- 1French-German Research Institute of Saint Louis, Saint-Louis, France
- 2ICube Laboratory, Strasbourg University, Strasbourg, France
Although blast-induced Traumatic Brain Injury (bTBI) has become a signature wound of conflict, its cause is not yet fully understood. Regarding primary blast injuries, i.e., those caused by the propagation of shock waves in the body, four direct and two indirect injury mechanisms have been mainly proposed in the literature. Since numerous authors have exposed instrumented animals, Post-Mortem Human Subjects (PMHS), and head substitutes to blast conditions, the aim of this review is to classify them in terms of threat, instrumentation, and investigated mechanisms. In the first part, data are collected from 6 studies on PMHS, 1 on primates, 11 on rodents, and 6 on swine for comparison purposes. Peak amplitudes of reflected pressures, intracranial pressures and cranial strains are extracted and analyzed to establish trends. Despite the small number of comparable studies, several similarities can be highlighted. Indeed, the analyses revealed a dose-response effect for most measurements. The results also depend on the orientation of the subject (forward, backward, and sideways) for the PMHS, primates, and swine. The second goal of this review is to evaluate the behavior of substitutes developed to replace PMHS experiments. Shell strains and internal pressures are thus collected on 19 geometric and anthropomorphic substitutes to assess whether they faithfully represent a human head. The results showed that these substitutes are for the most part not properly designed and therefore cannot yet reliably replace PMHS experimental data.
1 Introduction
In the current context of terrorist attacks and armed conflicts, explosive devices have become a common threat. Since the Second World War, more than 65% of war injuries are caused by the detonation of Improvised Explosive Devices (IEDs) (Belmont et al., 2010). Soldiers exposed to such phenomena are usually affected on multiple body parts (Champion et al., 2003). In particular, the head-neck segment is a vital and vulnerable area to this specific threat since it encloses the brain, bathing in the cerebrospinal fluid (CSF). This fact is supported by the increase in Traumatic Brain Injuries (TBIs) reported in the U.S. Armed Forces, whose incidence has doubled between 2000 and 2018 (DVBIC, 2020). These numbers can be further correlated with the massive use of IEDs on the battlefield: between 2001 and 2007, 63% of TBIs were caused by explosions (Wojcik et al., 2010). The symptoms of TBI may include memory loss, attention troubles and headaches (Trudeau et al., 1998; McGruk et al., 2008; Warden et al., 2009; Rosenfeld et al., 2013; Magnuson and Ling, 2018), but also severe brain damage such as convulsions, seizures and cerebral vasospasms (Armonda et al., 2006; Hicks et al., 2010). Hence, it is conspicuous that blast-induced Traumatic Brain Injury (bTBI) represents a societal cost and that understanding their injury mechanisms remains a critical milestone in the field.
Several studies characterize the physics of IEDs and describe the four phases of the “blast phenomenon”: the propagation of shock waves in the ambient air (primary phase), the penetration of debris and fragments energized from the munition and the environment (secondary phase), acceleration and deceleration effects (tertiary phase); and finally, miscellaneous effects such as thermal, chemical, pathogenic agents, etc. (quaternary phase) (Sochet, 2017; Needham, 2010). TBI can be caused by all blast phases (Depalma et al., 2005), nonetheless this study focuses solely on the propagation of shock waves. In free-field conditions, this shock wave results from the local compression of the surrounding air following the detonation of explosive charges. Its time evolution can be approximated by a quasi-ideal signal, known as the Friedlander waveform. The latter is characterized by an initial overpressure that reaches a maximum value called the incident pressure. The wave then decays exponentially, first in a positive phase lasting a few milliseconds, and later in a negative phase. Finally, the wave stabilizes at the ambient pressure level. To obtain quasi-ideal shock waves on a laboratory scale, shock tubes are often used. Under these conditions, a single planar shock wave can be observed inside the tube at an optimized distance from its end, while outside the shock tube the waveform becomes three-dimensional and is accompanied by a jet wind.
In order to understand the cerebral lesions observed during a blast exposure, several experiments have been performed on animals that were exposed to shock waves considering either free-field or shock tube conditions. Autopsies have been carried out and numerous cerebral lesions have been observed, such as vascular lesions (Readnower et al., 2010; Reneer et al., 2011; Reneer et al., 2014; Kabu et al., 2015; Walls et al., 2016), encephalic lesions (Kuehn et al., 2011; Ahlers et al., 2012; Gullotti et al., 2014; Kabu et al., 2015), and axonal injuries (Säljö et al., 2000; Rubovitch et al., 2011; Kallakuri et al., 2015). Nevertheless, these observations alone were not sufficient to explain bTBI: animals, Post-Mortem Human Subjects (PMHS), as well as head substitutes were thus instrumented with pressure sensors and strain gauges to further investigate. The results obtained were the basis for proposing six non-exclusive injury mechanisms (Courtney and Courtney, 2015; Ouellet and Philippens, 2018). These mechanisms are divided into two categories, depending on how the blast wave affects the head: direct and indirect. The four direct mechanisms are first a direct cranial entry of the waves through the foramina, a propagation through the head layers due to successive acoustic impedance mismatches called a transosteal propagation (Clemedson and Pettersson, 1955; Chavko et al., 2011; Sutar and Ganpule, 2019), a skull deflection that could cause cerebral lesions (Bolander, 2012; Chandra and Sundaramurthy, 2015; Salzar et al., 2017), and finally a cavitation phenomenon leading to the formation and collapse of microscopic bubbles in the cerebrospinal fluid (Salzar et al., 2017). On the other hand, two indirect mechanisms have been proposed: a relative brain/skull motion caused by head acceleration (Knudsen and Øen, 2003; Gullotti et al., 2014), and a compression of the thorax which could cause a pressure surge in the brain (Courtney and Courtney, 2009; Rubio et al., 2020). Each of the aforementioned studies were designed to investigate a specific injury mechanism; however, to date there exists no comparative study of all the mechanical metrics measured in the literature.
Hence, this paper aims to present the up-to-date research on instrumented experimental analyses performed to investigate primary bTBI. For this purpose, a summary is first made of the findings from studies exposing PMHS, large mammals, rodents and head substitutes to single blast waves. In a second part, measurements of metrics collected from those studies, such as cranial strains, reflected and intracranial pressures (ICPs), are compared and analyzed between species to establish specific trends and to investigate the proposed injury mechanisms.
2 Experimental analyses performed in the literature
This section reviews the 43 experimental studies in the literature in which instrumented animals, PMHS, and substitutes were exposed to the propagation of a single shock wave. The goal of each of these studies was to investigate one injury mechanism by measuring mechanical metrics against a specific shock wave. For the sake of comparison between the various studies, these shock waves are characterized here only in terms of their incident pressure Pi and positive phase duration T+ when available, since reflected pressures and impulses are detailed in only a limited number of studies in the literature. In the following, the PMHS conventions are used to describe the location of the sensors mounted on the head of each subject, as illustrated in Figure 1A. The same applies to the orientation of the subject relative to the direction of propagation of the shock wave (Figure 1B).
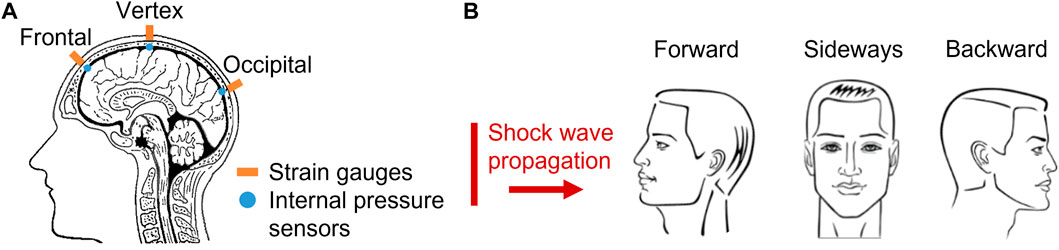
FIGURE 1. Illustration of experimental tests exposing PMHS for the investigation of blast-induced Traumatic Brain Injury. (A) Location of mounted sensors. (B) Orientation of test subjects to the propagation of shock waves.
2.1 Studies on large mammals
First, a total of 6 studies were performed to expose large mammals to blast conditions, more specifically swine and monkeys. A wide test range was considered for these studies, with incident pressures ranging from 15 kPa to 970 kPa, and positive phase durations varying from 0.1 to 7.5 ms.
In 1961 Romba et al. exposed 2 post-mortem rhesus monkeys to free-field blast conditions to investigate the thoracic compression mechanism (Romba and Martin, 1961). The monkeys were exposed with their head and thorax alternately protected to incident pressures in the range of 20—930 kPa (T+ = 0.1—2.3 ms). By quantifying the pressure differentials in the intracranial and intrathoracic cavities, the authors demonstrated a small contribution of the thoracic mechanism. The same conclusions were drawn by Säljö et al. (2008) while exposing 6 post-mortem swine to a focal blast of 15 kPa either to the head or thorax. Later, Bolander performed an in-vitro experiment on 5 swine to measure cranial strains and ICP to investigate the skull deflection hypothesis (Bolander, 2012). Subject orientation to the shock waves and sensor location effects were highlighted, and a relationship between strain and ICP was established: bowing of the cranial bones was correlated with an increase in ICP. Shridharani et al. (2012) evaluated the in-vivo biomechanical response of 20 swine, exposed to incident pressures of 107—741 kPa (T+ = 1.2—6.4 ms). ICPs were recorded, showing an attenuation of the wave amplitude within the cranial cavity compared to both reflected and incident pressures. Finally, Feng et al. exposed 5 swine to free-field conditions with incident pressures of 150 kPa, 280 kPa, 410 kPa to record ICP, linear accelerations as well as angular velocities of their heads (Feng et al., 2016). Orientation, sensor location and dose-response effects were observed.
2.2 Studies on rodents
Experiments on large mammals are often difficult to perform because of their size. Hence, to facilitate experimental procedures, a common choice is to expose rodents inside or outside shock tubes, as done in 10 published studies. Rodents were exposed to shock waves in the incident pressure range of 8—290 kPa, with positive phase durations between 4 and 10 ms.
Clemedson et al. exposed several instrumented rodents to blast waves of 40 kPa in free-field conditions (Clemedson and Pettersson, 1955). These post-mortem rabbits were instrumented with pressure sensors in their intracranial and intrathoracic cavities. The internal pressures were amplified compared to the incident pressures. However, these results contradicted those of more recent studies, the first of which was performed by Chavko et al. (2007). ICPs were measured inside the cranium of several in-vivo rats subjected to 40 ± 7 kPa (T+ = 4.5 ms) in forward and sideways orientations, and showed an amplitude equivalent to the incident pressures. In addition, experiments carried out by Säljö et al. (2008) involving 2 rats subjected to 8.2 ± 0.4 kPa outside a shock tube also recorded ICPs with amplitude equivalent to the incident pressures. On another note, Chavko et al. (2011) exposed rats to forward, sideways and backward orientations at 36 ± 2 kPa (T+ = 4 ms) to study the effects on ICP measurements, and showed non-deterministic variations. The same conclusions were reached in a study by Leonardi et al., in which 8 rats were exposed in various orientations to a 70 kPa overpressure (T+ = 7.5 ms) (Dal Cengio Leonardi et al., 2012). The same authors performed experiments on 25 rats exposed with and without an eye patch to incident pressures of 69 kPa (T+ = 7.5 ms) to study ICP variations and evaluate the plausibility of the direct cranial entry mechanism, but no conclusive conclusions could be drawn (Dal Cengio Leonardi et al., 2011a). Later, Bolander et al. (2011) measured ICPs and cranial strains on 10 rats to further investigate the skull deflection hypothesis (Pi = 69, 97, 117, 172 kPa/T+ = 7.5 ms). Moreover, two different studies recorded ICPs, reflected pressures as well as intrathoracic pressures on 5 rats each, with incident pressures of 100—225 kPa for (Sundaramurthy et al., 2012) and 130—290 kPa (T+ = 4—10 ms) for (Skotak et al., 2013). The aim was to investigate the thoracic mechanism, and for that same purpose, a recent study by Rubio et al. (2020) measured ICPs and carotid artery pressures of 16 in-vivo rats (Pi = 75, 133 kPa/T+ = 4—5 ms). Contradictory results were found between the post-mortem and in-vivo studies.
2.3 Studies on PMHS
Although animal experiments are important for understanding the emergence of cerebral lesions in in-vivo subjects, conducting experiments on Post-Mortem Human Subjects (PMHS) is a crucial step. Six studies were performed in the literature using 18 PMHS heads. The brains of 7/18 PMHS were removed and replaced with substitutes such as gels or saline solutions (PMHS-Substitute) (Shah et al., 2011; Ganpule et al., 2013a; Chandra and Sundaramurthy, 2015; Salzar et al., 2017). If brains were preserved (11/18 PMHS), the heads were pressurized with a saline solution to ensure having homeostatic pressure (PMHS-Brain) (Dal Cengio Leonardi, 2011; Bolander, 2012; Ott et al., 2013; Iwaskiw et al., 2018). Each PMHS-Brain and PMHS-Substitute were instrumented with sensors, and then subjected to quasi-ideal shock waves via shock tubes. The experiments can be divided into two groups of incident pressures: a low-level blast dose of 64 ± 28 kPa and a high-level blast dose of 175 ± 27 kPa. However, positive phase durations are rarely reported and vary from 4.4 ms to 7.5 ms.
The first studies to do so were performed by Dal Cengio Leonardi (2011) and Bolander (2012). A total of 4 PMHS-Brain were exposed to incident pressures of 69 kPa, 83 kPa and 104 kPa (T+ = 7.5 ms), with the objective to observe ICPs and cranial strains evolutions in forward, sideways and backward orientations. Later, Shah et al. (2011) exposed 1 PMHS-Substitute to lower shock waves of 21 kPa and 45 kPa, in order to highlight the variations of reflected pressures and ICPs in forward and sideways orientations. Ott et al. (2013) also frontally exposed 3 PMHS-Substitute to incident pressures of 50 and 75 kPa. Ganpule et al. presented results of reflected pressures, ICPs, and cranial strains recorded on 3 PMHS-Substitute exposed to 70 kPa, 140 kPa and 200 kPa in forward orientation (T+ = 5.4 ms) (Ganpule et al., 2013a; Chandra and Sundaramurthy, 2015). The latter study concluded the transosteal propagation to be the main injury mechanism. Salzar et al. (2017) investigated the CSF cavitation hypothesis by exposing 3 PMHS-Substitute in forward orientation (Pi = 80—350 kPa/T+ = 5 ms) while recording ICPs and cranial deformations. Negative ICP peaks were measured, suggesting the formation of cavitation bubbles. Finally, the most recent study by Iwaskiw et al. (2018) involved 4 PMHS-Brains placed in forward and backward orientations inside a shock tube. Incident pressures of 160 and 200 kPa were applied to the subjects to record reflected pressures, ICPs and brain displacements thanks to radiopaque markers. Orientation and sensor location effects were observed.
2.4 Studies on substitutes
Finally, with the goal of improving the reproducibility of the blast experiments and further investigate the bTBI injury mechanisms, 28 substitutes were created and presented in 21 studies. These substitutes are divided into two categories: 6 anthropomorphic and 22 geometric substitutes.
Among the anthropomorphic substitutes, Merkle et al. (2012) recorded displacements in a brain substitute exposed to 875 kPa, and observed a relative brain/skull displacements. In addition, internal pressures were recorded in the frontal and occipital positions, showing a dose-response as well as a sensor location effects (Merkle et al., 2009; Merkle et al., 2010; Merkle et al., 2012). Ouellet et al. (2014) also recorded reflected and internal pressures on a substitute exposed inside a shock tube in forward and sideways orientations (Pi = 80, 100, 120 kPa/T+ = 7.5—8.5 ms) and in free-field conditions (Pi = 55–250 kPa/T+ = 0.8—8.5 ms) (Ouellet and Philippens, 2018). Orientation, dose-response and sensor location effects were observed. Other studies performed on anthropomorphic substitutes focused solely on recording the reflected pressures at different locations to characterize the threat (Rafaels, 2010; Ganpule et al., 2013b; Ganpule and Chandra, 2013; Chandra et al., 2017; Alay et al., 2018; Banton et al., 2018; Skotak et al., 2019).
Regarding geometric substitutes, 1 cylindrical model (Selvan et al., 2013), 3 ellipsoidal models (Goeller et al., 2012; Zhu et al., 2012), a 1D model (Yu et al., 2020), and 17 spheres (Dal Cengio Leonardi et al., 2011a; Dal Cengio Leonardi, 2011; Alley et al., 2011; Varas et al., 2011; Hua et al., 2014; Josey et al., 2016; Rude, 2016) have been exposed to blast conditions exclusively using shock tubes. However, only two of these substitutes had dimensions close to a human head: a mean circumference of 180 mm (Bushby et al., 1992; Got et al., 1983; Haeussinger et al., 2011) and a frontal bone thickness of 7 mm (Got et al., 1983; Lynnerup, 2001; Haeussinger et al., 2011). Dal Cengio Leonardi (2011); Varas et al. (2011); Dal Cengio Leonardi et al. (2011b) exposed gel-filled Synbone spheres of 190 mm diameter and 7 mm thickness to measure reflected pressures, shell strains and internal pressures to highlight their orientation effects (Pi = 40–103 kPa/T+ = 6 ms). Orientation effects on internal pressures and shell strains were also observed by Alley et al. in a gel-filled PMMA substitute (Pi = 96, 103, 172, 760 kPa/T+ = 0.4—1.2 ms) (Alley et al., 2011); as well as in the studies by Selvan et al. (2013) and Hua et al. (2014) who respectively exposed a polycarbonate cylinder (Pi = 160 kPa) and a sphere (Pi = 130 kPa/T+ = 4.5 ms) to blast conditions. In addition, Josey et al. (2016) and Rude (2016) exposed polypropylene spheres filled with either water or gelatin (Pi = 60—207 kPa/T+ = 6 ms) to observe the shell’s vibrations and support the skull deflection hypothesis. Finally, Goeller et al. (2012) created an ellipsoidal model in polycarbonate, with a length of 192 mm and a width of 137 mm. Shell strains, internal and reflected pressures were recorded; and cavitation bubbles were observed at the contralateral position (Pi = 51—172 kPa/T+ = 3 ms). A recent study by Yu et al. (2020); Yu et al. (2022) also investigated the cavitation phenomenon in a 1D model with successive layers of acrylic, distilled water and agar gel (Pi = 111—127 kPa, T+ = 0.5—1.4 ms).
3 Comparison of studies in terms of mechanical quantities
The following section details the analysis performed on the reflected pressures, internal pressures, intra-thoracic pressures, and cranial/shell strains collected from the 43 previously mentioned studies. The purpose is to establish intra-species trends; therefore, for each metric, either extreme or first peak values are collected. The results are presented in terms of the mean and standard deviation values against the incident pressures. For each specific threat, linear regression curves are established, and a correlation analysis is performed between the proposed trends and the experimental data. The reported R2 values are adjusted Pearson’s coefficients of determination to indicate the quality of the fit.
3.1 Reflected pressures
Reflected pressures were recorded on 10 in-vitro rats (Sundaramurthy et al., 2012; Skotak et al., 2013); on the frontal bone of 11 PMHS’ heads (Shah et al., 2011; Ganpule et al., 2013a; Ott et al., 2013; Iwaskiw et al., 2018), on 2 monkeys (Romba and Martin, 1961); and on 1 geometric (Selvan et al., 2013) and 4 anthropomorphic substitutes (Rafaels, 2010; Ganpule et al., 2013b; Ganpule and Chandra, 2013; Chandra et al., 2017; Alay et al., 2018; Banton et al., 2018; Skotak et al., 2019). Figure 2 shows peak amplitude values of the reflected pressures measured during forward exposures. For all species, there is an increase in peak reflected pressure with the incident pressure.
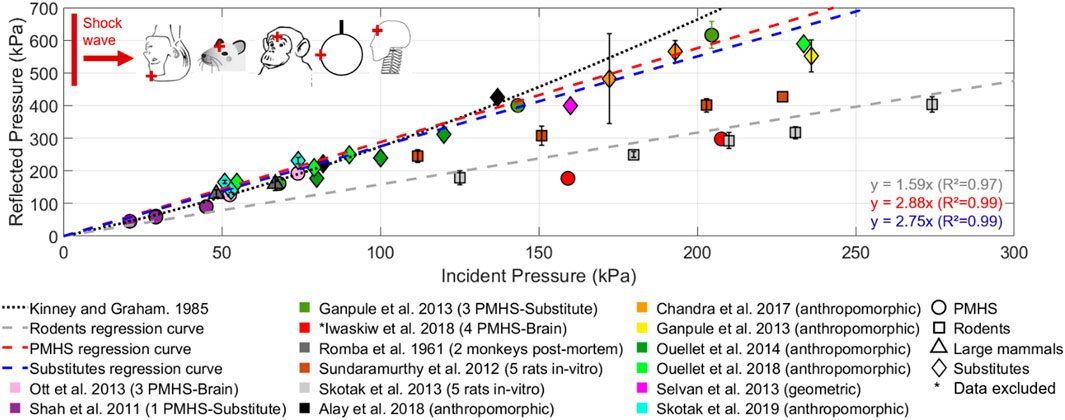
FIGURE 2. Reflected pressures plotted against incident pressures, obtained during the forward exposure of PMHS, rodents and substitutes to blast conditions. The cross markers on the schematic representations of species indicates the approximate position of the reflected pressure sensors.
However, in rats, the peak pressures were higher in the study by Sundaramurthy et al. (2012) than in the study by Skotak et al. (2013), even though the subjects were in the same configuration against the shock waves. Indeed, for an incident pressure of 210 kPa, Skotak et al. recorded a reflected pressure of 293 ± 25 kPa, whereas Sundaramurthy et al. recorded peak values of 402 ± 21 kPa. Signal post-processing (use of numerical filters, sampling frequency, etc.) or blast conditions such as different positive phase durations could explain these differences.
Regarding PMHS subjects, only one study compared the reflected pressures obtained at different orientations for the same 29 kPa incident pressure (Shah et al., 2011). The peak reflected pressures measured at ipsilateral locations had the same amplitude, i.e., for a forward exposure the reflected pressure recorded on the frontal bone was 61.3 kPa; while the temporal bone sensors recorded a reflected pressure of 58.4 kPa for a sideways exposure. In addition, the values reported by Iwaskiw et al. (2018) are lower than those of other authors, which could be explained by the fact that the PMHS were placed head down in the extension of a shock tube, possibly modifying the waves propagation. The PMHS values reported by Shah et al. (2011), Ganpule et al. (2013a) and Ott et al., (2013) appear to follow the approximation between reflected and incident pressures given by Kinney and Graham (1985), when considering a reflection off an infinite wall in a perfect gas at a constant atmospheric pressure.
The differences observed between rodents (y = 1.59x, R2 = 0.97) and PMHS evolutions could be explained by a snout effect that would attenuate the waves, or by the smaller surface area of the rodent head exposed to the waves.
Finally, the results measured in the frontal position of head substitutes (y = 2.75x, R2 = 0.99) follow a similar linear evolution as the PMHS values (y = 2.88x, R2 = 0.99).
3.2 Internal pressures
ICPs were systematically measured in the 11 PMHS-Brain and 7 PMHS-Substitute by implanting pressure sensors in the cranial cavity (Dal Cengio Leonardi, 2011; Shah et al., 2011; Ganpule et al., 2013b; Ott et al., 2013; Salzar et al., 2017; Iwaskiw et al., 2018). Furthermore, ICPs were recorded for 2 in-vitro monkeys exposed in backward orientation (Romba and Martin, 1961), 10 in-vitro swine exposed in forward and backward orientations (Bolander, 2012; Feng et al., 2016); as well as 10 in-vitro rats (Sundaramurthy et al., 2012; Skotak et al., 2013) and 33 in-vivo rats (Dal Cengio Leonardi et al., 2012; Dal Cengio Leonardi et al., 2011a). Figure 3 presents the peak ICPs recorded in the frontal (A) and occipital (B) positions during a forward exposure, and in the frontal (C) and occipital (D) positions during a backward exposure. For all sensors, there is an increase in ICP with the incident pressures.
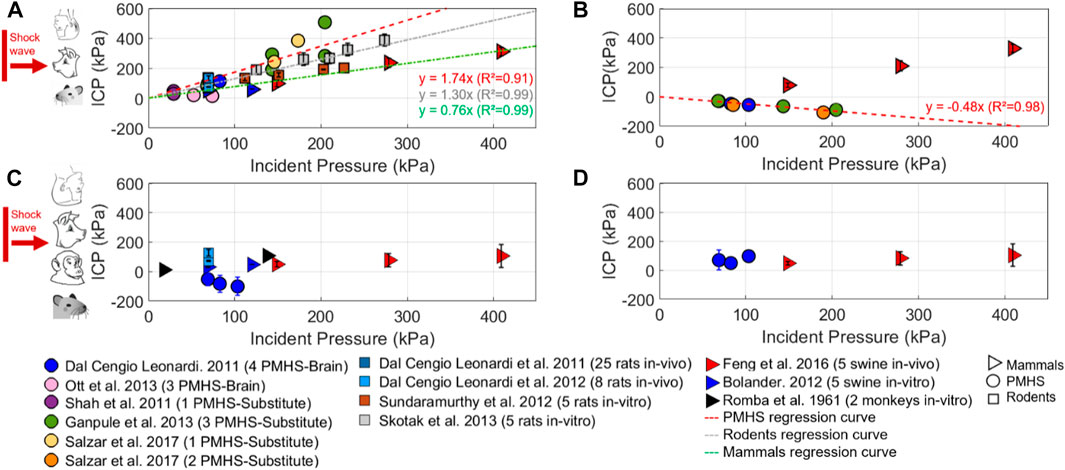
FIGURE 3. First peak values of intracranial pressures (ICP) measured for PMHS, rodents and mammals, plotted against incident pressures. (A) Frontal sensors measurements obtained during forward exposures. (B) Occipital sensors measurements obtained during forward exposures. (C) Frontal sensors measurements obtained during backward exposures. (D) Occipital sensors measurements obtained during backward exposures.
Nevertheless, specific intra-species trends can be observed for a forward exposure (Figures 3A–B): for PMHS (y = 1.74x, R2 = 0.91) and rodents (y = 1.30x, R2 = 0.99) there is an amplification of frontal ICPs; while an attenuation is detected for large mammals (y = 0.76x, R2 = 0.99). The differences observed could be explained by the presence of a snout in rodents and large mammals, and by the cranial thickness of the frontal bones in swine, which is approximately 10 mm, compared to 7 mm in humans and 0.7 mm in rats.
Another interesting point regarding internal pressure is that for rodents, results follow a linear trend regardless of sensor location. Moreover, the occipital ICP peaks of PMHS were all negative (y = −0.48x, R2 = 0.98), indicating an ipsi-controlateral effect between the frontal and occipital sensors.
Figures 3C–D shows that for a given incident pressure, the ICPs measured during backward exposures are inferior to those measured during forward orientations. For instance, Leonardi 2011 measured frontal ICPs of 4 PMHS exposed to 83 kPa: for forward exposures the mean value was 113 ± 29 kPa, compared to −83 ± 57 kPa for backward exposures (Dal Cengio Leonardi, 2011). However, no robust trend could be drawn due to the lack of experimental data and the large discrepancies observed in the results. These orientation and sensors location effects are observed for both PMHS and large mammals, but not for rodents.
Internal substitute pressures (ISPs) were also measured inside the shells of geometric (Alley et al., 2011; Dal Cengio Leonardi et al., 2011b; Goeller et al., 2012; Selvan et al., 2013; Hua et al., 2014: Yu et al., 2020) and anthropomorphic (Merkle et al., 2010; Ouellet et al., 2014; Banton et al., 2018; Ouellet and Philippens, 2018) substitutes. Figure 4 shows the peak values of ISP measured in frontal (A) and occipital (B) positions. The results vary considerably from one study to another because of the different materials properties and geometries used; therefore, it is not possible to establish robust trends. Nevertheless, the ISPs measured inside anthropomorphic substitutes are generally lesser than those measured in geometric models.
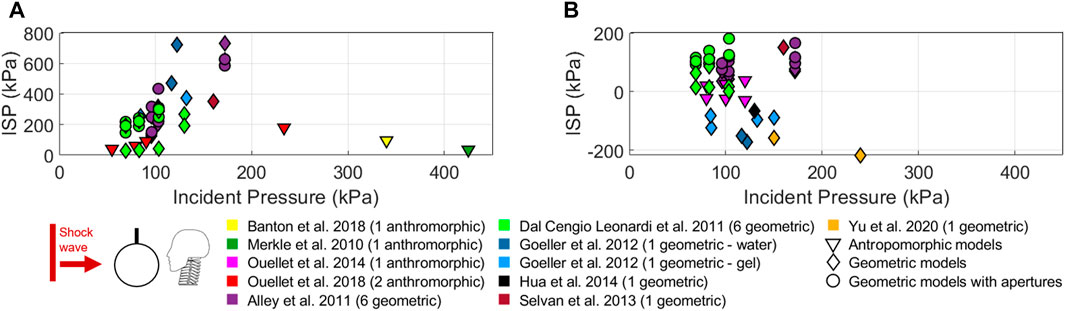
FIGURE 4. First peak values of internal substitute pressures (ISP) measured inside geometric and anthropomorphic substitutes during a forward exposure, and plotted against incident pressures. (A) Frontal sensors measurements. (B) Occipital sensors measurements.
A study carried out by Ouellet et al. (2014) on an anthropomorphic substitute is of particular interest: the time evolutions of internal pressures measured on the substitute were directly compared with those obtained during exposure of PMHS to similar blast conditions. The time histories were similar, with differences in amplitude that can be explained by the large standard deviations in the PMHS results. For instance, at an incident pressure of 100 kPa, Ouellet et al., 2014 recorded an occipital ISP of −25 kPa inside their substitute; compared to −55 ± 26 kPa in Leonardi’s PMHS study (Dal Cengio Leonardi et al., 2011b). Ouellet et al. also recorded an ipsi-controlateral effect between frontal and occipital positions (Ouellet et al., 2014; Ouellet and Philippens, 2018), a peculiarity already observed for PMHS trends; and other studies conducted with geometric substitutes (Dal Cengio Leonardi et al., 2011b; Goeller et al., 2012; Hua et al., 2014) reported the same phenomenon.
Finally, regarding geometric substitutes, two studies compared the ISPs measured inside a spherical shell with and without the presence of apertures (Alley et al., 2011; Dal Cengio Leonardi et al., 2011b). Only small non-deterministic variations in ISP were observed.
3.3 Intra-thoracic pressures
Two studies involving large mammals have investigated the thoracic compression injury mechanism. The heads and torsos of 2 in-vitro monkeys (Romba and Martin, 1961) and 6 in-vitro swine (Säljö et al., 2008) were successively exposed to a localized blast. Figure 5 presents the first peaks of ICPs and intrathoracic pressures measured during the vertex exposure of the animals, showing that both studies recorded low intra-thoracic pressures around 3 kPa, while ICP peaks reached 10 kPa when only heads were exposed to incident pressures of 20 kPa (Figure 5A).
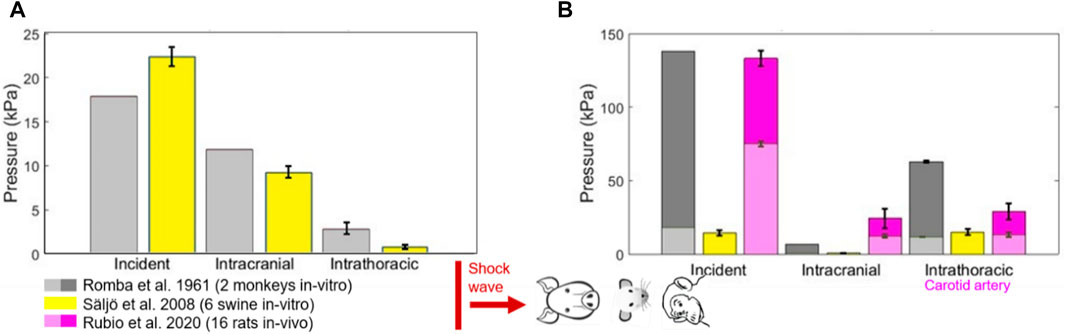
FIGURE 5. Incident pressures, intracranial pressures and intrathoracic first peaks pressures measured during vertex exposures of rodents and mammals. (A) Configuration with thoraces protected and heads exposed. (B) Configuration with heads protected and thoraces exposed.
In contrast, ICPs had a minor amplitude of 1 and 9 kPa; and 20 kPa was recorded in the intra-thoracic cavities when only the thoraces were exposed (Figure 5B). In a recent study, 16 in-vivo rats were exposed in a vertex orientation, with only their torso exposed (Rubio et al., 2020). At an incident pressure of 140 kPa, the ICP was 25 kPa whereas 30 kPa was recorded in the carotid artery. At that same incident pressure, only 10 kPa was recorded in the cranial cavities of the thoracic-protected monkeys and 70 kPa in their thoracic chambers.
3.4 Cranial and shell strains
In Bolander’s study (Bolander, 2012), the goal was to compare cranial strains measured on different species exposed to similar blast conditions. For this reason, 10 in-vitro rats, 5 in-vitro swine and 4 PMHS-Brain were instrumented with strain gauges glued to the outer bone sections, and then placed in a forward orientation. Figure 6 summarizes the cranial strain extrema measured by frontal (A) and occipital (B) sensors. For all species, the maximum strains tend to increase with the incident pressure intensity. It can also be mentioned that the 4 PMHS-Brain were exposed with different orientations (forward, sideways, backward). The author showed that the strain profiles depend on both the location of the sensors on the cranium and its orientation (Bolander, 2012).
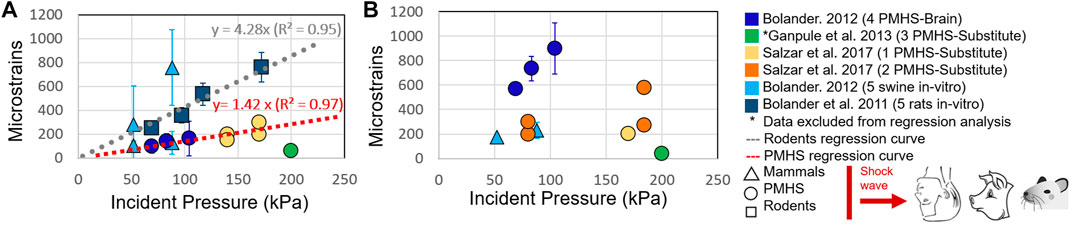
FIGURE 6. Cranial strains extrema measured on PMHS, swine and rodents during a forward exposure to blast conditions, plotted against incident pressures. (A) Frontal sensors measurements. (B) Occipital sensors measurements.
Additional data collected from two studies on PMHS-Substitutes (Ganpule et al., 2013a; Salzar et al., 2017) were also considered. The results recorded by Bolander (2012) and Salzar et al. (2017) showed a linear trend between frontal strains and incident pressures, as illustrated in Figure 6A (y = 1.42x, R2 = 0.97). Strains measured on 3 PMHS-Substitute by Ganpule et al. did not follow this trend (Ganpule et al., 2013a). These PMHS-Substitute were frozen for several months prior to the experiments, which may explain the observed discrepancies. Those results are thus discarded in the following. Indeed, the mechanical properties of cranial bones depend on the preservation conditions (Fallenstein et al., 1969; Delille, 2007). Furthermore, the PMHS trend is significantly lower than that of rodents’ (y = 4.28x, R2 = 0.95), suggesting that rodent craniums are more distorted by shock waves. Finally, the sensors located at the countercoup site, i.e., the occipital bone, recorded scattered maximum values with larger standard deviations, making it impossible to establish trend curves (Figure 6B).
In a second part, the cranial strains extrema obtained on PMHS are compared with data collected on substitutes. Eight geometric substitutes were instrumented with strain gauges and frontally exposed to blast conditions (Dal Cengio Leonardi, 2011; Dal Cengio Leonardi et al., 2011b; Goeller et al., 2012; Hua et al., 2014). Figure 7 shows the cranial strains extrema measured by the frontal and occipital sensors. Strains measured on substitutes are distributed over a wide range, following linear regression trends with a R2 of 0.80. This distribution of results can be explained by the different shell materials, geometries and dimensions. For frontal sensors, the linear trend followed by substitutes (y = 4.35x, R2 = 0.81) is much higher than that of PMHS, indicating that the substitutes tend to overestimate cranial strains. As for the occipital strains, values are slightly smaller than the frontal ones (y = 3.86x, R2 = 0.80).
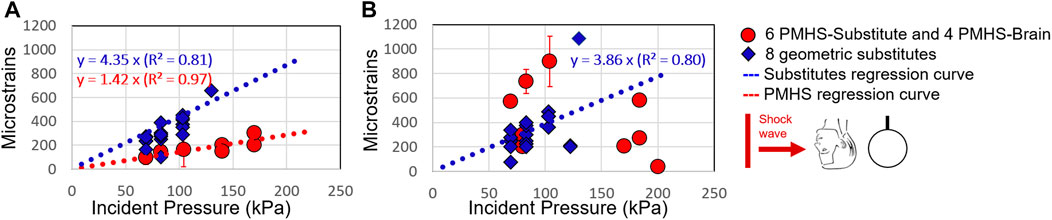
FIGURE 7. Cranial strains extrema measured on PMHS and substitutes during a forward exposure to blast conditions, plotted against incident pressures. (A) Frontal sensors measurements. (B) Occipital sensors measurements.
4 Discussion and relation to injury mechanisms
This review analyzed 43 studies performed on instrumented animals, PMHS and head substitutes subjected to air-propagated shock waves. The focus of this review was on the effects of a single quasi-ideal shock wave propagating through the head. Thus, the first step was to summarize the various studies to determine which ones could be compared. The exposure configurations were seldom similar from one author to another, whether in terms of test set-up (free field conditions, inside or outside shock tubes), or the orientation of the instrumented subjects towards the threat (forward, sideways, backwards). Finally, concerning the shock waves characteristics, the incident pressures chosen varied between 15 kPa and 1,000 kPa, the positive phase durations varied between 1 s and 10 s and were not systematically reported, and reflected and impulse pressures were rarely available. These disparities in exposure greatly modify the threat, and can thus lead to considerable experimental variability between the different studies. Furthermore, a large variability between instrumented subjects was also highlighted: in the case of living subjects, the first point to check was the instrumentation used (position of the device, sealing of the sensors, sampling frequency, data filtering, etc.). The second point of concern was the conditioning of the subjects, since some were exposed in-vivo and others in-vitro, sometimes with the removal of soft tissues or brain matter, and sometimes after the subjects had been thawed, thus modifying the mechanical properties of the tissues (Fallenstein et al., 1969; Delille, 2007). In the case of head substitutes, several types of geometry were considered (anthropomorphic or geometric), with varying dimensions that were not necessarily close to the 50th percentile human head geometry. In addition, the outer shell representing the cranium and the brain surrogates of the substitutes were made from different materials whose mechanical properties were not systematically specified.
Despite these latter experimental discrepancies, some tendencies were observed per species when correlating the measured mechanical metrics with the threat characteristics. Studies on PMHS have indeed shown an effect of sensor location and subject orientation on reflected pressures, ICP and cranial strains. An ipsi-controlateral phenomenon was also identified for ICP: in frontal orientation, for instance, there was an amplification of the first pressure peak relative to the incident pressure in the frontal position, whereas a negative peak pressure was recorded in the occipital position. For large mammals, an orientation effect was observed on reflected pressures, ICP, and cranial strains; as well as an ICP attenuation for all sensor locations and orientations with respect to incident pressures. For rodents, neither orientation nor sensor location effects were observed on ICP. These results could be explained by two factors. Either their cranial cavity is small enough to be unaffected by sensor localization effects, or the pressure sensors used are too large relative to the cranial volume of the rodent head. These initial trends led to the proposal of linear regression curves for reflected pressures, intracranial pressures, and cranial strains, with only incident pressure as input parameter. In the future, when new experiments will become available, other shock wave characteristics such as the positive phase duration or the positive impulse should be considered. Data resulting from the exposure of instrumented subjects to complex shock waves that do not follow a quasi-ideal waveform could also be incorporated (underwater detonation, repetitive blast exposure, two successive peak overpressures, etc.). It would also be interesting to scale the various trends based on geometric parameters of the different species (skull thickness, cranial volume, head mass, etc.) (Courtney and Courtney, 2015).
In a second part of the review, the ability of the existing head substitutes to reproduce human behavior in terms of reflected pressures, internal pressures and strains was questioned. Without complementary information from the authors, it was not possible to assess whether a specific ratio should be applied to the collected measurements. Thus, in the results section, the different metrics were compared to the PMHS data with a 1-1 ratio. Frontal reflected pressures measured on anthropomorphic substitutes followed a trend close to the PMHS data, but only the BI2PED created by Ouellet et al. compared its ISPs time-evolutions to those of PMHS and obtained similar results (Ouellet et al., 2014). Other studies using either geometric or anthropomorphic substitutes recorded ISP values spread over a wide range, due to disparities in the dimensions and material properties of the skull and brain substitutes. No trend could be established, however, several studies recorded an ipsi-controlateral effect between the frontal and occipital sensors when geometric (Dal Cengio Leonardi, 2011; Goeller et al., 2012; Hua et al., 2014) and anthropomorphic substitutes (Ouellet et al., 2014; Ouellet and Philippens, 2018) were exposed to blast waves in a forward orientation. In addition, further investigation into the variation of ISP in the presence of apertures did not reveal a clear trend. Finally, the current analysis suggests that the designed geometric substitutes overestimate the strain values compared to PMHS data, implying that they might not be properly dimensioned to reproduce the strain behavior of a human head subjected to blast conditions.
The remaining point to discuss is the plausibility of the various injury mechanisms that could explain blast-induced Traumatic Brain Injuries, bearing in mind that these mechanisms may not exclusive. Looking first at the indirect mechanisms, it appears that the relative brain/skull motion injury mechanism might not be caused directly by the primary blast phase, but rather results from acceleration effects that are generally attributed to the tertiary blast phase. Iwaskiw et al. implanted radiopaque markers in the brains of 4 PMHS-Brains (Iwaskiw et al., 2018) exposed in forward and backward orientations to incident pressures of 150 and 200 kPa. They measured peak displacements in the magnitude of 2—6 mm within the cerebral matter. These peak displacements were recorded only after the first 10 ms of the event, just as the PMHS heads began their rotational and translational motions. In addition, Knudsen and Øen (2003) exposed 37 Minke whales to underwater detonations and demonstrated that the induced cerebral lesions were similar to those observed in head impact injuries resulting from linear and angular acceleration. The thoracic compression hypothesis, in turn, which suggests that a deflection of the thorax could create a pressure surge propagating through the blood vessels to the brain, has yielded conflicting experimental results. In-vitro experiments carried out by exposing monkeys (Romba and Martin, 1961) and swine (Säljö et al., 2008) in configurations where the head was protected and the thorax exposed, showed that low ICPs were obtained despite large pressure peaks recorded in the thoracic cavity. These results are inconsistent with the supposed pressure wave propagating from the torso to the head. However, in the study by Rubio et al. (2020), 16 in-vivo rats were exposed to shock waves in a modified shock tube: the torsos of the animals were positioned inside the shock tube while the heads remained outside thanks to a hole. In this configuration, high pressure increases were recorded both in the thoracic cavity and in the carotid artery. Therefore, these different observations raise the question of the relevance of using post-mortem subjects to study the thoracic mechanism without homeostatic conditions.
Focusing next on the direct injury mechanisms, the transosteal wave propagation hypothesis suggests a propagation through the head layers due to acoustic impedance mismatches. This was first proposed by Clemedson and Pettersson (1955) while exposing rodents to the propagation of shock waves. In these experiments, it was observed that the shape of the ICP waveforms were similar to that of the incident pressures, with a slight difference in magnitude. According to the authors, the shock wave was thus only slightly modified as it propagated through the various head tissues (Clemedson and Pettersson, 1955). However, the current results showed that the recorded ICP time curves do not usually follow the incident pressures’ shapes. The linear trend curves established in this review showed an amplification of the frontal ICPs values against incident pressures for both PMHS and rodents. Due to the successive transmissions, reflections and absorptions of the wave as it propagates through the head layers, a pressure attenuation would be expected but some constructive interference might occur because of the head geometry (Sutar and Ganpule, 2019). On another note, supporting but insufficient results were found for the hypothesis of direct cranial wave entry through the various foramina. For a given incident pressure, ICPs measured during backward orientation of subjects were slightly lower than during forward orientation, suggesting that the presence of foramina may indeed facilitate the propagation of shock waves (Dal Cengio Leonardi, 2011). However, studies performed on substitutes and exposing them to shock waves in different orientations did not lead to substantial conclusions regarding the presence of apertures and its influence on the internal pressure variations (Alley et al., 2011; Dal Cengio Leonardi et al., 2011b). The third direct injury mechanism is the presumed cavitation of the cerebrospinal fluid in the contralateral head site. A depression peak was systematically observed in the occipital internal pressures of the PMHS during their forward exposure, as well as on some geometric and anthropomorphic substitutes. These recorded depression-overpressure cycles could indicate the formation and collapse of bubbles. Following this observation, Yu et al. created a transparent 1D model of the head with successive layers of a substitute cranium, CSF and cerebral matter to measure the occipital pressure and visualize the formation of bubbles in a fluid. The collapse of bubbles in the fluid correlated well with the depression peaks, supporting the CSF cavitation injury mechanism. Unfortunately, no clear correlation was established between the depression peaks and the formation of the cavitation bubbles (Yu et al., 2020; Yu et al., 2022). Lastly, the last injury mechanism is the skull deflection hypothesis, which is quite difficult to investigate when considering only time-domain strain data. Nevertheless, in this review, the peaks of cranial strains follow linear trends for PMHS, rodents and substitutes, indicating that the rodents’ craniums were more distorted by shock waves, possibly because of their lesser thickness. It remains to be proven, first, that these observed cranial strains are related to the cranial vibration modes, and second, that these skull deformations could actually cause cerebral lesions.
5 Conclusion
The current review first highlighted the limitations of the existing experimental studies, in which instrumented subjects were exposed to the propagation of quasi-ideal shock waves. Exposure conditions were widely variable from one study to another, suggesting that greater uniformity in future experimental setups would be appreciated. However, for the first time, intra-species trends were identified, showing the evolution of reflected pressure, internal pressure, and cranial deformation as a function of incident pressure. These initial trends may be more robustly complemented by further experimental studies, and extrapolation to other shock wave parameters as well as scaling ratios between species may be possible as more data become available.
Author contributions
NE contributed to the literature search, to the data collection, analysis and interpretation, and to the writing. JB and PM contributed equally to the data interpretation and made critical revisions to the manuscript. PN contributed to both the literature search and data interpretation. RW and CD both contributed to the literature search, study design and data interpretation. All authors contributed to the article and approved the submitted version.
Conflict of interest
The authors declare that the research was conducted in the absence of any commercial or financial relationships that could be construed as a potential conflict of interest.
Publisher’s note
All claims expressed in this article are solely those of the authors and do not necessarily represent those of their affiliated organizations, or those of the publisher, the editors and the reviewers. Any product that may be evaluated in this article, or claim that may be made by its manufacturer, is not guaranteed or endorsed by the publisher.
References
Ahlers, S. T., Vasserman-Stokes, E., Shaughness, M. C., Hall, A. A., Shear, D. A., Chavko, M., et al. (2012). Assessment of the effects of acute and repeated exposure to blast overpressure in rodents: Toward a greater understanding of blast and the potential ramifications for injury in humans exposed to blast. Front. Neurol. 3, 32. doi:10.3389/fneur.2012.00032
Alay, E., Skotak, M., Misistia, A., and Chandra, N. (2018). Dynamic loads on human and animal surrogates at different test locations in compressed-gas-driven shock tubes. Shock Waves 28 (1), 51–62. doi:10.1007/s00193-017-0762-4
Alley, M. D., Schimizze, B. R., and Son, S. F. (2011). Experimental modeling of explosive blast-related traumatic brain injuries. Neuroimage 54, S45–S54. doi:10.1016/j.neuroimage.2010.05.030
Armonda, R. A., Bell, R. S., Vo, A. H., Ling, G., DeGraba, T. J., Crandall, B., et al. (2006). Wartime traumatic cerebral vasospasm: Recent review of combat casualties. Neurosurgery 59 (6), 1215–1225. doi:10.1227/01.neu.0000249190.46033.94
Banton, R., Piehler, T., Zander, N., Benjamin, R., and Duckworth, J. (2018). “Comparison of numerical simulations with experiments of blast-induced pressure wave impact on a surrogate head model,” in Conference proceedings of the society for experimental mechanics series (Berlin, Germany: Springer), 181–187.
Belmont, P. J., Goodman, G. P., Zacchilli, M., Posner, M., Evans, C., and Owens, B. D. (2010). Incidence and epidemiology of combat injuries sustained during ‘the surge’ portion of operation iraqi freedom by a U.S. army brigade combat team. J. Trauma - Inj. Infect. Crit. Care 68 (1), 204–210. doi:10.1097/ta.0b013e3181bdcf95
Bolander, R. (2012). “A multi-species analysis of biomechanical responses of the head to a shock wave,” (United States: Wayne State University). Thesis.
Bolander, R., Mathie, B., Bir, C., Ritzel, D., and Vandevord, P. (2011). Skull flexure as a contributing factor in the mechanism of injury in the rat when exposed to a shock wave. Ann. Biomed. Eng. 39 (10), 2550–2559. doi:10.1007/s10439-011-0343-0
Bushby, K. M. D., Cole, T., Matthews, J. N. S., and Goodship, J. A. (1992). Centiles for adult head circumference. Arch. Dis. Child. 67 (10), 1286–1287. doi:10.1136/adc.67.10.1286
Champion, H. R., Bellamy, R. F., Roberts, C. P., and Leppaniemi, A. (2003). A profile of combat injury. J. Trauma - Inj. Infect. Crit. Care 54, S13–S19. doi:10.1097/01.ta.0000057151.02906.27
Chandra, N., and Sundaramurthy, A. (2015). “Acute pathophysiology of blast injury - from biomechanics to experiments and computations,” in Brain neurotrauma molecular, neuropsychological and rehabilitation aspects (Boca Raton (FL): CRC Press/Taylor), 199–258.
Chandra, N., Sundaramurthy, A., and Gupta, R. K. (2017). Validation of laboratory animal and surrogate human models in primary blast injury studies. Mil. Med. 182 (1), 105–113. doi:10.7205/milmed-d-16-00144
Chavko, M., Koller, W. A., Prusaczyk, W. K., and McCarron, R. M. (2007). Measurement of blast wave by a miniature fiber optic pressure transducer in the rat brain. J. Neurosci. Methods 159 (2), 277–281. doi:10.1016/j.jneumeth.2006.07.018
Chavko, M., Watanabe, T., Adeeb, S., Lankasky, J., Ahlers, S. T., and McCarron, R. M. (2011). Relationship between orientation to a blast and pressure wave propagation inside the rat brain. J. Neurosci. Methods 195 (1), 61–66. doi:10.1016/j.jneumeth.2010.11.019
Clemedson, C. J., and Pettersson, H. (1955). Propagation of a high explosive air shock wave through different parts of an animal body. Am. J. Physiol. 184, 119.
Courtney, A. C., and Courtney, M. W. (2009). A thoracic mechanism of mild traumatic brain injury due to blast pressure waves. Med. Hypotheses 72 (1), 76–83. doi:10.1016/j.mehy.2008.08.015
Courtney, A., and Courtney, M. (2015). The complexity of biomechanics causing primary blast-induced traumatic brain injury: A review of potential mechanisms. Front. Neurology 6. doi:10.3389/fneur.2015.00221
Dal Cengio Leonardi, A. (2011). “An investigation of the biomechanical response from shock wave loading to the head,” (United States: Wayne State University). Thesis.
Dal Cengio Leonardi, A., Bir, C. A., Ritzel, D. V., and VandeVord, P. J. (2011a). Intracranial pressure increases during exposure to a shock wave. J. Neurotrauma 28 (1), 85–94. doi:10.1089/neu.2010.1324
Dal Cengio Leonardi, A., Bir, C., Ritzel, D., and Vandevord, P. (2011b). “The effects of apertures on internal pressure measured during shock wave exposure,” in ASME Summer Bioengineering Conference, Farmington, Pennsylvania, USA, June 22–25, 2011.
Dal Cengio Leonardi, A., Keane, N. J., Bir, C. A., Ryan, A. G., Xu, L., and VandeVord, P. J. (2012). Head orientation affects the intracranial pressure response resulting from shock wave loading in the rat. J. Biomech. 45, 2595–2602. doi:10.1016/j.jbiomech.2012.08.024
Delille, R. (2007). “Contribution à la compréhension du comportement mécanique de l’os du crâne humain sous différents moyens de conservation et de sollicitation,” (France: de l’Université de Valenciennes et du Hainaut Cambrésis). Thèse.
Depalma, R. G., Burris, D. G., Champion, H. R., and Hodgson, M. J. (2005). Blast injuries. N. Engl. J. Med. 2005. doi:10.1056/NEJMra042083
DVBIC (2020). DoD TBI worldwide numbers. Available at: https://health.mil/Military-Health-Topics/Centers-of-Excellence/Traumatic-Brain-Injury-Center-of-Excellence/DOD-TBI-Worldwide-Numbers.
Fallenstein, G. T., Hulce, V. D., and Melvin, J. W. (1969). Dynamic mechanical properties of human brain tissue. J. Biomech. 2, 1–9. doi:10.1016/0021-9290(69)90079-7
Feng, K., Zhang, L., Jin, X., Chen, C., Kallakuri, S., Saif, T., et al. (2016). Biomechanical responses of the brain in swine subject to free-field blasts. Front. Neurol. 7, 179. doi:10.3389/fneur.2016.00179
Ganpule, S., Alai, A., Plougonven, E., and Chandra, N. (2013b). Mechanics of blast loading on the head models in the study of traumatic brain injury using experimental and computational approaches. Biomech. Model. Mechanobiol. 12 (3), 511–531. doi:10.1007/s10237-012-0421-8
Ganpule, S., and Chandra, N. (2013). Mechanics of interaction of blast waves on surrogate head: Effect of head orientation. New York City, U.S: ASME, 1–2.
Goeller, J., Wardlaw, A., Treichler, D., O’Bruba, J., and Weiss, G. (2012). Investigation of cavitation as a possible damage mechanism in blast-induced traumatic brain injury. J. Neurotrauma 29 (10), 1970–1981. –1981. doi:10.1089/neu.2011.2224
Got, C., Guillon, F., Patel, A., and Mack, P. (1983). “Morphological and biomechanical study of 146 human skulls used in experimental impacts, in relation with the observed injuries,” in 27th Stapp Car Crash Conference, San Diego, October 17-19, 1983.
Gullotti, D. M., Beamer, M., Panzer, M. B., Chia Chen, Y., Patel, T. P., Yu, A., et al. (2014). Significant head accelerations can influence immediate neurological impairments in a murine model of blast-induced traumatic brain injury. J. Biomech. Eng. 136, 091004. doi:10.1115/1.4027873
Haeussinger, F. B., Heinzel, S., Hahn, T., Schecklmann, M., Ehlis, A. C., and Fallgatter, A. J. (2011). Simulation of near-infrared light absorption considering individual head and prefrontal cortex anatomy: Implications for optical neuroimaging. PLoS One 6 (10), e26377. doi:10.1371/journal.pone.0026377
Hicks, R. R., Fertig, S. J., Desrocher, R. E., Koroshetz, W. J., and Pancrazio, J. J. (2010). Neurological effects of blast injury. J. Trauma - Inj. Infect. Crit. Care 68 (5), 1257–1263. doi:10.1097/ta.0b013e3181d8956d
Hua, Y., Akula, P. K., Gu, L., Berg, J., and Nelson, C. A. (2014). Experimental and numerical investigation of the mechanism of blast wave transmission through a surrogate head. J. Comput. Nonlinear Dyn. 9. doi:10.1115/1.4026156
Iwaskiw, A. S., Ott, K. A., Armiger, R. S., Wickwire, A. C., Alphonse, V. D., Voo, L. M., et al. (2018). The measurement of intracranial pressure and brain displacement due to short-duration dynamic overpressure loading. Shock Waves 28 (1), 63–83. doi:10.1007/s00193-017-0759-z
Josey, T., Donahue, L., Sawyer, T., and V Ritzel, D. (2016). “Blast response of a fluid-filled elastic shell,” in Proceedings of the MABS 24 (Military Aspects of Blast and Shock), Halifax.
Kabu, S., Jaffer, H., Petro, M., Dudzinski, D., Stewart, D., Courtney, A., et al. (2015). Blast-associated shock waves result in increased brain vascular leakage and elevated ROS levels in a rat model of traumatic brain injury. PLoS One 10 (5), e0127971. doi:10.1371/journal.pone.0127971
Kallakuri, S., Purkait, H. S., Dalavayi, S., Vandevord, P., and Cavanaugh, J. M. (2015). Blast overpressure induced axonal injury changes in rat brainstem and spinal cord. J. Neurosci. Rural. Pract. 06, 481–487. doi:10.4103/0976-3147.169767
Kinney, G. F. G., and Graham, K. J. K. (1985). Explosive shocks in air. Second edition. Berlin: Springer.
Knudsen, S. K., and Øen, E. O. (2003). Blast-induced neurotrauma in whales. Neurosci. Res. 46 (3), 377–386. doi:10.1016/s0168-0102(03)00101-9
Kuehn, R., Simard, P. F., Driscoll, I., Keledjian, K., Ivanova, S., Tosun, C., et al. (2011). Rodent model of direct cranial blast injury. J. Neurotrauma 28 (10), 2155–2169. doi:10.1089/neu.2010.1532
Lynnerup, N. (2001). Cranial thickness in relation to age, sex and general body build in a Danish forensic sample. Forensic Sci. Int. 117 (1–2), 45–51. doi:10.1016/s0379-0738(00)00447-3
Magnuson, J., and Ling, G. (2018). “Explosive blast mild traumatic brain injury,” in Traumatic brain injury - pathobiology, advanced diagnostics and acute management. doi:10.5772/intechopen.74035
McGruk, H., Castro, C. A., Cox, E., and Castro, T. (2008). Mild traumatic brain injury in U.S. Soldiers returning from Iraq. N. Engl. J. Med. 358 (5), 453–463. doi:10.1056/nejmoa072972
Merkle, A. C., Wing, I. D., Armiger, R. A., Carkhuff, B. G., and Roberts, J. C. (2009). Development of a human head physical surrogate model for investigating blast injury. ASME Int. Mech. Eng. Congr. Expo. Proc. 2, 91–93. doi:10.1115/IMECE2009-11807
Merkle, A. C., Wing, I. D., and Carneal, K. C. (2012). “The mechanics of brain motion during free-field blast loading,” in ASME 2012 Summer Bioengineering Conference, Fajardo, Puerto Rico Duration, Jun 20 2012 → Jun 23 2012, SBC2012–80880.
Merkle, A. C., Wing, I. D., and Roberts, J. C. (2010). “Human surrogate head response to dynamic overpressure loading in protected and unprotected conditions,” in IFMBE Proc, College Park, Maryland, USA, April 30 - May 2, 2010, 22–25.
Needham, C. E. (2010). Blast waves - shock wave and high pressure phenomena -. Berlin, Germany: Springer.
Ott, K., Voo, L., Merkle, A., Iwaskiw, A., Wester, B., and Armigir, R. (2013). “Experimental determination of pressure wave transmission to the brain during head-neck blast tests,” in ASME 2013 Summer Bioengineering Conference, SBC, Sunriver, Oregon, USA, June 26–29, 2013, SBC2013–14834.
Ouellet, S., Bir, C., and Bouamoul, A. (2014). Direct comparison of the primary blast response of a physical head model with post-mortem human subjects. Pass 2014.
Ouellet, S., and Philippens, M. (2018). The multi-modal responses of a physical head model subjected to various blast exposure conditions. Shock Waves 28 (1), 19–36. doi:10.1007/s00193-017-0771-3
Rafaels, K. A. (2010). “Blast wave attenuation: Ballistic protective helmets and the head,” in Personal Armour Systems Symposium, Quebec City, Canada, 13-17 September 2010.
Readnower, R. D., Chavko, M., Adeeb, S., Conroy, M. D., Pauly, J. R., McCarron, R. M., et al. (2010). Increase in blood-brain barrier permeability, oxidative stress, and activated microglia in a rat model of blast-induced traumatic brain injury. J. Neurosci. Res. 88 (16), 3530–3539. doi:10.1002/jnr.22510
Reneer, D. V., Crowdes, C. A., Ghosal, S., Corkins, J., Hisel, R. D., Lusk, B. T., et al. (2014). Extent of cerebrovascular disruption following blast exposure is influenced by the duration of the positive phase in addition to peak overpressure. J. Neurol. Neurophysiol. 05 (02). doi:10.4172/2155-9562.1000188
Reneer, D. V., Hisel, R. D., Hoffman, J. M., Kryscio, R. J., Lusk, B. T., and Geddes, J. W. (2011). A multi-mode shock tube for investigation of blast-induced traumatic brain injury. J. Neurotrauma 28 (1), 95–104. doi:10.1089/neu.2010.1513
Romba, J., and Martin, P. (1961). The propagation of air shock waves on a biophysical model. Technical memorandum, Armed Services Technical Information Agency, 17–61. Available at: https://apps.dtic.mil/sti/citations/AD0264932.
Rosenfeld, J. V., McFarlane, A. C., Bragge, P., Armonda, R. A., Grimes, J. B., and Ling, G. S. (2013). Blast-related traumatic brain injury. Lancet Neurology 12 (9), 882–893. doi:10.1016/s1474-4422(13)70161-3
Rubio, J. E., Skotak, M., Alay, E., Sundaramurthy, A., Subramaniam, D. R., Kote, V. B., et al. (2020). Does blast exposure to the torso cause a blood surge to the brain? Front. Bioeng. Biotechnol. 8, 573647. doi:10.3389/fbioe.2020.573647
Rubovitch, V., Ten-Bosch, M., Zohar, O., Harrison, C. R., Tempel-Brami, C., Stein, E., et al. (2011). A mouse model of blast-induced mild traumatic brain injury. Exp. Neurol. 232 (2), 280–289. doi:10.1016/j.expneurol.2011.09.018
Rude, G. (2016). “Methodologies and gauges for intracranial pressure measurements,” in PASS 2016 Personal Armour Systems Symposium, Amsterdam, Netherlands, September 2016.
Säljö, A., Arrhén, F., Bolouri, H., Mayorga, M., and Hamberger, A. (2008). Neuropathology and pressure in the pig brain resulting from low-impulse noise exposure. J. Neurotrauma 25 (12), 1397–1406. doi:10.1089/neu.2008.0602
Säljö, A., Bao, F., Haglid, K. G., and Hansson, H.-A. (2000). Blast exposure causes redistribution of phosphorylated neurofilament subunits in neurons of the adult rat brain. New York, United States: Mary Ann Liebert, Inc.
Salzar, R. S., Treichler, D., Wardlaw, A., Weiss, G., and Goeller, J. (2017). Experimental investigation of cavitation as a possible damage mechanism in blast-induced traumatic brain injury in post-mortem human subject heads. J. Neurotrauma 34 (8), 1589–1602. doi:10.1089/neu.2016.4600
Selvan, V., Ganpule, S., Kleinschmit, N., and Chandra, N. (2013). Blast wave loading pathways in heterogeneous material systems-experimental and numerical approaches. J. Biomech. Eng. 135, 61002–61014. doi:10.1115/1.4024132
Ganpule, S., Salzar, R., and Chandra, N., “Response of post-mortem human head under primary blast loading conditions-effect of blast overpressures,” ASME Int. Mech. Eng. Congr. Expo. Proc., November 12-17, 1995, San Francisco 2013a.
Shah, A. S., Stemper, B. D., Yoganandan, N., Pintar, F. A., Rangarajan, N., Hallman, J., et al. (2011). “Methodology to study attenuation of a blast wave through the cranium,” in ASME 2011 International Mechanical Engineering Congress & Exposition IMECE2011, Denver, Colorado, USA, November 11–17, 2011, 1–8.
Shridharani, J. K., Wood, G. W., Panzer, M. B., Capehart, B. P., Nyein, M. K., Radovitzky, R. A., et al. (2012). Porcine head response to blast. Front. Neurol. 3, 1–12. doi:10.3389/fneur.2012.00070
Skotak, M., Wang, F., Alai, A., Holmberg, A., Harris, S., Switzer, R. C., et al. (2013). Rat injury model under controlled field-relevant primary blast conditions: Acute response to a wide range of peak overpressures. J. Neurotrauma 30 (13), 1147–1160. doi:10.1089/neu.2012.2652
Skotak, M., Townsend, M. T., Alay, E., and Chandra, N. (2019). “The effect of geometrical factors on the surface pressure distribution on a human phantom model following shock exposure: A computational and experimental study,” in Fracture mechanics applications (London, UK: Intech Open).
Sochet, I. (2017). Shock wave and high pressure phenomena - blast effects - physical properties of shock waves. Berlin, Germany: Springer.
Sundaramurthy, A., Alai, A., Ganpule, S., Holmberg, A., Plougonven, E., and Chandra, N. (2012). Blast-induced biomechanical loading of the rat: An experimental and anatomically accurate computational blast injury model. J. Neurotrauma 29 (13), 2352–2364. doi:10.1089/neu.2012.2413
Sutar, S., and Ganpule, S. (2019). Investigation of wave propagation through head layers with focus on understanding blast wave transmission. Biomech. Model. Mechanobiol. 19, 875–892. doi:10.1007/s10237-019-01256-9
Trudeau, D. L., Anderson, J., Hansen, L. M., Shagalov, D. N., Schmoller, J., Nugent, S., et al. (1998). Findings of mild traumatic brain injury in combat veterans with PTSD and a history of blast concussion. J. Neuropsychiatry Clin. Neurosci. 10 (3), 308–313. doi:10.1176/jnp.10.3.308
Varas, J. M., Philippens, M., Meijer, S. R., van den Berg, A. C., Sibma, P. C., van Bree, J. L., et al. (2011). Physics of IED blast shock tube simulations for mTBI research. Front. Neurol. 2, 1–14. doi:10.3389/fneur.2011.00058
Walls, M. K., Race, N., Zheng, L., Vega-Alvarez, S. M., Acosta, G., Park, J., et al. (2016). Structural and biochemical abnormalities in the absence of acute deficits in mild primary blast-induced head trauma. J. Neurosurg. 124 (3), 675–686. doi:10.3171/2015.1.jns141571
Warden, D. L., French, L. M., Shupenko, L., Fargus, J., Riedy, G., Erickson, M. E., et al. (2009). Case report of a soldier with primary blast brain injury. Neuroimage 47 (2), T152–T153. doi:10.1016/j.neuroimage.2009.01.060
Wojcik, B. E., Stein, C. R., Bagg, K., Humphrey, R. J., and Orosco, J. (2010). Traumatic brain injury hospitalizations of U.S. army soldiers deployed to Afghanistan and Iraq. Am. J. Prev. Med. 38 (1), S108–S116. doi:10.1016/j.amepre.2009.10.006
Yu, X., Sharp, D., and Ghajari, M. (2020). “Experimental study of cerebrospinal fluid (CSF) cavitation in blast- and impact-induced traumatic brain injury,” in In Proceedings of International Conference on the Biomechanics of Impact, July 20-24, 2020, pp. 145–148.
Yu, X., Wu, T., Nguyen, T. T. N., and Ghajari, M. (2022). Investigation of blast-induced cerebrospinal fluid cavitation: Insights from a simplified head surrogate. Int. J. Impact Eng. 162, 104146. doi:10.1016/j.ijimpeng.2021.104146
Zhu, F., Wagner, C., Dal Cengio Leonardi, A., Jin, X., VandeVord, P., Chou, C., et al. (2012). Using a gel/plastic surrogate to study the biomechanical response of the head under air shock loading: A combined experimental and numerical investigation. Biomech. Model. Mechanobiol. 11 (3–4), 341–353. doi:10.1007/s10237-011-0314-2
Keywords: experimental testing, injury mechanisms, literature review, primary blast, Traumatic Brain Injury
Citation: Elster N, Boutillier J, Magnan P, Naz P, Willinger R and Deck C (2023) A critical review of experimental analyses performed on animals, post-mortem human subjects, and substitutes to explore primary blast-induced Traumatic Brain Injuries. Front. Mech. Eng 9:1185231. doi: 10.3389/fmech.2023.1185231
Received: 13 March 2023; Accepted: 13 July 2023;
Published: 28 July 2023.
Edited by:
Majid Baniassadi, University of Tehran, IranReviewed by:
Yuan Feng, Shanghai Jiao Tong University, ChinaMohammad Ali Nazari, University of Tehran, Iran
Copyright © 2023 Elster, Boutillier, Magnan, Naz, Willinger and Deck. This is an open-access article distributed under the terms of the Creative Commons Attribution License (CC BY). The use, distribution or reproduction in other forums is permitted, provided the original author(s) and the copyright owner(s) are credited and that the original publication in this journal is cited, in accordance with accepted academic practice. No use, distribution or reproduction is permitted which does not comply with these terms.
*Correspondence: Natacha Elster, TmF0YWNoYS5lbHN0ZXJAaXNsLmV1