- 1Institute of Clinical Sciences, Sahlgrenska Academy, University of Gothenburg, Gothenburg, Sweden
- 2Department of Orthopaedics, Sahlgrenska University Hospital, Mölndal, Sweden
- 3Laboratory of Biomechanical Orthopedics, Ecole Polytechnlque Fédérale, Lausanne, Lausanne, Switzerland
- 4Department of Engineering Sciences, Division of Applied Mat’l. Science, Uppsala University, Uppsala, Sweden
- 5Biomimetic Innovations Ltd, Shannon, Ireland
This study proposes a novel dual adhesive approach for fixing osteochondral fractures, aiming to address the limitations of current fixation methods by incorporating both a bone adhesive (phosphoserine modified calcium phosphate cement PM-CPC) and a cartilage adhesive (methacrylated phosphoserine-containing gelatin MePGa hydrogel). The feasibility and efficacy of this approach were investigated using an ex vivo bovine knee model. Results indicate successful gluing of osteochondral cylinders with both adhesives, with no significant difference in adhesion strength between the groups (adhesion strength mean of 1211.6 kPa, SD 602.4 kPa, and mean of 1299.6 kPa, SD 850.9 kPa for groups 1 and 2 respectively). Importantly, the inclusion of the hydrogel component in the dual adhesive system aims to enhance cartilage repair potential, complementing the mechanical support provided by the bone adhesive. Each adhesive offers distinctive benefits: PM-CPC for mechanical support and bone repair, and MePGa hydrogel for cartilage repair. The study demonstrates the potential of the dual adhesive strategy for osteochondral repair, though further refinement and in vivo validation are needed.
1 Introduction
Osteochondral fracture fixation is traditionally performed in the acute setting with internal fixation procedures using pins or compression screws through the fragment. Outcomes have generally been good, but cartilage thinning, subchondral bone remodeling, and tissue reactions can occur after internal fixation through the fragment (Vogel et al., 2020). Some fragments might be too brittle to fixate and break during the fixation attempt.
Thus, the fixation of articular fractures is still an unmet need where a tissue-specific adhesive would be a useful adjunct to standard treatments. Whilst there are no such adhesives in current clinical use, preclinical animal models have demonstrated good healing of bone in unloaded models using an adhesive based on phosphoserine modified calcium phosphate cement (PM-CPC adhesive) (Procter et al., 2021). An ex-vivo human bone core model has shown that this adhesive bonds freshly harvested human bone (Bojan et al., 2022). Since osteochondral bone fragments have both bone and cartilage components, a dual adhesive strategy in which tissue-specific components are used to achieve optimal healing is a sound approach to achieve healing and tissue restoration.
Only few tissue adhesives, e.g., Dermabond ® and fibrin glue, are already successfully used in clinical practice but the need for tissue-specific adhesive properties based on polymers and hydrogels for vessels, lungs, eyes, dura has been recognized (Mondal et al., 2022). Fibrin-based adhesives have been utilized in both preclinical and clinical settings with limitations and mixed outcomes (Brittberg et al., 1997; Kaplonyi et al., 1988). It should be noted that the use of such biomaterials in bone fragment stabilization is considered “off label” and is done without any safety or efficacy data to support this indication. Phosphoserine and magnesium phosphate-based adhesives present strong bond strengths to bone, representing a notable advancement in mineral-organic bone adhesives (Renner et al., 2023). PM-CPC adhesive has shown strong tissue adhesion to animal bone under both in vivo and ex vivo laboratory conditions (Pujari-palmer et al., 2018; Procter et al., 2021). The adhesive formulation used in the current study consists of an amino acid–phosphoserine, alpha-TCP (tricalcium phosphate), calcium silicate, and water (Mondal et al., 2022). The safety and non-toxicity of the adhesive have been demonstrated in a subcutaneous murine model (Hulsart-billström et al., 2020), along with good bonding strength demonstrated in a novel ex vivo model based on murine femoral condyle bone core (Procter et al., 2019). Wu et al. obtained superior screw augmentation effects of the adhesive in osteoporotic human femoral head bones compared to a calcium phosphate cement (Wu et al., 2020). In a recent murine in vivo study with the bone adhesive, good bonding strength (estimated up to 14.4 MPa in cancellous bone) and uneventful fracture healing were shown over 6 weeks (Procter et al., 2021). In addition, long-term biocompatibility was shown in a lapine model for up to 52 weeks for a similar class of PMC adhesive where tetra-calcium phosphate was used (Kirillova et al., 2018). In a large animal (ovine) model, at 2 years PMC adhesive showed no adverse local effects, signs of infection, or cytotoxicity in tissues either at or adjacent to implantation sites (Foley et al., 2021).
Simultaneously, a hydrogel with adhesive properties in cartilage has been recently proposed (Karami et al., 2021; Karami et al., 2024). The development of effective treatments for cartilage lesions hinges on establishing intimate contact to surrounding tissue, a crucial parameter in repair processes (Karami et al., 2023; Karami et al., 2018). A biocompatible and adhesive hydrogel system is utilized here (Karami et al., 2021). The adhesive performance of the hydrogel was successfully demonstrated in the early follow-up phase in a goat model. The photopolymerizable bioadhesive, designed for direct injection into damaged areas, cures in situ while displaying inherent adhesiveness to cartilage. The polymeric backbone of the hydrogel, methacrylated phosphoserine-containing gelatin (MePGa), enables the formation of a hybrid network, facilitating rapid adhesion formation via a photo-initiation process utilizing visible light exposure. Drawing inspiration from biological systems, the design paradigm involves a two-step modification process to integrate cross-linkable and adhesive components within various polymeric backbones. With meticulous control over both interface and bulk properties, the hydrogels exhibit a robust adhesion mechanism, demonstrating the synergistic interplay between interfacial bonds and mechanical strength.
In response to the unmet need of stabilizing osteochondral fragments, a dual adhesive strategy can be a promising solution, wherein tissue-specific adhesives are utilized to address the unique requirements of both bone and cartilage. This strategy circumvents the limitations of universal adhesives, which are unlikely to provide optimal performance across the diverse properties of tissues (Shah and Meislin, 2013). As an obvious example a tissue glue for repair of tendon may need a low calcium content to avoid the risk of tissue calcification, whilst in bone a higher calcium content could be advantageous. In this context, the application of an adhesive hydrogel, specifically designed for soft tissues, in combination with the bone adhesive, could represent a significant advancement.
Recent research on osteochondral repair has explored bilayer systems that combine bone- and cartilage-specific biomaterials to enhance regeneration. For example, biphasic hydrogels have been designed to promote site-specific differentiation of stem cells into both cartilage and bone, using phase-specific drug release mechanisms (Liu et al., 2020). Additionally, bilayer scaffolds incorporating photocurable silk hydrogels have shown promise in improving lateral integration, a key challenge in cartilage regeneration (Wu et al., 2021). While these systems focus on scaffolds for tissue regeneration, our approach is distinct in its use of a dual adhesive strategy specifically designed to bond osteochondral fragments. By employing our hybrid adhesives for both bone and cartilage, our method addresses the need of osteochondral fragment stabilization.
The present study is an ex vivo bovine model development to assess the feasibility of using dual component adhesive at the osteochondral level on the path to an in vivo study. In this model, bone/cartilage cores harvested from the articular knee surface are glued in place with the adhesives. At the subchondral bone level, the phosphoserine modified calcium phosphate cement (PM-CPC) is used. At the cartilage level, a methacrylated phosphoserine containing gelatine (MePGa) hydrogel designed specifically for soft tissues is applied (Karami et al., 2021).
Standardized laboratory tests for musculoskeletal adhesives typically assess either cartilage or bone adhesives individually, but they do not account for the dual-tissue environment in osteochondral defects. As there are no tissue adhesives currently approved for musculoskeletal applications, the development of qualifying tests recognised by regulatory bodies is an essential next step for adhesive developers. In the absence of a universal standard test protocol for this context, we developed a custom protocol to evaluate adhesive stability and mechanical properties. This approach adapts elements of existing methods to meet the specific challenges of simultaneous bone and cartilage adhesion, enabling a more accurate assessment of the dual adhesive system’s performance.
2 Methods
The bovine osteochondral bone core model was adjusted according to the method used in human femoral heads [3]. Bovine femoral and tibial condyles were harvested from two fresh frozen specimens. The cores were prepared and glued back with bone adhesive (group 1, n=7), and in group 2 (n=7), the cores were glued back with bone and cartilage adhesives, respectively. The push-out strength was evaluated at 1 h after gluing.
2.1 Specimen preparation
One to two cores per condyle were obtained. Osteochondral cores were drilled (from the cartilage surface towards the subchondral bone) using a dental trepan drill (outside diameter of 8 mm and max. drilling depth 10 mm, Dentra Instruments Limited United Kingdom). The cores did not have the base in order to enable the push out tests, Figure 1A. Table 1 describes the core dimensions.
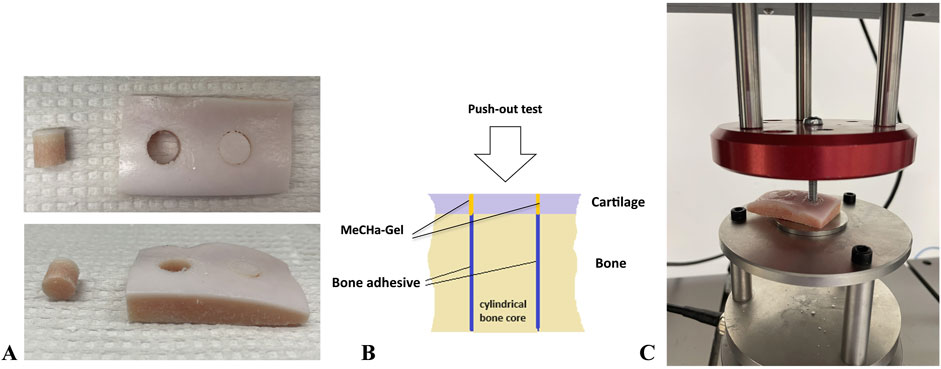
Figure 1. The mechanical setup for the push-out test. (A) The prepared samples, (B) dual adhesive model, (C) mechanical push-out setting.
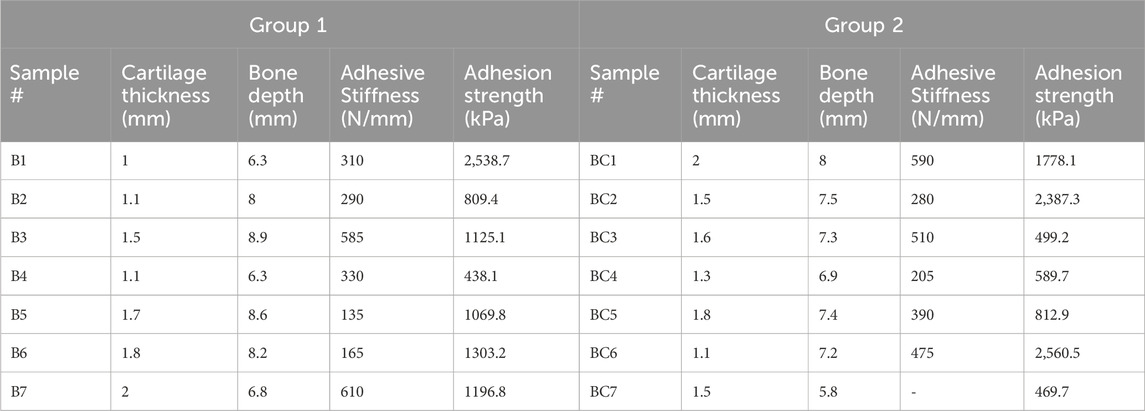
Table 1. Core dimensions of the osteochondral cores in the test groups. B represents the samples in group 1 (bone adhesive only) and BC represents group 2 (bone and cartilage adhesives).
2.2 Adhesion measurement
The samples were kept at room temperature throughout the experiment. In the group 1 (n=7), the bone cores were glued back in place using the PM-CPC bone adhesive as used in the previous study [3]. The powder components, alpha tricalcium phosphate and O-phospho-L-serine (Flamma AB), were mixed at a 30% molar ratio. The powders were then combined with the liquid, a 20% (w/v%) solution of trisodium citrate (Fluka), at a liquid-to-powder ratio of 0.25 mL/g. The adhesive was mixed for 20 s at room temperature by hand, and the whole volume (ca. 1 mL) was applied by spatula into the core cavity. The core was glued back in place whilst the condyle was placed on the smooth surface to ensure the original position of the core regarding the height. The overflow of the bone adhesive at the cartilage level was carefully removed with the help of the trephine drill, rotating the adhesive gently away by hand.
The adhesive procedure for the cores in group 2 (n=7) was identical with the addition of the hydrogel glue at the cartilage level after the bone glue had set (after 1 min), Figure 1B. The synthesis of MePGa-Gel, as outlined in our previous study (Karami et al., 2021), involves a two-step process. Gelatin methacryloyl is initially synthesized through the methacrylation process (methacrylation degree of 48%). Then, methacrylated gelatin is further modified by adding phosphoserine under EDC/NHS activation (3.5 mg mL−1). After filtration and dialysis, the resulting product is lyophilized to obtain MePGa polymer. The hydrogel precursor is fabricated by dissolving the lyophilized polymer and the LAP photo-initiator (6146, Tocris Bioscience) in PBS, with a final LAP photo-initiator concentration of 0.02% w/v in the resulting solution. After injection of the hydrogel into the gap at the cartilage level, the precursor mixture was exposed to light with a wavelength of 405 nm and intensity of 5 mW cm2 to polymerize and cross-link the polymeric network for 1 min.
2.3 Mechanical testing
The adhesive samples in both groups were tested for push-out strength after 1 h of gluing using an Instron E3000 linear mechanical testing machine (Norwood, MA, USA) equipped with a 3 kN load cell. The samples were placed on the base with a circle in the middle allowing for the push-out of the cylinder, the screw attached to the load-cell pressed on the cartilage side of the cylinder, Figure 1C. The bone core cylinders were pushed-out at a constant speed of 1 mm/min. The peak load obtained is defined as the push-out force (N). The adhesion strength was calculated by dividing the maximum obtained push-out force by the nominal contact area.
2.4 Statistical analysis
Statistical analysis was performed using a two-tailed t-test to assess the significance of differences between the two compared groups in the adhesion strength performance. Results were considered statistically significant at a threshold of p < 0.05. Data are presented as means ± standard deviation of the mean (SD).
3 Results
3.1 Feasibility of the dual adhesive procedure
In both groups, gluing the osteochondral cylinders in the bovine knee was possible and uneventful. Removing the excess of PM-CPC adhesive from the cartilage level did not pose any difficulties. The hydrogel adhesive was applied, cured, and adhered to the surface of the cartilage up until the mechanical testing.
3.2 Mechanical properties
All the specimens failed through the adhesive interface. The force-displacement characteristic was consistent with this observation as the bone adhesive is a strong, rigid, and brittle material.
The peak push-out force ranged from 77 N to 452 N (with an adhesion strength mean of 1211.6 kPa, SD 602.4 kPa) for group 1 and 83 N–521 N (with an adhesion strength mean of 1299.6 kPa, SD 850.9 kPa) for group 2. The difference was not statistically significant between the groups, as shown in Figure 2.
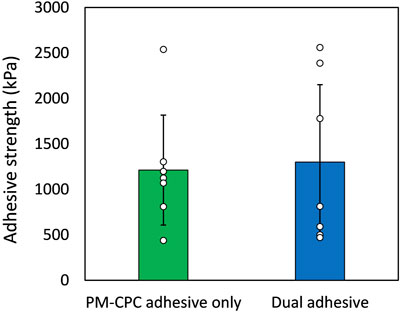
Figure 2. Adhesion strength performance of the dual adhesive system for gluing osteochondral cylinders (n=7 in each group). Data represent means and standard deviation of the mean for two compared groups, demonstrating no significant statistical difference.
The stiffness for group 1, PM-CPC adhesive-only, was 346.4 N/mm (SD 172.7 n=7) whilst that estimated from the force displacement curves shown in Bojan et al. (2022) at 2 h was 175 N/mm (please see Figure 3). Taking into account that the former is bovine and the latter human bone, the ratio of these magnitudes are consistent with the ratio of reported Young’s modulus (132.9 ± 52.3 versus 80.0 ± 37.3 MPa) (Poumarat and Squire, 1993).
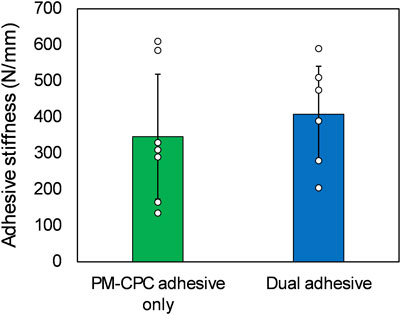
Figure 3. Adhesive stiffness performance of the dual adhesive system for gluing osteochondral cylinders (n=7 for group 1, n=6 for group 2, one data point was excluded as an outlier using the z-score method (z score 2.1). Data represent means and standard deviation of the mean for two compared groups, no significant statistical difference was observed.
4 Discussion
We presented the bovine model that demonstrated the feasibility of combining the two tissue-specific adhesive methods for the fixation of osteochondral fragments. This is thought to be the first model to explore such a concept.
The model showed that once the subchondral component is glued, the PM-CPC adhesive intruding into the cartilage gap can be removed before applying the cartilage adhesive. This enabled the MePGa adhesive to be injected between the cut cartilage edges and subsequently light-cured. The 1 MPa strength of the PM-CPC adhesive bonded core is sufficient for immediate stabilization of a bone fragment, and may be sufficient for load bearing but this has to be verified in an in vivo study. The addition of the MePGa adhesive did not add to the strength of the core. This two-stage gluing method is demanding, and an in vivo pilot will be necessary to perfect and prove the operative technique for applying both adhesives simultaneously in a wet field.
Whilst the concept of using the same adhesive throughout all tissue types is attractive it is inevitable that different tissue would have different property requirements. For example, the need for transparency for an adhesive designed for eye lens attachment may preclude adhesives that have significant calcium content. The PM-CPC adhesive and the MePGa adhesive used in the present study have both shown some effects in tissue healing, the former in bone and the latter in cartilage. Looking forward to the planned in vivo evaluation of the dual adhesive model it will be important show that there is no initial displacement of the glued bone core under loading, and that the core load bearing capacity is sustained without displacement until completely healed. This will enable further evaluation of the effects of the MePGa in a stable cartilage gap.
Many musculoskeletal bone adhesive candidates have been proposed however no bone adhesive candidate has been approved for clinical use. These candidates include numerous similar calcium-based bone void fillers, but these are designated as non-load-bearing and are contraindicated in fractures that extend into joints. This regulatory designation precludes their use in bonding of osteochondral bone fragments. The current regulatory environments in both US and Europe, are very significant barriers to new biomaterials that have no predicate device. That said the FDA has granted (2023) the bone adhesive used so called “breakthrough technology” designation which refers to a medical device that may provide a more effective treatment than the current standard of care. It is anticipated that a dual adhesive strategy will have an even more burdensome regulatory path in which the interactions between adjacent adhesives and their targeted tissues will have to prove both safety and efficacy.
5 Conclusion
In this study, we developed and evaluated a novel dual adhesive approach for fixing osteochondral fractures. By utilizing a bone adhesive (PM-CPC) at the subchondral bone level and a cartilage adhesive (MePGa hydrogel) at the cartilage level, we aimed to address the challenges associated with current fixation methods. Our results demonstrate the feasibility of this approach in an ex vivo bovine knee model, with successful gluing of osteochondral cylinders and comparable adhesion strength between groups. However, challenges such as the demanding nature of the two-stage gluing method and the need for in vivo validation remain. Further refinement and testing are necessary to optimize and validate this promising dual adhesive strategy for osteochondral repair.
Data availability statement
The raw data supporting the conclusions of this article will be made available by the authors, without undue reservation.
Ethics statement
Ethical approval was not required for the study involving animals in accordance with the local legislation and institutional requirements because this was an ex-vivo study conducted of fresh bovine bone obtained from an abattoir.
Author contributions
AB: Conceptualization, Formal Analysis, Investigation, Methodology, Writing–original draft, Writing–review and editing. PK: Conceptualization, Data curation, Formal Analysis, Investigation, Methodology, Visualization, Writing–original draft, Writing–review and editing. PP: Conceptualization, Methodology, Project administration, Supervision, Writing–review and editing. DP: Conceptualization, Methodology, Project administration, Supervision, Writing–review and editing.
Funding
The author(s) declare that financial support was received for the research, authorship, and/or publication of this article. This work was supported by the EPFL Innogrants, EPFL Switzerland.
Acknowledgments
The authors thank Sandra Jaccoud for providing bovine knees.
Conflict of interest
Author PP declares partial ownership in a company that owns all related intellectual property Biomimetic Innovations Ltd, Shannon, Ireland.
The remaining authors declare that the research was conducted in the absence of any commercial or financial relationships that could be construed as a potential conflict of interest.
Publisher’s note
All claims expressed in this article are solely those of the authors and do not necessarily represent those of their affiliated organizations, or those of the publisher, the editors and the reviewers. Any product that may be evaluated in this article, or claim that may be made by its manufacturer, is not guaranteed or endorsed by the publisher.
References
Bojan, A. J., Stadelmann, V. A., Wu, D., Pujari-Palmer, M., Insley, G., Sundh, D., et al. (2022). A new bone adhesive candidate-does it work in human bone? An ex-vivo preclinical evaluation in fresh human osteoporotic femoral head bone. Injury 53, 1858–1866. doi:10.1016/j.injury.2022.04.007
Brittberg, M., Sjögren-Jansson, E., Lindahl, A., and Peterson, L. (1997). Influence of fibrin sealant (Tisseel®) on osteochondral defect repair in the rabbit knee. Biomaterials 18, 235–242. doi:10.1016/s0142-9612(96)00117-2
Foley, K. T., Woodard, E. J., Slotkin, J. R., Mayotte, C. K., Baldwin, A. C., Brown, M. C., et al. (2021). Cranial flap fixation in sheep using a resorbable bone adhesive. J. Neurosurg. 134, 621–629. doi:10.3171/2019.11.jns192806
Hulsart-billström, G., Stelzl, C., Procter, P., Pujari-Palmer, M., Insley, G., Engqvist, H., et al. (2020). In vivo safety assessment of a bio-inspired bone adhesive. J. Mater. Sci. Mater. Med. 31, 24–10. doi:10.1007/s10856-020-6362-3
Kaplonyi, G., Zimmerman, I., Frenyo, A., Farkas, T., and Nemes, G. (1988). The use of fibrin adhesive in the repair of chondral and osteochondral injuries. Injury 19, 267–272. doi:10.1016/0020-1383(88)90043-5
Karami, P., Martin, R., Laurent, A., Nam, H. Y., Philippe, V., Applegate, L. A., et al. (2024). An adhesive hydrogel technology for enhanced cartilage repair: a preliminary proof of concept. Gels 10, 657. doi:10.3390/gels10100657
Karami, P., Nasrollahzadeh, N., Wyss, C., O'Sullivan, A., Broome, M., Procter, P., et al. (2021). An intrinsically-adhesive family of injectable and photo-curable hydrogels with functional physicochemical performance for regenerative medicine. Macromol. rapid Commun. 42, 2000660. doi:10.1002/marc.202000660
Karami, P., Stampoultzis, T., Guo, Y., and Pioletti, D. P. (2023). A guide to preclinical evaluation of hydrogel-based devices for treatment of cartilage lesions. Acta Biomater. 158, 12–31. doi:10.1016/j.actbio.2023.01.015
Karami, P., Wyss, C. S., Khoushabi, A., Schmocker, A., Broome, M., Moser, C., et al. (2018). Composite double-network hydrogels to improve adhesion on biological surfaces. ACS Appl. Mater. and interfaces 10, 38692–38699. doi:10.1021/acsami.8b10735
Kirillova, A., Kelly, C., VON Windheim, N., and Gall, K. (2018). Bioinspired mineral–organic Bioresorbable bone adhesive. Adv. Healthc. Mater. 7, 1800467. doi:10.1002/adhm.201800467
Liu, X., Chen, Y., Mao, A. S., Xuan, C., Wang, Z., Gao, H., et al. (2020). Molecular recognition-directed site-specific release of stem cell differentiation inducers for enhanced joint repair. Biomaterials 232, 119644. doi:10.1016/j.biomaterials.2019.119644
Mondal, P., Chakraborty, I., and Chatterjee, K. (2022). Injectable adhesive hydrogels for soft tissue reconstruction: a materials chemistry perspective. Chem. Rec. (New York, N.Y.) 22, e202200155. doi:10.1002/tcr.202200155
Poumarat, G., and Squire, P. (1993). Comparison of mechanical properties of human, bovine bone and a new processed bone xenograft. Biomaterials 14, 337–340. doi:10.1016/0142-9612(93)90051-3
Procter, P., Hulsart-Billström, G., Alves, A., Pujari-Palmer, M., Wenner, D., Insley, G., et al. (2021). Gluing living bone using a biomimetic bioadhesive: from initial cut to final healing. Front. Bioeng. Biotechnol. 9, 728042. doi:10.3389/fbioe.2021.728042
Procter, P., Pujari-Palmer, M., Hulsart-Billström, G., Wenner, D., Insley, G., Larsson, S., et al. (2019). A biomechanical test model for evaluating osseous and osteochondral tissue adhesives. BMC Biomed. Eng. 1, 11–19. doi:10.1186/s42490-019-0011-2
Pujari-palmer, M., Guo, H., Wenner, D., Autefage, H., Spicer, C. D., Stevens, M. M., et al. (2018). A novel class of injectable bioceramics that glue tissues and biomaterials. Materials 11, 2492. doi:10.3390/ma11122492
Renner, T., Otto, P., Kübler, A. C., Hölscher-Doht, S., and Gbureck, U. (2023). Novel adhesive mineral-organic bone cements based on phosphoserine and magnesium phosphates or oxides. J. Mater. Sci. Mater. Med. 34, 14. doi:10.1007/s10856-023-06714-6
Shah, N. V., and Meislin, R. (2013). Current state and use of biological adhesives in orthopedic surgery. Orthopedics 36, 945–956. doi:10.3928/01477447-20131120-09
Vogel, L. A., Fitzsimmons, K. P., and Pace, J. L. (2020). Osteochondral fracture fixation with fragment preserving suture technique. Arthrosc. Tech. 9, e761–e767. doi:10.1016/j.eats.2020.02.018
Wu, D., Pujari-Palmer, M., Bojan, A., Palmquist, A., Procter, P., Öhman-Mägi, C., et al. (2020). The effect of two types of resorbable augmentation materials–a cement and an adhesive–on the screw pullout pullout resistance in human trabecular bone. J. Mech. Behav. Biomed. Mater. 110, 103897. doi:10.1016/j.jmbbm.2020.103897
Keywords: articular fracture, cartilage, subchondral bone, hydrogel, calcium-phosphate cement
Citation: Bojan AJ, Karami P, Procter P and Pioletti DP (2024) Gluing osteochondral fragments: development of a novel strategy for dual adhesive application in a preclinical model. Front. Med. Eng. 2:1484232. doi: 10.3389/fmede.2024.1484232
Received: 21 August 2024; Accepted: 29 October 2024;
Published: 12 November 2024.
Edited by:
Clara Mattu, Polytechnic University of Turin, ItalyReviewed by:
Piergiorgio Gentile, Universitat Politècnica de València, SpainSourav Mandal, University of Liège, Belgium
Copyright © 2024 Bojan, Karami, Procter and Pioletti. This is an open-access article distributed under the terms of the Creative Commons Attribution License (CC BY). The use, distribution or reproduction in other forums is permitted, provided the original author(s) and the copyright owner(s) are credited and that the original publication in this journal is cited, in accordance with accepted academic practice. No use, distribution or reproduction is permitted which does not comply with these terms.
*Correspondence: Alicja J. Bojan, YWxpY2phLmJvamFuQHZncmVnaW9uLnNl