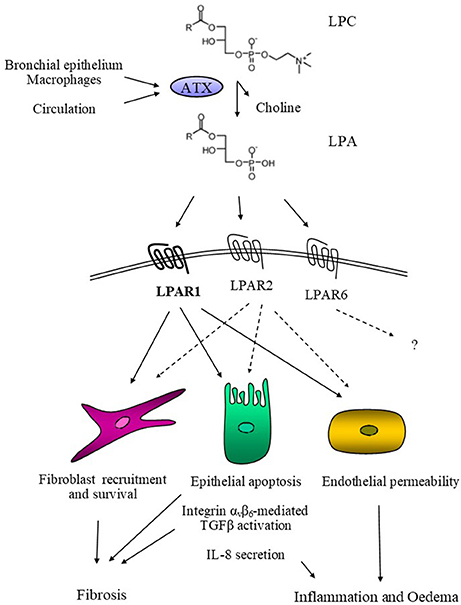
Figure 1. Schematic representation of ATX's mode of action in pulmonary fibrosis. ATX, derived from the bronchial epithelium and alveolar macrophages or extravasated from the circulation, catalyses the hydrolysis of LPC and the local production of LPA. In turn, LPA activates its cognate receptors LPAR1, possibly LPAR2, and hypothetically LPAR6, activating the corresponding G-protein-mediated signal transduction cascades. As a result, LPA induces epithelial apoptosis, the initiating pathogenetic event in modeled pulmonary fibrosis and possibly IPF. LPA also induces IL-8 secretion from epithelial cells, promoting inflammation, while it also stimulates endothelial permeability, thus promoting pulmonary oedema. Moreover, LPA stimulate the αvβ6-mediated TGFβ activation leading to the activation and trans-differentiation of pulmonary fibroblasts, for which LPA is additionally a pro-survival and chemotactic factor.
The ENPP2/Enpp2 Gene; Expression and Regulation
ENPP2 consists of 27 exons and resides in the human chromosomal region 8q24 (6, 7), a region with frequent somatic copy number alterations in cancer patients, containing potential susceptibility loci for various types of cancers (8, 9). The 8q24 locus has been suggested to regulate the expression of the proto-oncogene MYC, also residing in the region (10). In silico analysis of publicly available genomic data at The Cancer Genome Atlas (11) indicated genetic alterations, mostly amplifications, of ENPP2 in cancer patients, with the highest rates observed in ovarian (33%), breast (20%), liver (20%), and lung (11%) carcinomas (12). Moreover, a number of single nucleotide polymorphisms (SNPs) that associate with cancer susceptibility have been detected in or around ENPP2 (9). Promoter regions of ENPP2 were found hyper-methylated in primary invasive breast carcinomas (13), while inhibition of histone deacetylases 3 and 7 with trichostatin A also attenuated ENPP2 expression in colon cancer cells (14), suggesting that ENPP2 expression can be also amenable to epigenetic regulation. In mice, the highly (93%) homologous Enpp2 gene is located in chromosome 15 and has a similar structure (15, 16).
A variety of cell types and/or tissues have been reported to express ENPP2/Enpp2; the highest mRNA levels in healthy conditions have been observed in adipose tissue, brain, and spinal cord, testis and ovary, followed by lung, kidney, and pancreas (15, 17–19), suggesting that ATX/LPA may participate in the homeostasis of these tissues. In disease states, increased mRNA expression has been reported in a large variety of cancer types and cell lines, as well as in different cell types in chronic inflammatory disorders (20).
Several transcription factors have been suggested to control ENPP2/Enpp2 transcription in different cell types and pathophysiological states: Hoxa13 and Hoxd13 in mouse embryonic fibroblasts (21), v-jun in chick embryo fibroblasts (22), c-jun in soft tissue sarcomas (23), Stat3 in breast cancer cells (24), AP-1 in keratinocytes and neuroblastoma cells (25, 26), NFAT1 in melanoma and carcinoma cells (27, 28), as well as NF-kB in keratinocytes and hepatocytes (26, 29, 30). Enpp2 mRNA stability has been reported to be controlled by the RNA-binding Proteins HuR and AUF1 (31), adding an extra level of regulation.
Several extracellular, mainly pro-inflammatory, factors have been suggested to stimulate ENPP2/Enpp2 expression, many through the transcription factors indicated above: TNF in synovial fibroblasts, hepatocytes, hepatoma cell lines, and thyroid cancer cells (32–35), IL-1β in thyroid cancer cells (34), IL-6 in dermal fibroblasts (36), as well as galectin 3 in melanoma cells (27). Different TLR ligands, including LPS, CpG oligonucleotides and poly(I:C), were shown to stimulate ENPP2 expression in THP-1 monocytic cells, likely involving an IFN autocrine-paracrine loop (37, 38). Lysophatidylcholine (LPC), a major component of cell membranes and oxidized lipoproteins as well as the enzymatic substrate of ATX, is a potent inducer of Enpp2 expression in hepatocytes (32). On the other hand, the enzymatic product of ATX, LPA, as well as sphingosine 1 phosphate (S1P), have been suggested to create a negative feedback loop on Enpp2 expression or activity, under certain conditions (34, 39).
ATX Isoforms, Structure, and Enzymatic Activity
Alternative splicing of ENPP2/Enpp2 exons 12 and 21 leads to five, all catalytically active, protein isoforms, named α to ε (15, 40). Isoform β is the most abundant one, likely accounting for the majority of ATX/LPA reported pathophysiological effects. Isoform δ is also abundant, lacking an exon 19 encoded tetrapeptide of unknown function, also missing in isoform ε. Isoform γ is brain specific, and contains an exon 21 encoded 25 aa insert of unknown function (20). Isoforms α and ε are much less abundant, while they contain a 52 aa polybasic insert, encoded by exon 12, that has been shown to bind to heparin and heparin sulfate proteoglycans (41). Proteolytic cleavage of a N-terminal hydrophobic sequence that functions as a signal peptide (42, 43) and glycosylation at asparagine residues (42–45), are necessary for secretion and optimal enzymatic activity.
ATX can be found catalytically active in most biological fluids, such as serum/plasma, bronchoalveolar lavage fluid (BALF), blister fluid, cerebrospinal fluid, synovial fluid, peritoneal fluid, and urine (20). The major source of serum ATX is likely the adipose tissue, as conditional genetic deletion of Enpp2 in adipocytes resulted in a 38% decrease of plasma LPA (17), whereas ubiquitous heterozygous deletion results in a 50% reduction (46–48). Moreover, ATX has been shown to be secreted, in healthy conditions, from bronchial epithelial cells (49) and high endothelial venules (19), as well as choroid plexus epithelium cells (43), activated astrocytes and oligodendrocytes in the brain (50). Intriguingly, ATX has been also detected in exosomes (51), cell derived vesicles that have been suggested to mediate intercellular or cross-tissue signaling.
ATX consists of two N-terminal somatomedin B-like (SMB) domains, a central phosphodiesterase (PDE) domain and a nuclease-like domain (NUC) in its C-terminus (16, 52, 53). The SMB domains, stabilized by four pairs of disulphide bonds, likely mediate ATX binding to integrins, thus localizing LPA production to the cell surface (19, 52, 54–56). The PDE domain, which interacts with both SMB and NUC domains, contains the active catalytic site consisted of a threonine residue (Thr209/210, for mouse and human, respectively) and two zinc ions coordinated by conserved aspartate and histidine residues. It contains a hydrophobic lipid-binding pocket that can accommodate various LPC and LPA species and an open tunnel that could serve as an exit to LPA (53).
LPC, the enzymatic substrate of ATX, is highly abundant in the circulation, predominantly associated with albumin and lipoproteins (57). LPC is synthesized through the hydrolysis of phosphatidylcholine (PC) by phospholipases (PLA2, PLA1) and lecithin cholesterol acyltransferase (LCAT) enzymes (58). ATX has a preference for shorter and unsaturated fatty acid chains, depending on divalent cations such as Co2+ or Mn2+ (20, 53). Although ATX can also hydrolyze sphingosylphosphorylcholine (SPC, the precursor of S1P) and nucleotides in vitro, genetic and pharmacologic studies in mice established that the main enzymatic activity of ATX in vivo is LPC hydrolysis and the production of extracellular LPA (20, 53).
LPA, Receptors, and Signaling
LPA consists of a glycerol backbone, a single fatty acyl chain of varying length and saturation, and a free phosphate group as a polar head. It can be found in most biological fluids, mostly following the expression profile of ATX (57, 59). LPA levels in serum are much higher than those in plasma, due to the release of LPC and other phospholipids from activated platelets during coagulation and their hydrolysis by ATX (60, 61). Moreover, the LPA concentration in plasma (~0.7 μM) is significantly lower than LPC's (~200 μM), while the predominant LPA species (18:2 > 20:4 > 18:1) are not analogous to the corresponding LPC ones (16:0 > 18:1/18:0 > 20:4); similar observations were made in BALFs (62). This can be likely explained by the slow release of LPA from ATX, due to its high affinity for LPA (39, 63), as well by the rapid turnover of LPA, as shown after the pharmacological inhibition of ATX in vivo (64, 65). Although there are other biosynthetic routes for LPA production, any increases in the extracellular LPA content of biological fluids and local sites can be attributed to the lysoPLD activity of ATX (58). On the other hand, a group of membrane-associated lipid-phosphate phosphatases (LPPs) have been suggested as negative regulators of LPA levels, adding an extra layer of regulation of its effects (66, 67).
LPA signals through at least six type I rhodopsin-like receptors (LPARs) that exhibit widespread, but differential, tissue distribution, as well as overlapping specificities (68). The orphan GPR87 and P2Y10 receptors (69, 70), as well as the receptor for advanced glycation end products (RAGE) (71) and the intracellular peroxisome proliferator-activated receptor γ (PPARγ) (72), have also been suggested to mediate LPA signaling. Little is known on LPARs functional conformation and possible associations; LPAR1 has been detected in lipid rafts (73, 74) and suggested to heterodimerize with CD14 (74) and CD97 (75).
LPARs couple with G-proteins, crucial molecular switches activating numerous signal transduction pathways (76). G-protein coupled receptors (GPCRs) is the largest family of cell-surface molecules involved in signal transduction, and their aberrant function has been linked with various human diseases, thus representing almost 50% of current therapeutic targets (77). Many in vitro studies, extensively reviewed elsewhere (20, 57, 61), have shown that LPA: stimulates the mitogenic Ras-Raf-MEK-ERK pathway and the PI3K pathway promoting cell survival through Gαi; induces RhoA-mediated cytoskeletal remodeling, as well as cell migration and invasion through Gα12/13 in cooperation with the Gαi-mediated Rac activation pathway; activates phospholipase C, through Gαq, with consequent production of second messengers. Of note, most in vitro effects of LPA were reported at concentrations much higher than the physiological concentrations, as found in healthy biological fluids, suggesting that they likely concern pathophysiological situations with increased levels of LPA. Overall, any LPA effect in each cell type will depend on its local concentration, regulated by ATX and LPPs, the levels of possible agonists and antagonists and the relative abundance of the different receptor subtypes.
ATX/LPA in Pathophysiology
Ubiquitous genetic deletion of ATX and abrogation of LPA production resulted to embryonic lethality in mice due to malformations of the vascular and neural systems (46–48, 78), indicating a major role for ATX in development; reviewed in Moolenaar et al. (79). Of note, the embryonic phenotype of ATX knock out mice did not resemble the phenotype of any of the individual LPA receptors knock out mice (68), suggesting that coordinated LPA signaling through various receptors is necessary for the observed ATX effects in embryonic development; non-catalytic effects of ATX in development are also possible especially in the neural system (50, 80). Accordingly, elevated ATX levels have been detected in human pregnancy, further modulated in pregnancy-related pathophysiological conditions (81–86).
Notwithstanding the necessity for ATX in embryonic life, induced genetic deletion or long-term pharmaceutical targeting of ATX in adult mice was shown to be well tolerated (18), indicating that the majority of ATX/LPA (>80%) is dispensable in adult healthy life. The remaining ATX-mediated LPA levels, together possibly with LPA produced via other routes (58) are likely adequate to maintain a healthy tissue homeostasis. Given the importance of ATX in embryonic development but not in adult life, the overexpression of ATX in a pathophysiological condition suggests ATX/LPA as a developmental pathway aberrantly re-expressed in pathophysiological situations.
One of the main features of the embryonic lethal phenotype of ATX knock out mice was the aberrant vascular system, as also seen upon ATX knockdown in zebrafish (87) and in line with the suggested role of LPA in vascular homeostasis (88, 89). A similar phenotype was also seen in the embryos of transgenic mice overexpressing ATX (90) and LPP3 knock out mice (91, 92) that sustain much higher levels of LPA than wt mice, suggesting that LPA levels should be tightly regulated during development. Of note, Ga13−/− embryos display similar impairments in the vasculature as the Enpp2−/− embryos (46, 93), suggesting Ga13 as the predominant G-protein mediating ATX/LPA effects in the vasculature. In adult life, LPA has been suggested to modulate endothelial cell physiology, through the stimulation of the expression of angiogenesis related genes and the modulation of their permeability (88, 89, 91). Beyond endothelial cells, LPA has a plethora of effects on other cells of the vessel wall, as well as on blood cells including platelets. Moreover, LPA is generated during mild oxidation of LDL, while its levels accumulate in atherosclerotic plaques, suggesting a role for ATX/LPA in atherosclerosis (94, 95).
The possible involvement of ATX/LPA in atherosclerosis is further underscored by the fact that the adipose tissue is a major source of systemic ATX, while its effects, through LPA, can classify ATX as an adipokine. Although the effects of ATX/LPA in adiposity are not clear (17, 96, 97), the ATX-LPA pathway has been suggested to participate in obesity related insulin resistance and the regulation of glucose homeostasis (98), with many implications for the pathogenesis of different metabolic disorders. However, the autocrine and/or paracrine effects of ATX/LPA in metabolism and the consequent effects in disease pathogenesis have not yet been fully explored.
ATX was first isolated due to its ability to promote the motility of melanoma cells (1). Accordingly, many xenograft studies have shown that ATX knock down in melanoma cells, as well as pharmacological inhibition of ATX and LPAR antagonism, attenuate the metastasis of melanoma cells in the lungs of mice, well establishing a role for ATX/LPA in metastasis; reviewed in Leblanc and Peyruchaud (99). Beyond melanomas, interaction of ATX with integrin αvβ3 on tumor cells, has been reported to control the metastasis of breast cancer to the bone [reviewed in (56, 100)].
Transgenic over-expression of Enpp2, as well as Lpar 1, 2, or 3, in the mammary gland resulted in spontaneous breast cancer development (101), indicating a role for the ATX/LPA axis in breast cancer. However, spontaneous carcinogenesis was only observed in aged mice, suggesting that ATX/LPA act synergistically with oncogenic age-related signals. Notwithstanding the conflicting reports on ATX levels in breast cancer, the source of ATX in breast cancer was suggested to be the adjacent mammary fat pads, rather than the cancer cells themselves (102), suggesting that ATX can have paracrine effects in cancer development. In the liver, genetic deletion of Enpp2 from hepatocytes attenuated hepatocellular carcinoma (HCC) development, revealing ATX/LPA autocrine effects in hepatocyte metabolism (32, 103). Increased ATX expression has been reported in many other types of cancer, including thyroid and ovarian (20, 104).
Increased ATX levels have been also reported in neuroblastomas and glioblastomas (50) and given the abundant expression of the brain specific isoform ATXγ as well the neuronal defects of the Enpp2−/− mice, a role for ATX/LPA in brain cancer seems likely, but it remains yet unexplored. However, another major role for ATX/LPA was revealed in the brain, as it was shown that PLA2/ATX-dependent LPA/LPAR1 signaling is crucial for the initiation of neuropathic pain (105, 106). Moreover, ATX was shown to modulate oligodendrocyte physiology and differentiation via catalytic and non-catalytic functions (50, 107). In this context, increased ATX and LPA levels have been reported in the sera and cerebrospinal fluid (CSF) of multiple sclerosis patients (108–110), while pharmacologic inhibition of ATX attenuated the development of experimental autoimmune encephalomyelitis (111).
Besides multiple sclerosis, ATX/LPA were shown to have a role in the pathogenesis of other chronic inflammatory diseases. Conditional genetic deletion of ATX from synovial fibroblasts or pharmacologic inhibition attenuated the development of inflammatory arthritis in animal models (33, 112), suggesting a major role for ATX/LPA in rheumatoid arthritis (113, 114). TNF-induced ATX secretion from synovial fibroblasts was shown to result in increased production of LPA which in turn stimulated, in an autocrine mode, cytoskeletal re-organization, proliferation, and migration of synovial fibroblasts (33), the main effector cells in disease pathogenesis. Moreover, increased ATX staining was noted in lymphoid aggregates, in line with the suggestion that ATX can be an adhesive substrate for homing lymphocytes, facilitating their transmigration across endothelial layers in different modes (19, 115–118). Further to the possible regulation of immune responses by ATX/LPA, LPA was recently shown to convert monocytes to macrophages (119).
Chronic inflammation of the liver, due to cytotoxic, viral or metabolic stimuli, was shown to stimulate ATX secretion from hepatocytes, while LPA was shown to activate hepatic stellate cells and to amplify pro-fibrotic signals (32). Conditional genetic deletion of Enpp2 from hepatocytes or pharmacological inhibition of ATX, attenuated the development of fibrosis in a cytotoxic model (32). Increased ATX expression has been reported in patients with chronic liver diseases of different etiologies, suggesting ATX as a diagnostic marker of different forms of liver fibrosis (32, 120). ATX/LPA have been also implicated in the fibrosis of other tissues, such as renal fibrosis (121) and skin fibrosis (36, 122).
ATX/LPA in Pulmonary Fibrosis
Enpp2 has been suggested, using genome-wide linkage analysis coupled with expression profiling, as a candidate gene controlling lung function, development and remodeling (123). Accordingly, Enpp2−/− mice were found to be embryonically lethal (46–48, 78), while Lpar1−/− mice were shown to have reduced alveolar septal formation during development (124). In adult life, ATX is constitutively expressed by bronchial epithelial cells, in both humans, and mice, and can be detected in BALFs (49, 125). However, a 50% reduction of systemic ATX levels in the heterozygous Enpp2+/− mice or genetic abrogation of bronchial Enpp2 expression had no major phenotypic effect in the lungs of mice, suggesting that tissue homeostasis in health does not require large amounts of LPA (49, 126). On the other hand, transgenic overexpression of Enpp2 from the bronchial epithelium or from the liver resulting to 200% increases of ATX systemic levels, had no gross phenotypic effect in the lung either, suggesting that ATX/LPA are not sufficient to induce lung damage per se (126).
Subsegmental allergic challenge of asthma patients induced ATX/LPA levels in their BALFs (127, 128), while pharmacologic inhibition of ATX resulted in a marked attenuation of Th2 cytokines and allergic lung inflammation in a triple-allergen mouse asthma model (128); conflicting reports have suggested both pro-inflammatory and anti-inflammatory roles for LPAR2 (128–130). Therefore, a role for ATX/LPA in asthma seems likely and consistent with early reports on LPA effects in the proliferation and contraction of airway smooth muscle cells (131, 132).
Increased ATX staining has been detected in lung tissue samples from IPF and fibrotic non-specific interstitial pneumonia (fNSIP) patients, compared to other interstitial diseases and especially control samples (49). ATX localized mainly within the hyperplastic bronchiolar epithelium, but it was also detected weakly on alveolar epithelium around fibroblastic foci, interstitial macrophages, and fibroblast like cells. On the contrary, ATX was minimally localized within both the inflammatory components of cellular NSIP lung samples and in areas of loose connective tissue, called Masson bodies, representing the pathogenic hallmark of cryptogenic organizing pneumonia. These two latter forms of idiopathic interstitial pneumonias have a propitious prognosis and an excellent treatment response to corticosteroids, indicating that ATX up-regulation is closely associated with more progressive and irreversible forms of pulmonary fibrosis, such as IPF/UIP and fNSIP (49). Of note, as ATX has been suggested to bind to integrins at the surface of platelets and cancer cells (52, 54, 56), it cannot be excluded that ATX can bind to the surface of lung cells via integrins, thus avoiding clearance while exerting locally-produced LPA effects. In turn, the levels of specific LPA species have been found moderately increased in BALFs and exhaled breath condensates collected from IPF patients (133, 134); however, larger studies are needed.
A similar ATX staining profile was observed in the lungs of mice treated with bleomycin (BLM) (49), the most widely used animal model of pulmonary inflammation and fibrosis (135, 136), while increased ATX levels were detected in the corresponding BALFs (49, 62). Conditional genetic deletion of Enpp2 from bronchial epithelial cells (CC10+) and macrophages (LysM+), the main pulmonary cells expressing ATX, reduced BALF ATX levels and disease severity thus confirming the pulmonary ATX sources as well as establishing a pathogenic role for ATX. However, BALF ATX levels remained relatively high, while the modeled disease was not completely attenuated, suggesting additional, extrapulmonary sources of ATX. ATX levels in BALF correlated with total protein and albumin measurements, pointing to a possible extravasation of ATX from the circulation; paradoxically, no major effects in BLM-induced fibrosis development were noted in genetically modified mice with increased or decreased serum and systemic levels of ATX (49). Nevertheless, systemic pharmacologic inhibition of ATX, both with small molecules and DNA aptamers, decreased LPA levels, and attenuated pulmonary fibrosis (49, 137, 138). It should be noted that ATX inhibition with PAT-048 (Bristol Myers Squibb; WO2012024620) was reported to have no effects in BLM-induced pulmonary fibrosis (62), most likely due to experimental settings and compound characteristics. However, the therapeutic potential of targeting the ATX/LPA axis was recently re-evaluated, where yet another ATX inhibitor was shown to prevent BLM-induced pulmonary fibrosis (139). Many more small molecule ATX inhibitors have been reported (140, 141), however they are still not tested in animal models of pulmonary fibrosis. Intriguingly, the bile salt tauroursodeoxycholate (TUDCA) was recently reported to be a partial non-competitive inhibitor of ATX (142), suggesting that the previously reported therapeutic effects of TUDCA in BLM-induced fibrosis (143), could be due to ATX inhibition.
Moreover, an autocrine pathway linking ATX, LPA signaling and b-catenin was recently reported to contribute to fibrosis progression in lung allografts, one of the primary causes of long-term graft failure after organ transplantation (144). Pharmacologic ATX inhibition or LPAR1 antagonism decreased allograft fibrosis (144), further extending the therapeutic potential of targeting the ATX/LPA axis in lung fibroproliferative disorders.
In agreement with a pathogenic role of ATX/LPA in pulmonary fibrosis, ubiquitous genetic deletion of either Lpar1 or Lpar2 also abrogated BLM-induced disease development (133, 145). Pharmacologic antagonism of LPAR1 was shown to be beneficial for the treatment of BLM-treated mice (146), thus stimulating the respective on-going clinical trial (NCT 02068053). Moreover, simultaneous ATX inhibition and LPAR1 antagonism has been reported to have some additive effect in melanoma metastasis (147), warranting further investigation and/or optimization. Beyond LPAR1&2, LPAR6 is the highest expressing LPAR in the lung (not published data), but its possible role in pulmonary pathophysiology and fibrosis has not been explored yet (Figure 1).
Reduced numbers of TUNEL+ cells were noted in the alveolar and bronchial epithelium of BLM-treated Lpar1−/− and Lpar2−/− mice, suggesting that LPA, through LPAR1 and/or 2, promotes epithelial apoptosis (145, 148), the initiating pathogenetic event in this model (135) and, likely, in human patients (149). Interestingly, apoptosing epithelial cells post BLM were shown to express TNF that has a major contribution in the pathogenesis of the modeled disease (150), while TNF has been reported to stimulate ATX expression in other cell types (33, 35). Many other LPA possible effects in pulmonary epithelial cells in vitro have been reported and are detailed elsewhere (151), including the induction of IL-8 secretion resulting to neutrophil influx (152, 153).
LPA stimulation of normal human bronchial epithelial cells has been shown to increase stress fiber formation, and to reorganize integrin αvβ6 at their ends leading to TGF-β activation (154). Integrin αvβ6 has been shown previously to bind and activate TGF-β, a mechanism suggested to regulate pulmonary inflammation and fibrosis (155). TGF-β is the prototype pro-fibrotic factor with a well-documented involvement in the pathogenesis of both the human and the modeled disease, with effects on alveolar epithelial cell injury, myofibroblast differentiation, epithelial-to-mesenchymal transition, and ECM deposition and remodeling (156). TGF-β is produced by different cell types, including alveolar macrophages, while LPA was shown to induce TGF-β expression in pulmonary fibroblasts in vitro (145). Therefore, TGF-β activation and possibly expression is another important mechanism through which LPA promotes pulmonary fibrosis.
BALF isolated from BLM-treated mice stimulates the chemotaxis of pulmonary fibroblasts, which was found attenuated by more than 50% in the absence of Lpar1 expression, indicating that LPA is a major fibroblast chemoattractant (133). The structural organization of LPAR2 has been suggested to govern gradient sensing and the directional migration of fibroblasts in response to LPA (157), while LPA-induced mTORC2-mediated PKC-δ phosphorylation was shown to be critically important for fibroblast migration and pulmonary fibrosis development (158). LPA has been reported to promote, through GPCR-mediated pathways, the cytoskeletal reorganization and proliferation of lung fibroblasts (151), mediated likely from LPAR2 (145) but not from LPAR1 (133). Moreover, LPA signaling, specifically through LPAR1, has been found to suppress, under certain conditions, the apoptosis of primary mouse lung fibroblasts induced by serum deprivation (148). Similar anti-apoptotic effects of LPA have been reported in many cell lines (151), further supporting a role for ATX/LPA in mediating pathologic fibroblast accumulation, the main pathogenetic event in IPF.
Calcium second messenger signals are essential for many critical cellular functions (159). In fibroblasts, calcium homeostasis and ionic mechanisms have been proposed to orchestrate many of their functions, including proliferation, secretion of extracellular matrix components, as well as TGF-β production and differentiation to myofibroblasts (160). In this context, transient receptor potential vanilloid 4 (TRPV4) Ca2+ channels have been shown to get activated in response to matrix stiffness, as found in fibrotic lungs (161), and to mediate fibroblast activation and differentiation (162). Interestingly, LPA is well known to stimulate Ca2+ influx and/or mobilization in many cells (163), while it was recently shown to directly activate a TRPV1 ion channel (164). Although, the activation was intracellular (164), transbilayer LPA movement has been suggested before in the activation of the nuclear PPARγ receptor (72). Therefore, LPA-induced alterations in calcium homeostasis can have dominant effects in the physiology of fibroblasts, as well as many other cell types.
One of the hallmarks of the observed protection from BLM-induced fibrosis in Lpar1−/− mice was the attenuation of BLM-induced vascular leak, indicating a major role of LPA in promoting endothelial permeability upon damage (133). Accordingly, transgenic overexpression of ATX from the liver resulting to elevated circulating LPA levels induced a bleeding diathesis (55). However, the effects of LPA on endothelial permeability remain controversial, while different LPA receptors have been proposed to mediate different effects on endothelial physiology (23, 151, 164). Endothelial dysfunction mainly characterizes the development of atherosclerosis and cardiovascular diseases, however, interstitial lung diseases have all been reported to have a lung vascular disease component (165).
Therefore, ATX-mediated LPA production promotes pleiotropic effects in pulmonary cells stimulating the development of pulmonary fibrosis (Figure 1). Accordingly, ATX inhibition was shown to attenuate BLM-induced pulmonary fibrosis (49, 137, 138), thus providing the proof of principle for therapeutic interventions and stimulating the on-going clinical trial. In a phase 1 study, GLPG1690, a potent and orally bioavailable ATX inhibitor exhibiting a good PK/PD profile (137), was shown to be safe and well tolerated (166), as previously shown with another compound and genetic interventions in mice (18). An exploratory phase 2a study in IPF patients (FLORA; NCT 02738801) was just completed with promising results (expected to be published soon), leading to phase IIb, currently recruiting.
Author Contributions
IN and CM drafted the paper. VA edited it.
Funding
Supported by the Operational Program Competitiveness, Entrepreneurship and Innovation 2014-2020, co-financed by Greece and the European Union (European Regional Development Fund), Action code MIS 5002562. CM was supported by a scholarship from the State Scholarships Foundation (#4688; IKY/Siemens-excellence).
Conflict of Interest Statement
The authors declare that the research was conducted in the absence of any commercial or financial relationships that could be construed as a potential conflict of interest.
Acknowledgments
This review is dedicated to the memory of Andrew Tager, a pioneer in the field of the review.
References
1. Stracke ML, Krutzsch HC, Unsworth EJ, Arestad A, Cioce V, Schiffmann E, et al. Identification, purification, and partial sequence analysis of autotaxin, a novel motility-stimulating protein. J Biol Chem. (1992) 267:2524–9.
2. Murata J, Lee HY, Clair T, Krutzsch HC, Arestad AA, Sobel ME, et al. cDNA cloning of the human tumor motility-stimulating protein, autotaxin, reveals a homology with phosphodiesterases. J Biol Chem. (1994) 269:30479–84.
3. Stefan C, Jansen S, Bollen M. NPP-type ectophosphodiesterases: unity in diversity. Trends Biochem Sci. (2005) 30:542–50. doi: 10.1016/j.tibs.2005.08.005
4. Tokumura A, Majima E, Kariya Y, Tominaga K, Kogure K, Yasuda K, et al. Identification of human plasma lysophospholipase D, a lysophosphatidic acid-producing enzyme, as autotaxin, a multifunctional phosphodiesterase. J Biol Chem. (2002) 277:39436–42. doi: 10.1074/jbc.M205623200
5. Umezu-Goto M, Kishi Y, Taira A, Hama K, Dohmae N, Takio K, et al. Autotaxin has lysophospholipase D activity leading to tumor cell growth and motility by lysophosphatidic acid production. J Cell Biol. (2002) 158:227–33. doi: 10.1083/jcb.200204026
6. Kawagoe H, Soma O, Goji J, Nishimura N, Narita M, Inazawa J, et al. Molecular cloning and chromosomal assignment of the human brain-type phosphodiesterase I/nucleotide pyrophosphatase gene (PDNP2). Genomics (1995) 30:380–4. doi: 10.1006/geno.1995.0036
7. Lee HY, Murata J, Clair T, Polymeropoulos MH, Torres R, Manrow RE, et al. Cloning, chromosomal localization, and tissue expression of autotaxin from human teratocarcinoma cells. Biochem Biophys Res Commun. (1996) 218:714–9. doi: 10.1006/bbrc.1996.0127
8. Ciriello G, Miller ML, Aksoy BA, Senbabaoglu Y, Schultz N, Sander C. Emerging landscape of oncogenic signatures across human cancers. Nat Genet. (2013) 45:1127–33. doi: 10.1038/ng.2762
9. Brisbin AG, Asmann YW, Song H, Tsai YY, Aakre JA, Yang P, et al. Meta-analysis of 8q24 for seven cancers reveals a locus between NOV and ENPP2 associated with cancer development. BMC Med Genet. (2011) 12:156. doi: 10.1186/1471-2350-12-156
10. Grisanzio C, Freedman ML. Chromosome 8q24-Associated Cancers and MYC. Genes Cancer. (2010) 1:555–9. doi: 10.1177/1947601910381380
11. The Cancer Genome Atlas Research N. Comprehensive molecular profiling of lung adenocarcinoma. Nature (2014) 511:543–50. doi: 10.1038/nature13385
12. Federico L, Jeong KJ, Vellano CP, Mills GB. Autotaxin, a lysophospholipase D with pleomorphic effects in oncogenesis and cancer progression. J Lipid Res. (2015) 57:25–35. doi: 10.1194/jlr.R060020
13. Parris TZ, Kovacs A, Hajizadeh S, Nemes S, Semaan M, Levin M, et al. Frequent MYC coamplification and DNA hypomethylation of multiple genes on 8q in 8p11-p12-amplified breast carcinomas. Oncogenesis (2014) 3:e95. doi: 10.1038/oncsis.2014.8
14. Li S, Wang B, Xu Y, Zhang J. Autotaxin is induced by TSA through HDAC3 and HDAC7 inhibition and antagonizes the TSA-induced cell apoptosis. Mol Cancer. (2011) 10:18. doi: 10.1186/1476-4598-10-18
15. Giganti A, Rodriguez M, Fould B, Moulharat N, Coge F, Chomarat P, et al. Murine and human autotaxin alpha, beta, and gamma isoforms: gene organization, tissue distribution, and biochemical characterization. J Biol Chem. (2008) 283:7776–89. doi: 10.1074/jbc.M708705200
16. Nishimasu H, Okudaira S, Hama K, Mihara E, Dohmae N, Inoue A, et al. Crystal structure of autotaxin and insight into GPCR activation by lipid mediators. Nat Struct Mol Biol. (2011) 18:205–12. doi: 10.1038/nsmb.1998
17. Dusaulcy R, Rancoule C, Gres S, Wanecq E, Colom A, Guigne C, et al. Adipose-specific disruption of autotaxin enhances nutritional fattening and reduces plasma lysophosphatidic acid. J Lipid Res. (2011) 52:1247–55. doi: 10.1194/jlr.M014985
18. Katsifa A, Kaffe E, Nikolaidou-Katsaridou N, Economides AN, Newbigging S, McKerlie C, et al. The bulk of autotaxin activity is dispensable for adult mouse life. PLoS ONE (2015) 10:e0143083. doi: 10.1371/journal.pone.0143083
19. Kanda H, Newton R, Klein R, Morita Y, Gunn MD, Rosen SD. Autotaxin, an ectoenzyme that produces lysophosphatidic acid, promotes the entry of lymphocytes into secondary lymphoid organs. Nat Immunol. (2008) 9:415–23. doi: 10.1038/ni1573
20. Barbayianni E, Kaffe E, Aidinis V, Kokotos G. Autotaxin, a secreted lysophospholipase D, as a promising therapeutic target in chronic inflammation and cancer. Prog Lipid Res. (2015) 58:76–96. doi: 10.1016/j.plipres.2015.02.001
21. Williams TM, Williams ME, Kuick R, Misek D, McDonagh K, Hanash S, et al. Candidate downstream regulated genes of HOX group 13 transcription factors with and without monomeric DNA binding capability. Dev Biol. (2005) 279:462–80. doi: 10.1016/j.ydbio.2004.12.015
22. Black EJ, Clair T, Delrow J, Neiman P, Gillespie DA. Microarray analysis identifies Autotaxin, a tumour cell motility and angiogenic factor with lysophospholipase D activity, as a specific target of cell transformation by v-Jun. Oncogene (2004) 23:2357–66. doi: 10.1038/sj.onc.1207377
23. Sioletic S, Czaplinski J, Hu L, Fletcher JA, Fletcher CD, Wagner AJ, et al. c-Jun promotes cell migration and drives expression of the motility factor ENPP2 in soft tissue sarcomas. J Pathol. (2014) 234:190–202. doi: 10.1002/path.4379
24. Azare J, Doane A, Leslie K, Chang Q, Berishaj M, Nnoli J, et al. Stat3 mediates expression of autotaxin in breast cancer. PLoS ONE (2011) 6:e27851. doi: 10.1371/journal.pone.0027851
25. Farina AR, Cappabianca L, Ruggeri P, Di Ianni N, Ragone M, Merolle S, et al. Constitutive autotaxin transcription by Nmyc-amplified and non-amplified neuroblastoma cells is regulated by a novel AP-1 and SP-mediated mechanism and abrogated by curcumin. FEBS Lett. (2012) 586:3681–91. doi: 10.1016/j.febslet.2012.08.026
26. Ryborg AK, Johansen C, Iversen L, Kragballe K. Lysophosphatidylcholine induces keratinocyte differentiation and upregulation of AP-1- and NF-kappaB DNA-binding activity. Acta Derm Venereol. (2004) 84:433–8. doi: 10.1080/00015550410016930
27. Braeuer RR, Zigler M, Kamiya T, Dobroff AS, Huang L, Choi W, et al. Galectin-3 contributes to melanoma growth and metastasis via regulation of NFAT1 and autotaxin. Cancer Res. (2012) 72:5757–66. doi: 10.1158/0008-5472.CAN-12-2424
28. Chen M, O'Connor KL. Integrin alpha6beta4 promotes expression of autotaxin/ENPP2 autocrine motility factor in breast carcinoma cells. Oncogene (2005) 24:5125–30. doi: 10.1038/sj.onc.1208729
29. Lovas A, Weidemann A, Albrecht D, Wiechert L, Weih D, Weih F. p100 Deficiency is insufficient for full activation of the alternative NF-kappaB pathway: TNF cooperates with p52-RelB in target gene transcription. PLoS ONE (2012) 7:e42741. doi: 10.1371/journal.pone.0042741
30. Corcoran DL, Feingold E, Dominick J, Wright M, Harnaha J, Trucco M, et al. Footer: a quantitative comparative genomics method for efficient recognition of cis-regulatory elements. Genome Res. (2005) 15:840–7. doi: 10.1101/gr.2952005
31. Sun S, Zhang X, Lyu L, Li X, Yao S, Zhang J. Autotaxin expression is regulated at the post-transcriptional Level by the RNA-binding proteins HuR and AUF1. J Biol Chem. (2016) 291:25823–36. doi: 10.1074/jbc.M116.756908
32. Kaffe E, Katsifa A, Xylourgidis N, Ninou I, Zannikou M, Harokopos V, et al. Hepatocyte Autotaxin expression promotes liver fibrosis and cancer. Hepatology (2016) 65:1369–83. doi: 10.1002/hep.28973
33. Nikitopoulou I, Oikonomou N, Karouzakis E, Sevastou I, Nikolaidou-Katsaridou N, Zhao Z, et al. Autotaxin expression from synovial fibroblasts is essential for the pathogenesis of modeled arthritis. J Exp Med. (2012) 209:925–33. doi: 10.1084/jem.20112012
34. Benesch MG, Zhao YY, Curtis JM, McMullen TP, Brindley DN. Regulation of autotaxin expression and secretion by lysophosphatidate and sphingosine 1-phosphate. J Lipid Res. (2015) 56:1134–44. doi: 10.1194/jlr.M057661
35. Wu JM, Xu Y, Skill NJ, Sheng H, Zhao Z, Yu M, et al. Autotaxin expression and its connection with the TNF-alpha-NF-kappaB axis in human hepatocellular carcinoma. Mol Cancer (2010) 9:71. doi: 10.1186/1476-4598-9-71
36. Castelino FV, Bain G, Pace VA, Black KE, George L, Probst CK, et al. An Autotaxin/Lysophosphatidic Acid/Interleukin-6 Amplification Loop Drives Scleroderma Fibrosis. Arthrit Rheumatol. (2016) 68:2964–74. doi: 10.1002/art.39797
37. Li S, Xiong C, Zhang J. ATX and LPA receptor 3 are coordinately up-regulated in lipopolysaccharide-stimulated THP-1 cells through PKR and SPK1-mediated pathways. FEBS Lett. (2012) 586:792–7. doi: 10.1016/j.febslet.2012.01.044
38. Song J, Guan M, Zhao Z, Zhang J. Type I interferons function as autocrine and paracrine factors to induce autotaxin in response to TLR activation. PLoS ONE (2015) 10:e0136629. doi: 10.1371/journal.pone.0136629
39. van Meeteren LA, Ruurs P, Christodoulou E, Goding JW, Takakusa H, Kikuchi K, et al. Inhibition of autotaxin by lysophosphatidic acid and sphingosine 1-phosphate. J Biol Chem. (2005) 280:21155–61. doi: 10.1074/jbc.M413183200
40. Hashimoto T, Okudaira S, Igarashi K, Hama K, Yatomi Y, Aoki J. Identification and biochemical characterization of a novel autotaxin isoform, ATXdelta, with a four-amino acid deletion. J Biochem. (2012) 151:89–97. doi: 10.1093/jb/mvr126
41. Houben AJ, van Wijk XM, van Meeteren LA, van Zeijl L, van de Westerlo EM, Hausmann J, et al. The polybasic insertion in autotaxin alpha confers specific binding to heparin and cell surface heparan sulfate proteoglycans. J Biol Chem. (2013) 288:510–9. doi: 10.1074/jbc.M112.358416
42. Jansen S, Stefan C, Creemers JW, Waelkens E, Van Eynde A, Stalmans W, et al. Proteolytic maturation and activation of autotaxin (NPP2), a secreted metastasis-enhancing lysophospholipase D. J Cell Sci. (2005) 118(Pt 14):3081–9. doi: 10.1242/jcs.02438
43. Koike S, Keino-Masu K, Ohto T, Masu M. The N-terminal hydrophobic sequence of autotaxin (ENPP2) functions as a signal peptide. Genes Cells (2006) 11:133–42. doi: 10.1111/j.1365-2443.2006.00924.x
44. Jansen S, Callewaert N, Dewerte I, Andries M, Ceulemans H, Bollen M. An essential oligomannosidic glycan chain in the catalytic domain of autotaxin, a secreted lysophospholipase-D. J Biol Chem. (2007) 282:11084–91. doi: 10.1074/jbc.M611503200
45. Pradere JP, Tarnus E, Gres S, Valet P, Saulnier-Blache JS. Secretion and lysophospholipase D activity of autotaxin by adipocytes are controlled by N-glycosylation and signal peptidase. Biochim Biophys Acta (2007) 1771:93–102. doi: 10.1016/j.bbalip.2006.11.010
46. Fotopoulou S, Oikonomou N, Grigorieva E, Nikitopoulou I, Paparountas T, Thanassopoulou A, et al. ATX expression and LPA signalling are vital for the development of the nervous system. Dev Biol. (2010) 339:451–64. doi: 10.1016/j.ydbio.2010.01.007
47. van Meeteren LA, Ruurs P, Stortelers C, Bouwman P, van Rooijen MA, Pradere JP, et al. Autotaxin, a secreted lysophospholipase D, is essential for blood vessel formation during development. Mol Cell Biol. (2006) 26:5015–22. doi: 10.1128/MCB.02419-05
48. Tanaka M, Okudaira S, Kishi Y, Ohkawa R, Iseki S, Ota M, et al. Autotaxin stabilizes blood vessels and is required for embryonic vasculature by producing lysophosphatidic acid. J Biol Chem. (2006) 281:25822–30. doi: 10.1074/jbc.M605142200
49. Oikonomou N, Mouratis MA, Tzouvelekis A, Kaffe E, Valavanis C, Vilaras G, et al. Pulmonary autotaxin expression contributes to the pathogenesis of pulmonary fibrosis. Am J Respir Cell Mol Biol. (2012) 47:566–74. doi: 10.1165/rcmb.2012-0004OC
50. Yuelling LM, Fuss B. Autotaxin (ATX): A multi-functional and multi-modular protein possessing enzymatic lysoPLD activity and matricellular properties. Biochim Biophys Acta. (2008) 1781:525–30. doi: 10.1016/j.bbalip.2008.04.009
51. Jethwa SA, Leah EJ, Zhang Q, Bright NA, Oxley D, Bootman MD, et al. Exosomes bind autotaxin and act as a physiological delivery mechanism to stimulate LPA receptor signalling in cells. J Cell Sci. (2016) 129:3948–57doi: 10.1242/jcs.184424
52. Hausmann J, Kamtekar S, Christodoulou E, Day JE, Wu T, Fulkerson Z, et al. Structural basis of substrate discrimination and integrin binding by autotaxin. Nat Struct Mol Biol. (2011) 18:198–204. doi: 10.1038/nsmb.1980
53. Perrakis A, Moolenaar WH. Autotaxin: structure-function and signaling. J Lipid Res. (2014) 55:1010–8. doi: 10.1194/jlr.R046391
54. Fulkerson Z, Wu T, Sunkara M, Kooi CV, Morris AJ, Smyth SS. Binding of autotaxin to integrins localizes lysophosphatidic acid production to platelets and mammalian cells. J Biol Chem. (2011) 286:34654–63. doi: 10.1074/jbc.M111.276725
55. Pamuklar Z, Federico L, Liu S, Umezu-Goto M, Dong A, Panchatcharam M, et al. Autotaxin/lysopholipase D and lysophosphatidic acid regulate murine hemostasis and thrombosis. J Biol Chem. (2009) 284:7385–94. doi: 10.1074/jbc.M807820200
56. Leblanc R, Lee SC, David M, Bordet JC, Norman DD, Patil R, et al. Interaction of platelet-derived autotaxin with tumor integrin alphaVbeta3 controls metastasis of breast cancer cells to bone. Blood (2014) 124:3141–50. doi: 10.1182/blood-2014-04-568683
57. Sevastou I, Kaffe E, Mouratis MA, Aidinis V. Lysoglycerophospholipids in chronic inflammatory disorders: the PLA/LPC and ATX/LPA axes. Biochim Biophys Acta. (2013) 1831:42–60. doi: 10.1016/j.bbalip.2012.07.019
58. Aoki J, Inoue A, Okudaira S. Two pathways for lysophosphatidic acid production. Biochim Biophys Acta (2008) 1781:513–8. doi: 10.1016/j.bbalip.2008.06.005
59. Aikawa S, Hashimoto T, Kano K, Aoki J. Lysophosphatidic acid as a lipid mediator with multiple biological actions. J Biochem. (2015) 157:81–9. doi: 10.1093/jb/mvu077
60. Aoki J. Mechanisms of lysophosphatidic acid production. Semin Cell Dev Biol. (2004) 15:477–89. doi: 10.1016/j.semcdb.2004.05.001
61. van Meeteren LA, Moolenaar WH. Regulation and biological activities of the autotaxin-LPA axis. Prog Lipid Res. (2007) 46:145–60. doi: 10.1016/j.plipres.2007.02.001
62. Black KE, Berdyshev E, Bain G, Castelino FV, Shea BS, Probst CK, et al. Autotaxin activity increases locally following lung injury, but is not required for pulmonary lysophosphatidic acid production or fibrosis. Faseb J. (2016) 30:2435–50. doi: 10.1096/fj.201500197R
63. Saunders LP, Cao W, Chang WC, Albright RA, Braddock DT, De La Cruz EM. Kinetic analysis of autotaxin reveals substrate-specific catalytic pathways and a mechanism for lysophosphatidic acid distribution. J Biol Chem. (2011) 286:30130–41. doi: 10.1074/jbc.M111.246884
64. Gierse JK, Thorarensen A, Beltey K, Bradshaw-Pierce E, Cortes-Burgos L, Hall T, et al. A novel autotaxin inhibitor reduces lysophosphatidic acid levels in plasma and the site of inflammation. J Pharmacol Exp Therapeut. (2010) 334:310–7. doi: 10.1124/jpet.110.165845
65. Albers HM, Dong A, van Meeteren LA, Egan DA, Sunkara M, van Tilburg EW, et al. Boronic acid-based inhibitor of autotaxin reveals rapid turnover of LPA in the circulation. Proc Natl Acad Sci USA. (2010) 107:7257–62. doi: 10.1073/pnas.1001529107
66. Tang X, Benesch MG, Brindley DN. Lipid phosphate phosphatases and their roles in mammalian physiology and pathology. J Lipid Res. (2015) 56:2048–60. doi: 10.1194/jlr.R058362
67. Benesch MG, Tang X, Venkatraman G, Bekele RT, Brindley DN. Recent advances in targeting the autotaxin-lysophosphatidate-lipid phosphate phosphatase axis in vivo. J Biomed Res. (2016) 30:272–84. doi: 10.7555/JBR.30.20150058
68. Yung YC, Stoddard NC, Chun J. LPA receptor signaling: pharmacology, physiology, and pathophysiology. J Lipid Res. (2014) 55:1192–214. doi: 10.1194/jlr.R046458
69. Tabata K, Baba K, Shiraishi A, Ito M, Fujita N. The orphan GPCR GPR87 was deorphanized and shown to be a lysophosphatidic acid receptor. Biochem Biophys Res Commun. (2007) 363:861–6. doi: 10.1016/j.bbrc.2007.09.063
70. Murakami M, Shiraishi A, Tabata K, Fujita N. Identification of the orphan GPCR, P2Y(10) receptor as the sphingosine-1-phosphate and lysophosphatidic acid receptor. Biochem Biophys Res Commun. (2008) 371:707–12. doi: 10.1016/j.bbrc.2008.04.145
71. Rai V, Toure F, Chitayat S, Pei R, Song F, Li Q, et al. Lysophosphatidic acid targets vascular and oncogenic pathways via RAGE signaling. J Exp Med. (2012) 209:2339–50. doi: 10.1084/jem.20120873
72. McIntyre TM, Pontsler AV, Silva AR, St Hilaire A, Xu Y, Hinshaw JC, et al. Identification of an intracellular receptor for lysophosphatidic acid (LPA): LPA is a transcellular PPARgamma agonist. Proc Natl Acad Sci USA. (2003) 100:131–6. doi: 10.1073/pnas.0135855100
73. Gobeil F Jr. Bernier SG, Vazquez-Tello A, Brault S, Beauchamp MH, Quiniou C, et al. Modulation of pro-inflammatory gene expression by nuclear lysophosphatidic acid receptor type-1. J Biol Chem. (2003) 278:38875–83. doi: 10.1074/jbc.M212481200
74. Zhao J, He D, Su Y, Berdyshev E, Chun J, Natarajan V, et al. Lysophosphatidic acid receptor 1 modulates lipopolysaccharide-induced inflammation in alveolar epithelial cells and murine lungs. Am J Physiol Lung Cell Mol Physiol. (2011) 301:L547–56. doi: 10.1152/ajplung.00058.2011
75. Ward Y, Lake R, Yin JJ, Heger CD, Raffeld M, Goldsmith PK, et al. LPA receptor heterodimerizes with CD97 to amplify LPA-initiated RHO-dependent signaling and invasion in prostate cancer cells. Cancer Res. (2011) 71:7301–11. doi: 10.1158/0008-5472.CAN-11-2381
76. Oldham WM, Hamm HE. Heterotrimeric G protein activation by G-protein-coupled receptors. Nat Rev Mol Cell Biol. (2008) 9:60–71. doi: 10.1038/nrm2299
77. Dorsam RT, Gutkind JS. G-protein-coupled receptors and cancer. Nat Rev Cancer (2007) 7:79–94. doi: 10.1038/nrc2069
78. Ferry G, Giganti A, Cogι F, Bertaux F, Thiam K, Boutin JA. Functional invalidation of the autotaxin gene by a single amino acid mutation in mouse is lethal. FEBS Lett. (2007) 581:3572–8. doi: 10.1016/j.febslet.2007.06.064
79. Moolenaar WH, Houben AJ, Lee SJ, van Meeteren LA. Autotaxin in embryonic development. Biochim Biophys Acta (2012) 1831:13–9. doi: 10.1016/j.bbalip.2012.09.013
80. Greenman R, Gorelik A, Sapir T, Baumgart J, Zamor V, Segal-Salto M, et al. Non-cell autonomous and non-catalytic activities of ATX in the developing brain. Front Neurosci. (2015) 9:53. doi: 10.3389/fnins.2015.00053
81. Kremer AE, Bolier R, Dixon PH, Geenes V, Chambers J, Tolenaars D, et al. Autotaxin activity has a high accuracy to diagnose intrahepatic cholestasis of pregnancy. J Hepatol. (2015) 62:897–904. doi: 10.1016/j.jhep.2014.10.041
82. Masuda A, Fujii T, Iwasawa Y, Nakamura K, Ohkawa R, Igarashi K, et al. Serum autotaxin measurements in pregnant women: application for the differentiation of normal pregnancy and pregnancy-induced hypertension. Clin Chim Acta (2011) 412:1944–50. doi: 10.1016/j.cca.2011.06.039
83. Sozen I, Aslan Cetin B, Gezer A, Ekmekci H, Kaya B, Tuten A, et al. Placental autotaxin expression is diminished in women with pre-eclampsia. Hypert Preg. (2015) 41:1406–11. doi: 10.1111/jog.12742
84. Erenel H, Yilmaz N, Cift T, Bulut B, Sozen I, Aslan Cetin B, et al. Maternal serum autotaxin levels in early- and late-onset preeclampsia. Hypert Preg. (2017) 36:310–4. doi: 10.1080/10641955.2017.1388389
85. Tokumura A, Kanaya Y, Miyake M, Yamano S, Irahara M, Fukuzawa K. Increased production of bioactive lysophosphatidic acid by serum lysophospholipase D in human pregnancy. Biol Reprod. (2002) 67:1386–92. doi: 10.1095/biolreprod.102.004051
86. Tokumura A, Kume T, Taira S, Yasuda K, Kanzaki H. Altered activity of lysophospholipase D, which produces bioactive lysophosphatidic acid and choline, in serum from women with pathological pregnancy. Mol Human Reproduct. (2009) 15:301–10. doi: 10.1093/molehr/gap017
87. Yukiura H, Hama K, Nakanaga K, Tanaka M, Asaoka Y, Okudaira S, et al. Autotaxin regulates vascular development via multiple lysophosphatidic acid (LPA) receptors in zebrafish. J Biol Chem. (2011) 286:43972–83. doi: 10.1074/jbc.M111.301093
88. Kazlauskas A. Lysophosphatidic acid contributes to angiogenic homeostasis. Exp Cell Res. (2015) 333:166–70. doi: 10.1016/j.yexcr.2014.11.012
89. Chen Y, Ramakrishnan DP, Ren B. Regulation of angiogenesis by phospholipid lysophosphatidic Acid. Front Biosci. (2013) 18:852–61. doi: 10.2741/4148
90. Yukiura H, Kano K, Kise R, Inoue A, Aoki J. Autotaxin overexpression causes embryonic lethality and vascular defects. PLoS ONE (2015) 10:e0126734. doi: 10.1371/journal.pone.0126734
91. Ren H, Panchatcharam M, Mueller P, Escalante-Alcalde D, Morris AJ, Smyth SS. Lipid phosphate phosphatase (LPP3) and vascular development. Biochim Biophys Acta (2013) 1831:126–32. doi: 10.1016/j.bbalip.2012.07.012
92. Escalante-Alcalde D, Hernandez L, Le Stunff H, Maeda R, Lee HS Jr, Gang C, et al. The lipid phosphatase LPP3 regulates extra-embryonic vasculogenesis and axis patterning. Development (2003) 130:4623–37. doi: 10.1242/dev.00635
93. Offermanns S, Mancino V, Revel JP, Simon MI. Vascular system defects and impaired cell chemokinesis as a result of Galpha13 deficiency. Science (1997) 275:533–6. doi: 10.1126/science.275.5299.533
94. Schober A, Siess W. Lysophosphatidic acid in atherosclerotic diseases. Br J Pharmacol. (2012) 167:465–82. doi: 10.1111/j.1476-5381.2012.02021.x
95. Abdel-Latif A, Heron PM, Morris AJ, Smyth SS. Lysophospholipids in coronary artery and chronic ischemic heart disease. Curr Opin Lipidol. (2015) 26:432–7. doi: 10.1097/MOL.0000000000000226
96. Nishimura S, Nagasaki M, Okudaira S, Aoki J, Ohmori T, Ohkawa R, et al. ENPP2 contributes to adipose tissue expansion in diet-induced obesity. Diabetes (2014) 63:4154–64. doi: 10.2337/db13-1694
97. Federico L, Ren H, Mueller PA, Wu T, Liu S, Popovic J, et al. Autotaxin and its product lysophosphatidic acid suppress brown adipose differentiation and promote diet-induced obesity in mice. Mol Endocrinol. (2012) 26:786–97. doi: 10.1210/me.2011-1229
98. Rancoule C, Dusaulcy R, Treguer K, Gres S, Attane C, Saulnier-Blache JS. Involvement of autotaxin/lysophosphatidic acid signaling in obesity and impaired glucose homeostasis. Biochimie (2013) 96:140–3. doi: 10.1016/j.biochi.2013.04.010
99. Leblanc R, Peyruchaud O. New insights in the autotaxin/LPA axis in cancer development and metastasis. Exp Cell Res. (2014) 333:183–9. doi: 10.1016/j.yexcr.2014.11.010
100. Peyruchaud O, Leblanc R, David M. Pleiotropic activity of lysophosphatidic acid in bone metastasis. Biochim Biophys Acta (2013) 1831:99–104. doi: 10.1016/j.bbalip.2012.06.004
101. Liu S, Umezu-Goto M, Murph M, Lu Y, Liu W, Zhang F, et al. Expression of autotaxin and lysophosphatidic acid receptors increases mammary tumorigenesis, invasion, and metastases. Cancer Cell (2009) 15:539–50. doi: 10.1016/j.ccr.2009.03.027
102. Benesch MG, Tang X, Dewald J, Dong WF, Mackey JR, Hemmings DG, et al. Tumor-induced inflammation in mammary adipose tissue stimulates a vicious cycle of autotaxin expression and breast cancer progression. FASEB J. (2015) 29:3990–4000. doi: 10.1096/fj.15-274480
103. Kime C, Sakaki-Yumoto M, Goodrich L, Hayashi Y, Sami S, Derynck R, et al. Autotaxin-mediated lipid signaling intersects with LIF and BMP signaling to promote the naive pluripotency transcription factor program. Proc Natl Acad Sci USA. (2016) 113:12478–83. doi: 10.1073/pnas.1608564113
104. Benesch MGK, Yang Z, Tang X, Meng G, Brindley DN. Lysophosphatidate signaling: the tumor microenvironment's new nemesis. Trends Cancer. (2017) 3:748–52. doi: 10.1016/j.trecan.2017.09.004
105. Bickelhaupt S, Erbel C, Timke C, Wirkner U, Dadrich M, Flechsig P, et al. Effects of CTGF blockade on attenuation and reversal of radiation-induced pulmonary fibrosis. J Natl Cancer Inst. (2017) 109. doi: 10.1093/jnci/djw339
106. Sakai N, Chun J, Duffield JS, Lagares D, Wada T, Luster AD, et al. Lysophosphatidic acid signaling through its receptor initiates profibrotic epithelial cell fibroblast communication mediated by epithelial cell derived connective tissue growth factor. Kidney Int. (2017) 91:628–41. doi: 10.1016/j.kint.2016.09.030
107. Wheeler NA, Lister JA, Fuss B. The autotaxin-lysophosphatidic acid axis modulates histone acetylation and gene expression during oligodendrocyte differentiation. J Neurosci. (2015) 35:11399–414. doi: 10.1523/JNEUROSCI.0345-15.2015
108. Kim SE, Choo J, Yoon J, Chu JR, Bae YJ, Lee S, et al. Genome-wide analysis identifies colonic genes differentially associated with serum leptin and insulin concentrations in C57BL/6J mice fed a high-fat diet. PLoS ONE (2017) 12:e0171664. doi: 10.1371/journal.pone.0171664
109. Hino K, Horigome K, Nishio M, Komura S, Nagata S, Zhao C, et al. Activin-A enhances mTOR signaling to promote aberrant chondrogenesis in fibrodysplasia ossificans progressiva. J Clin Invest. (2017) 127:3339–52. doi: 10.1172/JCI93521
110. Schmitz K, Brunkhorst R, de Bruin N, Mayer CA, Haussler A, Ferreiros N, et al. Dysregulation of lysophosphatidic acids in multiple sclerosis and autoimmune encephalomyelitis. Acta Neuropathol Commun. (2017) 5:42. doi: 10.1186/s40478-017-0446-4
111. Sakai N, Nakamura M, Lipson KE, Miyake T, Kamikawa Y, Sagara A, et al. Inhibition of CTGF ameliorates peritoneal fibrosis through suppression of fibroblast and myofibroblast accumulation and angiogenesis. Sci Rep. (2017) 7:5392. doi: 10.1038/s41598-017-05624-2
112. Nikitopoulou I, Kaffe E, Sevastou I, Sirioti I, Samiotaki M, Madan D, et al. A metabolically-stabilized phosphonate analog of lysophosphatidic acid attenuates collagen-induced arthritis. PLoS ONE (2013) 8:e70941. doi: 10.1371/journal.pone.0070941
113. Bourgoin SG, Zhao C. Autotaxin and lysophospholipids in rheumatoid arthritis. Curr Opin Investig Drugs. (2010) 11:515–26.
114. Orosa B, Garcia S, Conde C. The Autotaxin-Lysophosphatidic acid pathway in pathogenesis of rheumatoid arthritis. Eur J Pharmacol. (2015) 765:228–33. doi: 10.1016/j.ejphar.2015.08.028
115. Nakasaki T, Tanaka T, Okudaira S, Hirosawa M, Umemoto E, Otani K, et al. Involvement of the lysophosphatidic acid-generating enzyme autotaxin in lymphocyte-endothelial cell interactions. Am J Pathol. (2008) 173:1566–76. doi: 10.2353/ajpath.2008.071153
116. Zhang Y, Chen YC, Krummel MF, Rosen SD. Autotaxin through lysophosphatidic acid stimulates polarization, motility, and transendothelial migration of naive t cells. J Immunol. (2012) 189:3914–24. doi: 10.4049/jimmunol.1201604
117. Bai Z, Cai L, Umemoto E, Takeda A, Tohya K, Komai Y, et al. Constitutive lymphocyte transmigration across the basal lamina of high endothelial venules is regulated by the autotaxin/lysophosphatidic acid axis. J Immunol. (2013) 190:2036–48. doi: 10.4049/jimmunol.1202025
118. Knowlden S, Georas SN. The Autotaxin-LPA Axis Emerges as a Novel Regulator of Lymphocyte Homing and Inflammation. J Immunol. (2014) 192:851–7. doi: 10.4049/jimmunol.1302831
119. Sun S, Wang R, Song J, Guan M, Li N, Zhang X, et al. Blocking gp130 signaling suppresses autotaxin expression in adipocytes and improves insulin sensitivity in diet-induced obesity. (2017) 58:2102–13. doi: 10.1194/jlr.M075655
120. Ikeda H, Yatomi Y. Autotaxin in liver fibrosis. Clin Chim Acta (2012) 413:1817–21. doi: 10.1016/j.cca.2012.07.014
121. Pradere JP, Gonzalez J, Klein J, Valet P, Gres S, Salant D, et al. Lysophosphatidic acid and renal fibrosis. Biochim Biophys Acta. (2008) 1781:582–7. doi: 10.1016/j.bbalip.2008.04.001
122. Zheng H, Högberg J, Stenius U. ATM-activated autotaxin (ATX) propagates inflammation and DNA damage in lung epithelial cells: a new mode of action for silica-induced DNA damage? Carcinogenesis (2017) 38:1196–206. doi: 10.1093/carcin/bgx100
123. Ganguly K, Stoeger T, Wesselkamper SC, Reinhard C, Sartor MA, Medvedovic M, et al. Candidate genes controlling pulmonary function in mice: transcript profiling and predicted protein structure. Physiol Genomics (2007) 31:410–21. doi: 10.1152/physiolgenomics.00260.2006
124. Funke M, Knudsen L, Lagares D, Ebener S, Probst CK, Fontaine BA, et al. Lysophosphatidic acid signaling through the lysophosphatidic acid-1 receptor is required for alveolarization. Am J Res Cell Mol Biol. (2016) 55:105–16. doi: 10.1165/rcmb.2015-0152OC
125. Yang Y, Mou L, Liu N, Tsao MS. Autotaxin expression in non-small-cell lung cancer. Am J Res Cell Mol Biol. (1999) 21:216–22. doi: 10.1165/ajrcmb.21.2.3667
126. Mouratis MA, Magkrioti C, Oikonomou N, Katsifa A, Prestwich GD, Kaffe E, et al. Autotaxin and endotoxin-induced acute lung injury. PLoS ONE. (2015) 10:e0133619. doi: 10.1371/journal.pone.0133619
127. Georas SN, Berdyshev E, Hubbard W, Gorshkova IA, Usatyuk PV, Saatian B, et al. Lysophosphatidic acid is detectable in human bronchoalveolar lavage fluids at baseline and increased after segmental allergen challenge. Clin Exp Allergy (2007) 37:311–22. doi: 10.1111/j.1365-2222.2006.02626.x
128. Park GY, Lee YG, Berdyshev E, Nyenhuis S, Du J, Fu P, et al. Autotaxin production of lysophosphatidic acid mediates allergic asthmatic inflammation. Am J Res Crit Care Med. (2013) 188:928–40. doi: 10.1164/rccm.201306-1014OC
129. Zhao Y, Tong J, He D, Pendyala S, Evgeny B, Chun J, et al. Role of lysophosphatidic acid receptor LPA2 in the development of allergic airway inflammation in a murine model of asthma. Respir Res. (2009) 10:114. doi: 10.1186/1465-9921-10-114
130. Emo J, Meednu N, Chapman TJ, Rezaee F, Balys M, Randall T, et al. Lpa2 is a negative regulator of both dendritic cell activation and murine models of allergic lung inflammation. J Immunol. (2012) 188:3784–90. doi: 10.4049/jimmunol.1102956
131. Cerutis DR, Nogami M, Anderson JL, Churchill JD, Romberger DJ, Rennard SI, et al. Lysophosphatidic acid and EGF stimulate mitogenesis in human airway smooth muscle cells. Am J Physiol. (1997) 273(1 Pt 1):L10–5. doi: 10.1152/ajplung.2002.282.1.L91
132. Toews ML, Ustinova EE, Schultz HD. Lysophosphatidic acid enhances contractility of isolated airway smooth muscle. J Appl Physiol. (1997) 83:1216–22. doi: 10.1152/jappl.1997.83.4.1216
133. Tager AM, LaCamera P, Shea BS, Campanella GS, Selman M, Zhao Z, et al. The lysophosphatidic acid receptor LPA1 links pulmonary fibrosis to lung injury by mediating fibroblast recruitment and vascular leak. Nat Med. (2008) 14:45–54. doi: 10.1038/nm1685
134. Martinez FJ, Collard HR, Pardo A, Raghu G, Richeldi L, Selman M, et al. Idiopathic pulmonary fibrosis. Nat Rev Dis Prim. (2017) 3:17074. doi: 10.1038/nrdp.2017.74
135. Valdes-Rives SA, Gonzalez-Arenas A. Autotaxin-Lysophosphatidic acid: from inflammation to cancer development. Med Inflamm. (2017) 2017:15. doi: 10.1155/2017/9173090
136. Baader M, Bretschneider T, Broermann A, Rippmann JF, Stierstorfer B, Kuttruff CA, et al. Characterisation of the properties of a selective, orally bioavailable autotaxin inhibitor in preclinical models of advanced stages of liver fibrosis. Br J Pharmacol. (2017) 175:693–707. doi: 10.1111/bph.14118
137. Desroy N, Housseman C, Bock X, Joncour A, Bienvenu N, Cherel L, et al. Discovery of 2-[[2-Ethyl-6-[4-[2-(3-hydroxyazetidin-1-yl)-2-oxoethyl]piperazin-1-yl]-8-methyli midazo[1,2-a]pyridin-3-yl]methylamino]-4-(4-fluorophenyl)thiazole-5-carbonitrile (GLPG1690), a first-in-class autotaxin inhibitor undergoing clinical evaluation for the treatment of idiopathic pulmonary fibrosis. J Med Chem. (2017) 60:3580–90. doi: 10.1021/acs.jmedchem.7b00032
138. Kato K, Ikeda H, Miyakawa S, Futakawa S, Nonaka Y, Fujiwara M, et al. Structural basis for specific inhibition of Autotaxin by a DNA aptamer. Nat Struct Mol Biol. (2016) 23:395–401. doi: 10.1038/nsmb.3200
139. Nikolaou A, Ninou I, Kokotou MG, Kaffe E, Afantitis A, Aidinis V, et al. Hydroxamic acids constitute a novel class of autotaxin inhibitors that exhibit in vivo efficacy in a pulmonary fibrosis model. J Med Chem. (2018) 61:3697–711. doi: 10.1021/acs.jmedchem.8b00232
140. Barbayianni E, Magrioti V, Moutevelis-Minakakis P, Kokotos G. Autotaxin inhibitors: a patent review. Expert Opin Ther Pat. (2013) 23:1123–32. doi: 10.1517/13543776.2013.796364
141. Nikolaou A, Kokotou MG, Limnios D, Psarra A, Kokotos G. Autotaxin inhibitors: a patent review (2012-2016). Expert Opin Ther Pat. (2017) 27:815–29. doi: 10.1080/13543776.2017.1323331
142. Keune WJ, Hausmann J, Bolier R, Tolenaars D, Kremer A, Heidebrecht T, et al. Steroid binding to Autotaxin links bile salts and lysophosphatidic acid signalling. Nat Commun. (2016) 7:11248. doi: 10.1038/ncomms11248
143. Tanaka Y, Ishitsuka Y, Hayasaka M, Yamada Y, Miyata K, Endo M, et al. The exacerbating roles of CCAAT/enhancer-binding protein homologous protein (CHOP) in the development of bleomycin-induced pulmonary fibrosis and the preventive effects of tauroursodeoxycholic acid (TUDCA) against pulmonary fibrosis in mice. Pharmacol Res. (2015) 99:52–62. doi: 10.1016/j.phrs.2015.05.004
144. Cao P, Aoki Y, Badri L, Walker NM, Manning CM, Lagstein A, et al. Autocrine lysophosphatidic acid signaling activates beta-catenin and promotes lung allograft fibrosis. J Clin Inv. (2017) 127:1517–30. doi: 10.1172/JCI88896
145. Huang LS, Fu P, Patel P, Harijith A, Sun T, Zhao Y, et al. Lysophosphatidic acid receptor-2 deficiency confers protection against bleomycin-induced lung injury and fibrosis in mice. Am J Res Cell Mol Biol. (2013) 49:912–22. doi: 10.1165/rcmb.2013-0070OC
146. Swaney JS, Chapman C, Correa LD, Stebbins KJ, Bundey RA, Prodanovich PC, et al. A novel, orally active LPA receptor antagonist inhibits lung fibrosis in the mouse bleomycin model. Br J Pharmacol. (2010) 160:1699–713. doi: 10.1111/j.1476-5381.2010.00828.x
147. Lee SC, Fujiwara Y, Liu J, Yue J, Shimizu Y, Norman DD, et al. Autotaxin, LPA receptors (1 and 5) exert disparate functions in tumor cells versus the host tissue microenvironment in melanoma invasion and metastasis. Mol Cancer Res. (2014) 13:174–85. doi: 10.1158/1541-7786.MCR-14-0263
148. Funke M, Zhao Z, Xu Y, Chun J, Tager AM. The lysophosphatidic acid receptor LPA1 promotes epithelial cell apoptosis after lung injury. Am J Res Cell Mol Biol. (2012) 46:355–64. doi: 10.1165/rcmb.2010-0155OC
149. Wynn TA. Integrating mechanisms of pulmonary fibrosis. J Exp Med. (2011) 208:1339–50. doi: 10.1084/jem.20110551
150. Oikonomou N, Harokopos V, Zalevsky J, Valavanis C, Kotanidou A, Szymkowski DE, et al. Soluble TNF mediates the transition from pulmonary inflammation to fibrosis. PLoS ONE (2006) 1:e108. doi: 10.1371/journal.pone.0000108
151. Magkrioti C, Aidinis V. ATX and LPA signalling in lung pathophysiology. World J Respirol. (2013) 3:77–103. doi: 10.5320/wjr.v3.i3.77
152. Cummings R, Zhao Y, Jacoby D, Spannhake EW, Ohba M, Garcia JG, et al. Protein kinase Cdelta mediates lysophosphatidic acid-induced NF-kappaB activation and interleukin-8 secretion in human bronchial epithelial cells. J Biol Chem. (2004) 279:41085–94. doi: 10.1074/jbc.M404045200
153. Saatian B, Zhao Y, He D, Georas SN, Watkins T, Spannhake EW, et al. Transcriptional regulation of lysophosphatidic acid-induced interleukin-8 expression and secretion by p38 MAPK and JNK in human bronchial epithelial cells. Biochem J. (2006) 393(Pt 3):657–68. doi: 10.1042/BJ20050791
154. Xu MY, Porte J, Knox AJ, Weinreb PH, Maher TM, Violette SM, et al. Lysophosphatidic acid induces alphavbeta6 integrin-mediated TGF-beta activation via the LPA2 receptor and the small G protein G alpha(q). Am J Pathol. (2009) 174:1264–79. doi: 10.2353/ajpath.2009.080160
155. Munger JS, Huang X, Kawakatsu H, Griffiths MJ, Dalton SL, Wu J, et al. The integrin alpha v beta 6 binds and activates latent TGF beta 1: a mechanism for regulating pulmonary inflammation and fibrosis. Cell (1999) 96:319–28. doi: 10.1016/S0092-8674(00)80545-0
156. Fernandez IE, Eickelberg O. The impact of TGF-beta on lung fibrosis: from targeting to biomarkers. Proc Am Thorac Soc. (2012) 9:111–6. doi: 10.1513/pats.201203-023AW
157. Ren A, Moon C, Zhang W, Sinha C, Yarlagadda S, Arora K, et al. Asymmetrical macromolecular complex formation of lysophosphatidic acid receptor 2 (LPA2) mediates gradient sensing in fibroblasts. J Biol Chem. (2014) 289:35757–69. doi: 10.1074/jbc.M114.595512
158. Gan X, Wang J, Wang C, Sommer E, Kozasa T, Srinivasula S, et al. PRR5L degradation promotes mTORC2-mediated PKC-delta phosphorylation and cell migration downstream of Galpha12. Nat Cell Biol. (2012) 14:686–96. doi: 10.1038/ncb2507
159. Berridge MJ, Bootman MD, Roderick HL. Calcium signalling: dynamics, homeostasis and remodelling. Nat Rev Mol Cell Biol. (2003) 4:517–29. doi: 10.1038/nrm1155
160. Janssen LJ, Mukherjee S, Ask K. Calcium homeostasis and ionic mechanisms in pulmonary fibroblasts. Am J Res Cell Mol Biol. (2015) 53:135–48. doi: 10.1165/rcmb.2014-0269TR
161. Kulkarni T, O'Reilly P, Antony VB, Gaggar A, Thannickal VJ. Matrix remodeling in pulmonary fibrosis and emphysema. Am J Res Cell Mol Biol. (2016) 54:751–60. doi: 10.1165/rcmb.2015-0166PS
162. Rahaman SO, Grove LM, Paruchuri S, Southern BD, Abraham S, Niese KA, et al. TRPV4 mediates myofibroblast differentiation and pulmonary fibrosis in mice. J Clin Invest. (2014) 124: 5225–38. doi: 10.1172/JCI75331
163. Meyer Zu Heringdorf D. Lysophospholipid receptor-dependent and -independent calcium signaling. J Cell Biochem. (2004) 92:937–48. doi: 10.1002/jcb.20107
164. Nieto-Posadas A, Picazo-Juarez G, Llorente I, Jara-Oseguera A, Morales-Lazaro S, Escalante-Alcalde D, et al. Lysophosphatidic acid directly activates TRPV1 through a C-terminal binding site. Nat Chem Biol. (2011) 8:78–85. doi: 10.1038/nchembio.712
165. Farkas L, Kolb M. Pulmonary microcirculation in interstitial lung disease. Proc Am Thorac Soc. (2011) 8:516–21. doi: 10.1513/pats.201101-007MW
166. Van Der Aar EM, Fagard L, Desrivot J, Dupont S, Heckmann B, Blanque R, et al. Favorable human safety, pharmacokinetics and pharmacodynamics of the autotaxin inhibitor GLPG1690, a potential new treatment in idiopathic pulmonary fibrosis. A103 IPF: More about therapy and outcomes. In: American Thoracic Society International Conference Abstracts: American Thoracic Society, San Francisco, CA (2016). p. A2701.
Keywords: autotaxin (ATX), lysophosphatidic acid (LPA), lysophosphatidic acid receptor (LPAR), g-proteins, pulmonary fibrosis
Citation: Ninou I, Magkrioti C and Aidinis V (2018) Autotaxin in Pathophysiology and Pulmonary Fibrosis. Front. Med. 5:180. doi: 10.3389/fmed.2018.00180
Received: 31 January 2018; Accepted: 25 May 2018;
Published: 13 June 2018.
Edited by:
Bethany B. Moore, University of Michigan, United StatesReviewed by:
Viswanathan Natarajan, University of Illinois at Chicago, United StatesSteve N. Georas, University of Rochester, United States
Copyright © 2018 Ninou, Magkrioti and Aidinis. This is an open-access article distributed under the terms of the Creative Commons Attribution License (CC BY). The use, distribution or reproduction in other forums is permitted, provided the original author(s) and the copyright owner are credited and that the original publication in this journal is cited, in accordance with accepted academic practice. No use, distribution or reproduction is permitted which does not comply with these terms.
*Correspondence: Vassilis Aidinis, di5haWRpbmlzQGZsZW1pbmcuZ3I=
†These authors have contributed equally to this work.