- 1Department of Mechanical Engineering, Faculty of Engineering, Universitas Muria Kudus, Kudus, Indonesia
- 2Department of Mechanical Engineering, Faculty of Engineering, Diponegoro University, Semarang, Indonesia
- 3Department of Industrial Engineering, Faculty of Industrial Technology University of Atma Jaya Yogyakarta, Yogyakarta, Indonesia
Bone disease and fractures are among the health issues that are becoming more prevalent year after year. A mandibular disorder is caused by an accident or disease to the largest and strongest bone in the human face. Because the natural healing process of mandibular bones takes a long time, a bone grafting procedure is used to speed up the patient’s recovery. Due to the limitations of bone grafting processes such as autographs, allographs, and xenografts, bone replacement is being developed using biomaterials via 3D printing. The purpose of the review was to evaluate research on the use of 3D printing in the replacement of mandible bones. The search algorithm found as many as 2,941 articles at the start of the search and 123 articles after initial selection. Up to February 2022, the Scopus electronic database was used to conduct the literature search. This research includes publications that employ 3D printers, additive manufacturing, or finite element analysis to build or analyze mandibular implants. Paper topics in engineering, materials science, biochemistry, genetics, molecular biology, medicine, dentistry, chemical engineering, and computer science are included in this study. Papers in physics, astronomy, and energy, book chapter document types, papers reviews, and documents in languages other than English were excluded from this study. After an initial screening that included the year, publication stage, source type, and language, as many as 70 articles were obtained, and after filtering titles and abstracts obtained 55 articles. After the full-text selection was obtained, 32 articles were included in this review. Some articles were unacceptable because the topics discussed were unrelated to mandibular bone scaffolds. As a result, the field of additive manufacturing for the repair and reconstruction of mandibular defects necessitates the development of novel tools and methodologies. A customized biological scaffold can be created using an appropriate 3D printing process based on the characteristics of various mandibular defects, allowing it to be perfectly matched to the defect region and reducing stress, thereby improving the scaffold’s healing function.
Introduction
The mandible is the lower third of the face’s bone structure. It is concerned with preserving the patient’s face contour and shape, as well as their chewing, articulation, and speaking. Bone tissue defects can be caused by trauma, tumors, infections, genetic illnesses, periodontitis, and iatrogenic injury (Zhang et al., 2019). Patients will experience distress and trauma as a result of mandibular deficiency. The majority of these people are more susceptible to a sense of beauty, and their emotions are quite sensitive, which can lead to pessimistic thoughts (Malá et al., 2015). Autograft, xenograft, and artificial materials are used to repair the mandibular defect. Autografts are graft materials derived from the same person and have long been thought to be the “gold standard.” (Misch, 2010). Autograft has a significant osteogenic effect and is frequently used as a bone substitute. On the other hand, autografts have several disadvantages, including increased donor site stress and limited bone availability (Shibuya and Jupiter, 2015). Allografts are graft materials from the same donor species that can be fresh/frozen, freeze-dried, or demineralized freeze-dried (Eppley et al., 2005). Because of the presence of proteins such as bone morphogenetic proteins, these allografts can function as both osteoconductive and osteoinductive scaffolds. Xenografts, which are derived from another species, are commonly used in therapeutic periodontal regeneration. However, xenografts have several disadvantages, including immunological rejection, ethical concerns, and poor osseointegration (Sheikh et al., 2017).
For bone tissue regeneration (BTR), the interaction of osteogenic cells, mechanical and structural properties of the surrounding extracellular matrix (ECM), and an ion- and growth factor-containing milieu are required (Szpalski et al., 2012). Because of the rapid development of biomaterials and the need for modern regenerative medicine, researchers have become more interested in investigating bone substitute biomaterials used for mandibular defects in recent years. Figure 1 shows a diagram of the biomaterial that can repair and rebuild a mandibular defect with 3D Printing. Biomaterials are not only biologically active or perform specific biological functions but are also extremely safe to use. Many biomaterials, however, can repair mandible defects, and their properties vary. Here they can be classified into three types: metal materials (such as titanium and its alloys), inorganic materials (such as bioactive ceramics, hydroxyapatite, and so on), and organic materials (Zhang et al., 2019). Metallic materials commonly used for mandibular defect implantation include 316 L stainless steel (ASTM F138), cobalt chromium-based alloys (primarily ASTM F75 and ASTM F799), and titanium alloys (Alvarez and Nakajima, 2009). Polymeric materials used as bone substitutes have a high degree of flexibility and help to prevent metal and bioceramic implant stress. By modifying their mechanical and degradation properties, they can be customized and targeted to implant-specific tissue (Pielichowska and Blazewicz, 2010). Bioactive ceramics can cause organisms to build new bone and have partial or complete absorption. When used as a scaffold, bioactive ceramics have bone conductivity and osteogenesis on their surface; they can also cover or fill bone deficiencies. As a result, these materials can potentially replace bones (Ciocca et al., 2013).
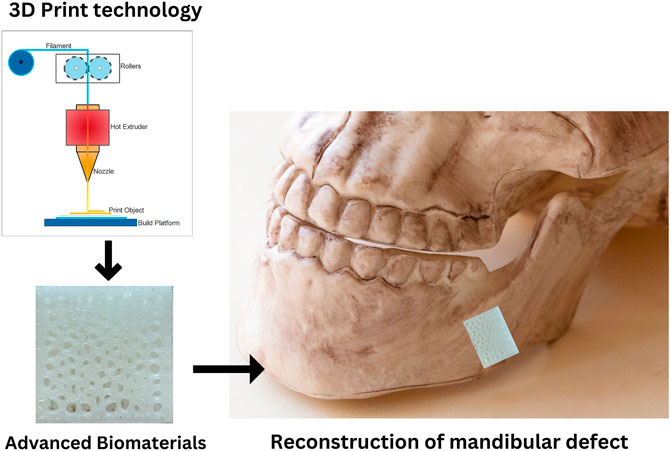
FIGURE 1. Biomaterial schematic for mandibular defect reconstruction. Adapted from Zhang et al. (2019).
Using additive manufacturing or 3D printing technology, the bone scaffold may be constructed rapidly and with the desired materials (Winarso et al., 2022). Various additive manufacturing methods for bone tissue engineering have recently been used. SLA, FDM, DMLS, 3DP, SLM, PolyJet Technology, and EBM are some methods used (Miljanovic et al., 2020). Mandibular bone replacement surgery has been the subject of increasing research. However, reviews on additive manufacturing in mandibular implants are still uncommon. This review aims to systematically map the research on additive manufacturing technology applied to the reconstruction of mandibular implants. However, different additive manufacturing processes may be used to repair mandible defects, and their quality varies. As a result, the emphasis of this review is on the technique’s application in this context.
Methods
Protocol
The PRISMA Extension for Scoping Reviews (PRISMA-ScR): A checklist and Explanation protocol were used to conduct this review (Tricco et al., 2018).
Eligibility criteria
In this review, eligibility criteria include both inclusion and exclusion criteria. All papers that investigate the use of 3D printer technology, additive manufacturing, or finite element analysis to produce or analyze mandibular implants are eligible for this study. Some topics covered include engineering, materials science, biochemistry, genetics, molecular biology, medicine, dentistry, chemical engineering, and computer science: only English journal articles and conference papers we included.
Papers in physics, astronomy, and energy, book chapter and review document types, documents in languages other than English were excluded from this study.
Information sources
The Scopus electronic database was used to conduct the literature search. This study did not include papers published after February 2022.
Search
The Scopus electronic database search strategy employed a combination of keywords with three criteria: method of creation and analysis, site or area, and form morphology. Table 1 displays the entire search strategy. The keywords were chosen and incorporated so that the scope of the field of study is broad, thorough, and accessible in determining papers relevant to the research theme; from the process, 134 documents consistent with keywords were obtained.
Selection of sources of evidence
The paper selection procedure was divided into five stages: keyword selection, tool limitation, title selection, abstract selection, and full-text selection. Keywords selection and tool limitation is directly generated from the Scopus database. Two reviewers assessed titles, abstracts, and full-text selection for inclusion and exclusion. Discussions between reviewers were used to settle disagreements. Table 2 shows a selection using limitation tools.
Data charting
Two reviewers designed a data-charting form to decide which variables should be extracted. Two reviewers abstracted the data into a priori-designed Microsoft Excel spreadsheet. Using a random spot-check approach, the reviewer validated each other’s data abstraction. Discussions amongst reviewers were used to settle disagreements until an agreement was achieved.
Data items
The extracted data contained authors, year, materials, additive manufacturing methods, model geometry, structural properties, mechanical properties, in vitro and in vivo testing. The extracted data is then classified by the material used, additive manufacturing method, microarchitecture model, and investigation process.
Results and discussion
The search algorithm first discovered 2,941 articles (combination 1 and 2 keywords), and 134 complete texts were collected using the second search algorithm (combination of all search keywords). The selection process, which included the use of limitation tools, yielded 75 papers. It has been shown from 75 articles that the frequency of publications has steadily increased since 2016, indicating rising interest in the topic, as shown in Figure 2. Although three countries have dominated the majority of articles, namely China, the United States, and South Korea, other countries such as Germany, India, Italy, the Netherlands, Australia, Taiwan, and others show the same interest. The subject area is not only dominated by a single field but also encompasses various disciplines such as engineering, material science, medicine, and others. Mandibular implant manufacturing is one of the intriguing issues for researchers to be developed in the future.
Titles and abstracts were chosen to determine the article’s relevance. Some articles were unacceptable because the topics discussed were unrelated to mandibular bone scaffolds, such as the temporomandibular joint and femoral osteofixation plate. The final step was to select full text to further clarify the paper’s relevance to the study’s theme, which included 32 papers. Figure 3 depicts the flowchart for article process selection. Table 3 contains detailed information on each study.
The classification of paper synthesis was influenced by the material used, additive manufacturing method, microarchitecture model, and investigation process. The element method’s application in the design process, as well as scaffold optimization, were investigated. Furthermore, polycaprolactone (PCL) accounts for 31% of the material used in the development of mandibular implants, titanium alloy (Ti6Al4V) accounts for 22%, and calcium phosphate (CP) accounts for 13%. Other materials used to create mandibular bone grafts include polylactic acid (PLA), polylactic glycolic acid (PLGA), boron-containing bioglass (B-BGs), hydroxyapatite (HA) + tricalcium phosphate (TCP), and polyether ketone (PEKK), as shown in Figure 4.
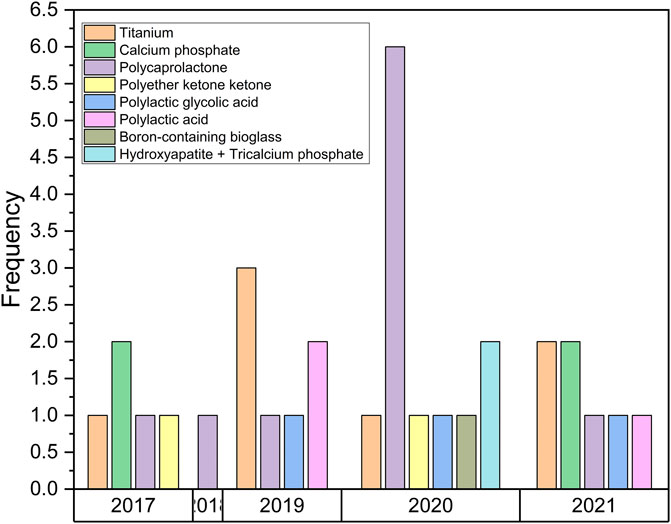
FIGURE 4. Material classificat the microarchitecture of the bone scaffold ion for the mandibular implant.
One parameter that influences biological performance and mechanical properties is the microarchitecture of the bone scaffold. The microarchitecture of the bone scaffold is divided into strut-based (periodic lattice) structures (e.g., cubic, BCC, FCC, and Voronoi structures) and shell-based structures (e.g., Triply Periodic Minimal Surface/TPMS). Any three-dimensional structure composed of nodes and struts is referred to as a “lattice structure” (Sun et al., 2020). Level-set equations and specific trigonometric equations determine TPMS structure, which includes gyroid, primitive, and diamond structures (Alizadeh-Osgouei et al., 2021). Figure 5 depict the microarchitecture of the bone scaffold design (a. cubic, b. BCC, c. FCC, d. Voronoi, e. primitive, f. gyroid, and g. diamond structures). According to the research, the microarchitecture used to develop the mandibular implant included a porous structure (94 percent of the remaining bulk structure). The porous structure has beneficial properties such as porosity, pore size, and pore interconnectivity, which significantly impact its biological and mechanical capabilities (Yang et al., 2019). Appropriate pore size and porosity, in particular, improve nutrient absorption and waste outflow, resulting in an ideal environment for bone scaffold (He et al., 2017).
Further, this study’s investigation process can be divided into three categories: Structural/mechanical, in vitro, and in vivo investigation. High porosity, pore, interconnectivity, hierarchical structure, and nano topography were among the structural properties. Young modulus, compressive strength, and adequate stiffness were mechanical properties. In vitro and in vivo biological properties included biodegradability, bioresorbability, biocompatibility, and non-toxicity (Kanwar and Vijayavenkataraman, 2021). Most articles (25 percent) conduct three types of investigations in their research. Some studies combined two investigations, while others used a single analysis. For the design process and scaffold bone optimization, 19% of articles use the finite element method (Figure 6).
In this review, all mandibular implants are made using additive manufacturing method. Table 4 summarizes the main reasons for using additive manufacturing in oral implants. The most common method was 3D printing/bioprinting, which was followed by fused deposition modeling (FDM), electron beam melting (EBM), robocasting, selective laser sintering (SLS), and selective laser melting (SLM), as shown in Figure 7.
Numerous scaffold materials, either alone or in combination, have been proposed to incorporate a variety of properties, including structural, mechanical, and biological properties. One of the most commonly used materials for mandibular implants is polycaprolactone (PCL). They have several advantages, including thermoplastic properties and recessions for 3D printing scaffold bone layers, biodegradability, and biocompatibility. Furthermore, PCL is a safe material that has been approved by the FDA for use in drug delivery devices and scaffold implants (Park et al., 2018).
Even with a design that reduced stress concentrations significantly, PCL alone was not strong enough to withstand the applied forces (Prasadh et al., 2020). Incorporating HA nanoparticles may improve the biological and mechanical properties of PCL scaffolds (Fucile et al., 2020). Mandibular reconstruction capability was demonstrated by 3D-printed PCL/-TCP scaffolds (Lee et al., 2020). A polycaprolactone/beta-tricalcium phosphate prosthesis implanted with tonsil-derived mesenchymal stem cells (TMSCs) demonstrated osteogenic potency, implying that their combination could allow for effective bone repair (Park et al., 2020). In terms of new bone development, the PCL with a broad lattice had an advantage. Although mechanical tests revealed that PCL scaffolds with a wider lattice performed worse than those with a narrower lattice, the large interconnected pore size may stimulate cell proliferation and osteogenic differentiation for new bone formation (Park et al., 2018). A 3D-printed polycaprolactone (PCL)/b-tricalcium phosphate (b-TCP) scaffold conjugated with rhBMP-2 can be used as an implant surgical guide and bone transplant in a major bone defect site (Bae et al., 2017). The injection of adipose-derived stem cells (ADSC) in conjunction with the 3D-printed PCL/TCP loaded with bdECM improved mandibular ossification in and around the pores of the scaffold (Lee et al., 2021). Polycaprolactone and beta-tricalcium phosphate scaffolds were found to be effective in delivering rhBMP-2 while allowing for bone growth in mandibular bone defects (Park et al., 2021).
Recently, titanium and its alloys, stainless steel, and cobalt-chromium alloys have all proven to be promising clinical candidates for metallic biomaterials for additive manufacturing (AM) (Pellizzari et al., 2020). Titanium-based bone substitutes have high mechanical strength and biocompatibility and have been widely used in biomedical applications to replace defective human hard tissue (ming Peng et al., 2021). Titanium and its alloys are frequently used for biomaterials bone implantation due to their advantageous properties (high strength-to-weight ratio, lightweight, biocompatibility, corrosion resistance, osteoconductive qualities, and good tissue integration) (Pellizzari et al., 2020). The main issue with titanium alloy implants in clinical practice is a mismatch between the elastic modulus of titanium alloy and bone tissue, which causes stress shielding, impairs load transfer, and ultimately reduces the success rate of recovery (Civantos et al., 2019). However, due to the risk of metal exposure and stress shielding caused by high elastic modulus, long-term stability and occlusal restoration are not possible (Kumar et al., 2016). To eliminate stress shielding at the bone-implant interface, an isotropic material with adjustable porosity or relative density could be incorporated to change the corresponding Young’s modulus and yield stress (Gibson, 2021). Long-term bone fixation and full bone ingrowth can be achieved with the titanium porous structure scaffold (Moiduddin et al., 2019). Macroporosity reduces the weight of the prosthesis and allows for new bone ingrowth via bone grafts (Farajpour et al., 2021). Combining a porous structure and the topology technique is an excellent way to improve titanium material’s mechanical stability and bone growth. Table 5 summarizes the benefits and drawbacks, current advances and achievements, and current limitations or challenges of PCL composites and Ti alloys.
The manufacturing process of mandibular implants
Bioprinting
In 3D printing (3DP), an ink-jet head deposits a binder substance on a powder (Moreno Madrid et al., 2019). The basic idea behind 3D printing is to read data from a Computer Aided Design (CAD) model and deposit material layers sequentially to create a three-dimensional model (Rengier et al., 2010). 3DP’s ability to process a wide range of ceramic, metallic, polymeric, and composite materials is a significant advantage. The only stipulation is that the substance be in powder form. Successful manufacturing, on the other hand, necessitates careful binder selection and process parameter optimization. As potential BTE scaffold materials, polymeric, ceramic, and composite powder materials have been investigated (Jariwala et al., 2015).
In particular, polycaprolactone is a material that is frequently used in the fabrication of mandibular bone scaffolds using a bioprinting system. Bioprinting was used by Bae et al. (2017) to evaluate the 3D-printed PCL/b-TCP/bdECM scaffold conjugated with RHBMP-2. In a major bone defect site, a 3D-printed scaffold with rhBMP-2 can be used as an implant surgical guide and a bone graft. Park et al. (2018) investigated the efficacy of a three-dimensional polycaprolactone (3D PCL) scaffold implant created using a 3D bioprinting system. These results demonstrated that 3D-printed porous PCL scaffolds could induce alveolar bone regeneration for defect healing in dentistry. Park et al. (2020) investigated the efficacy of a polycaprolactone/beta-tricalcium phosphate prosthesis implanted with tonsil-derived mesenchymal stem cells (TMSCs). The outcome demonstrates that their scaffold design is effective in osteogenesis. Zamani et al. (2020) investigated the effect of void sizes and build orientation on the mechanical properties of a polycaprolactone bone scaffold used for mandibular bone tissue engineering. According to the results, all scaffold types have higher compressive strength in the building direction than in the side direction. The compressive strength of the homogeneous scaffolds decreased as the void size of the scaffold increased. Park et al. (2018) used 3D-printed scaffold technology to deliver rhBMP-2 to bone defects in their study. Their findings showed that PCL/T50 scaffolds were effective at delivering rhBMP-2 while also preserving space for bone growth in mandibular bone defects.
On the other hand, poly (lactic-co-glycolic acid (PLGA) is a linear copolymer that can be made with different ratios of its constituent monomers, lactic acid (LA) and glycolic acid (GA). Composite materials such as PLGA and hydroxyapatite (HA) are commonly used in bone tissue engineering. This composite has advantages such as non-toxicity, bioactivity, osteoconductivity, resemblance to natural bone minerals, and the ability to be produced using 3D printing (Gentile et al., 2014). Deng et al. (2019) use bioprinting to create a PLGA/NHA scaffold containing BMP-2 Cell Growth Factor and then test it in vitro and in vivo. The results show that the PLGA/nHA/CS/rhBMP-2 scaffold complex successfully inhibited the early burst effect of rhBMP-2. Chang et al. (2021a) investigated the feasibility of using a biomaterial made of 3D-printed hydroxyapatite (HA) and poly (lactic-co-glycolic acid (PLGA) scaffold as a biomaterial for in vivo guided bone regeneration (GBR). The 3D-printed hydroxyapatite (HA) and poly (lactic-co-glycolic acid (PLGA) scaffold demonstrated adequate dimensional stability as well as excellent osteoregenerative capacity, satisfying the need for GBR.
Instead, calcium phosphate cement (CPC) is a manufactured bone substitute that forms hydroxyapatite and mimics the inorganic component of human bone. CPC has been approved by the Food and Drug Administration (FDA) to treat craniofacial defects and bone fractures (Xu et al., 2017). CPC is osteoconductive, which means it can direct the formation and multiplication of osteoblasts on its surface (Marongiu et al., 2020). One of the benefits of CPC is its injectability; it can be manufactured using 3D printing. Muallah et al. (2021) investigated the mechanical properties and cell migration of 3D-printed CPC cubes with varying pore sizes and printing orientations compared to commercially available bone substitutes. According to the findings, scaffolds should be printed with a pore size of 490 m for locations subjected to high pressure, and scaffolds should be printed with a pore size of 750 m for non-high-pressure areas. Thomas and Singh (2021) (Jariwala et al., 2015) used 3D printing to create a calcium phosphate-based bone bio-composite. According to the findings, the mechanical properties of the composite bone implant are comparable to those of natural bone.
On the other hand, Zhang et al. (2020) used boron-containing bioactive glass and three-dimensional (3D) printing technology to create an osteoinductive implant scaffold based on CT scan imaging instructions for bone defects. By providing transport channels for nutrients and metabolites, the pore structure promotes cell proliferation and bone repair. Chang et al. (2021b) investigate the feasibility of using hyperplastic biomaterials to create a scaffold for dentoalveolar apparatus regeneration (HB). The HB scaffold was created with microextrusion technology and implanted into a large prosthetic jaw bone defect. They measured osteoregenerative capacity, bone–scaffold integration, and tissue response to HB.
Fused deposition modeling (FDM)
FDM is an additive manufacturing method that layers a fused material to create parts (Sai and Yeole, 2001). FDM can produce structures with several hundred micrometres strut sizes, with the nozzle diameter and extrusion rate controlling most of the process. Several factors influence the resolution provided by FDM manufacturing equipment, which determines the quality of the resulting scaffold (Houben et al., 2017). FDM technology can reconstruct mandibular bone defects and is primarily used to process low-fusing polymers such as PCL, PLA, and each composite. Wulandari et al. (2019) used FDM technology to create a 3D-printed PLA scaffold with a hydroxyapatite-chitosan coating for mandibular reconstruction. For mandible rebuilding, the best hydroxyapatite-chitosan concentration as a coating material on PLA scaffold created using the FDM process is 70:30. Bouyer et al. (2021) used FDM technology to create a new critical size mandibular bone scaffold made of clinical-grade polylactic acid (PLA). A polyelectrolyte film is applied to the scaffold, delivering an osteogenic bioactive molecule (BMP-2). The results suggested that three-dimensional printed PLA scaffolds supplemented with low doses of BMP-2 could be a safe and simple treatment for major bone deformities seen in the clinic.
FDM has also been used to create polymer and ceramic composite scaffolds. Park et al. (2020) used FDM technology to create a suitable graft material for mandibular bone reconstruction using double-layered polycaprolactone (PCL)/tricalcium phosphate (TCP). The findings show that the dual-layered scaffold with an integrated body and supporting plate, as well as a microporous structure that facilitates osteoconduction and osteoinduction, effectively promoted new bone formation. Lee et al. (2020) created polycaprolactone/beta-tricalcium phosphate (PCL/-TCP) scaffolds using a multi-head FDM technique. Within the limitations of this study, 3D-printed PCL/- TCP scaffolds demonstrated sufficient promise for mandibular reconstruction. Lee et al. (2021) used FDM technology to create a PCL/TCP artificial scaffold. To improve osteoconductivity and osteoinductivity, the artificial scaffold is coated with bone demineralized and decellularized extracellular matrix (bdECM). The fixation of bdECM-coated 3D PCL/TCP scaffold with adipose-derived stem cells (ADSC) aggregates was validated as a simple and effective strategy for ossifying bone defect regions in this study. Hikmawati et al. (2019) designed the pore unit and size geometry of the scaffold, which was made from FDM with PLA and HA filaments. Both PLA and PLA-PCL-HA scaffolds were found to be biodegradable, and compressive strength is determined by pore size design. Using an optimized design technique for FDM technologies, Fucile et al. (2020) created the first PCL/HA nanocomposite scaffolds for complex tissue regeneration. The addition of HA nanoparticles to PCL scaffolds may improve their biological and mechanical properties.
FDM has recently incorporated polymers with a relatively high melting point. For example, a polyether ketone (PEKK) scaffold with an improved melting point between 330°C and 340°C was created using a self-designed FDM technique (Vaezi and Yang, 2015). Cheng et al. (jie Cheng et al., 2020) investigated the mechanical properties of a 3D-printed topologically optimized mandibular bone block containing polyether ketone PEKK) for mandibular surgical reconstruction. The biomechanical properties of the two models were compared using the finite element method (FEM). According to the FEM results, the topology optimized model’s maximum stresses and displacements were significantly lower than those with fibular bone graft.
Electron beam melting (EBM) technology
Among the various additive techniques, electron beam melting (EBM) has been hailed as a quick and efficient method for producing custom-designed metallic implants that have been approved by the Food and Drug Administration (FDA) and the Conformité Européenne (CE) (Chua et al., 2017). An extensive study on the application of EBM to the production of porous metal scaffolds has been conducted. Yan et al. (2018) use 3D reconstruction and EBM technology to develop a 3D mesh internal titanium scaffold for mandibular defect repair. The findings suggest that the titanium scaffold mesh created by EBM is biocompatible. Creating a personalized implant makes it easier for surgeons to perform a procedure, reducing surgery time. Moiduddin et al. (2020) evaluated two designs for customized titanium prosthesis scaffolds manufactured by EBM for mandibular continuity. This study demonstrated the feasibility and utility of tailored porous titanium implants in mandibular reconstruction. Moiduddin et al. (2019) used EBM to automate the extraction of the tailored titanium lattice implant for the large mandibular deformity. According to the EDS and -CT scan results, the EBM-fabricated lattice reconstruction plate had a consistent network of channels that were free of cracks and contaminants. Furthermore, the EBM-fabricated lattice reconstruction plate fits snugly against the mandibular bone, giving the implant firmness and flexibility. The cost study clearly demonstrated that, while the initial cost of the EBM system was significantly higher, the cost/part decreased significantly, making it more competitive once a sufficient quantity of reconstruction plates was manufactured.
Robocasting
Robocasting is an extrusion-based direct writing technology that uses highly solids-loaded paste-like suspensions (inks) to form three-dimensional objects through the layer-wise deposition of extruded cylinders (Lewis et al., 2006). Shao et al. (2018) used robocasting to create four types of bioceramic scaffolds (TCP, CSi, CSi-Mg10, and Bred). 3D-printed CSi-Mg10 scaffolds could be used for maxillofacial or craniofacial bone reconstruction. They have advantageous characteristics (linked three-dimensional porous structure, great mechanical strength, outstanding bioactivity, and biodegradability). Lopez et al. (2018) used a 3D direct-write micro printer gantry robot system to fabricate the b-TCP scaffolds via robocasting. In an adult rabbit model of the critical mandibular defect, the results show that 3D-printed bioactive ceramic scaffolds can heal key mandibular segmental lesions to levels comparable to natural bone.
Selective laser melting (SLM)
SLM is a method of manufacturing that involves melting and hardening layers of powder materials. These steps are repeated for each layer to create 3D objects. SLM is a powder bed fusion technique used to manufacture Ti–6AI–4V implants (Ciocca et al., 2013). This technology benefits from the ability to fabricate complex structures from powder materials, which aids in weight reduction, biological compatibility, and thermal control (Zhao et al., 2019). The primary disadvantages of this AM technology are poor surface quality, dimensional accuracy, and the requirement for specific material properties (Olakanmi et al., 2015). Farajpour et al. (2021) created a novel patient-specific prosthesis with good biomechanical properties that effectively repaired a bilateral ramus-condyle unit (RCU) defect after removing a benign mandibular tumour. The porous Ti6Al4V prosthesis was created using SLM technology. A new patient-specific prosthesis with good biomechanical features and successful repair of a bilateral RCU defect the following surgery to remove a benign mandibular tumour.
Selective laser sintering (SLS)
The SLS system consists of a laser, a powder bed, a vertically moving piston, and a roller that spreads a new powder layer. SLS refers to solid or semisolid consolidation methods at a sintering temperature below the melting point. SLS with semisolid consolidation processes is well suited for processing low melting point polymers (Chen and Gu, 2016). Researchers also used SLS to process polymers with high melting points, such as polyether ketone (PEKK). When implanted into a critical-sized bone lesion established in a rabbit model’s mandibular, Roskies et al. (Roskies et al., 2017) investigate the bone regeneration potential of customized 3D-printed PEKK scaffolds using SLS embedded with ADSCs. The results show that all scaffolds were successfully integrated into the neighbouring bone.
Finite element analysis (FEA)
FEA is a dependable, accurate, and non-invasive method for simulating complex anatomical structures and calculating internal stress and strain distributions within each tissue structure (feng Liu et al., 2017). A numerical homogenization approach based on Finite Element Analysis (FEA) was used to evaluate the effective material characteristics of scaffolds. Dutta et al. (2019) develop a numerical framework for creating a one-of-a-kind patient-specific CMC with 0°/90° circular Ti6Al4V alloy struts and variable pore architecture parameters. The findings suggest that strut diameter and inter-strut distance influence the effective homogenized orthotropic material properties of scaffolds. Lowe et al. (2021) used mandibular computed tomography scan data to run computational simulations to estimate stresses and the local scaffold adaption profile. According to the study’s results, the most significant cross-sectional stress was 2.7 MPa at the scaffold center and 4.12 MPa at the scaffold interface. The mandible’s bone bore the majority of the strain, with the scaffold initially bearing little strain but gradually increasing as more new bone was infiltrated. Prasadh et al. (2020) planned to use finite element analysis to compare two designs: one currently in use for mandibular segmental reconstruction (wing design) and one in clinical trials (endoprosthesis). Boundary conditions were established in the incisor area, and 300-N vertical stresses were applied. The wing design, when compared to an endoprosthesis, significantly reduced the stress concentration regions. Peng et al. (ming Peng et al., 2021) used three different fixation patterns to assess the stress and osteogenic properties of a multilayer porous implant in mandibular reconstruction (Model I with four screws, Model II with five screws, and Model III with six screws). Model III effectively reduced the stress shielding effect; stress within the optimized implant, defective mandible, and screws decreased by 48.18%, 44.23%, and 57.27%, respectively, compared to Model I. Following mandibular defect repair, the porous implant demonstrated significant stress transmission and maintained the same stress distribution as the intact mandible.
Using the finite element method, Gao et al. (2019) determine the optimal design of three-dimensional scaffolds for mandibular defects. The findings revealed a strong relationship between configurations and load-transmitting capacity, whereas mechanical failure depended more on strut size and architecture. This study will lay the scientific groundwork for designing and developing 3D mesh scaffolds for functional and esthetic mandibular reconstruction. A three-dimensional tetrahedral titanium scaffold for mandibular defect reconstruction is developed and optimized by Luo, Rong, and Chen (Luo et al., 2017). The findings indicate that tetrahedral structural titanium scaffolds can be used to repair mandibular defects. The proposed optimization strategy can result in improved scaffolds with high stability, porosity, and low weight.
Although our review benefits from predefined, organized search criteria and methodology, there are several limits to our scoping review, including limiting to one database and studies written in English. Some relevant articles may be overlooked if just one database and research published in English are used. This scoping review only maps the current information descriptively and exploratorily, rather than analytically and explanatorily, as a systematic review would.
Conclusion and future perspectives
Manufacturing of mandibular implants is one of the most intriguing issues for researchers to tackle in the future. Since 2016, the number of publications has steadily increased. Many countries are keen to conduct research in this area. The subject area is also not dominated by a single lot, but rather encompasses a wide range of topics. Scaffold microarchitecture is critical in the production of mandibular implants because it influences biological and mechanical properties. Microarchitecture scaffolds must have the same porosity, pore size, interconnectivity, strength, and morphology as natural bones. Design of microarchitecture scaffolds for mandibular implants in general-based cubes with uniform pore sizes that are less conducive to cell growth. In the future, a porous structure that perfectly corresponds with natural bone must be developed, namely scaffold microarchitecture with an irregular porous structure.
Additionally, polycaprolactone (PCL) was the dominant material used in the development of mandibular implants, but PCL alone was not strong enough to withstand the applied forces. Polymer materials combined with bioceramics can be used to create the ideal scaffold. They have several requirements, including high biocompatibility, precise microarchitecture, exact material composition, and optimized printing parameters. Further research into PCL Ceramic-composite is required in order to produce biomaterials with structural, mechanical, and biological properties similar to the original bone. Titanium-based bone substitutes are extremely strong and biocompatible. Still, the main issue with titanium alloy implants in clinical practice is a mismatch between the elastic modulus of titanium alloy and bone tissue, which results in stress shielding, poor load transfer, and, ultimately, a low recovery success rate.
3D printing (3DP), fused deposition modeling (FDM), selective laser melting (SLM), electron beam melting (EBM), Robocasting, and selective laser sintering (SLS) are the additive manufacturing methods commonly used in the study for reconstructing mandibular implants. The most common methods are 3D printing (3DP) and fused deposition modeling (FDM). Despite significant advances, it is difficult to identify a single method as the best option for promoting bone regeneration in various clinical situations. As a result, the field of additive manufacturing for the repair and reconstruction of mandibular defects necessitates the development of novel tools and methodologies. A customized biological scaffold can be made based on the characteristics of various mandibular defects using an appropriate 3D printing process, allowing it to be perfectly matched to the defect region and reducing stress, thus improving the scaffold’s healing function.
Author contributions
RW: collected the data; conceived and designed the analysis; wrote the paper. PA correspondent authors; performed the analysis; wrote the paper. RI: Performed the analysis. JJ: wrote the paper; conceived and designed the analysis. AB: correspondent authors; wrote the paper; funding recipient; performed the analysis.
Funding
This work was supported by the Deputy for Strengthening Research and Development, the Ministry of Research and Technology, Higher Education, the Republic of Indonesia under Ph.D. research grants of number: 345-35/UN7.6.1/PP/2022.
Conflict of interest
The authors declare that the research was conducted in the absence of any commercial or financial relationships that could be construed as a potential conflict of interest.
Publisher’s note
All claims expressed in this article are solely those of the authors and do not necessarily represent those of their affiliated organizations, or those of the publisher, the editors and the reviewers. Any product that may be evaluated in this article, or claim that may be made by its manufacturer, is not guaranteed or endorsed by the publisher.
References
Alizadeh-Osgouei, M., Li, Y., Vahid, A., Ataee, A., and Wen, C. (2021). High strength porous PLA gyroid scaffolds manufactured via fused deposition modeling for tissue-engineering applications. Smart Mater. Med. 2, 15–25. doi:10.1016/j.smaim.2020.10.003
Alvarez, K., and Nakajima, H. (2009). Metallic scaffolds for bone regeneration. Mater. (Basel) 2 (3), 790–832. doi:10.3390/ma2030790
Bae, J. C., Lee, J. J., Shim, J. H., Park, K. H., Lee, J. S., et al. (2017). Development and assessment of a 3D-printed scaffold with rhBMP-2 for an implant surgical guide stent and bone graft material: A pilot animal study. Mater. (Basel) 10, 1434–1512. doi:10.3390/ma10121434
Bouyer, M., Garot, C., Machillot, P., Vollaire, J., Fitzpatrick, V., Morand, S., et al. (2021). 3D-printed scaffold combined to 2D osteoinductive coatings to repair a critical-size mandibular bone defect. Mater. Today Bio 11, 100113. doi:10.1016/j.mtbio.2021.100113
Chang, P.-C., Luo, H., Lin, Z., Tai, W., Chang, Y., et al. (2021). Regeneration of critical-sized mandibular defect using a 3D-printed hydroxyapatite-based scaffold: An exploratory study. J. Periodontol. 92 (3), 428–435. doi:10.1002/JPER.20-0110
Chang, P. C., Luo, H. T., Lin, Z. J., Tai, W. C., Chang, Y. C., et al. (2021). Preclinical evaluation of a 3D-printed hydroxyapatite/poly(lactic-co-glycolic acid) scaffold for ridge augmentation. J. Formos. Med. Assoc. 120 (4), 1100–1107. doi:10.1016/j.jfma.2020.10.022
Chen, H., and Gu, D. (2016). Effect of metallurgical defect and phase transition on geometric accuracy and wear resistance of iron-based parts fabricated by selective laser melting. J. Mater. Res. 31 (10), 1477–1490. doi:10.1557/jmr.2016.132
Chua, C. K., Wong, C. H., and Yee Yeong, W. (2017). Standards, quality control, and measurement sciences in 3D printing and additive manufacturing.
Ciocca, L., Donati, D., Ragazzini, S., Dozza, B., Rossi, F., Fantini, M., et al. (2013). Mesenchymal stem cells and platelet gel improve bone deposition within CAD-CAM custom-made ceramic HA scaffolds for condyle substitution. Biomed. Res. Int. 2013, 1–10. doi:10.1155/2013/549762
Civantos, A., Domínguez, C., Juliana Pino, R., Setti, G., José Pavón, J., Martínez-Campos, E., et al. (2019). Designing bioactive porous titanium interfaces to balance mechanical properties and in vitro cells behavior towards increased osseointegration,” Surf. Coatings Technol. 368, 162–174. doi:10.1016/j.surfcoat.2019.03.001
Deng, N., Sun, J., Li, Y., Chen, L., Chen, C., Wu, Y., et al. (2019). Experimental study of rhBMP-2 chitosan nano-sustained release carrier-loaded PLGA/nHA scaffolds to construct mandibular tissue-engineered bone. Arch. Oral Biol. 102, 16–25. doi:10.1016/j.archoralbio.2019.03.023
Dutta, A., Mukherjee, K., Dhara, S., and Gupta, S. (2019). Design of porous titanium scaffold for complete mandibular reconstruction: The influence of pore architecture parameters, Comput. Biol. Med. 108, 31–41. doi:10.1016/j.compbiomed.2019.03.004
Eppley, B. L., Pietrzak, W. S., and Blanton, M. W. (2005). Allograft and alloplastic bone substitutes: A review of science and technology for the craniomaxillofacial surgeon. J. Craniofac. Surg. 16 (6), 981–989. doi:10.1097/01.scs.0000179662.38172.dd
Farajpour, H., Bastami, F., Bohlouli, M., and Khojasteh, A. (2021). Reconstruction of bilateral ramus-condyle unit defect using custom titanium prosthesis with preservation of both condyles. J. Mech. Behav. Biomed. Mater. 124 (), 104765. doi:10.1016/j.jmbbm.2021.104765
feng Liu, Y., ying Fan, Y., feng Jiang, X., and Baur, D. A. (2017). A customized fixation plate with novel structure designed by topological optimization for mandibular angle fracture based on finite element analysis. Biomed. Eng. Online 16 (1), 131–217. doi:10.1186/s12938-017-0422-z
Fucile, P., Papallo, I, Improta, G, De Santis, R, Gloria, A, Onofrio, I, et al. (2019). “Reverse Engineering and Additive Manufacturing towards the design of 3D advanced scaffolds for hard tissue regeneration,” in 2nd IEEE International Workshop on Metrology for Industry 4.0 and IoT, MetroInd 4.0 and IoT 2019, 33–37. doi:10.1109/METROI4.2019.8792891
Fucile, P., Onofrio, I., Papallo, I., Gallicchio, V., Rega, A., D'Anto, V., et al. (2020). Strategies for the design of additively manufactured nanocomposite scaffolds for hard tissue regeneration. Acta IMEKO 9 (4), 53–59. doi:10.21014/acta_imeko.v9i4.739
Gao, H., Li, X., Wang, C., Ji, P., and Wang, C. (2019). Mechanobiologically optimization of a 3D titanium-mesh implant for mandibular large defect: A simulated study, Mater. Sci. Eng. C 104, 109934. doi:10.1016/j.msec.2019.109934
Gentile, P., Chiono, V., Carmagnola, I., and Hatton, P. V. (2014). An overview of poly(lactic-co-glycolic) Acid (PLGA)-based biomaterials for bone tissue engineering. Int. J. Mol. Sci. 15 (3), 3640–3659. doi:10.3390/ijms15033640
He, J., Xu, F., Dong, R., Guo, B., and Li, D. (2017). Electrohydrodynamic 3D printing of microscale poly (ϵ-caprolactone) scaffolds with multi-walled carbon nanotubes. Biofabrication 9, 015007–15011. doi:10.1088/1758-5090/aa53bc
Hikmawati, D., Sundari, T. D., Aminatun, , , and Wardhani, I. F. (2019). “The design of pores in PCL/HA-associated PLA scaffold with 3D printing method,” in 2nd International Conference on Physical Instrumentation and Advanced Materials, ICPIAM, 2314. doi:10.1063/5.0034912
Houben, A., Van Hoorick, J., Van Erps, J., Thienpont, H., Van Vlierberghe, S., and Dubruel, P. (2017). Indirect rapid prototyping: Opening up unprecedented opportunities in scaffold design and applications. Ann. Biomed. Eng. 45 (1), 58–83. doi:10.1007/s10439-016-1610-x
Jariwala, S. H., Lewis, G. S., Bushman, Z. J., Adair, J. H., and Donahue, H. J. (2015). 3D printing of personalized artificial bone scaffolds. 3D Print. Addit. Manuf. 2 (2), 56–64. doi:10.1089/3dp.2015.0001
jie Cheng, K., Liu, Y. f., Wang, R., Zhang, J. x., Jiang, X. f., Dong, X. t., et al. (2020). Topological optimization of 3D printed bone analog with PEKK for surgical mandibular reconstruction. J. Mech. Behav. Biomed. Mater. 107, 103758. doi:10.1016/j.jmbbm.2020.103758
Kanwar, S., and Vijayavenkataraman, S. (2021). Design of 3D printed scaffolds for bone tissue engineering: A review, Bioprinting 24, e00167. doi:10.1016/j.bprint.2021.e00167
Kim, J. W., Yang, B. E., Hong, S. J., Choi, H. G., Byeon, S. J., Lim, H. K., et al. (2020). Bone regeneration capability of 3D printed ceramic scaffolds. Int. J. Mol. Sci. 21 (14), 4837–4913. doi:10.3390/ijms21144837
Kumar, B. P., Venkatesh, V., Kumar, K. A. J., Yadav, B. Y., and Mohan, S. R. (2016). Mandibular reconstruction: Overview. J. Maxillofac. Oral Surg. 15 (4), 425–441. doi:10.1007/s12663-015-0766-5
Lee, J. S., Park, T. H., Ryu, J. Y., Kim, D. K., Oh, E. J., Kim, H. M., et al. (2021). Osteogenesis of 3d-printed pcl/tcp/bdecm scaffold using adipose-derived stem cells aggregates; an experimental study in the canine mandible. Int. J. Mol. Sci. 22, 5409–5411. doi:10.3390/ijms22115409
Lee, S., Choi, D., Shim, J. H., and Nam, W. (2020). Efficacy of three-dimensionally printed polycaprolactone/beta tricalcium phosphate scaffold on mandibular reconstruction. Sci. Rep. 10 (1), 4979–9. doi:10.1038/s41598-020-61944-w
Lewis, J. A., Smay, J. E., Stuecker, J., and Cesarano, J. (2006). Direct ink writing of three-dimensional ceramic structures. J. Am. Ceram. Soc. 89 (12), 3599–3609. doi:10.1111/j.1551-2916.2006.01382.x
Lopez, C. D., Diaz-Siso, J. R., Witek, L., Bekisz, J. M., Cronstein, B. N., Torroni, A., et al. (2018). Three dimensionally printed bioactive ceramic scaffold osseoconduction across critical-sized mandibular defects. J. Surg. Res. 223, 115–122. doi:10.1016/j.jss.2017.10.027
Lowe, B., Huotilainen, E., Laitinen, M., Henell, A. M., Ye, Q., Troulis, M. J., et al. (2021). FEA evaluation of material stiffness changes for a polymer assisted 3D polycaprolactone/β-tricalcium phosphate scaffold in a mandibular defect reconstruction model. Ceram. Int. 47 (6), 8075–8081. doi:10.1016/j.ceramint.2020.11.162
Luo, D., Rong, Q., and Chen, Q. (2017). Finite-element design and optimization of a three-dimensional tetrahedral porous titanium scaffold for the reconstruction of mandibular defects. Med. Eng. Phys. 47, 176–183. doi:10.1016/j.medengphy.2017.06.015
Malá, E., Vejrazkova, E., Bielmeierova, J., Jindra, M., Vosmik, M., Novosad, J., et al. (2015). Long term monitoring of nutritional, clinical status and quality of life in head and neck cancer patients. Klin. Onkol. 28 (3), 200–214. doi:10.14735/amko2015200
Marongiu, G., Verona, M., Cardoni, G., and Capone, A. (2020). Synthetic bone substitutes and mechanical devices for the augmentation of osteoporotic proximal humeral fractures: A systematic review of clinical studies. J. Funct. Biomater. 11 (2), 29. doi:10.3390/jfb11020029
Miljanovic, D., Seyedmahmoudian, M., Stojcevski, A., and Horan, B. (2020). Design and fabrication of implants for mandibular and craniofacial defects using different medical-additive manufacturing technologies: A review. Ann. Biomed. Eng. 48 (9), 2285–2300. doi:10.1007/s10439-020-02567-0
ming Peng, W., Cheng, K. j., Liu, Y. f., Nizza, M., Baur, D. A., Jiang, X. f., et al. (2021). Biomechanical and Mechanostat analysis of a titanium layered porous implant for mandibular reconstruction: The effect of the topology optimization design,” Mater. Sci. Eng. C 124, 112056, 2021. doi:10.1016/j.msec.2021.112056
Misch, C. M. (2010). Autogenous bone: Is it still the gold standard? Implant Dent. 19 (5), 361. doi:10.1097/ID.0b013e3181f8115b
Moiduddin, K., Mian, S. H., Ahmed, N., Ameen, W., Al-Khalefah, H., Mohammed, M. k., et al. (2020). Integrative and multi-disciplinary framework for the 3D rehabilitation of large mandibular defects. Int. J. Adv. Manuf. Technol. 106 (9–10), 3831–3847. doi:10.1007/s00170-019-04762-3
Moiduddin, K., Mian, S. H., Alkhalefah, H., and Umer, U. (2019). Digital design, analysis and 3D printing of prosthesis scaffolds for mandibular reconstruction. Met. (Basel). 9, 569–575. doi:10.3390/met9050569
Moreno Madrid, A. P., Vrech, S. M., Sanchez, M. A., and Rodriguez, A. P. (2019), Advances in additive manufacturing for bone tissue engineering scaffolds, Mater. Sci. Eng. C 100, 631–644. doi:10.1016/j.msec.2019.03.037
Muallah, D., Sembdner, P., Holtzhausen, S., Meissner, H., Hutsky, A., Ellmann, D., et al. (2021). Adapting the pore size of individual, 3d-printed cpc scaffolds in maxillofacial surgery. J. Clin. Med. 10 (12), 2654–2715. doi:10.3390/jcm10122654
Olakanmi, E. O., Cochrane, R. F., and Dalgarno, K. W. (2015). A review on selective laser sintering/melting (SLS/SLM) of aluminium alloy powders: Processing, microstructure, and properties. Prog. Mater. Sci. 74, 401–477. doi:10.1016/j.pmatsci.2015.03.002
Park, J. H., Jung, S. Y., Lee, C., Ban, M. J., Lee, S. J., Kim, H. Y., et al. (2020). A 3D-printed polycaprolactone/β-tricalcium phosphate mandibular prosthesis: A pilot animal study. Laryngoscope 130 (2), 358–366. doi:10.1002/lary.27908
Park, S. A., Lee, H.-J., Kim, S.-Y., Kim, K.-S., Jo, D.-W., and Park, S.-Y. (2021). Three-dimensionally printed polycaprolactone/beta-tricalcium phosphate scaffold was more effective as an rhBMP-2 carrier for new bone formation than polycaprolactone alone. J. Biomed. Mater. Res. - Part A 109 (6), 840–848. doi:10.1002/jbm.a.37075
Park, S. A., Lee, H. J., Kim, K. S., Lee, S., Lee, J. T., Kim, S. Y., et al. (2018). In vivo evaluation of 3D-printed polycaprolactone scaffold implantation combined with β-TCP powder for alveolar bone augmentation in a beagle defect model. Materials 11, 238. doi:10.3390/ma11020238
Pellizzari, M., Jam, A., Tschon, M., Fini, M., Lora, C., and Benedetti, M. (2020). A 3D-printed ultra-low young’s modulus β-Ti alloy for biomedical applications. Materials 13 (12), 2792–2816. doi:10.3390/ma13122792
Pielichowska, K., and Blazewicz, S. (2010). Bioactive polymer/hydroxyapatite (nano) composites for bone tissue regeneration. Biopolymers,Advances Polym. Sci., 30–59. doi:10.1007/12_2010_50
Prasadh, S., Suresh, S., Hong, K. L., Bhargav, A., Rosa, V., and Wong, R. C. W. (2020). Biomechanics of alloplastic mandible reconstruction using biomaterials: The effect of implant design on stress concentration influences choice of material. J. Mech. Behav. Biomed. Mater. 103, 103548. doi:10.1016/j.jmbbm.2019.103548
Rengier, F., Mehndiratta, A., von Tengg-Kobligk, H., Zechmann, C. M., Unterhinninghofen, R., Kauczor, H. U., et al. (2010). 3D printing based on imaging data: Review of medical applications. Int. J. Comput. Assist. Radiol. Surg. 5 (4), 335–341. doi:10.1007/s11548-010-0476-x
Roskies, M. G., Fang, D., Abdallah, M. N., Charbonneau, A. M., Cohen, N., Jordan, J. O., et al. (2017). Three-dimensionally printed polyetherketoneketone scaffolds with mesenchymal stem cells for the reconstruction of critical-sized mandibular defects. Laryngoscope 127 (11), E392–E398. doi:10.1002/lary.26781
Shao, H., Sun, M., Zhang, F., Liu, A., He, Y., Fu, J., et al. (2018). Custom repair of mandibular bone defects with 3D printed bioceramic scaffolds. J. Dent. Res. 97 (1), 68–76. doi:10.1177/0022034517734846
Sheikh, Z., Hamdan, N., Ikeda, Y., Grynpas, M., Ganss, B., and Glogauer, M. (2017). Natural graft tissues and synthetic biomaterials for periodontal and alveolar bone reconstructive applications: A review. Biomater. Res. 21 (1), 9–20. doi:10.1186/s40824-017-0095-5
Shibuya, N., and Jupiter, D. C. (2015). Bone graft substitute: Allograft and xenograft. Clin. Podiatr. Med. Surg. 32 (1), 21–34. doi:10.1016/j.cpm.2014.09.011
Sun, Z. P., Guo, Y. B., and Shim, V. P. W. (2020). Characterisation and modeling of additively-manufactured polymeric hybrid lattice structures for energy absorption. Int. J. Mech. Sci. 191, 106101. doi:10.1016/j.ijmecsci.2020.106101
Szpalski, C., Wetterau, M., Barr, J., and Warren, S. M. (2012). Bone tissue engineering: Current strategies and techniques-Part I: Scaffolds. Tissue Eng. - Part B Rev. 18 (4), 246–257. doi:10.1089/ten.teb.2011.0427
Thomas, D., and Singh, D. (2021). 3D printing cross-linkable calcium phosphate biocomposites for biocompatible surgical implantation, Bioprinting, 22, e00141. doi:10.1016/j.bprint.2021.e00141
Tricco, A. C., Lillie, E., Zarin, W., O'Brien, K. K., Colquhoun, H., Levac, D., et al. (2018). PRISMA extension for scoping reviews (PRISMA-ScR): Checklist and explanation. Ann. Intern. Med. 169 (7), 467–473. doi:10.7326/M18-0850
Vaezi, M., and Yang, S. (2015). Extrusion-based additive manufacturing of PEEK for biomedical applications. Virtual Phys. Prototyp. 10 (3), 123–135. doi:10.1080/17452759.2015.1097053
Winarso, R., Anggoro, P. W., Ismail, R., Jamari, J., and Bayuseno, A. P. (2022). Application of fused deposition modeling (FDM) on bone scaffold manufacturing process: A review. SSRN Electron. J. 8, e11701. doi:10.2139/ssrn.4103975
Wulandari, A. F., Zulaihah, S., Rachmadanti, I., and Aminatun, (2019). “In-vitro characterization of 3D printed PLA scaffold with hydroxyapatite-chitosan coating for mandibula reconstruction,” in 2nd International Conference on Physical Instrumentation and Advanced Materials, ICPIAM, 20202314. doi:10.1063/5.0034693
Xu, H. H. K., Wang, P., Wang, L., Bao, C., Chen, Q., Weir, M. D., et al. (2017). Calcium phosphate cements for bone engineering and their biological properties. Bone Res. 5, 1–19. doi:10.1038/boneres.2017.56
Yan, R., Luo, D., Huang, H., Li, R., Yu, N., Liu, C., et al. (2018). Electron beam melting in the fabrication of three-dimensional mesh titanium mandibular prosthesis scaffold. Sci. Rep. 8 (1), 750–810. doi:10.1038/s41598-017-15564-6
Yang, Y., Wang, G., Liang, H., Gao, C., Peng, S., Shen, L., et al. (2019). Additive manufacturing of bone scaffolds. Int. J. Bioprinting 5 (1), 1–25. doi:10.18063/IJB.v5i1.148
Zamani, Y., Amoabediny, G., Mohammadi, J., Seddiqi, H., Helder, N. C., Zandieh-Doulabi, B., et al. (2020). 3D-printed poly(Ɛ-caprolactone) scaffold with gradient mechanical properties according to force distribution in the mandible for mandibular bone tissue engineering. J. Mech. Behav. Biomed. Mater 104, 103638. doi:10.1016/j.jmbbm.2020.103638
Zhang, P., Yang, K., Zhou, Z., Zhu, X., Li, W., Cao, C., et al. (2020). Customized borosilicate bioglass scaffolds with excellent biodegradation and osteogenesis for mandible reconstruction. Front. Bioeng. Biotechnol. 8, 610284. doi:10.3389/fbioe.2020.610284
Zhang, Q., Wu, W., Qian, C., Xiao, W., Zhu, H., Guo, J., et al. (2019). Advanced biomaterials for repairing and reconstruction of mandibular defects. Mater. Sci. Eng. C 103, 109858. doi:10.1016/j.msec.2019.109858
Keywords: 3D printing, mandibular implants, manufacturing process, bone tissue engineering, 3D print
Citation: Winarso R, Ismail R, Anggoro PW, Jamari J and Bayuseno AP (2023) A scoping review of the additive manufacturing of mandibular implants. Front. Mech. Eng 9:1079887. doi: 10.3389/fmech.2023.1079887
Received: 25 October 2022; Accepted: 16 January 2023;
Published: 25 January 2023.
Edited by:
Manuel Doblare, University of Zaragoza, SpainReviewed by:
José Sanz-Herrera, Universidad de Sevilla, SpainAndres Diaz Lantada, Universidad Politécnica de Madrid, Spain
Copyright © 2023 Winarso, Ismail, Anggoro, Jamari and Bayuseno. This is an open-access article distributed under the terms of the Creative Commons Attribution License (CC BY). The use, distribution or reproduction in other forums is permitted, provided the original author(s) and the copyright owner(s) are credited and that the original publication in this journal is cited, in accordance with accepted academic practice. No use, distribution or reproduction is permitted which does not comply with these terms.
*Correspondence: Paulus Wisnu Anggoro, cGF1bHVzd2lzbnVhbmdnb3JvQHltYWlsLmNvbQ==; Athanasius Priharyoto Bayuseno, YXBiYXl1c2Vub0BnbWFpbC5jb20=