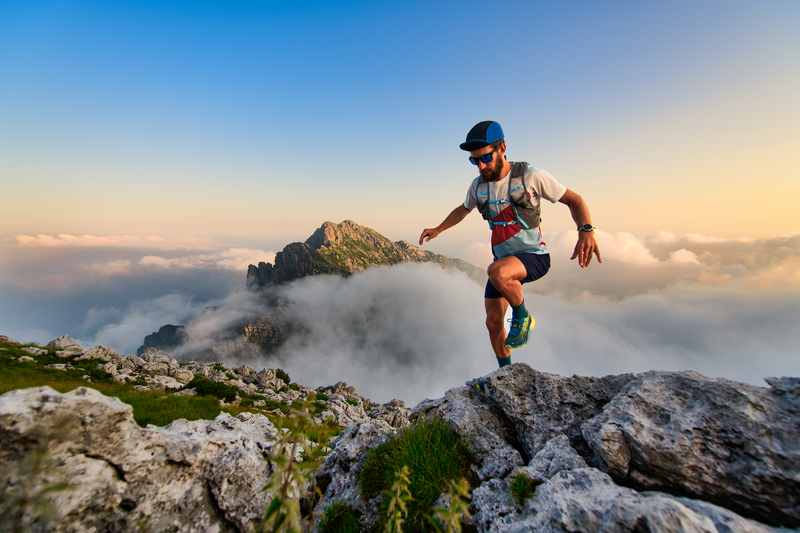
95% of researchers rate our articles as excellent or good
Learn more about the work of our research integrity team to safeguard the quality of each article we publish.
Find out more
PERSPECTIVE article
Front. Med. , 28 July 2015
Sec. Gastroenterology
Volume 2 - 2015 | https://doi.org/10.3389/fmed.2015.00048
A commentary has been posted on this article:
Commentary: A Hypothesis for Examining Skeletal Muscle Biopsy-Derived Sarcolemmal nNOSμ as Surrogate for Enteric nNOSα Function
The pathophysiology of gastrointestinal motility disorders is controversial and largely unresolved. This provokes empiric approaches to patient management of these so-called functional gastrointestinal disorders. Preliminary evidence demonstrates that defects in neuronal nitric oxide synthase (nNOS) expression and function, the enzyme that synthesizes nitric oxide (NO), the key inhibitory neurotransmitter mediating mechano-electrical smooth muscle relaxation, is the major pathophysiological basis for sluggishness of oro-aboral transit of luminal contents. This opinion is an ansatz of the potential of skeletal muscle biopsy and examining sarcolemmal nNOSμ to provide complementary insights regarding nNOSα expression, localization, and function within enteric nerve terminals, the site of stimulated de novo NO synthesis. The main basis of this thesis is twofold: (a) the molecular similarity of the structures of nNOS α and μ, similar mechanisms of localizations to “active zones” of nitrergic synthesis, and same mechanisms of electron transfers during NO synthesis and (b) pragmatic difficulty to routinely obtain full-thickness biopsies of gastrointestinal tract, even in patients presenting with the most recalcitrant manifestations of stasis and delayed transit of luminal contents. This opinion attempts to provoke dialog whether this approach is feasible as a surrogate to predict catalytic potential of nNOSα and defects in nitrergic neurotransmission. This discussion makes an assumption that similar molecular mechanisms of nNOS defects shall be operant in both the enteric nerve terminals and the skeletal muscles. These overlaps of skeletal and gastrointestinal dysfunction are largely unknown, thus meriting that the thesis be validated in future by proof-of-principle experiments.
Recent evidence of a novel C-terminal tail region mutation involving neuronal nitric oxide synthase (nNOS) as causative for global gastrointestinal achalasia has been reported in two probands of an Arab family (1). This study has highlighted the intrinsic shortcoming of detection of nNOS molecular pathologies (2), as the peripheral cultured fibroblasts from the patients (siblings) did not yield significant nNOS transcripts (1). The central pathophysiology of most gastrointestinal motility disorders remains unresolved, resulting in labeling of the conditions as “functional” or “idiopathic” (2–5). This remains at the root of current empiric approaches to the management of these conditions. Studies have corroborated the evidence that defects in nNOS expression and function, the enzyme that synthesizes nitric oxide (NO), the key inhibitory neurotransmitter manifesting mechano-electrical smooth muscle relaxation, underlies sluggishness of oro-aboral transit of luminal contents (1, 6–12). This opinion presents an argument of the potential of skeletal muscle biopsy and examining sarcolemmal nNOSμ to provide complementary insights regarding nNOSα expression, localization, and function within enteric nerve terminals, the site of stimulated de novo NO synthesis. The main basis of this argument is twofold: (a) the molecular similarity of the structures of nNOS α and μ, similar mechanisms of localizations to “active zones” of nitrergic synthesis, and same mechanisms of electron transfers during NO synthesis and (b) pragmatic difficulty to routinely obtain full-thickness biopsies of gastrointestinal tract, even in patients presenting with the most recalcitrant manifestations of stasis and delayed transit of luminal contents.
Nitric oxide synthesis involves oxidoreduction of the nitrogen atom of guanidino group (13) (Figure 1Ai). The electron transfer between the reductase to the oxidase domain of nNOS obviates that two molecules of nNOS need to be in close molecular proximity, as the electron transfer is intermolecular (2, 14). Thus, nNOS should exist as a dimer (6, 15–18) to be functionally active in nerve terminals (15, 16), neuronal soma (19), and other subcellular compartments that contain nNOS and enzymatically produce NO.
Figure 1. (A) (i) Key steps in nitric oxide synthesis by l-arginine oxidoreduction by electron flow between dimeric nNOS; (ii) cartoon depicting molecular architecture of the splice variants nNOSα and μ. nNOSα and μ are molecularly similar, with nNOSμ having an additional 34 amino-acid inserts between the calmodulin (CaM) and FMN domains. Note that nNOSμ has a PDZ domain-lacking isoform, similar to nNOSβ. (B) Striking peripheral distribution pattern of nNOSμ splice variants in skeletal muscle section. Note that, in contrast to the diffuse distribution of nNOSα in enteric nerve terminals and nNOSμ in cavernosal nerve terminals {depicted elsewhere, see (9, 28)}, nNOSμ in skeletal muscle shows a striking peripheral location lacing the boundary of the sarcolemma. Increasing evidence points toward membrane as the site of active nitrergic biosynthesis. Skeletal muscle biopsies may provide an excellent model for obtaining instant snapshot of membrane-localized nNOS splice forms. Note the membrane mislocalization of nNOS in some representative skeletal muscle diseases in the lower panels. Whether enteric nitrergic neurotransmission has affected these diseases has not been thoroughly examined, though dysphagia has been reported in association with LGMD. DMD, Duchenne muscular dystrophy; BMD, Becker muscular dystrophy; LGMD, limb-girdle muscular dystrophy [reproduced with permission from Ref. (20)]. (C) Reduction of membrane-bound nNOSμ in skeletal muscle and PIN (LC8) in an animal model of diabesity (Zucker fa/fa rat). Note that syntrophin expression remained unchanged in Zucker fa/fa rats, hinting that either genomic expression of nNOSμ or its intracellular transport by PIN/myosin Va or both may have contributed to the diminished membrane location of nNOSμ in diabesity. Myosin Va binding to nNOS has conserved mechanisms across tissues, utilizing PIN or LC8, the light chain of dynein and myosin Va. The transcription factors regulating myosin Va and nNOS genomic expression may be affected in diabetes. This may potentially effect nNOS distribution and localization in critical active zones within nerve terminals and impair enteric musculomotor neurotransmission. Skeletal muscle expression of nNOS, PIN, and potentially myosin Va may provide surrogate impression of changes of similar proteins in myenteric nerve terminals [images pseudocolored with ImageJ; reproduced with permission from Ref. (21)].
From a theoretical perspective, it may seem likely that dimeric nNOS can catalyze NO synthesis anywhere within the cell. Increasingly, however, signaling domains of nNOS is being recognized. Namely, NO is not synthesized stochastically anywhere within the cell, but rather occurs at discrete locations within the cell cortex underlying the membrane. There exists no direct evidence of detection of nitric oxide synthesis at the membrane. This is mainly because the synthesized nitric oxide, due to its very high diffusion coefficient, instantly saturates the reporter [like diaminofluorescein (DAF); DAF detects N2O3, the oxidized product of NO] (22). The rate kinetics of DAF⋅N2O3 formation is much faster than image acquisition frame rate by conventional microscopy like CLSM. DAF⋅N2O3 stains the entire membrane-delimited location and preclude detection of the precise site of synthesis. However, there are indirect evidences, which are strong evidentiary for the cell membrane as the site of nNOS enzymatic activity.
The main body of evidence comes from the intracellular localization site of nNOS. Light and electron micrographs of enteric and other nerve terminals have shown nNOS diffusely distributed in the cytoplasm as well as near the cell membrane (9, 23–26). In isolated enteric nerve terminals, nNOSα dimers have been demonstrated both within the cytosol and membrane-associated (16). However, preliminary evidence has shown that cytosolic nNOS dimers remain phosphorylated and enzymatically inactive (16). It has also been reported that specific motor proteins exist, like for example, myosin Va, which has the necessary molecular specificity for transposition of nNOS from the cytosol to the membrane (9, 27, 28). Much like the vesicular neurotransmitters, “active zones” exist for nNOS-mediated nitrergic synthesis at the membranes, though specializations like excitatory synapses are not seen (6, 16, 29). Cold SDS PAGE have revealed 320 kDa nNOS dimers that are both phosphorylated at serine847 and unphosphorylated forms, raising the possibility of a toggle between active and inactive isoforms that may initiate and terminate nitrergic neurotransmission (6, 15, 16).
Myosin Va facilitates transfer of melanosomes (30). Deficiency of myosin Va results in dilution of coat color (27, 31). Using an inbred mouse model of hypomorphic myosin Va mutant, the DBA/2J mice, it was shown that there is a reduction of membrane-bound nNOS within the enteric synaptosomes (27). Furthermore, KCl stimulation of plated DBA/2J varicosities failed to generate fluorescence product (27). These evidences suggested the existence and necessity for membrane localization for optimal nNOS activity in enteric nerve terminals.
The most likely reason seems to result from cooperative allostery that is needed for precision of regulation of nNOS catalysis (6). Because nNOS is a constitutive enzyme that is activated by calcium influx, it seems likely that molecular proximity of nNOS to N-type calcium channels within the enteric varicosity may ergonomically support nitrergic neurotransmission (6). In fact, membrane-restricted nNOS has been demonstrated in other non-neuronal and neuronal cells including cardiomyocytes and central nervous system neurons (32–35). There may be the need for integrating different signal transduction pathways and this is best optimized by its membrane location.
Neuronal nitric oxide synthase-α has a close splice variant, nNOSμ, which has been reported to be present in skeletal muscles and cavernosal nitrergic nerve terminals (14, 28, 36–40) (Figure 1Aii). nNOS α and μ isoforms are molecularly similar, with the μ isoform carrying an extra 34 amino-acid μ-exon insert (14, 36, 41–44). Importantly, the skeletal muscle is the only tissue where nNOS membrane localization is distinctly visualized and exhibits a striking appearance (20, 42, 45) (Figure 1B). Unlike all other cells in which nNOS appears diffusely, nNOS is located discretely under the submembranous zone, bordering the polygonal outline of skeletal muscle cross-sections, with scant staining in the cytosol. Dot blot assays and other studies confirmed these observations (36, 46). The functions of nNOS in skeletal muscles are diverse, including facilitation of arteriolar relaxation during skeletal muscle activity, muscular anaplerosis, and mitochondrial biogenesis (42, 43, 47).
Peripheral mislocalization of nNOS has been demonstrated in paraphysiologic conditions like hibernation and numerous primary and acquired skeletal muscle diseases, including age-related sarcopenia, ALS, chronic ventilation, long-term immobilization due to orthopedic cast, zero-gravity flight, Duchenne muscular dystrophy, Becker dystrophy, and myasthenia gravis (20, 45, 48–58) (Figure 1B). The function of membrane localization of nNOSμ is not entirely well defined (47), though restoration of membrane-bound nNOSμ has been used as an endpoint for the recovery of Duchenne muscular dystrophy after molecular therapy (59).
While this peripheral array of nNOSμ occurs in skeletal muscle due to dystrophin and syntrophin (60–63), almost nothing is known about nNOSμ transport in skeletal muscles. It may likely result from a discrete transcellular transport system and trapping of nNOS in the periphery. This unique cellular biology of nNOS costamere in the skeletal muscle makes it a unique model organ to test membrane distribution of nNOS (20, 21, 42, 45, 64). Thus, it may be posited that skeletal muscle punch biopsies may provide an optimal model to examine membrane-bound nNOS. Cryosections may be stained in the pathology gross laboratory settings to obtain instant visual snapshot of membrane distribution of nNOS. This may provide useful correlative evidence in refractory disorders of gastrointestinal motility. NADPH diaphorase examination of skeletal muscle biopsies of the affected probands in the recently described case report of achalasia (1) resulting from nNOSdel1203–1434 mutation shall tentatively yield negative staining, as well as impairment of nNOSμ enzymatic activity. Interestingly, this nNOSdel1203–1434 deletion mutant has an intact PDZ domain (1). Thus, membrane localization of nNOSμ would not be affected despite lack of NO biosynthesis by this membrane-localized nNOS (1, 2). The case report did not describe whether the affected children had any complaints of muscle fatigue, muscular ischemia, or metabolic problems like glucose intolerance (1). Examination of skeletal muscle nNOS would be one of the first steps toward identifying peripheral biomarker for gastrointestinal motility disorders resulting from defects in nitrergic neurotransmission.
The molecular transporters of nNOS within skeletal muscles have not been examined in depth. Preliminary evidence suggests that nNOSμ may be transported by myosin Va. Nitrergic relaxations are impaired in cavernosal tissues of DBA/2J mice (28). In cavernosal nerve fibers, nNOSμ and myosin Va localize (28). Thus, it is likely that nNOSμ in skeletal muscles may also be transported by myosin Va. Membrane clustering of nNOSμ and LC8 is diminished in gastrocnemius biopsies obtained from a rat model of diabesity (65) (Figure 1C). Recent observations of genomic suppression of myosin Va have been shown in streptozotocin-induced diabetes (9). It is possible that transcriptional inhibition of myosin Va is a fundamental early-stage molecular pathophysiology operant in all tissues in diabetes (9, 66), which likely contributes to the observed misalignment of membrane nNOS in skeletal muscles in the diabesity model. Myosin Va facilitates GLUT4-mediated uptake of glucose (67). Insulin-stimulated NO production stimulates glucose uptake in diverse tissues including skeletal muscles (68–70). The overall sluggishness of directed cytosolic movement of glucose transporters in skeletal muscle possibly contributes to insulin resistance, contributing to chronic pathophysiology of non-insulin-dependent diabetes mellitus (NIDDM). Diminished insulin sensitivity has been associated with reduced NOS function and impaired glucose uptake in T2DM skeletal muscle. It has been shown that nNOS undergoes phosphorylation in skeletal muscle in response to insulin and is associated with increased NO production (71). Myosin Va has been reported to cluster nicotinic acetylcholine receptors in skeletal muscle neuromuscular junctions, along with other clustering proteins like dystrophins (the classic NMJ, which is a fast synapse in contrast to the slow junction of enteric nerve terminal-smooth muscle junction) (72, 73). Whether subtle changes in nAChR due to myosin Va genotoxicity are contributory to fatigue in diabetes is a tempting hypothesis. It may be appreciated here that this opinion makes an assumption that similar molecular mechanisms of nNOS defects shall be operant in both the enteric nerve terminals and the skeletal muscles. These overlaps of skeletal and gastrointestinal dysfunction are virtually not known, thus meriting that the thesis be validated in future by proof-of-principle experiments. For example, recent pilot evidence has been provided that type I fibers of myalgic muscle is associated with mislocalization of membrane-bound nNOSμ (74). Whether there is an increased predisposition to gastrointestinal dysmotility in cohorts of subjects with restless legs syndrome is an avenue of significant translational investigation.
Though the promoter and exons of nNOS have been examined in detail (41, 75, 76), virtually nothing is known regarding the underlying basis of the differential splicing (6, 15, 16, 77). nNOSα is present in neuronal cells, including myenteric neurons (6, 15, 16, 27, 77), though it is present in non-neuronal but excitable cells like the cardiomyocyte (34, 35). Similarly, it is also not known why nNOSμ is seen in cavernosal nerve fibers (28), as well as extensive distribution throughout the skeletal muscles (42). Some recent results show the differential calmodulin affinity of these two isoforms, with nNOSμ relying less on calmodulin for electron transfer, as well as with lower rates of electron flow in the reductase domain (though similar potentials of NO synthesis). How these differences relate to the tissue distribution and tissue-specific function is not known at present. The jury is still out regarding the precise need for membrane-localization of nNOS in skeletal muscles (47). However, this opinion has possibly convincingly argued that introduction of skeletal muscle biopsy for nNOS examination is potentially an important step in the neurogastroenterology clinic. Subtle differences do exist between nNOS α and μ. Apart from the extra amino-acid inserts, crude-solubilized muscle extracts have shown that nNOSμPDZ− is present in the pellet fraction, though further studies are needed to distinguish the distribution in the sarcolemma per se from the associated subcellular cytoskeleton (36). The role of nNOSμPDZ− has been examined in a few other studies (46). It is possible that because of submembranous location of nuclei in skeletal muscles, this isoform is detected near the membranes but may translocate to the cytosol post-synthesis. This hypothesis remains to be tested. It is not known whether the nNOSμPDZ+–nNOSμPDZ− heterodimer exists in skeletal muscles, though theoretically such a heterodimer has the potential to synthesize NO, as the oxidase domain exists beyond AA409, well beyond the N-terminal PDZ domain. nNOSβ, the isoform similar to nNOSμPDZ− is present in cytosolic fraction of enteric nerve terminals and remains serine847-phosphorylated, but absent in purified membrane fractions (15, 16, 77). Whether nNOSα/β heterodimers are formed in enteric nerve terminals have not been examined.
Though the stoichiometry of gene expression and protein translation of myenteric nNOSα and skeletal muscle nNOSμ may not match, examination of skeletal muscle nNOSμ from biopsy samples has the potential to provide three important information: (a) microscopic visualization shall provide the location site of nNOS, including at the membrane, the active site of nitrergic neurotransmission; (b) nNOS may be extracted and purified for in vivo mutational analyses; (c) differential transcriptional and post-transcriptional regulations of myosin Va and nNOS, both in terms of the specific factors involved and the temporal relationships. Given the pragmatic problem of obtaining regional and full-thickness biopsies of the gut, examination of skeletal muscle biopsies might provide extremely useful information relating to pathophysiology of enteric nitrergic neurotransmission. Despite the invasive nature of the proposed biopsies, endeavor may be directed to obtain primary clinical evidence to obtain precision in the management of gastrointestinal motility disorders.
The author declares that the research was conducted in the absence of any commercial or financial relationships that could be construed as a potential conflict of interest.
1. Shteyer E, Edvardson S, Wynia-Smith SL, Pierri CL, Zangen T, Hashavya S, et al. Truncating mutation in the nitric oxide synthase 1 gene is associated with infantile achalasia. Gastroenterology (2015) 148:533–6. doi:10.1053/j.gastro.2014.11.044
2. Chaudhury A. Tail tale: nNOSdel1203-1434 predicts global defects in esophagogastrointestinal transit. Gastroenterology (2015) 149(1):260–1. doi:10.1053/j.gastro.2015.03.054
3. Chaudhury A. 2D DIGE does not reveal all: a scotopic report suggests differential expression of a “Calponin family member” protein for tetany of sphincters! Front Med (2015) 2:42. doi:10.3389/fmed.2015.00042
4. Koch KL. Gastroparesis and neuromuscular disorders of the stomach. Minerva Gastroenterol Dietol (2003) 49:107–22.
5. Schäppi MG, Staiano A, Milla PJ, Smith VV, Dias JA, Heuschkel R, et al. A practical guide for the diagnosis of primary enteric nervous system disorders. J Pediatr Gastroenterol Nutr (2013) 57:677–86. doi:10.1097/MPG.0b013e3182a8bb50
6. Chaudhury A. Molecular handoffs in nitrergic neurotransmission. Front Med (2014) 1:8. doi:10.3389/fmed.2014.00008
7. Mitolo-Chieppa D, Mansi G, Rinaldi R, Montagnani M, Potenza MA, Genualdo M, et al. Cholinergic stimulation and nonadrenergic, noncholinergic relaxation of human colonic circular muscle in idiopathic chronic constipation. Dig Dis Sci (1998) 43:2719–26. doi:10.1023/A:1026615730533
8. Takahashi T. Pathophysiological significance of neuronal nitric oxide synthase in the gastrointestinal tract. J Gastroenterol (2003) 38:421–30. doi:10.1007/s00535-003-1094-y
9. Chaudhury A, De Miranda-Neto MH, Pereira RV, Zanoni JN. Myosin Va but not nNOSα is significantly reduced in jejunal musculomotor nerve terminals in diabetes mellitus. Front Med (2014) 1:17. doi:10.3389/fmed.2014.00017
10. Tomita R. Are there any functional differences of the enteric nervous system between the right-sided diverticular colon and the left-sided diverticular colon? An in vitro study. Int J Colorectal Dis (2014) 29:571–7. doi:10.1007/s00384-014-1837-7
11. Lefebvre RA, Smits GJ. Investigation of the influence of pregnancy on the role of nitric oxide in gastric emptying and non-adrenergic non-cholinergic relaxation in the rat. Naunyn Schmiedebergs Arch Pharmacol (1998) 357:671–6. doi:10.1007/PL00005223
12. Chaudhury A, Shariff A, Srinivas M, Sabherwal U. Changes in nitrergic innervation of defunctionalized rat colon after diversion colostomy. Neurogastroenterol Motil (2004) 16:475–87. doi:10.1111/j.1365-2982.2004.00565.x
13. Tierney DL, Huang H, Martasek P, Masters BS, Silverman RB, Hoffman BM. ENDOR spectroscopic evidence for the position and structure of NG-hydroxy-l-arginine bound to holo-neuronal nitric oxide synthase. Biochemistry (1999) 38:3704–10. doi:10.1021/bi982904r
14. Alderton WK, Cooper CE, Knowles RG. Nitric oxide synthases: structure, function and inhibition. Biochem J (2001) 357:593–615. doi:10.1042/0264-6021:3570593
15. Rao YM, Chaudhury A, Goyal RK. Active and inactive pools of nNOS in the nerve terminals in mouse gut: implications for nitrergic neurotransmission. Am J Physiol Gastrointest Liver Physiol (2008) 294:G627–34. doi:10.1152/ajpgi.00519.2007
16. Chaudhury A, He XD, Goyal RK. Role of PSD95 in membrane association and catalytic activity of nNOSalpha in nitrergic varicosities in mice gut. Am J Physiol Gastrointest Liver Physiol (2009) 297:G806–13. doi:10.1152/ajpgi.00279.2009
17. Gangula PR, Maner WL, Micci MA, Garfield RE, Pasricha PJ. Diabetes induces sex-dependent changes in neuronal nitric oxide synthase dimerization and function in the rat gastric antrum. Am J Physiol Gastrointest Liver Physiol (2007) 292:G725–33. doi:10.1152/ajpgi.00406.2006
18. Showkat Ali M, Tiscareno-Grejada I, Locovei S, Smiley R, Collins T, Sarosiek J, et al. Gender and estradiol as major factors in the expression and dimerization of nNOSα in rats with experimental diabetic gastroparesis. Dig Dis Sci (2012) 57:2814–25. doi:10.1007/s10620-012-2230-4
19. Rivera LR, Poole DP, Thacker M, Furness JB. The involvement of nitric oxide synthase neurons in enteric neuropathies. Neurogastroenterol Motil (2011) 23:980–8. doi:10.1111/j.1365-2982.2011.01780.x
20. Kobayashi YM, Rader EP, Crawford RW, Iyengar NK, Thedens DR, Faulkner JA, et al. Sarcolemma-localized nNOS is required to maintain activity after mild exercise. Nature (2008) 456:511–5. doi:10.1038/nature07414
21. Mezghenna K, Leroy J, Azay-Milhau J, Tousch D, Castex F, Gervais S, et al. Counteracting neuronal nitric oxide synthase proteasomal degradation improves glucose transport in insulin-resistant skeletal muscle from Zucker fa/fa rats. Diabetologia (2014) 57:177–86. doi:10.1007/s00125-013-3084-9
22. Chaudhury A. Evidence for dual pathway for nitrergic neuromuscular transmission in doubt: evidence favors lack of role of ICC. Gastroenterology (2013) 145:1160–1. doi:10.1053/j.gastro.2013.09.039
23. Rothe F, Canzler U, Wolf G. Subcellular localization of the neuronal isoform of nitric oxide synthase in the rat brain: a critical evaluation. Neuroscience (1998) 83:259–69. doi:10.1016/S0306-4522(97)00373-4
24. Belai A, Schmidt HHHW, Hoyle CHV, Hassall CJS, Saffrey MJ, Moss J, et al. Colocalization of nitric oxide synthase and NADPH-diaphorase in the myenteric plexus of the rat gut. Neurosci Lett (1992) 143:60–4. doi:10.1016/0304-3940(92)90233-W
25. Llewellyn-Smith IJ, Song Z-M, Costa M, Bredt DS, Snyder SH. Ultrastructural localization of nitric oxide synthase immunoreactivity in guinea-pig enteric neurons. Brain Res (1992) 577:337–42. doi:10.1016/0006-8993(92)90294-J
26. Schmidt HHHW, Gagne GD, Nakane M, Pollock JS, Miller M, Murad F. Mapping of neural nitric oxide synthase in the rat suggests frequent co-localization with NADPH diaphorase but not with soluble guanylyl cyclase, and novel paraneural functions for nitrinergic signal transduction. J Histochem Cytochem (1992) 40:1439–56. doi:10.1177/40.10.1382087
27. Chaudhury A, He XD, Goyal RK. Myosin Va plays a key role in nitrergic neurotransmission by transporting nNOSα to enteric varicosity membrane. Am J Physiol Gastrointest Liver Physiol (2011) 301:G498–507. doi:10.1152/ajpgi.00164.2011
28. Chaudhury A, Cristofaro V, Carew JA, Goyal RK, Sullivan MP. Myosin Va plays a role in nitrergic smooth muscle relaxation in gastric fundus and corpora cavernosa of penis. PLoS One (2014) 9:e86778. doi:10.1371/journal.pone.0086778
29. Goyal RK, Chaudhury A. Structure activity relationship of synaptic and junctional neurotransmission. Auton Neurosci (2013) 176:11–31. doi:10.1016/j.autneu.2013.02.012
30. Boissy RE. Melanosome transfer to and translocation in the keratinocyte. Exp Dermatol (2003) 12:5–12. doi:10.1034/j.1600-0625.12.s2.1.x
31. Huang JD, Mermall V, Strobel MC, Russell LB, Mooseker MS, Copeland NG, et al. Molecular genetic dissection of mouse unconventional myosin-VA: tail region mutations. Genetics (1998) 148:1963–72.
32. Marques-da-Silva D, Gutierrez-Merino C. Caveolin-rich lipid rafts of the plasma membrane of mature cerebellar granule neurons are microcompartments for calcium/reactive oxygen and nitrogen species cross-talk signaling. Cell Calcium (2014) 56:108–23. doi:10.1016/j.ceca.2014.06.002
33. El-Mlili N, Rodrigo R, Naghizadeh B, Cauli O, Felipo V. Chronic hyperammonemia reduces the activity of neuronal nitric oxide synthase in cerebellum by altering its localization and increasing its phosphorylation by calcium-calmodulin kinase II. J Neurochem (2008) 106:1440–9. doi:10.1111/j.1471-4159.2008.05495.x
34. Cartwright EJ, Oceandy D, Neyses L. Physiological implications of the interaction between the plasma membrane calcium pump and nNOS. Pflugers Arch (2009) 457:665–71. doi:10.1007/s00424-008-0455-z
35. Mohamed TM, Oceandy D, Zi M, Prehar S, Alatwi N, Wang Y, et al. Plasma membrane calcium pump (PMCA4)-neuronal nitric-oxide synthase complex regulates cardiac contractility through modulation of a compartmentalized cyclic nucleotide microdomain. J Biol Chem (2011) 286:41520–9. doi:10.1074/jbc.M111.290411
36. Baum O, Schläppi S, Huber-Abel FA, Weichert A, Hoppeler H, Zakrzewicz A. The beta-isoform of neuronal nitric oxide synthase (nNOS) lacking the PDZ domain is localized at the sarcolemma. FEBS Lett (2011) 585(20):3219–23. doi:10.1016/j.febslet.2011.09.016
37. Villanueva C, Giulivi C. Subcellular and cellular locations of nitric oxide synthase isoforms as determinants of health and disease. Free Radic Biol Med (2010) 49:307–16. doi:10.1016/j.freeradbiomed.2010.04.004
38. Magee T, Fuentes AM, Garban H, Rajavashisth T, Marquez D, Rodriguez JA, et al. Cloning of a novel neuronal nitric oxide synthase expressed in penis and lower urinary tract. Biochem Biophys Res Commun (1996) 226:145–51. doi:10.1006/bbrc.1996.1324
39. Ignarro LJ, Bush PA, Buga GM, Wood KS, Fukuto JM, et al. Nitric oxide and cyclic GMP formation upon electrical field stimulation cause relaxation of corpus cavernosum smooth muscle. Biochem Biophys Commun (1990) 170:843–50.
40. Gonzalez-Cadavid NF, Burnett AL, Magee TR, Zeller CB, Vernet D, Smith N, et al. Expression of penile neuronal nitric oxide synthase variants in the rat and mouse penile nerves. Biol Reprod (2000) 63:704–14. doi:10.1095/biolreprod63.3.704
41. Huber A, Saur D, Kurjak M, Schusdziarra V, Allescher HD. Characterization and splice variants of neuronal nitric oxide synthase in rat small intestine. Am J Physiol (1998) 275:G1146–56.
42. Stamler JS, Meissner G. Physiology of nitric oxide in skeletal muscle. Physiol Rev (2001) 81:209–37.
43. Baldelli S, Lettieri Barbato D, Tatulli G, Aquilano K, Ciriolo MR. The role of nNOS and PGC-1α in skeletal muscle cells. J Cell Sci (2014) 127:4813–20. doi:10.1242/jcs.154229
44. Silvagno F, Xia H, Bredt DS. Neuronal nitric-oxide synthase-mu, an alternatively spliced isoform expressed in differentiated skeletal muscle. J Biol Chem (1996) 271:11204–8. doi:10.1074/jbc.271.19.11204
45. Finanger Hedderick EL, Simmers JL, Soleimani A, Andres-Mateos E, Marx R, Files DC, et al. Loss of sarcolemmal nNOS is common in acquired and inherited neuromuscular disorders. Neurology (2011) 76:960–7. doi:10.1212/WNL.0b013e31821043c8
46. Percival JM, Anderson KN, Huang P, Adams ME, Froehner SC. Golgi and sarcolemmal neuronal NOS differentially regulate contraction-induced fatigue and vasoconstriction in exercising mouse skeletal muscle. J Clin Invest (2010) 120:816–26. doi:10.1172/JCI40736
47. Tidball JG, Wehling-Henricks M. Nitric oxide synthase deficiency and the pathophysiology of muscular dystrophy. J Physiol (2014) 592:4627–38. doi:10.1113/jphysiol.2014.274878
48. Suzuki N, Mizuno H, Warita H, Takeda S, Itoyama Y, Aoki M. Neuronal NOS is dislocated during muscle atrophy in amyotrophic lateral sclerosis. J Neurol Sci (2010) 294:95–101. doi:10.1016/j.jns.2010.03.022
49. Percival JM, Anderson KN, Gregorevic P, Chamberlain JS, Froehner SC. Functional deficits in nNOSmu-deficient skeletal muscle: myopathy in nNOS knockout mice. PLoS One (2008) 3:e3387. doi:10.1371/journal.pone.0003387
50. Li D, Yue Y, Lai Y, Hakim CH, Duan D. Nitrosative stress elicited by nNOSμ delocalization inhibits muscle force in dystrophin-null mice. J Pathol (2011) 223:88–98. doi:10.1002/path.2799
51. Gentil C, Leturcq F, Ben Yaou R, Kaplan JC, Laforet P, Penisson-Besnier I, et al. Variable phenotype of del45–55 Becker patients correlated with nNOSμ mislocalization and RYR1 hypernitrosylation. Hum Mol Genet (2012) 21:3449–60. doi:10.1093/hmg/dds176
52. Cordani N, Pisa V, Pozzi L, Sciorati C, Clementi E. Nitric oxide controls fat deposition in dystrophic skeletal muscle by regulating fibro-adipogenic precursor differentiation. Stem Cells (2014) 32:874–85. doi:10.1002/stem.1587
53. Anderson JE. A role for nitric oxide in muscle repair: nitric oxide-mediated activation of muscle satellite cells. Mol Biol Cell (2000) 11:1859–74. doi:10.1091/mbc.11.5.1859
54. Meinen S, Lin S, Rüegg MA, Punga AR. Fatigue and muscle atrophy in a mouse model of myasthenia gravis is paralleled by loss of sarcolemmal nNOS. PLoS One (2012) 7:e44148. doi:10.1371/journal.pone.0044148
55. Samengo G, Avik A, Fedor B, Whittaker D, Myung KH, Wehling-Henricks M, et al. Age-related loss of nitric oxide synthase in skeletal muscle causes reductions in calpain S-nitrosylation that increase myofibril degradation and sarcopenia. Aging Cell (2012) 11:1036–45. doi:10.1111/acel.12003
56. Sander M, Chavoshan B, Harris SA, Iannaccone ST, Stull JT, Thomas GD, et al. Functional muscle ischemia in neuronal nitric oxide synthase-deficient skeletal muscle of children with Duchenne muscular dystrophy. Proc Natl Acad Sci U S A (2000) 97:13818–23. doi:10.1073/pnas.250379497
57. Xu R, Andres-Mateos E, Mejias R, MacDonald EM, Leinwand LA, Merriman DK, et al. Hibernating squirrel muscle activates the endurance exercise pathway despite prolonged immobilization. Exp Neurol (2013) 247:392–401. doi:10.1016/j.expneurol.2013.01.005
58. Wells KE, Torelli S, Lu Q, Brown SC, Partridge T, Muntoni F, et al. Relocalization of neuronal nitric oxide synthase (nNOS) as a marker for complete restoration of the dystrophin associated protein complex in skeletal muscle. Neuromuscul Disord (2003) 13:21–31. doi:10.1016/S0960-8966(02)00191-8
59. Giudice E, Molza AE, Laurin Y, Nicolas A, Le Rumeur E, Delalande O. Molecular clues about the dystrophin-neuronal nitric oxide synthase interaction: a theoretical approach. Biochemistry (2013) 52:7777–84. doi:10.1021/bi400794p
60. Zhang Y, Duan D. Novel mini-dystrophin gene dual adeno-associated virus vectors restore neuronal nitric oxide synthase expression at the sarcolemma. Hum Gene Ther (2012) 23:98–103. doi:10.1089/hum.2011.131
61. Kameya S, Miyagoe Y, Nonaka I, Ikemoto T, Hanaoka K, Nabeshima Y, et al. A1-syntrophin gene disruption results in the absence of neuronal-type nitric-oxide synthase at the sarcolemma but does not induce muscle degeneration. J Biol Chem (1999) 274:2193–200. doi:10.1074/jbc.274.4.2193
62. Brenman JE, Chao DS, Gee SH, McGee AW, Craven SE, Santillano DR, et al. Interaction of nitric oxide synthase with the postsynaptic density protein PSD-95 and alpha1-syntrophin mediated by PDZ domains. Cell (1996) 84:757–67. doi:10.1016/S0092-8674(00)81053-3
63. Estrella NL, Naya FJ. Transcriptional networks regulating the costamere, sarcomere, and other cytoskeletal structures in striated muscle. Cell Mol Life Sci (2014) 71:1641–56. doi:10.1007/s00018-013-1512-0
64. Baum O, Planitzer G, Richter H, Gossrau R. Irregular costameres represent nitric oxide synthase-1-positive sarcolemma invaginations enriched in contracted skeletal muscle fibres. Histochem J (2000) 32:743–51. doi:10.1023/A:1004153111532
65. Chaudhury A. Similarity in transcytosis of nNOSα in enteric nerve terminals and beta cells of pancreatic islet. Front Med (2014) 1:20. doi:10.3389/fmed.2014.00020
66. Klip A, Sun Y, Chiu TT, Foley KP. Signal transduction meets vesicle traffic: the software and hardware of GLUT4 translocation. Am J Physiol Cell Physiol (2014) 306:C879–86. doi:10.1152/ajpcell.00069.2014
67. Lira VA, Soltow QA, Long JH, Betters JL, Sellman JE, Criswell DS. Nitric oxide increases GLUT4 expression and regulates AMPK signaling in skeletal muscle. Am J Physiol Endocrinol Metab (2007) 293(4):E1062–8. doi:10.1152/ajpendo.00045.2007
68. de Castro Barbosa T, Jiang LQ, Zierath JR, Nunes MTL-. Arginine enhances glucose and lipid metabolism in rat L6 myotubes via the NO/c-GMP pathway. Metabolism (2013) 62:79–89. doi:10.1016/j.metabol.2012.06.011
69. Kraus RM, Houmard JA, Kraus WE, Tanner CJ, Pierce JR, Choi MD, et al. Obesity, insulin resistance, and skeletal muscle nitric oxide synthase. J Appl Physiol (1985) 2012(113):758–65.
70. Hinchee-Rodriguez K, Garg N, Venkatakrishnan P, Roman MG, Adamo ML, Masters BS, et al. Neuronal nitric oxide synthase is phosphorylated in response to insulin stimulation in skeletal muscle. Biochem Biophys Res Commun (2013) 435:501–5. doi:10.1016/j.bbrc.2013.05.020
71. Röder IV, Choi KR, Reischl M, Petersen Y, Diefenbacher ME, Zaccolo M, et al. Myosin Va cooperates with PKA RIalpha to mediate maintenance of the endplate in vivo. Proc Natl Acad Sci U S A (2010) 107:2031–6. doi:10.1073/pnas.0914087107
72. Adams ME, Anderson KN, Froehner SC. The alpha-syntrophin PH and PDZ domains scaffold acetylcholine receptors, utrophin, and neuronal nitric oxide synthase at the neuromuscular junction. J Neurosci (2010) 30:11004–10. doi:10.1523/JNEUROSCI.1930-10.2010
73. Saur D, Seidler B, Paehge H, Schusdziarra V, Allescher HD. Complex regulation of human neuronal nitric-oxide synthase exon 1c gene transcription. Essential role of Sp and ZNF family members of transcription factors. J Biol Chem (2002) 277:25798–814. doi:10.1074/jbc.M109802200
74. Jensen L, Andersen LL, Schrøder HD, Frandsen U, Sjøgaard G. Neuronal nitric oxide synthase is dislocated in type I fibers of myalgic muscle but can recover with physical exercise training. Biomed Res Int (2015) 2015:265278. doi:10.1155/2015/265278
75. Nagl F, Schönhofer K, Seidler B, Mages J, Allescher HD, Schmid RM, et al. Retinoic acid-induced nNOS expression depends on a novel PI3K/Akt/DAX1 pathway in human TGW-nu-I neuroblastoma cells. Am J Physiol Cell Physiol (2009) 297:C1146–56. doi:10.1152/ajpcell.00034.2009
76. Saur D, Paehge H, Schusdziarra V, Allescher HD. Distinct expression of splice variants of neuronal nitric oxide synthase in the human gastrointestinal tract. Gastroenterology (2000) 118:849–58. doi:10.1016/S0016-5085(00)70171-5
Keywords: biomarker, neurotransmission, nNOS splice variants, biopsy, idiopathic
Citation: Chaudhury A (2015) A hypothesis for examining skeletal muscle biopsy-derived sarcolemmal nNOSμ as surrogate for enteric nNOSα function. Front. Med. 2:48. doi: 10.3389/fmed.2015.00048
Received: 18 May 2015; Accepted: 10 July 2015;
Published: 28 July 2015
Edited by:
Akira Andoh, Shiga University of Medical Science, JapanReviewed by:
Yuji Naito, Kyoto Prefectural University of Medicine, JapanCopyright: © 2015 Chaudhury. This is an open-access article distributed under the terms of the Creative Commons Attribution License (CC BY). The use, distribution or reproduction in other forums is permitted, provided the original author(s) or licensor are credited and that the original publication in this journal is cited, in accordance with accepted academic practice. No use, distribution or reproduction is permitted which does not comply with these terms.
*Correspondence: Arun Chaudhury, GIM Foundation, P. O. Box 250099, Little Rock, AR 72225, USA,Z2ltLmFjaGF1ZGh1cnlAZ21haWwuY29t
Disclaimer: All claims expressed in this article are solely those of the authors and do not necessarily represent those of their affiliated organizations, or those of the publisher, the editors and the reviewers. Any product that may be evaluated in this article or claim that may be made by its manufacturer is not guaranteed or endorsed by the publisher.
Research integrity at Frontiers
Learn more about the work of our research integrity team to safeguard the quality of each article we publish.