- 1Department of Pulmonary Medicine, Tehran University of Medical Sciences, Tehran, Iran
- 2School of Mechanical Engineering, College of Engineering, University of Tehran, Tehran, Iran
- 3Pediatric Urology and Regenerative Medicine Research Center, Children’s Medical Center, Pediatric Center of Excellence, Tehran University of Medical Sciences, Tehran, Iran
- 4Tracheal Diseases Research Center, National Research Institute of Tuberculosis and Lung Diseases (NRITLD), Shahid Beheshti University of Medical Sciences, Tehran, Iran
- 5Institut de Recherche Robert Sauvé en Santé et en Sécurité du Travail, Montréal, QC, Canada
Recently, endotracheal stenting has become critical in treating respiratory diseases. Due to the COVID-19 pandemic in recent years, many patients had stenosis because of long-term intubation, and silicone stents can be used to treat tracheal stenosis in these patients. Standard airway stents are silicone tubes that provide immediate relief but are prone to migration. In this work, we design different silicone stents and analyze them in the trachea to evaluate silicone airway stents’ performance to overcome undesired migration. A finite-element model of the trachea was employed to evaluate anti-migration forces in each stent. The geometry of the trachea is brought from a computerized tomography scan of the chest of a 68-year-old healthy man. The results are shown based on the least migration of stents based on anti-migration forces. Also, the conditions of stent placement have been considered based on two different assumed friction factors, and the importance of choosing the type of silicone for stent construction has been analyzed. The results show that increasing the diameter of the stent reduces the displacement and migration of it in the trachea. Furthermore, the 23 mm stent with a 45° angle revealed the best implementation against compression under the impact of respiratory pressure differences.
1 Introduction
Recently, in the wake of diseases like coronavirus, a large number of researchers have focused on the airway stent that has been applied during tracheomalacia. The placement of airway stents has come foremost in treating tracheal stenosis such as benign and malignant tracheobronchial diseases since 1915 (Walser, 2005). Tracheal stenosis is expressed by structural tracheal constriction, and it can progress from many reasons, including external injury to the trachea, benign or malignant tumor, relapsing polychondritis, sarcoidosis, and infections (Ho and Koltai, 2008; Alzahrany and Banerjee, 2015). An airway stent has a significant role in healing or controlling the airway trachea, and it can support the airway wall against collapse or external compression (Folch and Keyes, 2018). Airway stents can be classified into four categories: silicone, balloon-dilated metal stent, self-expanding metal stent, and covered self-expanding metal stent (Chin et al., 2008). One of the advantages of metal stents is that they have less migration compared to silicone stents, but their main disadvantage is that they are difficult to remove, which makes it necessary to use silicone stents (Saad et al., 2003; Bolliger et al., 2004; Mughal et al., 2005). The ideal airway stent should 1) be easy to place and remove; 2) be large enough to maintain the position and not migrate; 3) not be very large and be able to avoid granulation tissue reactions; 4) be flexible enough to act like airway physiology but have the sufficient firmness to resist extrinsic compression, and 5) not affect mucociliary clearance (Guibert et al., 2020). The properties of airway stents depend on their materials, shapes, and other characteristics (Petersen et al., 1995). Silicone stents by customization and dimensional design can prevent migration and achieve a better fit (Zakaluzny et al., 2003). In contrast to metal stents, silicone stents have various hardness and flexibilities, have the ability to achieve greater mechanical strength by reinforcement, are less expensive, and are easily manufactured by additive manufacturing (Colpani et al., 2020).
One procedure to improve tracheal stents’ geometry and performance is using finite-element methods to simulate and analyze the effect of structural parameters. The first implantation was performed in the 1980s to overcome and treat narrowed or blocked coronary arteries (Dumoulin and Cochelin, 2000). Due to the incomplete attachment of balloon-expandable stents to the aortic wall, which caused undesired migration, self-expanding nitinol aortic stents were presented (Tyagi et al., 1999). The self-expanding nitinol stent can be easily crimped below room temperature, and when it arrives at the vessel wall, it expands until it hits the vessel wall and fits into it (Lally et al., 2005). In particular, limitations of self-expanding stents, such as difficult adjustment, tissue (tumor) ingrowth, and being a permanent and irremovable cause to silicone stents, have been discussed in the recent decade (Saito and Imamura, 2005; Ruan et al., 2018).
Gildea et al. (2006) modified the silicone stent by presenting the Polyflex stent, which had a thinner wall and could be more easily removed if required. Dutau et al. (2010) published the clinical efficacy and safety of silicone stents in anastomotic complications of lung transplantation and concluded that silicone stents are acceptable and effective for airway disorders. Stents must be able to respond to cough and breathing pressures by undergoing reversible reduction of the cross-sectional area. Considering the breathing and coughing processes in the healthy trachea after stent implantation is necessary to design better-adapted and personalized stents. Computational modeling of the stenting procedure provides a powerful tool for predicting the patient’s possible risk of a specific stent (Rouhani et al., 2020). Malvè et al. (2011), in order to analyze human tracheal conformability after stent implantation, developed a finite-element model of the trachea based on a computerized tomography (CT) scan under normal breathing and coughing pressure, and they showed that the stent could prevent tracheal muscle deflections, particularly during coughing. Ratnovsky et al. (2015) performed numerical simulations for eight different stent geometries inserted into trachea models. They demonstrated a clear interrelation between the stent diameter and the stress applied to the trachea wall, and silicone stents produced the lowest levels of stress, which explained their predilection to migration. Luo et al. characterized the inspiratory flow and modeled the human lung by employing a CT scan. Zhang et al. (2016) used the CT scan to reconstruct 3D human tracheal stenosis. They implanted the stent and then analyzed the contact between the trachea wall and the implanted stent using finite-element methods. They have shown stress concentration after stenting is directly linked to granulation tissue. Safshekan et al. evaluated the mechanical properties of the human trachea, cartilage, connective tissue, and smooth muscle using uniaxial tensile tests and found that Yeoh and Mooney–Rivlin models were best able to describe the hyperelastic behavior of all three tracheal components (Safshekan et al., 2016). Hollister et al. (2017) evaluated non-linear finite-element models to obtain the effect of malacic segment length, tracheal diameter, and reduction in tissue mechanical properties on the mechanism and intensity of tracheal collapse under exhalation pressure. A linear viscoelastic constitutive model was developed to determine the crimping behavior of a viscoelastic polymeric braided stent and demonstrated the possibility of predicting the behavior of the braided stent during crimping and uncramping process (Shanahan et al., 2017). Vearick et al. (2018) proposed a fiber-reinforced silicone for tracheobronchial stents to analyze the reinforcement test for silicone with three different fibers and to compare these fibers in terms of their mechanical properties. McGrath et al. (2016) presented the mechanical effects that adhered covers may have on stent performance. Zheng et al. (2019) utilized the finite-element method to obtain the optimum design of braided stents. They characterized the effects of the geometrical parameter in self-expanding stents, such as crossing pattern, braiding angles, wire diameters, and strand numbers, on the mechanical performance. In addition, both the geometry and contact issues in self-expanding stents were analyzed by importing three braided stent models in a finite-element code and used to compare the response of different contact modeling techniques to different idealized boundary conditions (Malvè et al., 2011).
This study aimed to evaluate silicone airway stents’ performance to overcome undesired migration. In this study, the simulated trachea has been performed based on experimental data, which was reported by Kamel et al. (2009). In order to optimize the airway stent, different stents were evaluated considering parameters such as diameter, hollow or solid type, and angle of the stud. In addition, the effect of the friction factor was also investigated. In all these cases, anti-migration forces are considered our benchmark. Moreover, the effect of the stent design parameters on stent removal and its undesirable displacement due to the difference in respiratory pressure is analyzed under radial and axial (longitudinal) forces.
2 Materials and models
Silicone airway stents were designed to minimize migration, mucostasis, and granulation tissue formation. Since the shape and size of the trachea depend on age and sex, a trachea was considered our fixed criterion. Then, the silicone stents and their types used in this research are mentioned.
2.1 Solid model of the trachea
The trachea is a cartilaginous tube structure in the human body that attaches the larynx to the bronchi of the lungs, which allows airflow to pass through. In order to simulate the implantation of different stents under distinct conditions in the trachea, a finite-element method was used. The geometrical model of the human trachea was made based on the high-resolution CT scan of the chest from 40 males and 20 females with an age range of 22–88, as reported in morphometric studies (Kamel et al., 2009). All patients had healthy trachea with no distorting intrathoracic pathology and used a standardized breath-holding technique for performing scans. The finite-element model of the human trachea in this research was made based on a CT scan performed, as reported in Table 1 and Figure 1.
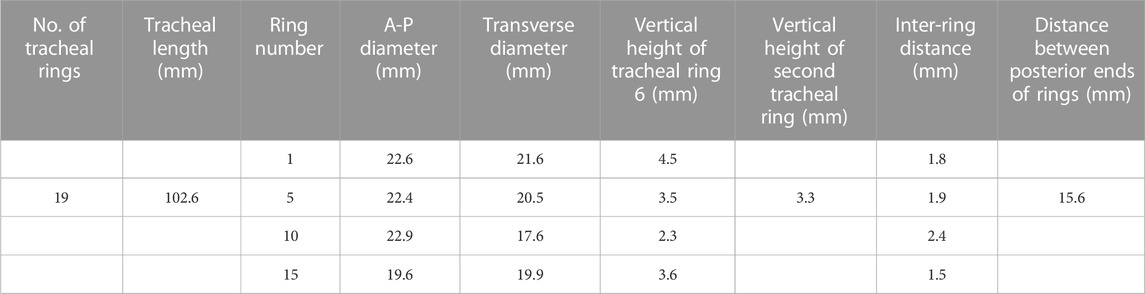
TABLE 1. Tracheal dimensions from a computerized tomography scan of the chest of a 68-year-old healthy man were used to simulate the cartilage and muscular membrane. For tracheal dimensions, see the work of Kamel et al. (2009).
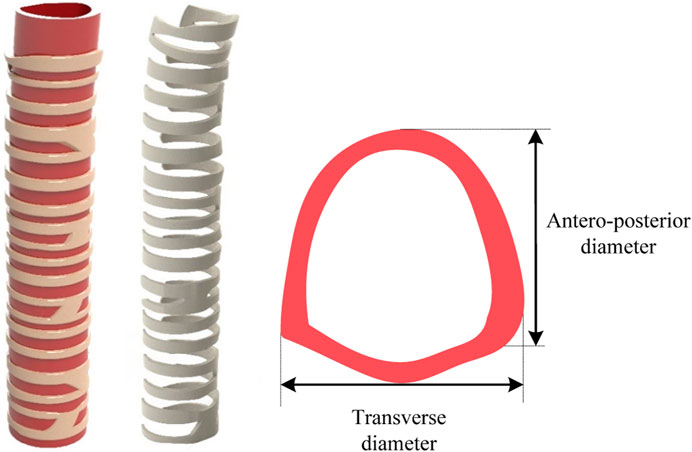
FIGURE 1. Geometrical dimension of the human trachea on a 68-year-old healthy man with 19 rings (Kamel et al., 2009). A commercial software SolidWorks was utilized to create a 3D tracheal model with 19 rings of 102.6 mm in length.
Finally, with the software ABAQUS, a full four-node linear tetrahedron mesh of approximately 101,992 elements for the healthy trachea was generated. For the thickness, delicate variations along the tracheal axis were seen and, therefore, chosen to model it as a constant.
The tracheal components comprise three parts: cartilage, smooth muscle, and connective tissue, and each part has different mechanical properties. Safshekan et al. (2016) suggested that every three parts are considered incompressible and isotropic hyperelastic tissues. Moreover, the Yeoh hyperelastic model was used to determine coefficients for tracheal tissues, where Ci are material constant coefficients and I1 is the first invariant of the Cauchy–Green tensor defined by
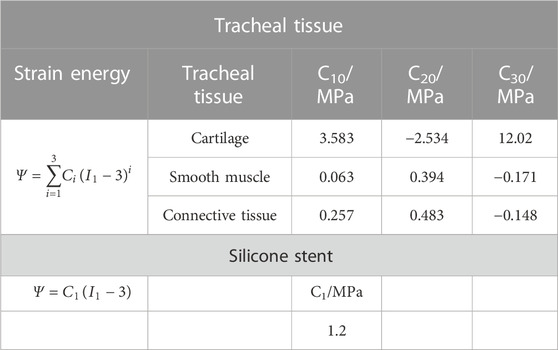
TABLE 2. Material parameters of tracheal tissues borrowed from the work of Safshekan et al. (2016) and stent based on the work of Larson (2016).
In this research, different stents were implanted using one tracheal model, and the result may vary in other dimensions of other human trachea. The top and bottom surfaces of the trachea are fixed when placing the trachea. However, when we want to remove it, we deactivated the top fixed surface and only the bottom fixed boundary remained to capture the behavior of the trachea.
2.2 Stent design
Airway stents prevent tracheal obstruction, such as bronchogenic carcinoma and malignant central airway diseases, and enhance pulmonary function in patients suffering from airway obstruction (Lee et al., 2017). When designing stents, advantages such as minimal granulation, prevention of migration, resisting external compression, and ease of removal must be considered. A silicone stent is a tube-like device that is widely used in airway obstruction due to its benefits, such as low cost and easy removability. The outside surface of the silicone stent maintains small studs to prevent proximal or distal migration into the trachea (Dumon, 1990). In order to obtain an optimized stent, 64 different studded silicone stents were designed and compared. As shown in Figure 2, in the center of half of the designed stent models, a hole was created into the studs to prevent mucus plugging and decrease the radial force embedding the stent into the surrounding tissue. These holes allow mucus to pass through them and decrease the radial displacement of stents under breathing pressure. Therefore, 64 designed models were simulated with four different inner diameters and stud angles, including hollow studs and solid studs. The internal diameter, length, and thickness of the stents are reported in Table 3.
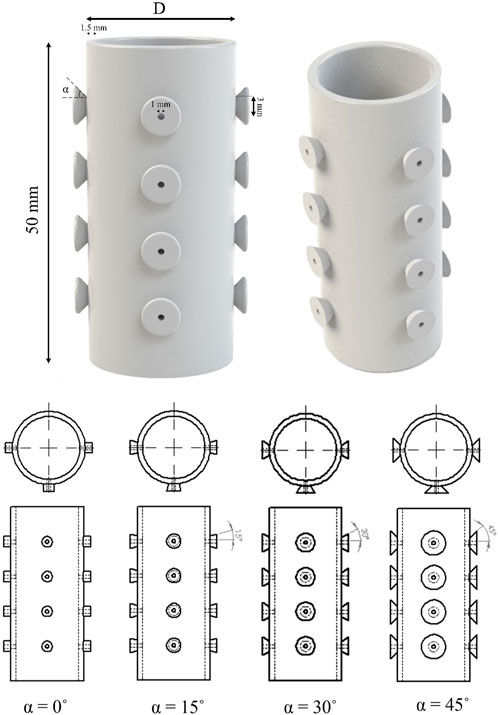
FIGURE 2. Proposed design of the silicone stent with hollow studs and various dimensional parameters. Stent length remains constant throughout the study, and a parametric study investigates different stent diameters (20, 21, 22, and 24 mm) and stud angles (0˚, 15˚, 30˚, and 45°).
Larson (2016) represented the methodology to determine the properties of silicone stents. The stents were considered hyperelastic and incompressible, and a neo-Hookean model was used (Bakhtiyari et al., 2023a; Bakhtiyari et al., 2023b). In this function, I1 is the first invariant of the Cauchy–Green tensor as explained earlier and C1 is the material constant. The coefficient for silicone stents in the current study is shown in Table 2 for shore 48. It should be noted that the penalty friction model has been implemented, and the effect of different friction coefficients has been studied.
Finally, stents’ CAD files imported in ABAQUS and a full four-node linear tetrahedron mesh of approximately 24,457 elements for each stent were generated. In all cases mentioned, anti-migration forces are investigated. In addition, the effects of coughing and sneezing were considered by applying external pressure to stents.
3 Results and discussion
The pressure difference between breathing and coughing in distinct sections of the trachea extracted stents from the trachea. In addition, a pressure difference between the inner and outer stent wall might reduce the diameter and cause stent disposal out of the trachea. The resulting behavior is significantly different in each case. Results corresponding to case 1 are shown in Figure 3. According to the previous section, three parameters for designing stents are considered: diameter, the angle of studs, and existing holes in studs, and each parameter influences stent displacement and needed pressure to anti-migration forces resistance stents into the trachea. In addition, the results for the healthy trachea are considered after stent implantation. The distribution of von Mises stress at the stent and trachea during the growth of the silicone stent while breathing in the healthy trachea is shown in Figure 3. Even though this design structure might not conform precisely to the patient’s actual occasions, utilizing different models facilitates the mechanical aspects of silicone stent function, preventing lung injuries (Walser, 2005; Thornton et al., 2006; Dutau and Dumon, 2017).
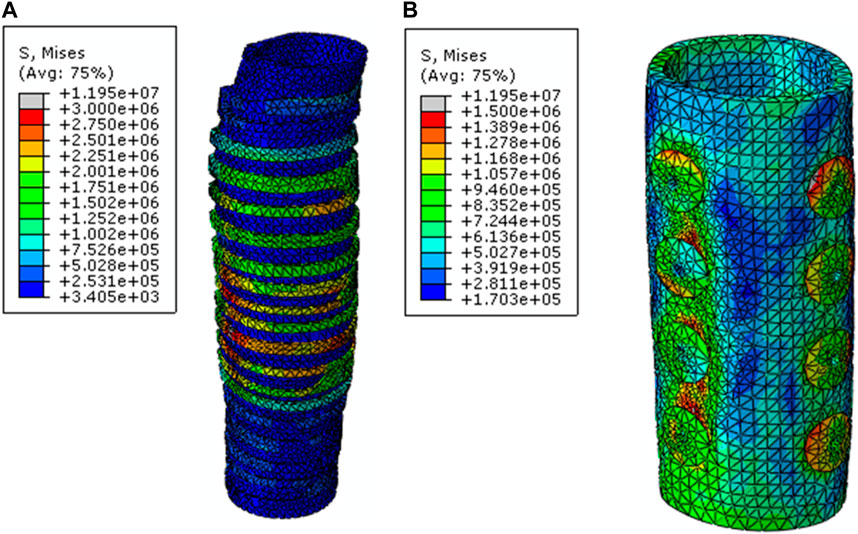
FIGURE 3. von Mises stress distribution (in Pa) at (A) the trachea and (B) stent during the growth of the silicone stent. Diameter of the stent is 23 mm, the angle of the studs is 45°, and the friction factor is assumed to be 0.1.
As shown in Figure 4, the longitudinal forces for removing the stent from the trachea are compared in different diameters and other parameters are assumed to be constant.
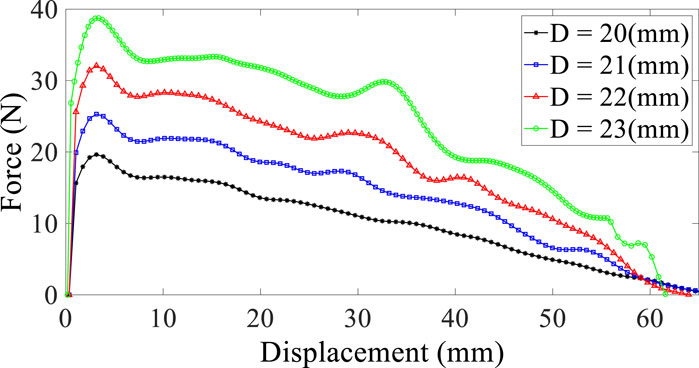
FIGURE 4. Reaction force–displacement curve for different diameters; stud angle is 30° (solid studs), and the friction factor is assumed to be 0.1.
As predicted, individually increasing the diameter of stents increases the force required to remove the stent from the trachea, and the possibility of displacement and migration in larger diameters decreases. Thus, selecting a larger diameter of the stent is also appropriate to avoid patient discomfort. Furthermore, it should be considered that deploying a stent in a larger diameter can cause injury to the tracheal wall and may be a risk factor for long-term usage (Golden and Hellenbrand, 2007).
As explained in the previous section, the placement of studs on the external stent tube wall prevents migration because of good contact pressure between the airway wall and the studs. However, it is not ideal for tracheobronchomalacia or to bridge tracheoesophageal fistula (Lee et al., 2016). In this study, an angle has been defined for the studs, which changes the contact surface between the tracheal wall and the stent, and then, its effects on reaction force have been analyzed (Figure 2). The angle is indicated by α, and its value varies between 0, 15°, 30°, and 45°. The result corresponding to the effect of stud angle difference on reaction forces for removing stent from the trachea is shown in Figure 5.
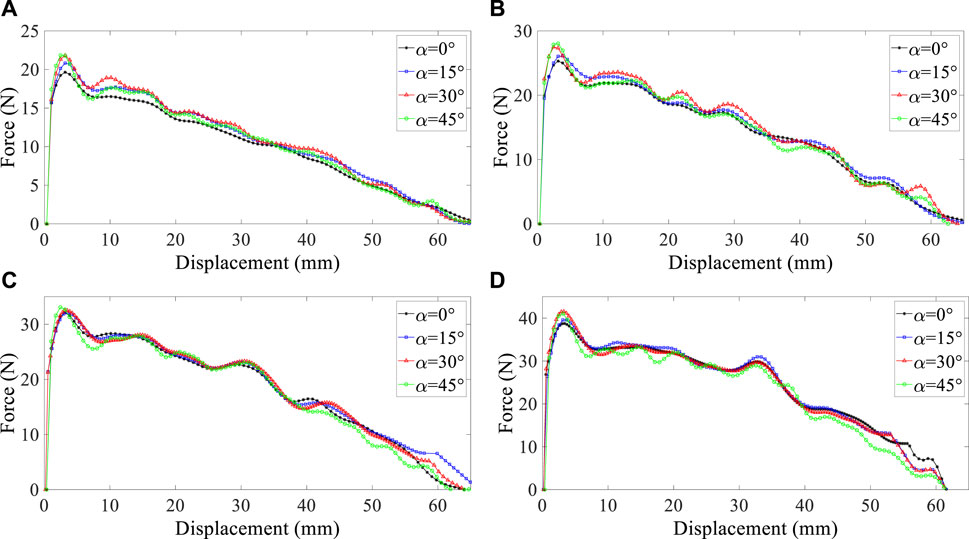
FIGURE 5. Reaction force–displacement curve for distinct angles in four diameters: (A) 20 mm, (B) 21 mm, (C) 22 mm, and (D) 23 mm; solid stud and friction factor is assumed to be 0.1.
Reaction force measurements have been reported based on stud angle (Figure 5), improved mainly by increasing the angle from 0° to 45° because the contact region between the tracheal wall and stent increased. The results show that existing studs with a higher angle can improve the function implantation of a prosthesis, especially at the angle of 45°; pressure difference, which causes displacement at coughing, can be controlled. When considering the impact of influential factors on stent migration, it is essential to mention that the influence of angle is less significant than the effectiveness of diameter on stent migration.
As mentioned in the previous section, the placement of hollow studs on stents makes mucus feasible to pass through them and then prevent mucus plugging. In order to analyze the effect of having a hole on studs, reaction forces were determined and reported in Figure 6.
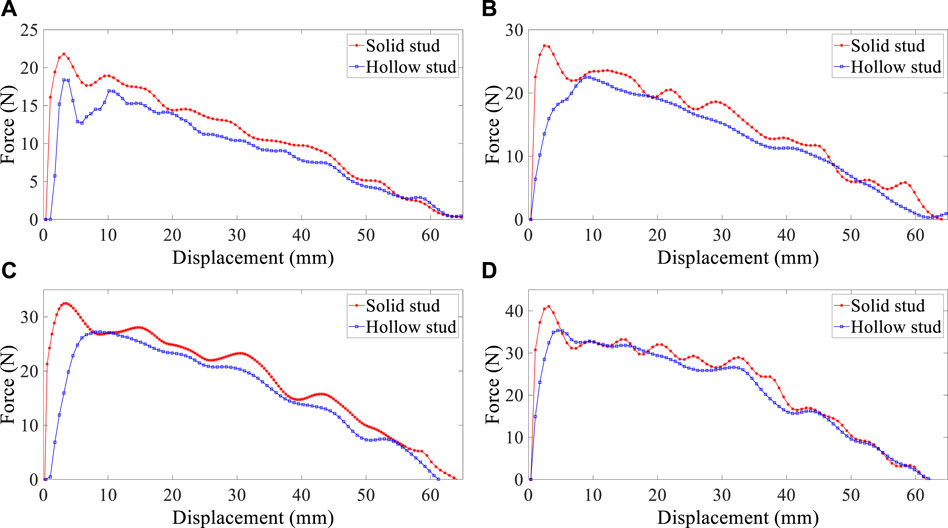
FIGURE 6. Reaction force–displacement curve for solid and hollow studs in four diameters: (A) 20 mm, (B) 21 mm, (C) 22 mm, and (D) 23mm; friction factor is assumed to be 0.1, and α is 30°.
Figure 6 shows that hollow studs reduce reaction forces and cause stent displacement easier than solid studs. However, this decrease is insignificant, and as for hollow stud benefits, this reduction force can be deficient. Furthermore, it is evident that this reduction differential at reaction forces is more significant in the larger diameter of the stent. In addition to the function of mucus, the presence of holes in studs decreases the pressure difference between breathing pressure and coughing pressure that is being applied to the stent. The mucus may build-up in the airways and obscures bronchoscopic visualization (Xu et al., 2019). In the case of silicone stents, immediate intubation using the hollow stud stents, passing distal of the stenosis, and cleaning the distal airways of pus and mucus can be lifesaving. Hence, although the presence of holes on silicone stents increases a slight probability of undesirable migration, it reduces the concern of mucus plugs.
According to the silicone properties, friction should be on the silicone stent’s contact surface and the trachea wall, which prevents the stent from coming out of the lungs due to the pressure difference. In previous analytical works, the effect of friction was not considered, which contradicts the reality of the human respiratory system. As illustrated in Figure 7 in this study, two small friction factors have been assumed to estimate the effect of changing the friction factor on the reaction forces: 0.1 and 0.05.
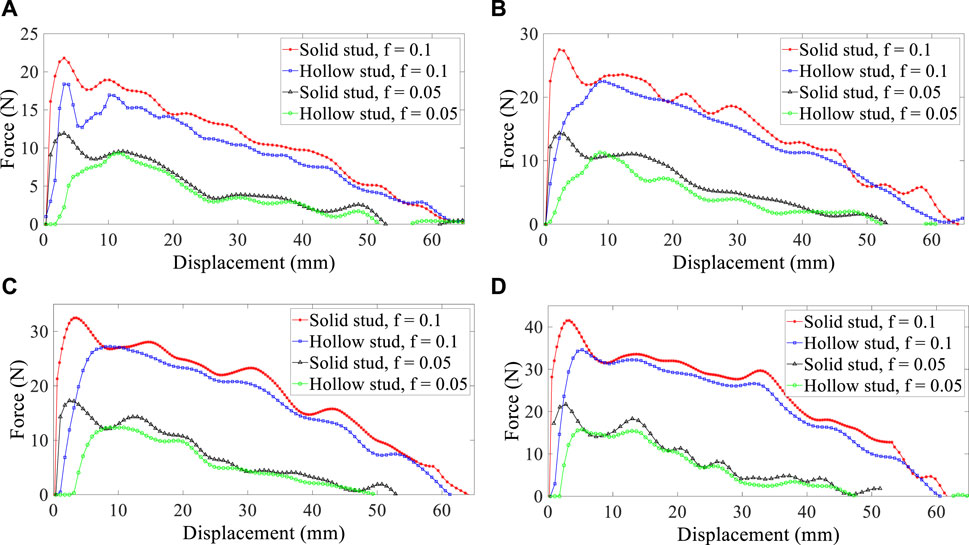
FIGURE 7. Reaction force–displacement curve for two friction factors in four diameters: (A) 20 mm, (B) 21 mm, (C) 22 mm, and (D) 23 mm.
Figure 7 shows that friction significantly affects the reaction forces and, subsequently, the migration of stents. The effect of friction has increased significantly by doubling the friction factor, and it can explain significant differences in reaction forces. The effect of friction evolves essentially when silicones with different properties are used to construct stents. All effective parameters, including the angle of the stud (α), friction factor, stent inner diameter, and hole on studs, are reported in Figure 8 based on the maximum reaction forces required to extract stents from the trachea.
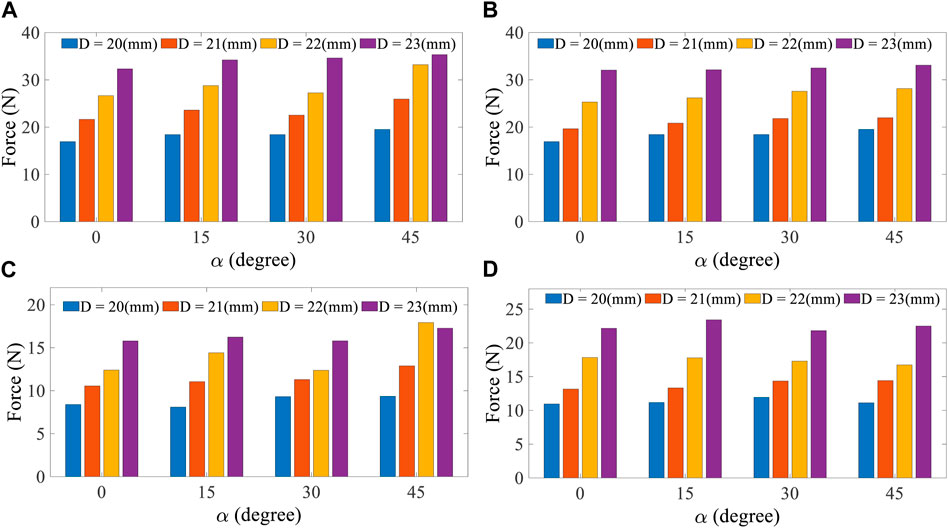
FIGURE 8. Anti-migration forces based on diameter in each stud angle. Friction factor = 0.1 is assumed for (A) and (B), and friction factor = 0.05 is assumed for (C) and (D).
Figure 8 shows that in the friction factor 0.1, the maximum reaction forces belong to 23 diameters, an angle of 45° at the hollow stud state and 23 diameters and 45° at the solid stud state. It is evident that by increasing the diameter of the stent, the force laying the trachea increases, and the possibility of the stent migrating out of the trachea decreases. As the α angle increases, the contact surface between the trachea and the stent increases, which pushes the stent to migrate out or displace under the respiratory pressure difference condition. The results for the friction factor 0.05 are similar to those for the friction factor 0.1. By comparing the results of Figure 8, it is apparent that with the increase of the friction factor between the inner trachea wall and the silicone stent, the pressure difference required to migrate out of the stent increases. Therefore, the essence of preferring the silicone grade employed for the stent is figured out. Finally, according to the selected trachea dimensions, the optimum model with the best performance for opening the respiratory tract and having the most negligible migration is a model with a diameter of 23 mm and an angle of 45° in the solid stud state.
The primary aim of stent implantation is overcoming the extrinsic force or central obstruction causing a loss of the airway structure (Binkert and Petersen, 2002). The different external radial forces over the trachea wall, such as coughing, may cause necrosis of the mucosa and fistula formation, leading to the compression of the silicone stent and removing it from the trachea if the external force is significant (Binkert and Petersen, 2002; Fonesca et al., 2015). The compressed silicone stent exerts a radial force, such as breathing and coughing, which may have weaker function, obstruction, or migration (Vondrys et al., 2011). Furthermore, the high external radial force reduced the stent radius and caused stent displacement and may set patients at high risk; thus, selecting a suitable diameter and properties for the stent is necessary due to low migration. In this investigation, the external radial pressure was applied to hollow stud stents and caused to compress hollow stents. The outer stent wall pressure assumed a 10 kPa–60 kPa range, where forces and structure differences were simulated. Considering that the stent with a 45° angle had the best performance in keeping the airway open, a hollow stent with a 45° angle is used in this section.
Figure 9 shows the force required to remove the stent decreases by increasing the external pressure applied to the stents. However, when the pressure rises above a specific limit due to the asymmetric pressure that the trachea puts on the stents, the stents turn into Figure 9B that has lost its function. Therefore, although more force is required to remove the stent in a larger diameter, more external pressure is exerted when coughing or sneezing, reducing its efficiency and performance. Finally, in each trachea, there is an optimal diameter that, in addition to requiring more anti-migration force, external pressure has less effect on it. Therefore, according to Lausted et al.’s (2006) study, the maximum static pressure is 60 kPa, and a stent with a diameter of 21 has a better performance than a stent with a diameter of 23.
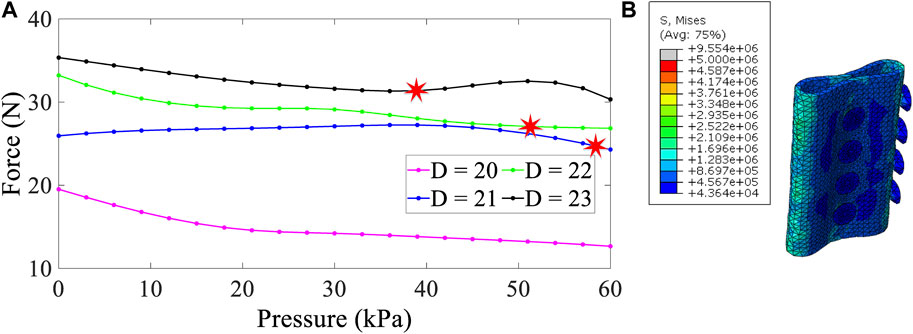
FIGURE 9. Force–pressure curve for four diameters (A) and distributed von Mises stress (Pa) in a compressed hollow stent (B); stud angle is 45°, and the friction factor is assumed to be 0.1.
4 Conclusion
Today, stenting the central airways is a common operation in tracheal airway diseases. Treatment of respiratory diseases due to stent migration and mucus formation is still challenging using silicone stents, but endotracheal stenting provides an alternative to surgical resection in patients with tumor-related extraluminal compression in the central airway. Therefore, it is necessary to understand the stent’s impact on the trachea and the factors affecting its performance better, including the material and dimensions of its design. In order to facilitate the patient’s healing process, various types of stents with different dimensions, geometries, and materials have been designed and produced, and one is preferred and placed according to the patient’s requirement.
In this study, 64 different stent models were designed based on differential geometry and sizes and were placed in the trachea with specific dimensions and analyzed. The results were reported based on the least migration of stents based on anti-migration forces. Furthermore, the conditions of stent placement have been evaluated based on different friction factors, and the importance of selecting the type of silicone for stent construction has been investigated. Based on the reported results, stent diameter and assumed friction are more influential than other factors on the migration possibility of the stent in the trachea, and both directly affect reaction forces. By choosing larger diameters, the reaction forces increase which can prevent stent migration or displacement. Considering the higher fraction factor, the force required to migrating stents increases, reducing the undesirable displacement of the stent in the trachea.
Also, a stent model with a hole on studs has been presented, allowing the mucus’s path. The results of finite-element analysis showed that this model allows the passage of mucus simultaneously with a slight increase in migration. Another geometric parameter that has been studied in this research is the effect of the stud angle on the maximum reaction force and migration of stents. According to the results, with the increase in the stud angle from 0º to 45°, the maximum reaction force also increased, which can affect the holes’ presence and partially moderate the increase in stent migration. In the continuation of this analysis, the 21 mm stent, with a 45° angle, revealed the best implementation against compression under the impact of the respiratory pressure difference. Finally, in each trachea, there is an optimal point for the diameter that, in addition to requiring more anti-migration force, external pressure has less effect on it.
Data availability statement
The original contributions presented in the study are included in the article/Supplementary Material, further inquiries can be directed to the corresponding author.
Author contributions
MF: methodology and writing—original draft. AB: methodology, simulation, and writing—original draft. SB: simulation and writing—original draft. AbK: conceptualization. ArK: conceptualization. MaB: conceptualization and methodology. AE: co-supervision. MoB: co-supervision. All authors contributed to the article and approved the submitted version.
Conflict of interest
The authors declare that the research was conducted in the absence of any commercial or financial relationships that could be construed as a potential conflict of interest.
Publisher’s note
All claims expressed in this article are solely those of the authors and do not necessarily represent those of their affiliated organizations, or those of the publisher, the editors, and the reviewers. Any product that may be evaluated in this article, or claim that may be made by its manufacturer, is not guaranteed or endorsed by the publisher.
References
Alzahrany, M., and Banerjee, A. (2015). A biomechanical model of pendelluft induced lung injury. J. Biomechanics 48, 1804–1810. doi:10.1016/j.jbiomech.2015.04.046
Bakhtiyari, A., Baniasadi, M., and Baghani, M. (2023a). A modified constitutive model for shape memory polymers based on nonlinear thermo-visco-hyperelasticity with application to multi-physics problems. Int. J. Appl. Mech. 15, 2350032. doi:10.1142/s1758825123500321
Bakhtiyari, A., Baghani, M., and Sohrabpour, S. (2023b). An investigation on multilayer shape memory polymers under finite bending through nonlinear thermo-visco-hyperelasticity. Appl. Math. Mech. 44, 73–88. doi:10.1007/s10483-023-2952-6
Binkert, C. A., and Petersen, B. D. (2002). Two fatal complications after parallel tracheal-esophageal stenting. Cardiovasc. interventional radiology 25, 144–147. doi:10.1007/s00270-001-0088-5
Bolliger, C. T., Breitenbuecher, A., Brutsche, M., Heitz, M., and Stanzel, F. (2004). Use of studded polyflex™ stents in patients with neoplastic obstructions of the central airways. Respiration 71, 83–87. doi:10.1159/000075654
Chin, C. S., Litle, V., Yun, J., Weiser, T., and Swanson, S. J. (2008). Airway stents. Ann. Thorac. Surg. 85, S792–S796. doi:10.1016/j.athoracsur.2007.11.051
Colpani, A., Fiorentino, A., and Ceretti, E. (2020). Design and fabrication of customized tracheal stents by additive manufacturing. Procedia Manuf. 47, 1029–1035. doi:10.1016/j.promfg.2020.04.318
Dumon, J.-F. (1990). A dedicated tracheobronchial stent. Chest 97, 328–332. doi:10.1378/chest.97.2.328
Dumoulin, C., and Cochelin, B. (2000). Mechanical behaviour modelling of balloon-expandable stents. J. Biomechanics 33, 1461–1470. doi:10.1016/s0021-9290(00)00098-1
Dutau, H., Cavailles, A., Sakr, L., Badier, M., Gaubert, J.-Y., Boniface, S., et al. (2010). A retrospective study of silicone stent placement for management of anastomotic airway complications in lung transplant recipients: Short-and long-term outcomes. J. Heart Lung Transplant. 29, 658–664. doi:10.1016/j.healun.2009.12.011
Dutau, H., and Dumon, J.-F. (2017). Airway stenting revisited: 30 years, the age of reason? J. Bronchology & Interventional Pulmonol. 24, 257–259. doi:10.1097/lbr.0000000000000433
Folch, E., and Keyes, C. (2018). Airway stents. Ann. Cardiothorac. Surg. 7, 273–283. doi:10.21037/acs.2018.03.08
Fonesca, H., Iuamoto, L., Minamoto, H., Abdalla, L., Fernandes, L., Camargo, P., et al. (2015). Stents for bronchial stenosis after lung transplantation: Should they be removed? Transplant. Proc. 47, 1029–1032. doi:10.1016/j.transproceed.2015.04.005
Gildea, T. R., Murthy, S. C., Sahoo, D., Mason, D. P., and Mehta, A. C. (2006). Performance of a self-expanding silicone stent in palliation of benign airway conditions. Chest 130, 1419–1423. doi:10.1378/chest.130.5.1419
Golden, A. B., and Hellenbrand, W. E. (2007). Coarctation of the aorta: Stenting in children and adults. Catheter. Cardiovasc. Interventions 69, 289–299. doi:10.1002/ccd.21009
Guibert, N., Saka, H., and Dutau, H. (2020). Airway stenting: Technological advancements and its role in interventional pulmonology. Respirology 25, 953–962. doi:10.1111/resp.13801
Ho, A. S., and Koltai, P. J. (2008). Pediatric tracheal stenosis. Otolaryngologic Clin. N. Am. 41, 999–1021. doi:10.1016/j.otc.2008.04.006
Hollister, S. J., Hollister, M. P., and Hollister, S. K. (2017). Computational modeling of airway instability and collapse in tracheomalacia. Respir. Res. 18, 62–68. doi:10.1186/s12931-017-0540-y
Kamel, K. S., Lau, G., and Stringer, M. D. (2009). In vivo and in vitro morphometry of the human trachea. Clin. Anat. 22, 571–579. doi:10.1002/ca.20815
Lally, C., Dolan, F., and Prendergast, P. (2005). Cardiovascular stent design and vessel stresses: A finite element analysis. J. Biomechanics 38, 1574–1581. doi:10.1016/j.jbiomech.2004.07.022
Larson, K. (2016). Can you estimate modulus from durometer hardness for silicones. Midland: Dow Corning Corporation, 1–6.
Lausted, C. G., Johnson, A. T., Scott, W. H., Johnson, M. M., Coyne, K. M., and Coursey, D. C. (2006). Maximum static inspiratory and expiratory pressures with different lung volumes. Biomed. Eng. online 5, 29–36. doi:10.1186/1475-925x-5-29
Lee, H. J., Labaki, W., Diana, H. Y., Salwen, B., Gilbert, C., Schneider, A. L., et al. (2017). Airway stent complications: The role of follow-up bronchoscopy as a surveillance method. J. Thorac. Dis. 9, 4651–4659. doi:10.21037/jtd.2017.09.139
Lee, P., Kupeli, E., and Mehta, A. C. (2016). Airway stents. Interv. Pulm. Med. 7, 61–76. doi:10.21037/acs.2018.03.08
Malvè, M., del Palomar, A. P., Mena, A., Trabelsi, O., López-Villalobos, J., Ginel, A., et al. (2011). Numerical modeling of a human stented trachea under different stent designs. Int. Commun. heat mass Transf. 38, 855–862. doi:10.1016/j.icheatmasstransfer.2011.04.012
McGrath, D., O’Brien, B., Bruzzi, M., Kelly, N., Clauser, J., Steinseifer, U., et al. (2016). Evaluation of cover effects on bare stent mechanical response. J. Mech. Behav. Biomed. Mater. 61, 567–580. doi:10.1016/j.jmbbm.2016.04.023
Mughal, M. M., Gildea, T. R., Murthy, S., Pettersson, G., DeCamp, M., and Mehta, A. C. (2005). Short-term deployment of self-expanding metallic stents facilitates healing of bronchial dehiscence. Am. J. Respir. Crit. care Med. 172, 768–771. doi:10.1164/rccm.200410-1388oc
Petersen, B. D., Uchida, B. T., Barton, R. E., Keller, F. S., and Rösch, J. (1995). Gianturco-rösch z stents in tracheobronchial stenoses. J. Vasc. Interventional Radiology 6, 925–931. doi:10.1016/s1051-0443(95)71214-3
Ratnovsky, A., Regev, N., Wald, S., Kramer, M., and Naftali, S. (2015). Mechanical properties of different airway stents. Med. Eng. Phys. 37, 408–415. doi:10.1016/j.medengphy.2015.02.008
Rouhani, F., Fereidoonnezhad, B., Zakerzadeh, M. R., and Baghani, M. (2020). Modeling of damage evolution in a patient-specific stenosed artery upon stent deployment. Int. J. Appl. Mech. 12, 2050101. doi:10.1142/s175882512050101x
Ruan, X. L., Li, J. J., Song, X. K., Zhou, H. J., Yuan, W. X., Wu, W. W., et al. (2018). Mechanical design of antichiral-reentrant hybrid intravascular stent. Int. J. Appl. Mech. 10, 1850105. doi:10.1142/s1758825118501053
Saad, C. P., Ghamande, S. A., Minai, O. A., Murthy, S., Pettersson, G., DeCamp, M., et al. (2003). The role of self-expandable metallic stents for the treatment of airway complications after lung transplantation. Transplantation 75, 1532–1538. doi:10.1097/01.tp.0000061229.83500.a0
Safshekan, F., Tafazzoli-Shadpour, M., Abdouss, M., and Shadmehr, M. B. (2016). Mechanical characterization and constitutive modeling of human trachea: Age and gender dependency. Materials 9, 456. doi:10.3390/ma9060456
Saito, Y., and Imamura, H. (2005). Airway stenting. Surg. today 35, 265–270. doi:10.1007/s00595-004-2942-y
Shanahan, C., Tofail, S. A., and Tiernan, P. (2017). Viscoelastic braided stent: Finite element modelling and validation of crimping behaviour. Mater. Des. 121, 143–153. doi:10.1016/j.matdes.2017.02.044
Thornton, R. H., Gordon, R. L., Kerlan, R. K., LaBerge, J. M., Wilson, M. W., Wolanske, K. A., et al. (2006). Outcomes of tracheobronchial stent placement for benign disease. Radiology 240, 273–282. doi:10.1148/radiol.2401042169
Tyagi, S., Kaul, U. A., and Arora, R. (1999). Endovascular stenting for unsuccessful angioplasty of the aorta in aortoarteritis. Cardiovasc. interventional radiology 22, 452–456. doi:10.1007/s002709900431
Vearick, S. B., Demétrio, K. B., Xavier, R. G., Moreschi, A. H., Muller, A. F., Sanches, P. R. S., et al. (2018). Fiber-reinforced silicone for tracheobronchial stents: An experimental study. J. Mech. Behav. Biomed. Mater. 77, 494–500. doi:10.1016/j.jmbbm.2017.10.013
Vondrys, D., Elliott, M. J., McLaren, C. A., Noctor, C., and Roebuck, D. J. (2011). First experience with biodegradable airway stents in children. Ann. Thorac. Surg. 92, 1870–1874. doi:10.1016/j.athoracsur.2011.07.042
Walser, E. M. (2005). Stent placement for tracheobronchial disease. Eur. J. radiology 55, 321–330. doi:10.1016/j.ejrad.2005.03.005
Xu, J., Ong, H. X., Traini, D., Byrom, M., Williamson, J., and Young, P. M. (2019). The utility of 3d-printed airway stents to improve treatment strategies for central airway obstructions. Drug Dev. Industrial Pharm. 45, 1–10. doi:10.1080/03639045.2018.1522325
Zakaluzny, S. A., Lane, J. D., and Mair, E. A. (2003). Complications of tracheobronchial airway stents. Otolaryngology—Head Neck Surg. 128, 478–488. doi:10.1016/s0194-5998(03)00002-0
Zhang, Z., Zhu, M., Shi, X., and Deng, L. (2016). “Numerical simulation on human trachea stenosis and stent implantation,” in A79. Lung imaging: Ct and beyond (American Thoracic Society), A2497.
Keywords: airway silicone stents, tracheal simulation, stent migration modeling, anti-migration forces, optimizing stent geometry
Citation: Farahani MM, Bakhtiyari A, Beshkoofe S, Kajbafzadeh A, Kiani A, Eskandari AH, Baniassadi M and Baghani M (2023) Numerical simulation of the effect of geometric parameters on silicone airway stent migration. Front. Mech. Eng 9:1215895. doi: 10.3389/fmech.2023.1215895
Received: 02 May 2023; Accepted: 21 June 2023;
Published: 06 July 2023.
Edited by:
Manuel Doblare, University of Zaragoza, SpainReviewed by:
Arya Amiri, University of Waterloo, CanadaHamid Shahsavari, Karlsruhe Institute of Technology (KIT), Germany
Copyright © 2023 Farahani, Bakhtiyari, Beshkoofe, Kajbafzadeh, Kiani, Eskandari, Baniassadi and Baghani. This is an open-access article distributed under the terms of the Creative Commons Attribution License (CC BY). The use, distribution or reproduction in other forums is permitted, provided the original author(s) and the copyright owner(s) are credited and that the original publication in this journal is cited, in accordance with accepted academic practice. No use, distribution or reproduction is permitted which does not comply with these terms.
*Correspondence: Mostafa Baghani, YmFnaGFuaUB1dC5hYy5pcg==