- 1Department of Medicine and Surgery, Kore University of Enna, Enna, Italy
- 2Institute for the Electromagnetic Sensing of the Environment, National Research Council of Italy, Naples, Italy
- 3Department of Radiology, IRCCS San Raffaele Scientific Institute, Milan, Italy
- 4Department of Radiology, IRCCS Istituto Ortopedico Galeazzi, Milan, Italy
- 5Department of Biomedical Sciences for Health, University of Milan, Milan, Italy
- 6Department of Radiology, Zucchi Clinical Institutes, Monza, Italy
Sarcopenia is a prevalent condition with significant clinical implications, and it is expected to escalate globally, demanding for effective diagnostic strategies, possibly at an early stage of the disease. Imaging techniques play a pivotal role in comprehensively evaluating sarcopenia, offering insights into both muscle quantity and quality. Among all the imaging techniques currently used for the diagnosis and follow up of sarcopenia, it is possible to distinguish two classes: Rx based techniques, using ionizing radiations, and non-invasive techniques, which are based on the use of safe and low risk diagnostic procedures. Dual-energy x-ray Absorptiometry and Computed Tomography, while widely utilized, entail radiation exposure concerns. Ultrasound imaging offers portability, real-time imaging, and absence of ionizing radiation, making it a promising tool Magnetic Resonance Imaging, particularly T1-weighted and Dixon sequences, provides cross- sectional and high-resolution images and fat-water separation capabilities, facilitating precise sarcopenia quantification. Bioelectrical Impedance Analysis (BIA), a non-invasive technique, estimates body composition, including muscle mass, albeit influenced by hydration status. Standardized protocols, such as those proposed by the Sarcopenia through Ultrasound (SARCUS) Working Group, are imperative for ensuring consistency across assessments. Future research should focus on refining these techniques and harnessing the potential of radiomics and artificial intelligence to enhance diagnostic accuracy and prognostic capabilities in sarcopenia.
1 Introduction
Sarcopenia is recognized as a prevalent and clinically relevant condition, particularly in aging populations (1). Depending on the criteria used for diagnosis and the screened population, prevalence rates range from 0.2% to 86.5% (2). Research findings from systematic reviews highlight variations in prevalence, with higher rates observed among individuals residing in long-term residential care settings (14%–33%) compared to those leading an active lifestyle (1%–29%) (1). Projections indicate a substantial increase in sarcopenia's worldwide prevalence, expected to rise from 50 million individuals in 2010 to approximately 200 million by 2050 (3). In addition to muscle weakness and loss of muscle mass, sarcopenia may manifest with various clinical symptoms affecting overall physical function and quality of life. These symptoms can include reduced mobility, balance problems, increased risk of falls and fractures, difficulty performing activities of daily living, and an overall decline in functional independence (4, 5). Sarcopenia can also contribute to metabolic changes, such as insulin resistance, and may exacerbate existing health conditions like diabetes, cardiovascular disease, and obesity (6). Sarcopenia arises from a complex interplay of factors involving both age-related physiological changes and various pathological mechanisms (7). The etiopathogenesis of sarcopenia is multifactorial and involves alterations in muscle protein turnover, hormonal changes (such as reduced levels of testosterone, growth hormone, and insulin-like growth factor-1, chronic inflammation, oxidative stress, mitochondrial dysfunction, and neuronal deficits (8–10). These factors contribute to an imbalance between muscle protein synthesis and degradation, ultimately leading to muscle wasting and weakness (11). Physiologically, sarcopenia is characterized by structural and functional changes within skeletal muscle, including fiber atrophy, decreased muscle fiber number and size, increased intramuscular fat deposition, impaired muscle contractility, and reduced muscle metabolic activity (12, 13). Histologically, sarcopenia is associated with alterations in muscle fiber composition, such as a shift from type II (fast-twitch) to type I (slow-twitch) muscle fibers, as well as increased fibrosis and infiltration of adipose tissue within muscle (14, 15). Biochemically, sarcopenia is marked by dysregulation of various signaling pathways involved in muscle protein synthesis and degradation, including the mTOR (mechanistic target of rapamycin) pathway, the ubiquitin-proteasome system, and autophagy (16–18). Although the diagnosis of sarcopenia is currently mainly performed via clinical examination, evaluating parameters like muscle strength and muscle mass, imaging diagnostic techniques can play a key role in the comprehensive evaluation of sarcopenia, providing valuable insights into both the quantitative and qualitative aspects of muscle structure, as well as providing information for the early detection of the disease (19, 20). Imaging techniques can be gathered into two main categories: invasive and non-invasive ones. The first category includes techniques and procedures that pose some risks for patients and clinical operators. The main representatives of this class of imaging techniques are those using ionizing radiations (x-rays) as a means to inspect the human body. Among the techniques used in sarcopenia evaluation, dual-energy x-ray Absorptiometry (DEXA) employs x-ray beams to measure bone density and soft tissue composition, including muscle mass (21). However, it cannot quantify intramuscular adipose, impacting muscle quality estimation, and factors like body thickness and hydration status can influence results. DEXA may overestimate muscle mass in cases of fluid accumulation and may provide inaccurate assessments of fat and muscle mass in obese individuals (22). Computed Tomography (CT) is also commonly employed in both oncological and non-oncological diagnostic settings and is increasingly utilized for screening sarcopenia due to its efficacy in assessing muscle mass and quality. CT is considered a gold standard for body composition analysis, particularly in nutritionally vulnerable patients (23). Its prevalence in medical practice is rising, with increased usage projected globally. CT's versatility and increased usage are attributed to its effectiveness in diagnosing various acute and chronic illnesses associated with aging. However, a significant drawback of this technique is radiation exposure, limiting its role as a screening tool for sarcopenia (24). Non-invasive imaging technologies, on the other hand, are based on the use of non-ionizing radiations, which can be safely adopted, even for repeated examinations, without increasing the carcinogenic risk to patients. This work aims to explore the significance of non-radiation techniques in the quantification of sarcopenia, shedding light on their unique capabilities, advantages, and potential applications in advancing our understanding of musculoskeletal health in aging populations. In particular, ultrasounds, magnetic resonance imaging (MRI)-based techniques, and bio-impedance assessment (BIA) will be described in detail, and a critical review of their use and application in sarcopenia diagnosis and evaluation will be reported.
2 Ultrasounds
2.1 Principles of ultrasound imaging in sarcopenia assessment
Ultrasound (US) imaging utilizes high-frequency sound waves to produce real-time images of soft tissues, including muscles. In the context of sarcopenia assessment, US involves the measurement of muscle size, architecture, and composition. The procedure typically involves positioning the subject in a supine position with legs fully extended. A linear probe, usually operating at frequencies ranging from 5 to 12 MHz, is commonly employed, with the anterior compartment of the thigh being one of the most frequently targeted sites (25). The imaging process involves the transmission of sound waves into the body, which then bounce back (echo) from the tissues, producing detailed images that can be analyzed for quantitative and qualitative assessments of muscle health and integrity.
2.2 Quantitative parameters
Muscle size is typically quantified by assessing muscle thickness (MT) and cross-sectional area (CSA), which have demonstrated strong correlations with other primary methods for studying muscle quantity, such as MRI, CT, and DXA (26, 27). MT refers to the depth or thickness of a muscle (28). In individuals with sarcopenia, muscle thickness may decrease due to the loss of muscle fibers and atrophy of existing muscle tissue (29). This reduction in muscle thickness can be observed in various muscle groups throughout the body, including the quadriceps, biceps, and calf muscles (30). CSA, in the context of muscle physiology, refers to the area of a muscle when viewed in a cross-section (31). It provides valuable information about the size and mass of the muscle. In sarcopenia, a reduction in CSA is linked to the decline in muscle strength and physical performance (32). Qualitative descriptions of muscle characteristics include parameters such as echo intensity (EI), pennation angle (PA), physiological cross-sectional area (PCSA), and fascicle length (FL) (33) (Table 1). EI is used to assess the quality or composition of tissues, including muscles. It refers to the brightness or darkness of the echoes produced by tissues in response to ultrasound waves (34). Higher EI values typically indicate greater amounts of intramuscular fat or fibrosis, whereas lower values suggest healthier muscle tissue. EI is quantified by analyzing the grayscale levels of the ultrasound images (35, 36). PA represents the angle between the muscle fibers and the tendon to which they attach (37). A larger PA indicates that the muscle fibers are oriented more obliquely relative to the tendon, suggesting a greater PCSA and the potential for greater force generation (38). Muscles with larger PA typically have more fibers per unit area, allowing for increased force production (39, 40). FL refers to the length of individual muscle fibers within a muscle. Longer fascicles generally allow for greater excursion and range of motion, which can be advantageous for activities requiring large muscle movements. Reduction in FL may be indicative of muscle atrophy or degeneration in individuals with sarcopenia (41, 42). Alternative US techniques, such as sonoelastography and contrast-enhanced US, have been suggested as potential tools for evaluating different aspects of muscle health in sarcopenia. Sonoelastography assesses changes in muscle stiffness, providing insights into tissue composition and integrity by measuring the elasticity of muscles (43). Contrast-enhanced US evaluates the microvasculature within muscles, which may be compromised in sarcopenia due to reduced blood flow and perfusion (44). However, research on these techniques in the context of sarcopenia is still limited, and further studies are needed to validate their utility and establish their role in clinical practice (21).
2.3 Clinical application
US has shown promise in various applications related to sarcopenia assessment (Table 2). In clinical practice, ultrasound imaging can be used for screening, diagnosis, and monitoring of sarcopenia (45). However, the proficiency and expertise of operators can significantly influence the accuracy and reliability of ultrasound measurements (46). A recent meta-analysis conducted by Wang et al. highlighted substantial methodological variation across studies, including differences in the type of ultrasonographic probe used, the positioning of the probe on the body, the specific side of the body measured, the posture of the subjects during measurements, and the specific anatomical points targeted for measurement (47). These methodological disparities underscore the need for standardized protocols and guidelines to ensure consistency and comparability across studies in the field of ultrasound-based assessment of sarcopenia. The Sarcopenia through Ultrasound (SARCUS) Working Group has recently issued two consensuses aimed at standardizing ultrasound measurements for assessing appendicular muscles. However, due to a lack of sufficient evidence, the SARCUS consensuses have not yet established specific cutoff points for US parameters across different muscle groups to diagnose sarcopenia (48). More research is needed to establish ideal cutoffs for ultrasound parameters across diverse populations, enhancing the accuracy and utility of ultrasound in sarcopenia assessment.
3 Magnetic resonance imaging
Magnetic Resonance Imaging (MRI) relies on the principles of nuclear magnetic resonance to generate detailed images of the body's internal structures, offering excellent contrast and resolution for tissue characterization. Within the context of sarcopenia assessment, MRI techniques such as T1-weighted imaging, Dixon imaging, T2 mapping, diffusion-weighted imaging (DWI), and magnetic resonance spectroscopy (MRS) provide unique insights into muscle tissue characteristics. In the following subsections the main imaging sequences used in sarcopenia diagnosis and monitoring are recalled and details, while Table 3 summarizes pros and cons of each considered imaging sequence.
3.1 T1-Weighted imaging
In the quantification of sarcopenia, T1-weighted imaging plays a crucial role as an MRI sequence. T1-weighted sequences provide excellent contrast between muscle and adipose tissue, forming the foundation for qualitative or semi-quantitative radiological assessments. An appropriate segmentation technique of these sequences is employed for quantitatively measuring the area or volume of tissue compartments. Mainly they are subcutaneous adipose tissue (SAT), defined as the layer of fat located directly beneath the skin but above the underlying muscle tissue (49), intramuscular adipose tissue (IMAT), the presence of adipose tissue within the muscle structure, specifically located between and within muscle fibers (50), or muscle tissue (MT) (51). In comparison to gradient echo sequences, SE sequences demonstrate lower sensitivity to susceptibility artifacts originating from fatty inflation (52). SE T1-weighted sequences can achieve favorable spatial resolution (0.5 × 0.5 × 3 mm3), and in extremities, a stack of 30 images can be acquired in less than 5 min (53). While T1-weighted sequences remain standard across all MR scanners and are user-friendly, their intensity values, though valuable for segmentation guidance, offer limited utility for other quantitative measurements. Despite their continued suitability for muscle volume measurement and semi-quantitative assessment of muscle fat infiltration, computed tomography presents a faster alternative less susceptible to motion artifacts (54).
3.2 Dixon imaging
Dixon imaging, also known as fat-water separation imaging, is a valuable technique in the quantification of sarcopenia through MRI (53). This method exploits the different resonance frequencies of fat and water protons, allowing for the selective visualization and quantification of these tissue components. Dixon imaging typically involves acquiring multiple image sets at varying echo times, enabling the separation of fat and water signals during post-processing. Dixon imaging employs a variety of sequences, including multi-echo gradient-echo or spin-echo sequences, to acquire images sensitive to both fat and water signals, commonly referred to as proton density fat fraction (PDFF) and proton density water fraction (PDWF) maps. The PDFF is a quantitative MRI parameter used to assess the concentration of fat in a specific tissue or region. It represents the ratio of the density of mobile protons from fat (triglycerides) to the total density of protons from both mobile triglycerides and mobile water in each voxel (55). PDFF is expressed as a percentage and reflects the proportion of the MR signal arising from fat within the imaged tissue. Mathematically, PDFF is defined as: PDFF = (Total density of protons from mobile triglycerides and mobile water/ Density of mobile protons from fat) × 100. PDFF is proposed as the most suitable standardized biomarker of tissue fat concentration, particularly when measured accurately using quantitative MRS or MRI techniques (56), with reported conversions to absolute fat mass in grams (57). The relationship PDFF + PDWF = 1 holds for a given voxel, as the measured MR signal is contributed solely by water and fat protons. However, in the presence of conditions such as edema or fibrosis, PDWF may differ from absolute water content. This underlines the importance of considering potential alterations in water content when interpreting PDFF values, particularly in clinical scenarios involving tissue abnormalities such as edema or fibrotic changes (58). A limitation arises from the inability to separate intramyocellular lipid (IMCL) and extramyocellular lipid (EMCL) in PDFF results. Consequently, PDFF measurements include contributions from IMCL, which are essential for cellular energy metabolism, while the fatty muscle infiltration attributed to EMCL can negatively impact muscle function. While Dixon imaging offers faster acquisition compared to T1-weighted images, its spatial resolution is typically inferior (59). T1-weighted images are often preferred for assessing muscle volume and segmentation tasks, although Dixon images can also serve these purposes (60). A potential drawback of Dixon imaging is the potential for bias in T1-weighted images, resulting in non-uniform contrast across images, a factor mitigated in Dixon images (61).
3.3 T2 mapping
In addition to PDFF, T2 relaxation times have gained increasing recognition as a quantitative MRI marker for assessing disease activity in skeletal muscle tissue. This role is particularly relevant in replacing Short Tau Inversion Recovery (STIR) sequences, commonly utilized for semi-quantitative edema assessment (62). Skeletal muscle edema leads to increased T2 relaxation times, serving as an indicator for inflammatory processes that precede the replacement of muscle tissue by adipose tissue. While alterations in T2 are generally non-specific, they can signify the intensity of underlying pathological changes (63). The assessment of T2 in skeletal muscles often involves the use of multi-spin-echo (MSE) sequences, with various fitting approaches proposed to evaluate signal evolution and determine T2 relaxation times (64). The simplest approach involves fitting a mono-exponential signal decay, resulting in an overall “global T2 relaxation time” that encompasses the T2 relaxation times of both water and fat (65). However, in conditions involving fat replacement, this combined T2 is predominantly influenced by the fat signal, masking more nuanced alterations in T2 resulting from other modifications in muscle tissue (66). Consequently, increased intramuscular fat content leads to a higher global T2. This effect is particularly prominent in individuals with elevated intramuscular fat, such as those with neuromuscular disorders. The rise in global T2 due to fat infiltration may obscure subtle pathological changes in T2water, typically of smaller magnitude (65). Various methods have been suggested to selectively assess the T2 of muscle water when there is fat infiltration. These approaches include selectively exciting the water signal or employing conventional fat suppression techniques to suppress the fat signal. However, the efficacy of fat suppression is contingent on precise flip angles and could be compromised in areas with an inhomogeneous transmit (B1+) field (67). An alternative approach involves multi-exponential T2 fitting, a detailed method that allows for a more nuanced analysis of signal decay. This technique goes beyond the simplicity of mono-exponential fitting and provides a comprehensive assessment of T2 relaxation times by considering multiple exponential components within the signal (68). Multi-exponential T2 fitting is particularly advantageous in situations where the conventional mono-exponential approach might oversimplify complex tissue compositions, enabling a more accurate depiction of variations in relaxation times. However, these fitting models often overlook B1 field inhomogeneities and stimulated echoes, affecting T2 values (69). To address issues such as B1 field inhomogeneities and stimulated echoes, an alternative T2 fitting method using extended phase graphs (EPG) has been proposed. EPG simulates signal evolution for various combinations of T2, relative B1, and fat fraction (FF) values, enhancing stability in an inhomogeneous transmit field and correcting for stimulated echoes. This method provides a more robust and accurate assessment of T2 relaxation times in the presence of fat infiltration and other confounding factors (66).
3.4 Diffusion weighted imaging
The utility of Diffusion Weighted Imaging (DWI) lies in its ability to assess the movement of water molecules within tissues, providing information on tissue cellularity, integrity, and the presence of pathological changes (70). However, within tissues such as muscle, the presence of impermeable or semi-permeable barriers surrounding muscle fibers and other structures imposes constraints on the diffusion of molecules. This restriction exhibits an anisotropic nature, meaning that the diffusion is directionally dependent (71). Diffusion Tensor Imaging (DTI), as an extension of DWI (72), goes beyond merely measuring the diffusion of water molecules. It specifically assesses the anisotropy of diffusion, providing a more detailed and directional understanding of water molecule movement in biological tissues. The impact of diffusion anisotropy becomes apparent through the assessment of changes in diffusion measurements when altering the direction of gradient pulses (73). Fractional anisotropy (FA) serves as a metric to quantify the directional alignment of water molecules within the tissue, with FA values ranging from 0 to 1. In cases where tissue integrity is maintained, water exhibits movement primarily in a specific direction, resulting in an FA value close to 1. Conversely, in the presence of micro- or macro-structural damages, water molecules assume multiple directions of movement, leading to a decrease in the FA value towards 0 (74). In muscle tissue due to the predominant alignment of water diffusion along the major axis of fibrillary tissues facilitated by their structural arrangement, DTI proves instrumental for fiber tractography. The computation of the diffusion tensor necessitates the acquisition of DWI with high b-values along a minimum of six non-collinear directions, supplemented by a low b-value DWI or a T2-weighted sequence (75). The precision of anisotropy calculation is directly proportional to the number of directions along which diffusion gradients are applied. It is evident that augmenting the number of directions enhances accuracy but comes at the cost of increased scan times. An additional metric derived from the DTI is the mean diffusivity (MD), often interchangeably termed the apparent diffusion coefficient (ADC) (76). Mean diffusivity is a quantitative measure that characterizes the average diffusion of water molecules within a given tissue. MD represents the overall magnitude of diffusion in all directions and is calculated as the average of the three eigenvalues obtained from the diffusion tensor. In the context of DTI, each eigenvalue corresponds to the rate of diffusion along a principal axis within the tissue (77). The mean diffusivity, therefore, provides a comprehensive assessment of the diffusion properties by considering diffusion in both the axial and radial directions (78). DTI remains relatively underutilized in the realm of muscle imaging. However, DTI offers a versatile application in uncovering alterations in muscle diffusivity associated with a spectrum of conditions. One noteworthy instance involves the investigation of changes in muscle diffusivity linked to denervation (79) and metabolic conditions (80). Muscle fiber tears, a common occurrence in various physical activities, also fall within the scope of DTI applications. The technique enables the detection and characterization of muscle fiber tears, contributing to a comprehensive assessment of musculoskeletal injuries (81, 82). Moreover, differences in muscle diffusivity have been explored in the context of distinguishing between osteoporotic and osteoarthritic subjects (83). An area of compelling investigation explores the intricate relationship between muscle properties and external muscle strength, holding profound implications for optimizing training outcomes in athletes and guiding interventions for conditions such as sarcopenia and frailty (84–86). The consistent elevation of FA serves as a notable indicator of inflammatory infiltration and muscle regeneration. These occurrences manifest as transient responses to acute injury and persist as adaptive mechanisms in reaction to the aging process (86).
3.5 MRS
MRS has been effectively employed by physiologists for numerous decades, specifically in the study of high-energy phosphates (using 31P-MRS) and glycogen (utilizing 13C-MRS), to measure concentrations of high-energy phosphates or muscular glycogen, respectively (87). Alternatively, 1H-MRS is utilized to evaluate muscle lipid composition. In 1H-MRS, an intensity spectrum is generated based on the relative chemical shifts of lipid metabolites measured in parts per million (ppm) with respect to the universal reference, the methyl 1H signal of tetramethylsilane (TMS), assigned a value of 0 ppm (88). Within this reference system, water protons in living tissues resonate at −4.7 ppm, EMCL at −1.5 ppm, and IMCL at −1.28 ppm. In MR imaging, we use the chemical shift between water and fat to either suppress the fat signal and highlight the water signal or in techniques like Dixon imaging, to separate fat and water signals. However, Dixon imaging struggles to detect the small chemical shift difference (−0.2 ppm) between EMCL and IMCL (89). On the other hand, MRS can distinguish between these lipids, due to structural differences. This requires longer acquisition times, and the MRS signal is typically obtained from a small volume of interest, known as the spectroscopy voxel (about 1 cm3) (90). Recent consensus recommendations provide guidance on performing 1H-MRS in skeletal muscle (91). Repositioning the spectroscopic voxel in MRS poses a challenge in muscle imaging due to the elasticity of muscle tissue. This challenge is heightened by the difficulty in reproducible positioning, especially with the high and uneven fat infiltration seen in elderly and diseased individuals. Consequently, MRS exhibits lower precision compared to Dixon imaging (92). In contrast, Dixon imaging is less affected in the liver, an organ frequently imaged using this technique, owing to its more uniform tissue composition. While MRS could potentially overcome these challenges, its applications in muscle imaging are currently limited (93). While 1H-MRS has historically been utilized in muscular dystrophies, it is progressively being supplanted by Dixon imaging and T2 mapping (94). The quantification of IMCLs proves to be a potent tool in exercise and nutrition research, bioenergetics studies, and investigations into insulin resistance (95).
4 Bioelectrical impedance analysis (BIA)
BIA is a non-invasive technique that measures the impedance of body tissues to electrical currents to estimate body composition, including muscle mass (96). BIA devices vary in complexity, from handheld devices to multi-frequency analyzers. A systematic review by Aleixo et al. shows that BIA is feasible for evaluating low lean muscle mass for the diagnosis of sarcopenia (97). Two studies demonstrated that BIA yielded comparable results to gold standard methods such as CT and MRI in detecting sarcopenia (98, 99). This evidence has led to the acceptance of BIA as a reliable method in various sarcopenia guidelines, including those in Europe and Asia, as well as the international cachexia consensus (100). However, other authors have indicated that a limitation of BIA in assessing sarcopenia is its reliance on hydration status (101). BIA relies on the assumption that the body is adequately hydrated for accurate measurements of lean body mass. However, variations in hydration levels, such as dehydration or overhydration, can affect the accuracy of BIA results. Additionally, BIA may not provide detailed information about muscle quality or distribution, which could limit its ability to assess sarcopenia comprehensively (102). Moreover, BIA measurements can be influenced by factors such as age, sex, ethnicity, and body composition, which may introduce variability in the interpretation of results (103). Therefore, while BIA is a convenient and non-invasive method for estimating body composition, its limitations should be considered when using it for sarcopenia assessment.
5 Emerging technologies
5.1 Microwave based diagnostics
Besides the well-known and assessed instrumentations recalled above, an emerging technique which is proposed for various medical diagnostic applications is represented by microwave imaging and sensing. More precisely, studies on the dielectric properties (electric permittivity and conductivity) of human tissues have proved that, in the microwave frequency range, different tissues exhibit different properties, but also differences can be detected in the same tissue according to its physio-pathological status (104–106). This occurrence entails the possibility of exploiting microwave sensing and imaging for diagnostic purposes through the detection and quantification of the electric contrast existing between tissues, made possible by the proper processing of the microwave signal which is transmitted, attenuated and backscattered by the tissues. Microwave imaging has been proposed for several medical applications, ranging from breast cancer diagnosis, brain stroke imaging and monitoring (106–109). Motivated by the evidence that sarcopenia, from a histological viewpoint, entails the substitution of muscle fibers with adipose tissue and given the high electric contrast existing between fat and muscle, recently microwaves have been proposed as a possible non-invasive means to diagnose sarcopenia at an early stage (1, 2, 110). The possibility of exploiting this technique is at a very preliminary phase and, so far, a microwave sensor for the evaluation of muscle quality has been proposed, but no relevant clinical assessment of such a sensor is reported in the literature (111). On the other hand, the great potential of providing 3D images of the examined body area is completely underinvestigated for sarcopenia diagnosis. However, the non-ionizing nature of microwaves and the expected high specificity of the diagnosis make MWI as a very promising tool, for the screening of sarcopenia and, even more, for the monitoring and follow up of the disease.
5.2 Near-infrared spectroscopy (NIRS)
Near-Infrared Spectroscopy (NIRS) is an emerging non-invasive method that can be utilized to assess muscle function and possibly sarcopenia. This technique measures the absorption of near-infrared light by the tissues, which can provide insights into tissue composition and blood flow dynamics. NIRS works by emitting near-infrared light into the body's tissues using sensors placed on the skin surface. The light penetrates the tissues and is either absorbed or scattered by the muscles and blood vessels (112). In a preliminary physiological study conducted by Beever et al. involving 26 healthy participants, NIRS proved to be a dependable method for examining skeletal muscle oxidative capacity (113). This result was confirmed by an investigation of Tandirerung et al. in a cohort of 25 young and old non-athletic adult patients (114). NIRS can be particularly pertinent in the study of sarcopenia, where mitochondrial function and muscle metabolism are often compromised due to declining muscle mass and qualitative changes within muscle tissues. A study by Shin et al. demonstrated a high level of agreement between portable NIRS devices and BIA in measuring muscle mass among community-dwelling older adults. This study suggests that NIRS it could be used more widely as a convenient, non-invasive tool for screening and monitoring sarcopenia (115). However, NIRS also has several limitations. The accuracy of NIRS can be affected by factors such as subcutaneous fat thickness, skin color, and tissue heterogeneity. These factors can influence the penetration of near-infrared light into tissues, potentially leading to variations in data accuracy and reliability (116). Another drawback is the limited penetration depth of NIRS, which restricts its use to superficial tissues. This limitation makes it less effective for studying deeper muscles or organs compared to other imaging modalities like MRI or CT. Additionally, NIRS data interpretation can be complex. The signals obtained are influenced by both arterial and venous blood, as well as by the water content in the tissue, which can complicate the distinction between changes in blood flow and actual muscle oxygen consumption (117). Thus, while NIRS offers significant benefits for non-invasive physiological monitoring, its limitations require careful consideration in the design and interpretation of studies or clinical assessments.
6 Conclusions
In conclusion, sarcopenia presents a growing global challenge, underscoring the importance of effective diagnostic tools. As summarized in Table 4, various imaging modalities offer valuable insights into muscle quantity and quality, with MRI emerging as particularly promising due to its high-resolution capabilities and fat-water separation techniques. Additionally, ultrasound provides essential information on muscle size and composition, while BIA offers a non-invasive alternative for assessing body composition. The inclusion of NIRS also adds a layer of depth, as it has proven to be a reliable tool in assessing skeletal muscle oxidative capacity, further enhancing our toolkit for diagnosing and monitoring sarcopenia. Standardized protocols are crucial for ensuring consistency in assessments across modalities. Future research should focus on refining these techniques towards a unified framework. Also, the potential of radiomics and artificial intelligence in improving diagnostic accuracy as well as in predicting outcomes in sarcopenia are worthy of consideration in the future research.
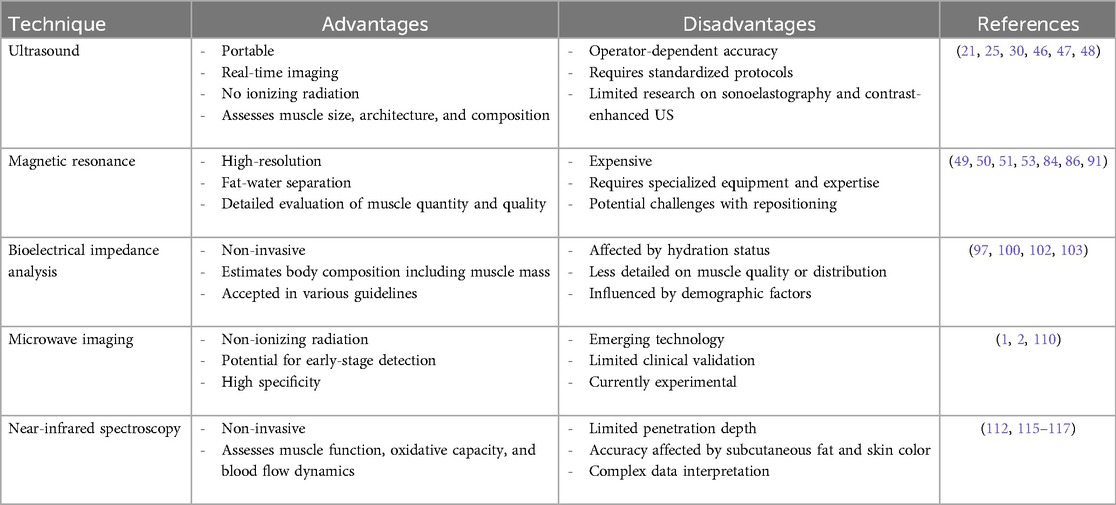
Table 4. A comparison of the different techniques, highlighting their strengths and limitations in the context of sarcopenia evaluation.
Author contributions
SL: Data curation, Methodology, Writing – original draft, Writing – review & editing. RS: Formal Analysis, Resources, Writing – original draft, Writing – review & editing. EM: Methodology, Writing – review & editing. CM: Formal Analysis, Methodology, Writing – review & editing. AA: Data curation, Resources, Writing – original draft. VM: Supervision, Visualization, Writing – review & editing. AR: Supervision, Visualization, Writing – review & editing. FP: Conceptualization, Supervision, Writing – original draft, Writing – review & editing.
Funding
The author(s) declare that no financial support was received for the research, authorship, and/or publication of this article.
Conflict of interest
The authors declare that the research was conducted in the absence of any commercial or financial relationships that could be construed as a potential conflict of interest.
Publisher's note
All claims expressed in this article are solely those of the authors and do not necessarily represent those of their affiliated organizations, or those of the publisher, the editors and the reviewers. Any product that may be evaluated in this article, or claim that may be made by its manufacturer, is not guaranteed or endorsed by the publisher.
References
1. Cruz-Jentoft AJ, Landi F, Schneider SM, Zuniga C, Arai H, Boirie Y, et al. Prevalence of and interventions for sarcopenia in ageing adults: a systematic review. Report of the international sarcopenia initiative (EWGSOP and IWGS). Age Ageing. (2014) 43(6):748–59. doi: 10.1093/ageing/afu115
2. Petermann-Rocha F, Balntzi V, Gray SR, Lara J, Ho FK, Pell JP, et al. Global prevalence of sarcopenia and severe sarcopenia: a systematic review and meta-analysis. J Cachexia Sarcopenia Muscle. (2022) 13(1):86–99. doi: 10.1002/jcsm.12783
3. Davydov DM, Boev A, Gorbunov S. Making the choice between bioelectrical impedance measures for body hydration status assessment. Sci Rep. (2021) 11(1):7685. doi: 10.1038/s41598-021-87253-4
4. Larsson L, Degens H, Li M, Salviati L, Lee YI, Thompson W, et al. Sarcopenia: aging-related loss of muscle mass and function. Physiol Rev. (2019) 99(1):427–511. doi: 10.1152/physrev.00061.2017
5. Papadopoulou S. Sarcopenia: a contemporary health problem among older adult populations. Nutrients. (2020) 12(5):1293. doi: 10.3390/nu12051293
6. Hong SH, Choi KM. Sarcopenic obesity, insulin resistance, and their implications in cardiovascular and metabolic consequences. Int J Mol Sci. (2020) 21(2):494. doi: 10.3390/ijms21020494
7. Lavalle S, Valerio MR, Masiello E, Gebbia V, Scandurra G. Unveiling the intricate dance: how cancer orchestrates muscle wasting and sarcopenia. In Vivo. (2024) 38(4):1520–9. doi: 10.21873/invivo.13602
8. Liguori I, Russo G, Aran L, Bulli G, Curcio F, Della-Morte D, et al. Sarcopenia: assessment of disease burden and strategies to improve outcomes. Clin Interv Aging. (2018) 13:913–27. doi: 10.2147/CIA.S149232
9. Oudbier SJ, Goh J, Looijaard SMLM, Reijnierse EM, Meskers CGM, Maier AB. Pathophysiological mechanisms explaining the association between low skeletal muscle mass and cognitive function. J Gerontol A. (2022) 77(10):1959–68. doi: 10.1093/gerona/glac121
10. Arosio B, Calvani R, Ferri E, Coelho-Junior HJ, Carandina A, Campanelli F, et al. Sarcopenia and cognitive decline in older adults: targeting the muscle–brain axis. Nutrients. (2023) 15(8):1853. doi: 10.3390/nu15081853
11. Sartori R, Romanello V, Sandri M. Mechanisms of muscle atrophy and hypertrophy: implications in health and disease. Nat Commun. (2021) 12(1):330. doi: 10.1038/s41467-020-20123-1
12. Cho MR, Lee S, Song SK. A review of sarcopenia pathophysiology, diagnosis, treatment and future direction. J Korean Med Sci. (2022) 37(18):e146. doi: 10.3346/jkms.2022.37.e146
13. Aslam MA, Ma EB, Huh JY. Pathophysiology of sarcopenia: genetic factors and their interplay with environmental factors. Metab Clin Exp. (2023) 149:155711. doi: 10.1016/j.metabol.2023.155711
14. Ciciliot S, Rossi AC, Dyar KA, Blaauw B, Schiaffino S. Muscle type and fiber type specificity in muscle wasting. Int J Biochem Cell Biol. (2013) 45(10):2191–9. doi: 10.1016/j.biocel.2013.05.016
15. Marzetti E, Lozanoska-Ochser B, Calvani R, Landi F, Coelho-Júnior HJ, Picca A. Restoring mitochondrial function and muscle satellite cell signaling: remedies against age-related sarcopenia. Biomolecules. (2024) 14(4):415. doi: 10.3390/biom14040415
16. Vainshtein A, Sandri M. Signaling pathways that control muscle mass. Int J Mol Sci. (2020) 21(13):4759. doi: 10.3390/ijms21134759
17. Bodine SC. The role of mTORC1 in the regulation of skeletal muscle mass. Fac Rev. (2022) 11:11–32. doi: 10.12703/r/11-32
18. Sirago G, Picca A, Calvani R, Coelho-Júnior HJ, Marzetti E. Mammalian target of rapamycin (mTOR) signaling at the crossroad of muscle fiber fate in sarcopenia. Int J Mol Sci. (2022) 23(22):13823. doi: 10.3390/ijms232213823
19. Ackermans LLGC, Rabou J, Basrai M, Schweinlin A, Bischoff SC, Cussenot O, et al. Screening, diagnosis and monitoring of sarcopenia: when to use which tool? Clin Nutr ESPEN. (2022) 48:36–44. doi: 10.1016/j.clnesp.2022.01.027
20. Locquet M, Beaudart C, Reginster JY, Petermans J, Bruyère O. Comparison of the performance of five screening methods for sarcopenia. Clin Epidemiol. (2017) 10:71–82. doi: 10.2147/CLEP.S148638
21. Albano D, Messina C, Vitale J, Sconfienza LM. Imaging of sarcopenia: old evidence and new insights. Eur Radiol. (2020) 30(4):2199–208. doi: 10.1007/s00330-019-06573-2
22. Bredella MA, Ghomi RH, Thomas BJ, Torriani M, Brick DJ, Gerweck AV, et al. Comparison of DXA and CT in the assessment of body composition in premenopausal women with obesity and anorexia nervosa. Obesity. (2010) 18(11):2227–33. doi: 10.1038/oby.2010.5
23. Bhuachalla ÉB N, Daly LE, Power DG, Cushen SJ, MacEneaney P, Ryan AM. Computed tomography diagnosed cachexia and sarcopenia in 725 oncology patients: is nutritional screening capturing hidden malnutrition? J Cachexia Sarcopenia Muscle. (2018) 9(2):295–305. doi: 10.1002/jcsm.12258
24. Lenchik L, Lenoir KM, Tan J, Boutin RD, Callahan KE, Kritchevsky SB, et al. Opportunistic measurement of skeletal muscle size and muscle attenuation on computed tomography predicts 1-year mortality in medicare patients. J Gerontol A. (2019) 74(7):1063–9. doi: 10.1093/gerona/gly183
25. Abe T, Patterson KM, Stover CD, Geddam DAR, Tribby AC, Lajza DG, et al. Site-specific thigh muscle loss as an independent phenomenon for age-related muscle loss in middle-aged and older men and women. Age (Omaha). (2014) 36(3):9634. doi: 10.1007/s11357-014-9634-3
26. Reeves ND, Maganaris CN, Narici M V. Ultrasonographic assessment of human skeletal muscle size. Eur J Appl Physiol. (2004) 91(1):116–8. doi: 10.1007/s00421-003-0961-9
27. Paris MT, Lafleur B, Dubin JA, Mourtzakis M. Development of a bedside viable ultrasound protocol to quantify appendicular lean tissue mass. J Cachexia Sarcopenia Muscle. (2017) 8(5):713–26. doi: 10.1002/jcsm.12213
28. Van den Broeck J, Héréus S, Cattrysse E, Raeymaekers H, De Maeseneer M, Scafoglieri A. Reliability of muscle quantity and quality measured with extended-field-of-view ultrasound at nine body sites. Ultrasound Med Biol. (2023) 49(7):1544–9. doi: 10.1016/j.ultrasmedbio.2023.02.018
29. Rustani K, Kundisova L, Capecchi PL, Nante N, Bicchi M. Ultrasound measurement of rectus femoris muscle thickness as a quick screening test for sarcopenia assessment. Arch Gerontol Geriatr. (2019) 83:151–4. doi: 10.1016/j.archger.2019.03.021
30. Sanz-Paris A, González-Fernandez M, Hueso-Del Río LE, Ferrer-Lahuerta E, Monge-Vazquez A, Losfablos-Callau F, et al. Muscle thickness and echogenicity measured by ultrasound could detect local sarcopenia and malnutrition in older patients hospitalized for hip fracture. Nutrients. (2021) 13(7):2401. doi: 10.3390/nu13072401
31. Naimo MA, Varanoske AN, Hughes JM, Pasiakos SM. Skeletal muscle quality: a biomarker for assessing physical performance capabilities in young populations. Front Physiol. (2021) 12:706699. doi: 10.3389/fphys.2021.706699
32. Ozturk Y, Koca M, Burkuk S, Unsal P, Dikmeer A, Oytun MG, et al. The role of muscle ultrasound to predict sarcopenia. Nutrition. (2022) 101:111692. doi: 10.1016/j.nut.2022.111692
33. Papenkort S, Böl M, Siebert T. Architectural model for muscle growth during maturation. Biomech Model Mechanobiol. (2021) 20(5):2031–44. doi: 10.1007/s10237-021-01492-y
34. Mayans D, Cartwright MS, Walker FO. Neuromuscular ultrasonography: quantifying muscle and nerve measurements. Phys Med Rehabil Clin N Am. (2012) 23(1):133–48. doi: 10.1016/j.pmr.2011.11.009
35. Young H, Jenkins NT, Zhao Q, Mccully KK. Measurement of intramuscular fat by muscle echo intensity. Muscle Nerve. (2015) 52(6):963–71. doi: 10.1002/mus.24656
36. Watanabe Y, Ikenaga M, Yoshimura E, Yamada Y, Kimura M. Association between echo intensity and attenuation of skeletal muscle in young and older adults: a comparison between ultrasonography and computed tomography. Clin Interv Aging. (2018) 13:1871–8. doi: 10.2147/CIA.S173372
37. Infantolino BW, Challis JH. Short communication: pennation angle variability in human muscle. J Appl Biomech. (2014) 30(5):663–7. doi: 10.1123/jab.2013-0334
38. Sopher RS, Amis AA, Davies DC, Jeffers JR. The influence of muscle pennation angle and cross-sectional area on contact forces in the ankle joint. J Strain Anal Eng Des. (2017) 52(1):12–23. doi: 10.1177/0309324716669250
39. Trevino M, Perez S, Sontag S, Olmos A, Jeon S, Richardson L. Influence of pennation angle and muscle thickness on mechanomyographic amplitude–torque relationships and sex-related differences in the vastus lateralis. J Funct Morphol Kinesiol. (2023) 8(2):53. doi: 10.3390/jfmk8020053
40. Eng CM, Azizi E, Roberts TJ. Structural determinants of muscle gearing during dynamic contractions. Integr Comp Biol. (2018) 58(2):207–18. doi: 10.1093/icb/icy054
41. Tumkur Anil Kumar N, Oliver JL, Lloyd RS, Pedley JS, Radnor JM. The influence of growth, maturation and resistance training on muscle-tendon and neuromuscular adaptations: a narrative review. Sports. (2021) 9(5):59. doi: 10.3390/sports9050059
42. Narici M, McPhee J, Conte M, Franchi MV, Mitchell K, Tagliaferri S, et al. Age-related alterations in muscle architecture are a signature of sarcopenia: the ultrasound sarcopenia index. J Cachexia Sarcopenia Muscle. (2021) 12(4):973–82. doi: 10.1002/jcsm.12720
43. Janczyk EM, Champigny N, Michel E, Raffaelli C, Annweiler C, Zory R, et al. Sonoelastography to assess muscular stiffness among older adults and its use for the diagnosis of sarcopenia: a systematic review. Ultraschall Med. (2021) 42(06):634–42. doi: 10.1055/a-1293-8057
44. Nguyen T, Davidson BP. Contrast enhanced ultrasound perfusion imaging in skeletal muscle. J Cardiovasc Imaging. (2019) 27(3):163. doi: 10.4250/jcvi.2019.27.e31
45. Salaffi F, Carotti M, Di Matteo A, Ceccarelli L, Farah S, Villota-Eraso C, et al. Ultrasound and magnetic resonance imaging as diagnostic tools for sarcopenia in immune-mediated rheumatic diseases (IMRDs). Radiol Med. (2022) 127(11):1277–91. doi: 10.1007/s11547-022-01560-y
46. Perkisas S, Baudry S, Bauer J, Beckwée D, De Cock AM, Hobbelen H, et al. Application of ultrasound for muscle assessment in sarcopenia: towards standardized measurements. Eur Geriatr Med. (2018) 9(6):739–57. doi: 10.1007/s41999-018-0104-9
47. Wang JC, Wu WT, Chang KV, Chen LR, Chi SY, Kara M, et al. Ultrasound imaging for the diagnosis and evaluation of sarcopenia: an Umbrella review. Life. (2021) 12(1):9. doi: 10.3390/life12010009
48. Perkisas S, Baudry S, Bauer J, Beckwée D, De Cock AM, Hobbelen H, et al. The SARCUS project: evidence-based muscle assessment through ultrasound. Eur Geriatr Med. (2019) 10(1):157–8. doi: 10.1007/s41999-018-0141-4
49. Bunch PM, Rigdon J, Niazi MKK, Barnard RT, Boutin RD, Houston DK, et al. Association of CT-derived skeletal muscle and adipose tissue metrics with frailty in older adults. Acad Radiol. (2024) 31(2):596–604. doi: 10.1016/j.acra.2023.06.003
50. Vettor R, Milan G, Franzin C, Sanna M, De Coppi P, Rizzuto R, et al. The origin of intermuscular adipose tissue and its pathophysiological implications. Am J Physiol Endocrinol Metab. (2009) 297(5):E987–98. doi: 10.1152/ajpendo.00229.2009
51. Ogawa M, Lester R, Akima H, Gorgey A. Quantification of intermuscular and intramuscular adipose tissue using magnetic resonance imaging after neurodegenerative disorders. Neural Regen Res. (2017) 12(12):2100. doi: 10.4103/1673-5374.221170
52. Markl M, Leupold J. Gradient echo imaging. J Magn Reson Imaging. (2012) 35(6):1274–89. doi: 10.1002/jmri.23638
53. Engelke K, Chaudry O, Gast L, Eldib MAB, Wang L, Laredo JD, et al. Magnetic resonance imaging techniques for the quantitative analysis of skeletal muscle: state of the art. J Orthop Translat. (2023) 42:57–72. doi: 10.1016/j.jot.2023.07.005
54. Huber FA, Del Grande F, Rizzo S, Guglielmi G, Guggenberger R. MRI In the assessment of adipose tissues and muscle composition: how to use it. Quant Imaging Med Surg. (2020) 10(8):1636–49. doi: 10.21037/qims.2020.02.06
55. Sollmann N, Zoffl A, Franz D, Syväri J, Dieckmeyer M, Burian E, et al. Regional variation in paraspinal muscle composition using chemical shift encoding-based water-fat MRI. Quant Imaging Med Surg. (2020) 10(2):496–507. doi: 10.21037/qims.2020.01.10
56. Reeder SB, Hu HH, Sirlin CB. Proton density fat-fraction: a standardized MR-based biomarker of tissue fat concentration. J Magn Reson Imaging. (2012) 36(5):1011–4. doi: 10.1002/jmri.23741
57. Hu J, Li G, Xu J, Song Y. Calculation methods of message time parameters considering different first production times of signals in automotive embedded networked control system. Int J Adv Comput Technol. (2011) 3(3):111–22. doi: 10.4156/ijact.vol3.issue3.11
58. Burakiewicz J, Sinclair CDJ, Fischer D, Walter GA, Kan HE, Hollingsworth KG. Quantifying fat replacement of muscle by quantitative MRI in muscular dystrophy. J Neurol. (2017) 264(10):2053–67. doi: 10.1007/s00415-017-8547-3
59. Lins CF, Salmon CEG, Nogueira-Barbosa MH. Applications of the dixon technique in the evaluation of the musculoskeletal system. Radiol Bras. (2021) 54(1):33–42. doi: 10.1590/0100-3984.2019.0086
60. Grimm A, Meyer H, Nickel MD, Nittka M, Raithel E, Chaudry O, et al. Repeatability of dixon magnetic resonance imaging and magnetic resonance spectroscopy for quantitative muscle fat assessments in the thigh. J Cachexia Sarcopenia Muscle. (2018) 9(6):1093–100. doi: 10.1002/jcsm.12343
61. Omoumi P. The dixon method in musculoskeletal MRI: from fat-sensitive to fat-specific imaging. Skeletal Radiol. (2022) 51(7):1365–9. doi: 10.1007/s00256-021-03950-1
62. Giraudo C, Cavaliere A, Lupi A, Guglielmi G, Quaia E. Established paths and new avenues: a review of the main radiological techniques for investigating sarcopenia. Quant Imaging Med Surg. (2020) 10(8):1602–13. doi: 10.21037/qims.2019.12.15
63. Willcocks R. Global T2 versus water T2 in NMR imaging of fatty infiltrated muscles: different methodology, different information, and different implications. Neuromuscul Disord. (2014) 24(12):1120–1. doi: 10.1016/j.nmd.2014.07.002
64. Keene KR, Beenakker JM, Hooijmans MT, Naarding KJ, Niks EH, Otto LAM, et al. T2 relaxation-time mapping in healthy and diseased skeletal muscle using extended phase graph algorithms. Magn Reson Med. (2020) 84(5):2656–70. doi: 10.1002/mrm.28290
65. Carlier PG. Global T2 versus water T2 in NMR imaging of fatty infiltrated muscles: different methodology, different information and different implications. Neuromuscul Disord. (2014) 24(5):390–2. doi: 10.1016/j.nmd.2014.02.009
66. Marty B, Baudin P, Reyngoudt H, Azzabou N, Araujo ECA, Carlier PG, et al. Simultaneous muscle water T2 and fat fraction mapping using transverse relaxometry with stimulated echo compensation. NMR Biomed. (2016) 29(4):431–43. doi: 10.1002/nbm.3459
67. Del Grande F, Santini F, Herzka DA, Aro MR, Dean CW, Gold GE, et al. Fat-suppression techniques for 3-T MR imaging of the musculoskeletal system. RadioGraphics. (2014) 34(1):217–33. doi: 10.1148/rg.341135130
68. Azzabou N, Loureiro de Sousa P, Caldas E, Carlier PG. Validation of a generic approach to muscle water T2 determination at 3T in fat-infiltrated skeletal muscle. J Magn Reson Imaging. (2015) 41(3):645–53. doi: 10.1002/jmri.24613
69. Lebel RM, Wilman AH. Transverse relaxometry with stimulated echo compensation. Magn Reson Med. (2010) 64(4):1005–14. doi: 10.1002/mrm.22487
70. Fornasa F. Diffusion-weighted magnetic resonance imaging: what makes water run fast or slow? J Clin Imaging Sci. (2011) 1:27. doi: 10.4103/2156-7514.81294
71. Cotten A, Haddad F, Hayek G, Lefebvre G, Dodré E, Budzik JF. Tractography: possible applications in musculoskeletal radiology. Semin Musculoskelet Radiol. (2015) 19(04):387–95. doi: 10.1055/s-0035-1563736
72. Chianca V, Albano D, Messina C, Cinnante CM, Triulzi FM, Sardanelli F, et al. Diffusion tensor imaging in the musculoskeletal and peripheral nerve systems: from experimental to clinical applications. Eur Radiol Exp. (2017) 1(1):12. doi: 10.1186/s41747-017-0018-1
73. Le Bihan D. Molecular diffusion nuclear magnetic resonance imaging. Magn Reson Q. (1991) 7(1):1–30.2043461
74. Oudeman J, Nederveen AJ, Strijkers GJ, Maas M, Luijten PR, Froeling M. Techniques and applications of skeletal muscle diffusion tensor imaging: a review. J Magn Reson Imaging. (2016) 43(4):773–88. doi: 10.1002/jmri.25016
75. Soares JM, Marques P, Alves V, Sousa N. A hitchhiker’s guide to diffusion tensor imaging. Front Neurosci. (2013) 7:7–31. doi: 10.3389/fnins.2013.00031
76. Martinez-Heras E, Grussu F, Prados F, Solana E, Llufriu S. Diffusion-weighted imaging: recent advances and applications. Seminars in ultrasound. CT MRI. (2021) 42(5):490–506. doi: 10.1053/j.sult.2021.07.006
77. O’Donnell LJ, Westin CF. An introduction to diffusion tensor image analysis. Neurosurg Clin N Am. (2011) 22(2):185–96. doi: 10.1016/j.nec.2010.12.004
78. Winklewski PJ, Sabisz A, Naumczyk P, Jodzio K, Szurowska E, Szarmach A. Understanding the physiopathology behind axial and radial diffusivity changes—what do we know? Front Neurol. (2018) 9:9–92. doi: 10.3389/fneur.2018.00092
79. Tan ET, Zochowski KC, Sneag DB. Diffusion MRI fiber diameter for muscle denervation assessment. Quant Imaging Med Surg. (2022) 12(1):80–94. doi: 10.21037/qims-21-313
80. Edalati M, Hastings MK, Sorensen CJ, Zayed M, Mueller MJ, Hildebolt CF, et al. Diffusion tensor imaging of the calf muscles in subjects with and without diabetes Mellitus. J Magn Reson Imaging. (2019) 49(5):1285–95. doi: 10.1002/jmri.26286
81. Biglands JD, Grainger AJ, Robinson P, Tanner SF, Tan AL, Feiweier T, et al. MRI in acute muscle tears in athletes: can quantitative T2 and DTI predict return to play better than visual assessment? Eur Radiol. (2020) 30(12):6603–13. doi: 10.1007/s00330-020-06999-z
82. Giraudo C, Motyka S, Weber M, Karner M, Resinger C, Feiweier T, et al. Normalized STEAM-based diffusion tensor imaging provides a robust assessment of muscle tears in football players: preliminary results of a new approach to evaluate muscle injuries. Eur Radiol. (2018) 28(7):2882–9. doi: 10.1007/s00330-017-5218-9
83. Di Pietro G, Scimeca M, Iundusi R, Celi M, Gasbarra E, Tarantino U, et al. Differences between muscle from osteoporotic and osteoarthritic subjects: in vitro study by diffusion-tensor MRI and histological findings. Aging Clin Exp Res. (2020) 32(12):2489–99. doi: 10.1007/s40520-020-01483-6
84. Yamauchi K, Someya K, Kato C, Kato T. The relationship between quadriceps femoris muscle function and MRI-derived water diffusion and adipose tissue measurements in young healthy males. J Magn Reson Imaging. (2023) 58(2):548–56. doi: 10.1002/jmri.28525
85. Keller S, Yamamura J, Sedlacik J, Wang ZJ, Gebert P, Starekova J, et al. Diffusion tensor imaging combined with T2 mapping to quantify changes in the skeletal muscle associated with training and endurance exercise in competitive triathletes. Eur Radiol. (2020) 30(5):2830–42. doi: 10.1007/s00330-019-06576-z
86. Esposito A, Campana L, Palmisano A, De Cobelli F, Canu T, Santarella F, et al. Magnetic resonance imaging at 7T reveals common events in age-related sarcopenia and in the homeostatic response to muscle Sterile injury. PLoS One. (2013) 8(3):e59308. doi: 10.1371/journal.pone.0059308
87. Boesch C. Musculoskeletal spectroscopy. J Magn Reson Imaging. (2007) 25(2):321–38. doi: 10.1002/jmri.20806
88. Harris RK, Becker ED, Cabral de Menezes SM, Goodfellow R, Granger P. NMR nomenclature: nuclear spin properties and conventions for chemical shifts. Solid State Nucl Magn Reson. (2002) 22(4):458–83. doi: 10.1006/snmr.2002.0063
89. Pola A, Sadananthan SA, Yaligar J, Nagarajan V, Han W, Kuchel PW, et al. Skeletal muscle lipid metabolism studied by advanced magnetic resonance spectroscopy. Prog Nucl Magn Reson Spectrosc. (2012) 65:66–76. doi: 10.1016/j.pnmrs.2012.02.002
90. Boesch C, Slotboom J, Hoppeler H, Kreis R. In vivo determination of intra-myocellular lipids in human muscle by means of localized 1H-MR-spectroscopy. Magn Reson Med. (1997) 37(4):484–93. doi: 10.1002/mrm.1910370403
91. Krššák M, Lindeboom L, Schrauwen-Hinderling V, Szczepaniak LS, Derave W, Lundbom J, et al. Proton magnetic resonance spectroscopy in skeletal muscle: experts’ consensus recommendations. NMR Biomed. (2021) 34(5):e4266. doi: 10.1002/nbm.4266
92. Alhulail AA, Patterson DA, Xia P, Zhou X, Lin C, Thomas MA, et al. Fat-water separation by fast metabolite cycling magnetic resonance spectroscopic imaging at 3T: a method to generate separate quantitative distribution maps of musculoskeletal lipid components. Magn Reson Med. (2020) 84(3):1126–39. doi: 10.1002/mrm.28228
93. Nagarajan R, Carpenter CL, Lee CC, Michael N, Sarma MK, Souza R, et al. Assessment of lipid and metabolite changes in obese calf muscle using multi-Echo Echo-planar correlated spectroscopic imaging. Sci Rep. (2017) 7(1):17338. doi: 10.1038/s41598-017-17529-1
94. Barnard AM, Willcocks RJ, Finanger EL, Daniels MJ, Triplett WT, Rooney WD, et al. Skeletal muscle magnetic resonance biomarkers correlate with function and sentinel events in Duchenne muscular dystrophy. PLoS One. (2018) 13(3):e0194283. doi: 10.1371/journal.pone.0194283
95. Prompers JJ, Jeneson JAL, Drost MR, Oomens CCW, Strijkers GJ, Nicolay K. Dynamic MRS and MRI of skeletal muscle function and biomechanics. NMR Biomed. (2006) 19(7):927–53. doi: 10.1002/nbm.1095
96. Gába A, Kapuš O, Cuberek R, Botek M. Comparison of multi- and single-frequency bioelectrical impedance analysis with dual-energy X-ray absorptiometry for assessment of body composition in post-menopausal women: effects of body mass index and accelerometer-determined physical activity. J Hum Nutr Diet. (2015) 28(4):390–400. doi: 10.1111/jhn.12257
97. Aleixo GFP, Shachar SS, Nyrop KA, Muss HB, Battaglini CL, Williams GR. Bioelectrical impedance analysis for the assessment of sarcopenia in patients with cancer: a systematic review. Oncologist. (2020) 25(2):170–82. doi: 10.1634/theoncologist.2019-0600
98. Cruz-Jentoft AJ, Bahat G, Bauer J, Boirie Y, Bruyère O, Cederholm T, et al. Sarcopenia: revised European consensus on definition and diagnosis. Age Ageing. (2019) 48(1):16–31. doi: 10.1093/ageing/afy169
99. Chen LK, Liu LK, Woo J, Assantachai P, Auyeung TW, Bahyah KS, et al. Sarcopenia in Asia: consensus report of the Asian working group for sarcopenia. J Am Med Dir Assoc. (2014) 15(2):95–101. doi: 10.1016/j.jamda.2013.11.025
100. Blum D, Stene GB, Solheim TS, Fayers P, Hjermstad MJ, Baracos VE, et al. Validation of the consensus-definition for cancer cachexia and evaluation of a classification model—a study based on data from an international multicentre project (EPCRC-CSA). Ann Oncol. (2014) 25(8):1635–42. doi: 10.1093/annonc/mdu086
101. Lemos T, Gallagher D. Current body composition measurement techniques. Curr Opin Endocrinol Diabetes Obes. (2017) 24(5):310–4. doi: 10.1097/MED.0000000000000360
102. Moore ML, Benavides ML, Dellinger JR, Adamson BT, Tinsley GM. Segmental body composition evaluation by bioelectrical impedance analysis and dual-energy x-ray absorptiometry: quantifying agreement between methods. Clin Nutr. (2020) 39(9):2802–10. doi: 10.1016/j.clnu.2019.12.009
103. D’Hondt J, Waterplas J, Chapelle L, Clarys P, D’Hondt E. A comparative and sex-specific study of bio-electrical impedance analysis and dual energy x-ray absorptiometry for estimating whole-body and segmental body composition in healthy young adults. Appl Sci. (2022) 12(15):7686. doi: 10.3390/app12157686
104. Gabriel C, Gabriel S, Corthout E. The dielectric properties of biological tissues: I. Literature survey. Phys Med Biol. (1996) 41(11):2231–49. doi: 10.1088/0031-9155/41/11/001
105. Gabriel S, Lau RW, Gabriel C. The dielectric properties of biological tissues: III. Parametric models for the dielectric spectrum of tissues. Phys Med Biol. (1996) 41(11):2271–93. doi: 10.1088/0031-9155/41/11/003
106. Lazebnik M, McCartney L, Popovic D, Watkins CB, Lindstrom MJ, Harter J, et al. A large-scale study of the ultrawideband microwave dielectric properties of normal breast tissue obtained from reduction surgeries. Phys Med Biol. (2007) 52(10):2637–56. doi: 10.1088/0031-9155/52/10/001
107. Aldhaeebi MA, Alzoubi K, Almoneef TS, Bamatraf SM, Attia H, Ramahi OM. Review of microwaves techniques for breast cancer detection. Sensors. (2020) 20(8):2390. doi: 10.3390/s20082390
108. Vasquez JAT, Scapaticci R, Turvani G, Bellizzi G, Rodriguez-Duarte DO, Joachimowicz N, et al. A prototype microwave system for 3D brain stroke imaging. Sensors. (2020) 20(9):2607. doi: 10.3390/s20092607
109. Rodriguez-Duarte DO, Tobon Vasquez JA, Scapaticci R, Turvani G, Cavagnaro M, Casu MR, et al. Experimental validation of a microwave system for brain stroke 3-D imaging. Diagnostics. (2021) 11(7):1232. doi: 10.3390/diagnostics11071232
110. Liu D, Wang S, Liu S, Wang Q, Che X, Wu G. Frontiers in sarcopenia: advancements in diagnostics, molecular mechanisms, and therapeutic strategies. Mol Aspects Med. (2024) 97:101270. doi: 10.1016/j.mam.2024.101270
111. Mattsson V, Ackermans LLGC, Mandal B, Perez MD, Vesseur MAM, Meaney P, et al. MAS: standalone microwave resonator to assess muscle quality. Sensors. (2021) 21(16):5485. doi: 10.3390/s21165485
112. Miranda-Fuentes C, Chirosa-Ríos LJ, Guisado-Requena IM, Delgado-Floody P, Jerez-Mayorga D. Changes in muscle oxygen saturation measured using wireless near-infrared spectroscopy in resistance training: a systematic review. Int J Environ Res Public Health. (2021) 18(8):4293. doi: 10.3390/ijerph18084293
113. Beever AT, Tripp TR, Zhang J, MacInnis MJ. NIRS-derived skeletal muscle oxidative capacity is correlated with aerobic fitness and independent of sex. J Appl Physiol. (2020) 129(3):558–68. doi: 10.1152/japplphysiol.00017.2020
114. Tandirerung FJ, Jamieson A, Hendrick E, Hughes AD, Jones S. Near-infrared spectroscopy (NIRS) in vivo assessment of skeletal muscle oxidative capacity: a comparison of results from short versus long exercise protocols and reproducibility in non-athletic adults. Front Physiol. (2024) 15:1429673. doi: 10.3389/fphys.2024.1429673
115. Shin J, Park E. Comparison between discrete multi-wavelength near-infrared spectroscopy and bioelectrical impedance analysis in the assessment of muscle mass for community-dwelling older people. J Clin Med. (2024) 13(8):2350. doi: 10.3390/jcm13082350
116. Mizukoshi K, Hamanaka Y, Niwayama M. Investigation of oxygen saturation in regions of skin by near infrared spectroscopy. Skin Res Technol. (2022) 28(5):695–702. doi: 10.1111/srt.13169
Keywords: sarcopenia, dual-energy x-ray absorptiometry, computed tomography, ultrasound imaging, magnetic resonance imaging, bioelectrical impedance analysis, quantitative parameters, non-ionizing radiation
Citation: Lavalle S, Scapaticci R, Masiello E, Messina C, Aliprandi A, Mario Salerno V, Russo A and Pegreffi F (2024) Advancements in sarcopenia diagnosis: from imaging techniques to non-radiation assessments. Front. Med. Technol. 6:1467155. doi: 10.3389/fmedt.2024.1467155
Received: 19 July 2024; Accepted: 13 September 2024;
Published: 9 October 2024.
Edited by:
Sridhar Poosapadi Arjunan, SRM Institute of Science and Technology, IndiaReviewed by:
Darren Player, University College London, United KingdomCopyright: © 2024 Lavalle, Scapaticci, Masiello, Messina, Aliprandi, Mario Salerno, Russo and Pegreffi. This is an open-access article distributed under the terms of the Creative Commons Attribution License (CC BY). The use, distribution or reproduction in other forums is permitted, provided the original author(s) and the copyright owner(s) are credited and that the original publication in this journal is cited, in accordance with accepted academic practice. No use, distribution or reproduction is permitted which does not comply with these terms.
*Correspondence: Rosa Scapaticci, c2NhcGF0aWNjaS5yQGlyZWEuY25yLml0