- Department of Naval Architecture, Ocean, and Marine Engineering, Maritime Safety Research Centre, University of Strathclyde, Glasgow, United Kingdom
Pursuing net-zero emission operations in the shipping industry are quintessential for this sector to mitigate the environmental impact caused by hydrocarbon fuel combustion. Significant contributions to this are expected from the substitution of conventional marine fuels by alternative, emission-free fuels with lower emission footprints. This study aims to conduct a comprehensive literature review for delineating the main characteristics of the considered alternative fuels, specifically focusing on hydrogen, methanol, and ammonia, which have recently attracted attention from both industry and academia. This study comparatively assesses the potential of using these fuels in marine engines, and their subsequent performance characteristics as well as the associated environmental benefits. In addition, the required storage conditions, space, as well as the associated costs, are reviewed. Special attention is given to the safety characteristics and requirements for each alternative fuel. The results of this study demonstrate that the environmental benefits gained from alternative fuel use are pronounced only when renewable energy is considerably exploited for their production, whereas the feasibility of each fuel depends on the vessel type used and pertinent storage constraints. Hydrogen, ammonia, and methanol are considered best-fit solutions for small scale shipping, requiring minimal on-board storage. In addition, the need for comparative assessments between diesel and alternative fuels is highlighted and sheds light on marine engines’ operational characteristics. Moreover, using combinations of alternative and diesel fuels is identified as a direction towards decarbonisation of the maritime sector; intensifying the need for optimisation studies on marine engine design and operation. This study concludes with recommendations for future research directions, thus contributing to fuel research concepts that can facilitate the shipboard use of alternative fuels.
1 Introduction
With 80% of international trade volume being transported by ships (UNCTAD, 2020), the maritime industry is highly acknowledged for its importance to global trade and therefore economy. Shipping sustainability has been proven crucial to ensure economic growth and has attracted considerable interest in recent years. Supply chain resilience is vital for ensuring economic development and financial impact worldwide and is also associated with cargo delivery costs and supply chain optimisation (Gürel & Shadmand, 2019; Abioye, et al., 2021).
Economic growth is unavoidably associated with a significant contribution to greenhouse gas emissions, which are mainly caused by the exhaust gases of marine engines. According to (Buhaug, et al., 2009) and (Anderson & Bows, 2012), ship greenhouse gas emissions are set to increase by 250% in 2050. By 2020, shipping emissions accounted for 2.76% of the total, according to (Faber, et al., 2020) and a projected increase to 17% is expected by 2050 (Cames, et al., 2015). According to (Wan, et al., 2016), the shipping industry’s contribution to global nitrogen oxides (NOx) and sulphur oxides (SOx) levels in 2012 accounted for 15% and 13%, respectively, of the total. Both pollutants have a tremendous impact on the shipping environmental footprint. To mitigate these pollutants, as well as their impact, the International Maritime Organisation (IMO) has developed a number of restrictions (limits) within the scope of MARPOL Annex VI and has introduced Emission Control Areas (ECAs) (IMO, 2021). These measures are specifically designed to avoid high concentrations of emissions close to cities, as 70% of emissions are released in zones that are 400 km away from the shore (EyringaIvar, et al., 2010). To mitigate carbon dioxide (CO2) emissions, the IMO introduced regulations including the Ship Energy Efficiency Management Plant (SEEMP), the Energy Efficiency Design Index (EEDI), and the Energy Efficiency Operating Indicator (EEOI) for newbuilt ships (Johnson, et al., 2013), as well as the Energy Efficiency Index (EEXI) and the Carbon Intensity Index (CII) for existing vessels (IMO, 2020).
Maritime sector sustainability is a relatively new area of focus. It combines economic, environmental, and social criteria, embedding energy efficiency and emission mitigation methods. From a technical perspective, the adoption of indexes such as the Energy Efficiency Index (EEXI) and the Carbon Intensity Index (CII) (IMO, 2020) applies to most existing vessels. These indexes match the existing Ship Energy Efficiency Management Plant (SEEMP) and Energy Efficiency Design Index (EEDI), which promote and set minimum levels for energy efficiency in each vessel type (Johnson, et al., 2013). Romano and Yang (2021) described the strengths and weaknesses of several shipping decarbonisation technologies aimed at enhancing the sectors’ sustainability, highlighting the use of ammonia and hydrogen as well as LNG fuel, which can be potentially useful for different ship types. Furthermore, the use of decarbonisation policies is highlighted, including those for carbon taxation (Ding, et al., 2020) and national laws (Chen, et al., 2020).
Marine engines are critical systems that provide propulsion and auxiliary power to ships, and are responsible for releasing greenhouse gas (GHG) and non-GHG emissions to the atmosphere (Miola & Ciuffo, 2011). Pertinent research, both experimental or numerical, has focused on methodologies to determine ship exhaust emissions (Kumar, et al., 2013), (Petzold, et al., 2008), (Li, et al., 2015), as well as engine performance parameters such as the brake specific fuel consumption (bsfc) and the engine efficiency (brake, indicated, combustion). Regardless of the approach considered, the determination of emission factors (fuel-based and energy-based) are useful for the investigation of alternative fuels in marine engines and their environmental footprint.
Conventional fuels used in the maritime sector include marine gas oil, marine diesel oil, and heavy fuel oil, the combustion of which releases a significant amount of emissions (Streibel, et al., 2017), (Corbett & Winebrake, 2008), (Nabi & Hustad, 2010). According to (Karvounis & Blunt, 2021), the unconstrained production and utilisation of conventional fossil fuels may result in reaching the available carbon budget much earlier than expected, intensifying climate change implications. Thus, the development and adoption of emission-free fuels are essential. Currently, a wide range of alternative fuels are proposed for use in marine engines including gas fuels such as methane (CH4), ethane (C2H6), propane (C3H8), and dimethyl ether (DME); these offer considerable emission reductions at the expense of increased storage space requirements (Kanna, et al., 2019), (Deng, et al., 2021). Other fuels like hydrogen (H2), ammonia (NH3), and biodiesel are considered carbon-free and have attracted the interest of both industry and academia, as established production methods already exist. Methanol (CH3OH) is a liquid fuel with a similar density to diesel, whilst its technical maturity is at medium levels (Achuthanandam, 2021). Therefore, methanol can be used as an alternative to marine diesel oil in the short-to medium-term timeline (Cheng, et al., 2008).
Focus on the topic of alternative fuels is growing worldwide, as demonstrated in the plot shown in Figure 1. Due to the recent upsurge in publications and developments, review studies on the various aspects of alternative fuels, as well as on their sustainable and safe use for shipping, are required to identify research gaps and to point out research pathways. Hence, this study aims to conduct a comprehensive literature review pertinent to the performance and emission characteristics of marine engines that use hydrogen, ammonia, and methanol as fuels. The main characteristics of the considered alternative fuels, as well as their environmental footprint, usability, storage/space requirements, and cost factors are comparatively assessed. Moreover, their safety characteristics are reviewed and safety measures are recommended based on current technological advancements. This study addresses the literature gap for comprehensive reviews on hydrogen, ammonia, and methanol use in marine engines, and discusses the specific barriers and challenges for future research to decarbonise the shipping sector, promoting emission-free, sustainable, and safe operations.
The remainder of this study is structured as follows: Section 2 describes the research approach implemented. Section 3 elaborates on recent developments in alternative fuel use. Section 4 discusses the safety, emissions, cost, and storage requirements of the considered alternative fuels. Section 5 provides recommendations for future research initiatives. Lastly, Section 6 concludes this study.
2 Research approach
The employed methodology consists of six steps, as shown in the flowchart in Figure 2. The steps are described as follows:
Step 1:. The scope and boundaries of the study are identified, along with the key data sources.The purpose of this study is to understand the combustion characteristics of the designated alternative fuels and to draw important conclusions regarding engine performance and emissions. It will also address the issues of safety, storage, and cost of changes essential to facilitate shipboard use of the considered alternative fuels. Several publications are identified in scientific journals, conference papers, and industrial reports, which are subsequently filtered, categorised, and analysed. Most of the identified publications address the topics of hydrogen, ammonia, and methanol combustion in internal combustion engines, either as pure fuels or as mixtures with diesel in order to identify their benefits and drawbacks.
Step 2:. The key performance indicators examined herein include the engine performance characteristics (efficiency and brake specific fuel consumption), the safety and regulations, the emissions factors related to production and operating phases, as well as the technical maturity, cost, and storage space requirements.
Step 3:. Developments and trends for the considered fuels and fuel combinations are identified.
Step 4:. A comparative assessment, based on the set of key performance indicators, is conducted for all the considered fuels. This allows for the provision of information on potential short- or long-term fuel solutions to reduce the environmental footprint of shipping operations.
Step 5:. Lastly, the key research gaps are identified, and recommendations for future research directions are provided.By following the preceding steps, the most important aspects of the shipboard use of alternative fuels, namely, methanol, hydrogen, and ammonia, are examined, providing valuable insights and recommendations for further research studies. The challenges of this study include the lack of specific information on the performance/emissions parameters for marine engines that use hydrogen, methanol, or ammonia as fuels, as well as the lack of information pertinent to the safety aspects of the shipboard use of these fuels.Table 1 lists the key performance indicators (KPIs) discussed in the next sections of this study. These are grouped into the following categories: 1) engine performance; 2) emissions; 3) safety; 4) cost; and 5) fuel storage.
3 Developments in alternative fuels use
This section considers recent developments in alternative fuels used in compression-ignition internal combustion engines, which constitute the engine type mostly employed in the marine sector. The following subsections address the fuels considered in the study, namely, hydrogen, ammonia, and methanol. A table summarising the main characteristics of each identified study and the key findings for the considered alternative fuels is provided in the Appendix.
3.1 Hydrogen
The pertinent literature examines the behaviour of hydrogen in both compression-ignition and dual-fuel engines. Due to its high autoignition temperature (800 K), hydrogen is mostly used along with diesel in dual-fuel combustion (Naber & Siebers, 1998). Ikegami (1982) studied a four-stroke, compression-ignition engine operating on pure hydrogen and reported extended limitations of its operating envelope. Several studies on dual-fuel CI engines operating with diesel as an ignition fuel injected close to the top dead centre (TDC) are present in the pertinent literature. Nagarajan (2009) experimentally studied the injection timing, injection duration, and hydrogen flow rate in a H2-Diesel dual-fuel CI engine, reporting optimal port injection at 5oCA before TDC (open cycle) and flow rates of 9.5 lpm required to minimise the knock effect and to achieve optimal emissions reduction. Kavtaradze et al. (2019) numerically investigated, using computational fluid dynamics (CFD), the performance parameters of a heavy duty, hydrogen-diesel dual-fuel CI engine, reporting an indicated engine efficiency of approximately 50% and a NOx concentration of 920 ppm (at full load).
Babayev et al. (2022) compared the operation of a direct-injection CI engine using either hydrogen or diesel. To achieve CO2-free output, instead of using diesel as an ignition fuel, the authors introduced a two-step ignition of hydrogen using a small H2 pilot injection and a subsequent main H2 direct injection at 10oCA before TDC; a method that was also validated by McTaggart-Cowan (2015). The results of these studies demonstrated that the high temperatures in the combustion chamber resulted in high thermal fluxes and heat losses, and therefore efficiency reduction, which, for hydrogen operation, was 4% lower compared with diesel operation. Dimitriou et al. (2018) experimentally studied the operation of a four-stroke dual-fuel CI engine operating with different combinations of diesel-hydrogen fuels. At low load conditions, smooth operation was achieved with no pre-ignition, for H2 ratios of up to 98%. NOx and soot emission reductions of 90% and 85%, respectively, were reported for 98% H2, whilst for lower ratios, NOx emissions increased compared with conventional engines. The hydrogen-diesel fuel combination was also investigated by Saravanan and Nagarajan (2010) to assess engine performance parameters and emissions. At low loads, both smoke and NOx emissions were reduced due to lean mixture operation. At full load conditions, hydrogen share resulted in extensive knocking, which restricted overall engine performance improvements, an argument also supported by Abdelaal et al. (2013) and Torregrosa (2011). Chintala and Subramanian (2014) conducted an exergy analysis on the utilisation of useful work in a compression-ignition engine that employed directly injected hydrogen (18%) and diesel fuels. The authors concluded that the energy efficiency of the engine increased by 10%, whilst CO2 emissions reduced by 36% at low loads. Miyamoto et al. (2011) experimentally evaluated the use of directly injected hydrogen (up to 10%) and diesel fuels, concluding that significant reductions in CO and CO2 emissions were obtained at high loads, associated with an increase in NOx emissions and a constant thermal efficiency.
Spark-ignited engines may offer an easier technology for the use of hydrogen; however, several challenges may arise, including hydrogen ignition during the injection process. Dhyani and Subramanian (2021) reported that this could lead to damage of the engine intake and fuel supply systems, as well as combustion instabilities. Spark-ignition engines are not preferred where high torque levels are required at low engine speeds; the case for marine engines (Karim, 2003).
3.2 Ammonia
A main challenge pertinent to ammonia combustion is the slow speed of the flame front. Li et al. (2021) and Law (2006) investigated the burning velocity of an ammonia-air mixture, and was found to be relatively low (6–8 cm/s) when compared with a methane-air mixture (35 cm/s flame velocity). A change in the intake pressure modestly affected the flame velocity according to Ronney (1988), whilst temperature variation diversely affected the velocity for different equivalence ratio ranges. Li et al. (2015) suggested oxygen enrichment as a promising technology to increase the burning velocity. Moreover, the use of ammonia in a compression-ignition engine faces similar challenges to hydrogen, due to its extremely high autoignition temperature. Blarigan (2000) reported that the optimal compression ratios for ammonia fuel combustion are between 35:1 and 100:1, significantly higher than that of marine engine operation: close to 25:1. As a result, research on ammonia-only operation is scarce. However, for dual-fuel operation, its carbonless chemical formula presents significant environmental benefits. Gray et al. (1966) recommended that the compression ratio for engines operating with diesel-ammonia fuel combinations can be reduced to 15:1, whilst complete combustion was achieved when ammonia was injected no later than 40 CA before the end of diesel injection. Reiter and Kong (2008) tested the use of different ratios of ammonia-diesel in a dual-fuel engine, concluding that the optimal range of ammonia (compromising between combustion efficiency and emissions) is in the range 40%–80%, whilst NOx formation was reduced for ammonia rates up to 60%. Moreover, the engine brake specific fuel consumption (BSFC) increased significantly upon changing from medium to high loads. However, Kong (2007) found that, due to low combustion temperatures achieved for high ammonia ratios, the unburned ammonia concentration at the engine exhaust was close to 1,000 ppm. Niki et al. (2016) experimentally examined different flowrates of ammonia injection in a diesel engine and reported a CO2 reduction of approximately 10%, with other emissions remaining unchanged. A 10% BSFC reduction was also observed. The authors proposed multi-injection strategies to reduce the unburnt ammonia concentrations to as low as 10 ppm (Rujub, 2020). numerically investigated an ammonia-MDO fuel combination in a 6-cylinder marine engine, demonstrating that significant NOx emission reductions can be achieved by retarding the ammonia fuel injection timing up to 58oCA after TDC. A NOx emissions reduction of close to 60%, accompanied by 30 ppm ammonia slip, was reported by Lamas and Rodriguez (2019) who employed a tri-fuel operation using diesel-hydrogen-ammonia, with the ammonia fuel injection timing set at 43.2oCA after TDC. Galdo et al. (2020) examined the emissions of a marine DI engine operating at 8% ammonia substitution, and concluded that significant NOx emissions reduction (70%) is possible at full load, when ammonia injection occurred at 58.4°CA after TDC. Pochet et al. (2020) examined a 22:1 compression ratio homogenous charge compression-ignition engine operating with hydrogen-ammonia fuel combinations. Different ranges of fuel mixtures from 0% to 94% ammonia, an equivalence ratio from 0.1 to 0.6, and intake temperatures from 50°C to 240°C were investigated. For ammonia-rich mixtures, NOx emissions increased, whilst for combustion temperatures below 1400 K, unwanted production of N2O was observed. The combustion efficiency remained almost constant, whilst the indicated mean effective pressure (IMEP) increased by approximately 50% at a maximum ammonia mixture.
3.3 Methanol
The use of methanol as a single fuel in compression-ignition engines is challenging due to its low reactivity. Regarding its combustion characteristics, Sayin et al. (2010) studied a four-stroke direct-injection diesel engine with methanol blends reaching 15% and observed an increase in NOx emissions at high loads (approximately 60%) and a reduction in smoke and CO2 emissions due to the low carbon content in methanol, whilst the brake specific fuel consumption increased as well. Canakci et al. (2009) studied a single cylinder naturally-aspirated diesel engine with methanol blends up to 15%, showcasing that an increased combustion efficiency was associated with increased NOx and CO2 emissions, whilst a reduction in NOx emissions was achieved by lowering the injection pressure. Datta and Mandal (2016) numerically investigated a four-stroke direct-injection variable compression ratio (CR) diesel engine with methanol blends up to 15%, concluding that NOx and CO2 emission reductions were achieved (more significant variations were achieved at high loads), whilst the combustion efficiency was reduced and the exhaust gas temperature increased. Saxena et al. (2021) concluded that methanol fuel is potent enough to partially replace fossil fuels, either as a mixture with diesel fuels or using dual-fuel combustion. A decreased brake efficiency at high methanol fractions (using methanol-diesel blends) was reported. In dual-fuel engines utilising directly-injected methanol and diesel fuels, the opposite trend was observed. Dual-fuel engines also exhibited lower NOx, CO, and particulate emissions compared with diesel engines. Higher methanol fractions resulted in increased NOx emissions. Bayraktar (2008) experimentally studied a single-cylinder four-stroke CI engine using methanol-diesel blends with a methanol fraction in the range of 2.5%–15%. The results showed that the fuel-air equivalence ratio of the diesel-methanol blend decreased as the methanol ratio increased. The methanol fraction increase also resulted in an increase in the brake efficiency by approximately 2%, whilst the brake specific fuel consumption increased by 10% at high loads. Ten percent of methanol in the methanol-diesel blend was proposed as optimum, whereas further performance improvements could only be achieved by increasing the fuel cetane number. Guo et al. (2011) studied higher methanol-diesel blends reaching a methanol fraction of 30% in a single cylinder four-stroke direct-injection engine, demonstrating an increased fuel consumption of approximately 8% and increased NOx emissions by 10% at low loads, whereas a reduction of approximately 10% was observed at higher loads (Liu, et al., 2022). Numerically examined the contribution of 50% methanol in a dual-fuel engine and reported significant reductions in NOx and soot emissions by 12% and 26%, respectively (Wei, et al., 2016). Examined the effect of diesel injection timings on the emissions and performance of a six-cylinder direct-injection engine with high methanol ratios (up to 90%). For all injection timings, high methanol substitution resulted in deterioration of the engine’s brake efficiency by approximately 3%, whereas delayed diesel injection positively affected NOx and soot emissions, reducing them by 20% and 50%, respectively.
The above analysis intended to highlight some of the key findings on compression-ignition engines that utilise the designated alternative fuels, or their combinations, usually substituting diesel. With regards to hydrogen and ammonia combustion in compression-ignition engines, the main challenges are the high auto-ignition temperature and the slow speed of flame, causing efficiency deterioration. These issues can be addressed by optimising the injection parameters and/or by employing multi-injection. To increase the low laminar burning velocity of ammonia, oxygen enrichment was proven to have significant benefits, minimising deterioration effects during fuel combustion. For methanol, two contradictory effects were noted in many cases; NOx emissions were increased due to higher in-cylinder temperatures as a consequence of oxygen in the fuel molecules, and in some cases NOx emissions were inhibited due to the increased amount of fuel injected in-cylinder, quenching the mixture and therefore reducing the temperature. These issues were addressed by most studies, along with fuel consumption increases. Possible strategies suggested a reduction in the injection pressure to reduce NOx emissions and to enhance the thermal efficiency; however, CO2 and CO emissions tended to increase. Despite this, delayed diesel injection in dual-fuel engines was found to be very effective in reducing overall emissions.
4 Fuel Use Assessment
This section discusses several aspects of the use of the considered fuels (hydrogen, ammonia, and methanol), focusing on the areas of safety, emissions, fuels energy density, and storage as well as cost.
4.1 Safety
A critical aspect of the use of alternative fuels in ship power plants and marine engines concerns safety. Safety involves increasing the reliability of the simple system under investigation while minimising the effects of potential consequences, ensuring that the system maintains an acceptable level of safety (Verma, Ajit, and Karanki, 2015). On the other hand, in more complex systems, increased reliability does not itself increase safety. In this respect, this section gathers information and metrics pertinent to the reliabilities, severities, and maintainabilities of systems required for the operation of marine engines that use the considered alternative fuels. Since the commercial use of alternative fuels in the maritime sector is currently limited, the available data are scarce thus generating high uncertainty. However, studies from other industries provide useful insights into the risk characteristics of the investigated fuels.
4.1.1 Hydrogen
Hydrogen is a fuel that is characterised by a high flammability (Popp and Müller, 2021). As a result, leaks may create an explosive atmosphere or fire hazards, especially in the engine room’s confined spaces. Gao et al. (2022) studied a nuclear hydrogen-production system, concluding that the leakage diameter of a hydrogen tank affects the diffusion distance, and can lead to an explosion. To prevent these hazards, appropriate safety measures must be taken, including proper gas management, proper ventilation, hydrogen gas detection, fire detection, and alarm systems (ABS, 2021). Regarding hydrogen combustion, its high-octane number and auto-ignition temperature can lead to potential safety issues (Inal, Zincir, and Dere, 2022). Deflagration and detonation events can also be initiated following hydrogen gas release/leakage (Mukherjee et al., 2017). Notwithstanding, used as a mixture with conventional marine fuels, hydrogen can be directly combusted (direct-injection) by marine engines with no major risk of engine damage (Ampah et al., 2021). Liquid hydrogen, which liquefies below 20 K, requires special apparatus for cryogenic storage and appropriate risk control measures. In particular, pressure-relief valves are necessary to prevent pressure build-up (ABS, 2021). In addition, storage at low temperatures requires thick insulation layers or vacuum insulation (ABS, 2021). Provisions for boil-off gas handling, re-liquefication, gas valve unit/train, vent piping systems, and exhaust masts increase the complexity of safe storage even more (ABS, 2021). Proper care should be given to material selection regarding the storage and transfer of hydrogen. Hydrogen, due to its small molecular size, can easily permeate into various materials and certain materials suffer from hydrogen embrittlement (ABS, 2021), (Mukherjee et al., 2017). The IGF code provides standards for ships using low-flash point fuels, satisfying functional requirements (IMO, 2015). In particular, the standards concern the arrangement, installation, control monitoring, and safety of components and systems pertinent to the use of low-flash point fuels (DNV GL, 2019). Since hydrogen has a low flash point, its use onboard ships is subject to the IGF code. However, hydrogen storage is not covered in the latest edition; consequently, additional considerations are required to deal with the intrinsic characteristics of hydrogen (DNV GL, 2019). Table 2 presents several studies that detailed the most important findings concerning the safety analysis of hydrogen, used mainly in storage tanks and fuelling stations.
4.1.2 Ammonia
Duijm et al. (2005) argue that the risks of using ammonia as marine fuel are comparable to the risk of using other marine fuels (such as LPG). Reports of accidents in the maritime sector are rare with pertinent accident records coming from fishing vessels, where ammonia is used for refrigeration purposes, and from a containership where ammonia leakage was reported by Trivyza et al. (2021). Ammonia is used in Selective Catalytic Reduction (SCR) systems in shipping and also is transported by chemical carriers, which render its handling feasible (ABS, 2020). Compared with hydrogen, ammonia has a narrower flammability range; however, it is extremely reactive (ABS, 2020). It should be kept away from potential ignition sources and several chemicals; it violently reacts with halogens, interhalogens, and oxidizers, leading to explosions (ABS, 2020). The potential leakage of ammonia can create situations where high toxicity and corrosiveness are present, increasing the probability of an explosion (Popp and Müller, 2021). Nonetheless, the risk of fire is considered lower than for other fuels (Zincir, 2022). Ammonia has a high auto-ignition temperature, which can lead to unstable combustion or even unburned ammonia emissions at low engine loads and high engine speeds (Zincir, 2022), hence generating potential implications for engine health. One major drawback of ammonia is that it is toxic to humans, leading to severe health complications (Duijm, et al., 2005). In addition, the IGC code has strict regulations concerning materials that can be used for ammonia. Iron, steel, and specific non-ferrous alloys should be selected for storage and piping (ABS, 2020). According to (ABS, 2020), to reduce the risks related to ammonia, appropriate deluge systems, personal protective equipment, independent ventilation for ammonia spaces, emergency extraction ventilation, and closed-fuel systems are required. In addition, systems for boil off gas handling, reliquefication, gas valve unit/train, nitrogen generating plants, as well as vent piping systems and masts are required, potentially increasing the complexity of ammonia usage (ABS, 2020). Double-walled supply lines are needed to assure safety (Zincir, 2022). However, ammonia can be stored either in pressurised tanks or as a cryogenic liquid (Zincir, 2022). The IGF code does not explicitly cover ammonia (ABS, 2020); therefore, stricter safety measures should be introduced (Popp and Müller, 2021). Table 3 presents several studies and their most important findings pertinent to the safety analysis of ammonia, used mainly in storage tanks, fuelling stations, and ammonia fuel cells.
4.1.3 Methanol
In general, methanol presents a lower number of safety challenges compared with hydrogen or ammonia (Ampah et al., 2021). Since methanol is a liquid at ambient conditions, it can be stored in conventional tanks like ordinary fuels, without any additional equipment required. Only minor amendments to storage systems are potentially needed (Zincir and Deniz, 2021). Nevertheless, methanol has a low flashpoint, quite a wide flammability range, and its flame is invisible (Zincir and Deniz, 2021). Since the combustibility of methanol is similar to fossil fuels that are currently used in marine engines (diesel fuels), fire and explosion requirements could potentially be similar (Popp and Müller, 2021). The considerably high auto-ignition temperature of methanol decreases the risks related to self-ignition or explosion (Zincir and Deniz, 2021). Moreover, the exposure of methanol to some materials (aluminium and titanium alloys) can lead to corrosion (SSPA Sweden AB, 2014), whereas the direct exposure of humans to methanol can lead to severe health complications (SSPA Sweden AB, 2014). Etemad and Choi (2017) argue that shipboard methanol use readily complies with existing regulations. Although methanol has not yet been covered in the IGF code, following a goals-based design approach can provide a means to approval (DNV GL, 2019). Consequently, protective fuel tank location, double barriers on fuel supply lines, ventilation, gas detection, hazardous area classification, and explosion mitigation are among the key safety measures required (SSPA Sweden AB, 2014). Table 4 presents several studies and their most important findings pertinent to the safety analysis of methanol, used in fuel cells and as a fuel in a marine engine.
4.2 Emissions
It is vital to determine the environmental performance of the shipboard use of alternative fuels in order to quantify their environmental impact, as well as their associated decarbonisation potential. Both the fuel production and consumption phases must be considered. Ship operating emissions are related to the engine parameters and are also strongly influenced by the type of fuel used, whereas lifecycle emissions may vary according to the fuel production methodology, as illustrated in Figure 3. In this review, the use of the considered alternative fuels is assessed, based on the corresponding emission factors and the production methodology.
Brown ammonia refers ammonia produced according to the conventional Haber-Bosch process, using natural gas as a feedstock (Kyriakou, et al., 2020). Green ammonia production involves the use of renewable energy sources and biomass or other municipal waste feedstocks. The process includes hydrogen production through electrolysis, as well as nitrogen production by the cryogenic separation of ambient air. These two constituents are then combined in a process called ammonia synthesis (Eason & Biegler, 2015) to produce gaseous ammonia.
Hydrogen production is also classified as green or brown; the former produced by electrolysis or electrical energy from renewable sources, whereas the latter involves natural gas as a feedstock. The hydrogen production phase characteristics reported herein are based on electricity generated by renewable wind power.
Two methods are employed for methanol production: the conventional method that uses natural gas as a feedstock, and the renewable, biomass-based method. The former utilises an energy-intensive process called hydrogenation of CO and CO2 as well as reversed water-gas shift reactions (Pirola, et al., 2018). The latter uses forest and agricultural residues, which are reformed to produce synthetic gas in a treatment unit. This gas is then fed into the synthesis unit to produce methanol (Svanberg, et al., 2018). Other renewable processes include hydrogen production from water electrolysis, which is then fed into a methanol synthesis reactor, along with carbon captured from the air, to produce methanol (Holmgren, et al., 2012).
Apart from the considered alternative fuels, two conventional fuels are also detailed in this section, namely, liquefied natural gas (LNG) and marine diesel oil (MDO), facilitating a comparison of the relative metrics for the production and operation phases. Table 5 lists the calorific values and engine performance parameters for the considered fuels. It must be noted that fuel consumption is specific to the engine’s characteristics. Since it is not possible to use the same engine (type and size) for all selected fuels, the brake specific fuel consumption and the brake efficiency of each engine is reported. The fuel consumption data were retrieved from (Smith, et al., 2014) and (Gilbert, et al., 2018) and efficiency data from (Gill, et al., 2012), (Cheng, et al., 2008), and (Chen, et al., 2017).
Achuthanandam (2021) argued that the technical maturity of the alternative fuels, compared with other established technologies (such as LNG), was a key driver for further developments towards their wider usage. As ammonia and hydrogen are carbon-free fuels, they do not produce carbon emissions in the ship operation phase. Table 6 provides the emission parameters for the production and operation phases, as well as the technical maturity of several fuels.
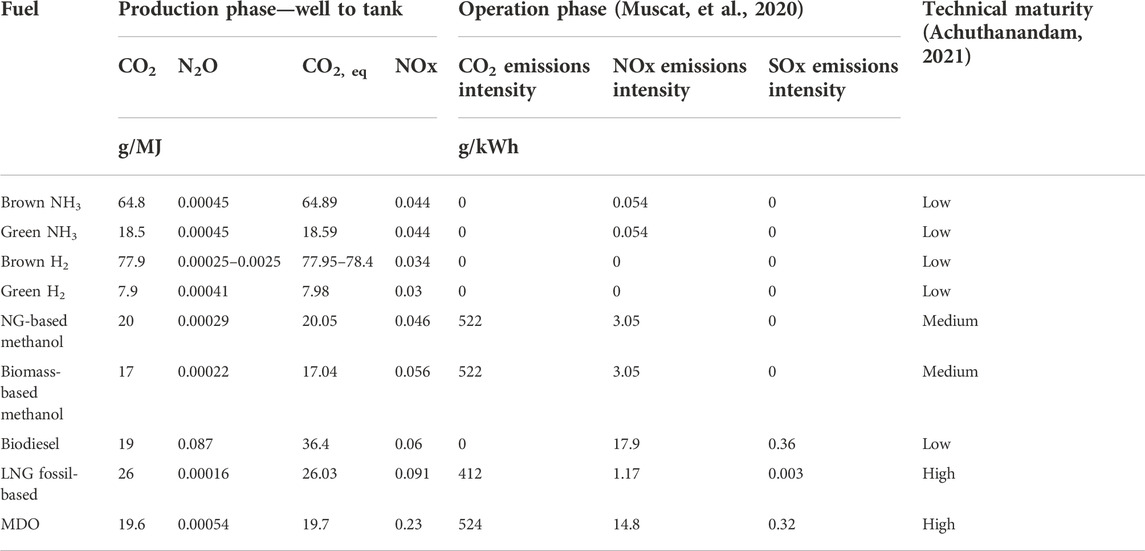
TABLE 6. Emission parameters for production and operation phases, as well as the technical maturity of several fuels (Hwang, et al., 2020), (Achuthanandam, 2021), (Muscat, et al., 2020).
According to this table, the nitrogen oxide emission intensities of alternative fuels are much lower compared with other fossil-based and bio-based fuels, contributing to their lower environmental impacts. Gilbert et al. (2018) reported the NOx-free operation of hydrogen combustion engines; however, since pure hydrogen combustion is difficult, pilot fuel was used. Therefore, NOx was expected to be present at the outlet of the engine (Lilik, et al., 2010). On the other hand, depending on the engine settings, hydrogen combustion led to significant temperature increases in-cylinder (2,000–2,300 K), which resulted in NOx formation, ranging between 40 and 300 ppm (Kikuchi, et al., 2022), (Lewis, 2021).
Regarding methanol, despite having almost half the gravimetric energy density of diesel, its lower carbon content results in reduced CO2 emissions by approximately 25% (compared with diesel fuels). Methanol combustion also results in reduced NOx emission, whilst SOx and soot emissions were almost eliminated, as demonstrated in the previous chapter. The shipping industry requires further investigation into its utilisation of marine fuel, since its technological maturity is still at medium levels. Although retrofitted engines are in use in some cases, a proper investigation is essential to address limitations regarding supply chain and increased costs. It must be noted that hydrogen, ammonia, and methanol have high scalabilities since technologies for their production are already established.
Another metric that needs to be considered is the global warming potential (GWP) related to the fuel production phase emissions. The metric used to assess this is nitrous oxide emissions (N2O), produced in various processes during production. According to the IPCC (Myhre, et al., 2013), N2O’s 20-year GWP is 280 and its 100-year GWP exceeds 300, making it a significant contributor to climate change.
Green and brown ammonia production processes release similar amounts of N2O and NOx emissions; however, the former results in a significant reduction in CO2 emissions (70%), leading to a lower GWP (Bicer, et al., 2016). For hydrogen production, much lower GWP is achieved (reducing both CO2 and NOx emissions) when the energy intensive steam reforming process is substituted with wind energy-based electrolysis (Cetinkaya, et al., 2012). For methanol production, lower GWP is obtained when biomass feedstock is used; both N2O and CO2 emissions are reduced (Chen, et al., 2019). Biodiesel production is associated with significant GWP, compared with conventional fuels such as MDO and LNG, since CO2,eq releases are higher. From the preceding, it is deduced that the use of alternative fuels is advantageous in terms of GWP (GHG emissions) and non-GHG emissions (NOx), compared with the currently employed marine fuels (MDO and others).
4.3 Fuels energy density and storage
In this section, the storage volumes required for the specified fuels are assessed to determine their suitability for shipboard use. Liquified fuels require cryogenic equipment and insulation as well as certain tank volumes. Comparative assessments and suitable ship types are determined based on gravimetric and volumetric energy densities, considering marine diesel fuel as a reference. Higher density and storage-intensive fuels are less suitable for ocean-going ships; however, they can be used in ships operating on short routes, thus requiring less fuel storage space (DNV, 2019). According to Figure 4, liquified petroleum gas (LPG) and liquified natural gas (LNG) exhibit similar volumetric energy densities and require approximately 40% more fuel storage space compared with MGO. Both methanol and ammonia are denser than and require more space than MGO, making their adoption for long voyage ocean-going ships challenging. Liquid hydrogen exhibits an 80% lower volumetric energy density than MGO and is much lighter than other alternative fuels, thus requiring a substantially increased storage volume.
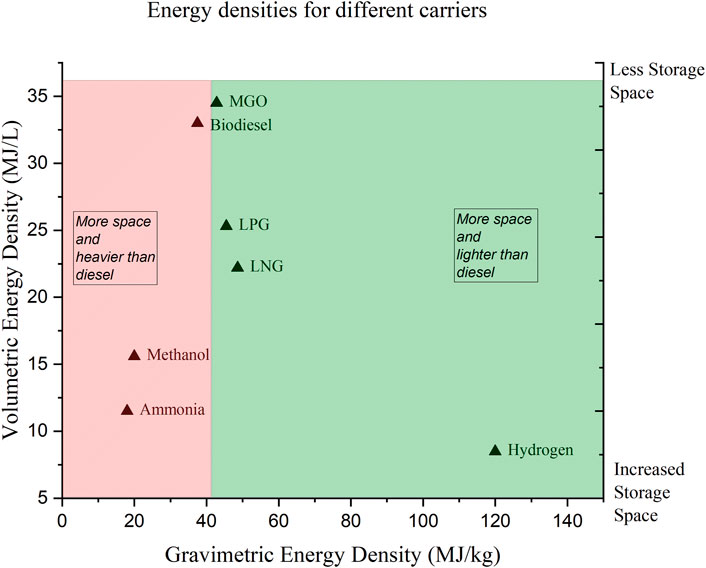
FIGURE 4. Energy densities for different energy carriers. Methanol: (de Vries, 2019), LNG, LPG: (Elgas), Ammonia: (Kim, et al., 2020), Hydrogen: (Cames, et al., 2015), Biodiesel, MGO: (Speirs, et al., 2019).
Generally, the use of methanol, ammonia, and hydrogen as marine fuels is more suitable for small ships (operating on shorter routes), whereas fuels such as LNG, CNG, or biodiesel can be considered as short-term solutions to decarbonise ocean-going shipping. For newbuilt ships, the design of which incorporates fuel storage space, methanol and ammonia can also be adopted as marine fuels in the medium-to longer-terms (Cames, et al., 2021), (Taccani et al., 2020).
Table 7 shows the fuels’ characteristics as well as typical/expected shipboard storage conditions. Liquid hydrogen storage requires cryogenic conditions at temperatures of 20 K (Züttel, 2003). Wang et al. (2021) reported several storage methods using fuel cells for hydrogen-fuelled ships operating at short distances. Markowski and Place (2019) studied a passenger ship operating with two internal combustion engines, using hydrogen stored in compressed form at 200 bar in 12 hydrogen tanks (205 L each). The main advantage of liquid hydrogen (stored at 20–30 K and 1–12.7 bar) is its higher volumetric energy density, at the cost of requiring appropriate insulation. The hydrogen liquefaction process is considered very energy intensive, consuming over 40% of the total energy required by the entire storage process (Rivard, et al., 2019). McKinlay et al. (2021) comparatively assessed ammonia, methanol, and hydrogen storage in ships, concluding that liquid hydrogen was the most volume-intensive, requiring almost double the storage space of ammonia and four times more than methanol. Another method used for hydrogen storage is liquid organic hydrogen carriers (LOHC). These are high purity aromatic compounds that can absorb and release hydrogen through chemical reactions (Elmoe, et al., 2006). Examined a large-ship installation that included a 40 MW engine, and calculated that a consumption of 1 GWh is essential for the production of 38 tonnes of hydrogen, requiring 680 tonnes of LOHC storage space; this is not feasible for onboard applications.
4.4 Cost
In this section, the costs of the production and operation phases for the investigated fuels are analysed. The costs are categorised into primary fuel costs (market based) and production methods, as shown in Figure 5. Nikolaidis and Poullikas (2017) and El-Emam and Özcan (2019) reported that the market cost of conventional hydrogen production from natural gas feedstocks is close to 2.08 $/kg (0.017 $/MJ). The cost of wind-based electrolysis is more than double this, as a significant amount of energy is needed (Ji & Wang, 2021).
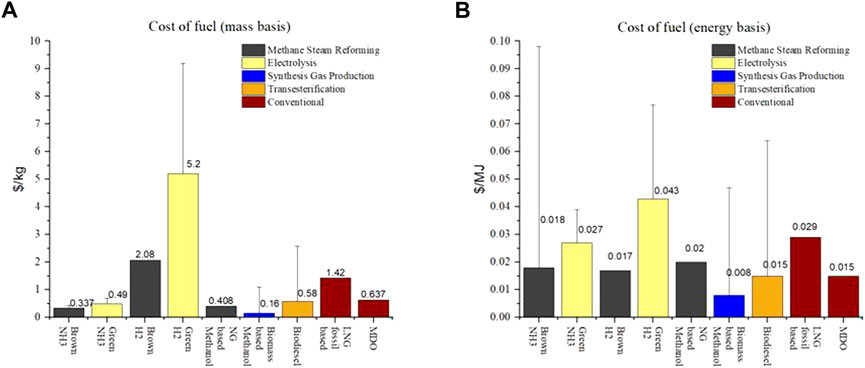
FIGURE 5. Production method costs for brown NH3 (Boulamanti & Moya, 2017), (Kurien & Mittal, 2019); green NH3 (Armijo & Philibert, 2020); brown H2 (Nikolaidis & Poullikkas, 2017); green H2, (Nikolaidis & Poullikkas, 2017), (El-Emam & Özcan, 2019); NG-based methanol, (Boulamanti & Moya, 2017); biomass-based methanol, (IEA, 2013); biodiesel, (Gebremariam & Marchetti, 2018); and the market fuel prices for LNG fossil-based fuel, (Bunker, Global Average 20 Ports Bunker Prices); MDO, (Bunker, Global Average 20 Ports Bunker Prices). (A) Mass-based, (B) energy-based.
Armijo and Philibert (2020) estimated the cost of ammonia production in different regions of Chile and Argentina to be in the range 0.49–0.7 $/kg (0.026–0.037 $/MJ), considering water electrolysis and the use of electricity from wind and photovoltaics (PV). Nayak-Luke and Alcántara (2020) concluded that green ammonia production is highly dependent on both climate conditions (wind speed and solar irradiance) and material costs, estimating a market cost of ammonia in the range 0.5–0.9 $/kg (0.026–0.047 $/MJ).
Boulamanti and Moya (2017) and Kurien and Mittal (2022) reported that the cost of methanol fuel production using conventional techniques with natural gas as a feedstock is significantly smaller than the biomass-based production, at the expense of an increased carbon footprint. In terms of conventional techniques, the steam reforming process is the most capital-intensive. This is also the case for methanol production from natural gas, where the cost is similar to ammonia: approximately 0.4$/kg or 0.021 $/MJ. The cost of biomass-based methanol production is highly dependent on the primary feedstock cost, generating considerable production cost discrepancies. Hamelinck and Faaij (2006) reported methanol prices of approximately 0.16 $/kg (0.008 $/MJ), whereas IRENA (IEA, 2013) reported prices of approximately 1 $/kg (0.05 $/MJ) for a wood-based feedstock. Recent studies (Methanol Institute, 2020) estimated the production cost of conventional methanol in Europe to be close to 0.47 $/kg (0.024 $/MJ).
The cost of biodiesel production is in the range 0.58–2.41 $/kg (0.015–0.06 $/MJ), depending on the primary feedstock type. Sakai et al. (2009) and Karmee et al. (2015) reported production costs in the range 0.58–1.04 $/kg (0.015–0.028 $/MJ) for a waste cooking oil feedstock, whereas Gebremariam and Marchetti (2018) reported biodiesel production costs of 1.16–2.41 $/kg (0.031–0.064 $/MJ) for a palm oil feedstock.
For reference, the Marine Diesel Oil (MDO) market price average over the last 3 years was estimated to be 0.631 $/kg (0.015 $/MJ) (Bunker, Global Average 20 Ports Bunker Prices), whereas the respective LNG average price is 0.568 $/kg (0.012 $/MJ).
Apart from fuel production costs and market prices, the costs (capital and operational) of ship power plants and their components are essential. Livanos, et al. (2014) reported an average capital cost factor for marine diesel engines of approximately 493 $/kW. For ammonia-fuelled, dual-fuel marine engines, capital cost factors in the range 360–600 $/kW were reported by Lloyd’s (2020) and MAN (2018). For marine dual-fuel engines using methanol, the capital cost factor is around 265–$/kW (Baldi, et al., 2019). Korberg et al. (2021) reported a capital cost factor for dual-fuel marine engines operating using hydrogen in the range 470-700 $/kW.
5 Recommendations for future research initiatives
Based on the literature review and the discussed findings, major research directions are identified that can fuel future studies and ideas. These are summarised as follows:
1-Investigations using simulation tools of various complexities, complemented by small scale experimental studies to reveal insights into alternative fuel combustion and to estimate trade-offs in performance and emission parameters. This direction will lead to the development of adequate combustion models which can subsequently be used to optimise engine settings and configurations.
2-Lifecycle assessment combined with detailed technoeconomic and environmental analyses of alternative options, considering all phases of fuel production from well-to-engine. This is expected to identify the most promising solutions, allowing holistic assessment of performance and environmental and economic parameters, required for designing individual parts of the complete ecosystem.
3- Developing methods and frameworks to assure adequate safety of the technologies/systems pertinent to the shipboard use of alternative fuels. This is expected to support the development of required safety systems and to specify mitigation measures for the safe and cost-effective design, operation, and maintenance of fuel storage and usage systems.
4-Holistic design of studies that include optimisation based on several objectives related to emissions, performance, safety, reliability, maintainability, and costs in order to define feasible engines and power plant layouts for the shipboard use of alternative fuels and their specifications. This activity can also include consideration of other compatible technologies and the identification of optimal and feasible power plant configurations that can be used in various ship types/sizes.
5-Investigation of the complete supply chain, from production to use, to proposed phases and steps that allow for optimal operational efficiencies and minimum environmental footprints, considering the future outlook and expansion of both supply and demand.
6-Use of fourth industrial revolution concepts (AI, machine learning, digital twins) for developing trustworthy digital representations of the systems used for the production, bunkering, and power plants related to alternative fuels. This is expected to provide tools for developing cost-effective intelligent systems for monitoring, operation, and maintenance of the relative systems.
These identified areas are illustrated in Figure 6.
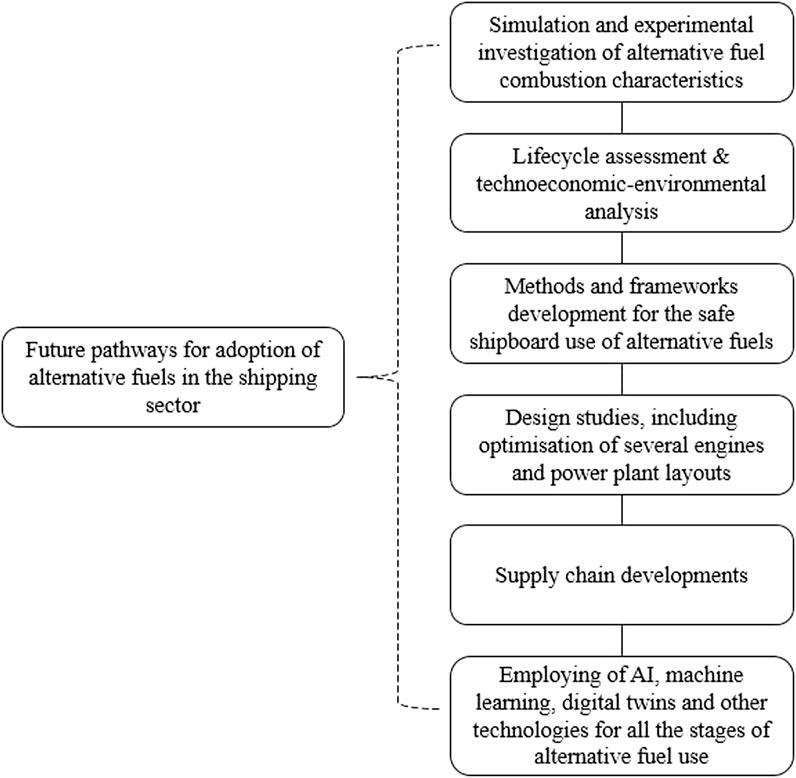
FIGURE 6. Recommendations and future initiatives for the adoption of alternative fuels in the shipping sector.
6 Conclusion
This study conducted a comprehensive literature review aimed at identifying several aspects pertinent to the use of hydrogen, ammonia, and methanol as alternative fuels in marine engines and shipping operations. Several key performance indicators, including engine performance/emissions, safety aspects, technical maturity, cost, and storage space requirements were employed to comparatively assess the characteristics of both the production and operating phases. Developments and trends for the considered fuels and fuel combinations were identified, and recommendations for future research directions were provided. The main highlights of this study are summarised as follows.
Several challenges were identified and discussed regarding the utilisation of hydrogen and ammonia fuels in compression-ignition internal combustion engines. In addition, the expected benefits from these alternative fuels used in dual-fuel engines are prominent and include CO2 emissions reduction as well as the curtailment of other pollutants. NOx emissions significantly depend on the engine settings and injection strategy for both diesel and alternative fuel. Alternative fuels present are advantageous for substantially reducing GHG and non-GHG emissions during a ship’s operation phase (lifetime), compared with conventional fuels. For the fuel production phase, alternative fuels produced using fossil fuel feedstocks exhibit higher GWPs compared with MGO and LNG. Due to storage constraints, ammonia, hydrogen, and methanol are promising solutions for the short voyage sea shipping sector. Production methods and feedstocks play an important role in the final price of fuels, and therefore their adoption.
The main research gaps were identified based on the provided recommendations for future research directions. The main pillars of future research are grouped into the following directions: 1) quantification of combustion characteristics for marine engines, 2) lifecycle assessment and comprehensive techno-economic-environmental analyses for the considered alternative fuels, 3) safety frameworks and methods for cost-effective risk mitigation, 4) holistic design of the complete ecosystem, considering shipboard and shore plants, 5) supply chain optimisation to increase operational efficiencies and resilience, and 6) the development of digital representations and the use of machine learning methods/tools for enabling the efficient, safe, and environmentally friendly operation of ship power plants using alternative fuels.
This study is limited by the availability of public information pertinent to the use of alternative fuels in marine engines and ships. It is expected that future studies will be published in the short-to medium-term; therefore, an update on this study will be required. Other limitations are pertinent to areas that are not investigated herein. Future studies can expand on analyses design and the improvement of production methods for alternative fuels, energy security, and cost-effective materials to enable the shipboard use of alternative fuels (Bastug et al., 2022), (Bunker), (Durbin and Jugroot, 2013), (Gkerekos et al., 2019)., (Kaliszewski et al., 2020).
Author contributions
GT and EB, conceived and designed the analysis, PK and CT collected the data, performed the analysis and wrote the paper.
Funding
The study was carried out in the framework of the Carnot Marine Vessel APUs—Feasibility project, which is funded by the Innovate UK and the Grant Number is 10008878.
Acknowledgments
The authors greatly acknowledge the funding received from DNV AS and RCCL for MSRC establishment and operation. The opinions expressed herein are those of the authors and should not be construed to reflect the views of Innovate UK, DNV AS, or RCCL.
Conflict of interest
The authors declare that the research was conducted in the absence of any commercial or financial relationships that could be construed as a potential conflict of interest.
Publisher’s note
All claims expressed in this article are solely those of the authors and do not necessarily represent those of their affiliated organizations, or those of the publisher, the editors and the reviewers. Any product that may be evaluated in this article, or claim that may be made by its manufacturer, is not guaranteed or endorsed by the publisher.
References
Abdelaal, M., Rabee, B., and Hegab, A. (2013). Effect of adding oxygen to the intake air on a dual-fuel engine performance, emissions, and knock tendency. Energy, 612–620.
Abioye, O., Dulebenets, M. A., Kavoosi, M., Pasha, J., and Theophilus, O. (2021). Vessel schedule recovery in liner shipping: Modeling alternative recovery options. IEEE Trans. Intell. Transp. Syst. 20, 6420–6434. doi:10.1109/tits.2020.2992120
Anderson, K., and Bows, A. (2012). Executing a scharnow turn: Reconciling shipping emissions with international commitments on climate change. Carbon Management, 615–628.
Armijo, J., and Philibert, C. (2020). Flexible production of green hydrogen and ammonia from variable solar and wind energy: Case study of Chile and Argentina. Int. J. Hydrogen Energy 45, 1541–1558. doi:10.1016/j.ijhydene.2019.11.028
Babayev, R., Andersson, A., Dalmau, S. A., Im, H. G., and Johansson, B. (2022). Computational comparison of the conventional diesel and hydrogen direct-injection compression-ignition combustion engines. Fuel, 121909.
Baldi, F., Brynolf, S., and Maréchal, F. (2019). International conference on efficiency, cost, optimization, simulation and environmental impact of energy systems.The cost of innovative and sustainable future ship energy systems
Bastug, S., Haralambides, H., Esmer, S., and Eminoglu, E. (2022). Port competitiveness: Do container terminal operators and liner shipping companies see eye to eye? Mar. Policy 135, 104866. doi:10.1016/j.marpol.2021.104866
Bayraktar, H. (2008). An experimental study on the performance parameters of an experimental CI engine fueled with diesel–methanol–dodecanol blends. Fuel, 158–216.
Bicer, Y., Dincer, I., Zamfirescu, C., Vezina, G., and Raso, F. (2016). Comparative life cycle assessment of various ammonia production methods. J. Clean. Prod. 135, 1379–1395. doi:10.1016/j.jclepro.2016.07.023
Blarigan, P. (2000). “Advanced internal combustion engine research,” in Proceedings of the DOE hydrogen program review.
Boulamanti, A., and Moya, J. (2017). Production costs of the chemical industry in the EU and other countries: Ammonia, methanol and light olefins. Renew. Sustain. Energy Rev. 68, 1205–1212. doi:10.1016/j.rser.2016.02.021
Buhaug, O., Corbett, J. J., Endresen, Ø., Eyring, V., Faber, J., Hanayama, S., et al. (2009). Second IMO GHG study 2009 update of the 2000 GHG study: Final report. London: IMO.
Bunker, S. &., Global average 20 ports bunker prices. [Online]Available at: https://shipandbunker.com/prices/emea/nwe/nlrtm-rotterdam#MGO.
Cames, M., Graichen, J., Siemons, A., and Cook, V. (2015). Emission reduction targets for international aviation and shipping. Brussels: EU.
Cames, M., Wissner, N., and Sutter, J. (2021). Ammonia as a marine fuel Risks and perspectives. Berlin: sn.
Canakci, M., Sayin, C., Ozsezen, A., and Turkcan, A. (2009). Effect of injection pressure on the combustion, performance, and emission characteristics of a diesel engine fueled with methanol-blended diesel fuel. Energy & Fuels, 2908–2920.
Cetinkaya, E., Dincer, I., and Naterer, G. (2012). Life cycle assessment of various hydrogen production methods. Int. J. Hydrogen Energy 37, 2071–2080. doi:10.1016/j.ijhydene.2011.10.064
Chen, K., Xin, X., Niu, X., and Zeng, Q. (2020). Coastal transportation system joint taxation-subsidy emission reduction policy optimization problem. J. Clean. Prod. 247, 119096. doi:10.1016/j.jclepro.2019.119096
Chen, Z., Shen, Q., Sun, N., and Wei, W. (2019). Life cycle assessment of typical methanol production routes: The environmental impacts analysis and power optimization. J. Clean. Prod. 220, 408–416. doi:10.1016/j.jclepro.2019.02.101
Chen, Z., Zhang, F., Xu, B., Zhang, Q., and Liu, J. (2017). Influence of methane content on a LNG heavy-duty engine with high compression ratio. Energy 128, 329–336. doi:10.1016/j.energy.2017.04.039
Cheng, C., Cheung, C., Chan, T., Lee, S., and Yao, C. (2008). Experimental investigation on the performance, gaseous and particulate emissions of a methanol fumigated diesel engine. Sci. Total Environ. 389, 115–124. doi:10.1016/j.scitotenv.2007.08.041
Cheng, C., Cheung, C., Chan, T., Lee, S., and Yao, C. (2008). Experimental investigation on the performance, gaseous and particulate emissions of a methanol fumigated diesel engine. Sci. total Environ. 389, 115–124. doi:10.1016/j.scitotenv.2007.08.041
Chintala, V., and Subramanian, K. (2014). Assessment of maximum available work of a hydrogen fueled compression-ignition engine using exergy analysis. Energy, 162–175.
Corbett, J., and Winebrake, J. (2008). Emissions tradeoffs among alternative marine fuels: Total fuel cycle analysis of residual oil, marine gas oil, and marine diesel oil. J. Air & Waste Manag. Assoc. 58, 538–542. doi:10.3155/1047-3289.58.4.538
Datta, A., and Mandal, B. (2016). Impact of alcohol addition to diesel on the performance combustion and emissions of a compression-ignition engine. Appl. Therm. Eng. 98, 670–682. doi:10.1016/j.applthermaleng.2015.12.047
de Vries, N. (2019). Safe and effective application of ammonia as a marine fuel, s.l. Delft University of Technology.
Deng, J., Wnag, X., Wei, Z., Wang, L., Wang, C., and Chen, Z. (2021). A review of NOx and SOx emission reduction technologies for marine diesel engines and the potential evaluation of liquefied natural gas fueled vessels. Science of the Total Environment, 144319.
Dhyani, V., and Subramanian, K. (2021). Development of online control system for elimination of backfire in a hydrogen fuelled spark ignition engine. Int. J. Hydrogen Energy 46, 14757–14763. doi:10.1016/j.ijhydene.2020.08.148
Dimitriou, P., Kumar, M., Tsujimura, T., and Suzuki, Y. (2018). Combustion and emission characteristics of a hydrogen-diesel dual-fuel engine. Int. J. Hydrogen Energy 43, 13605–13617. doi:10.1016/j.ijhydene.2018.05.062
Ding, W., Wang, Y., Dai, L., and Hu, H. (2020). Does a carbon tax affect the feasibility of Arctic shipping. Transp. Res. Part D Transp. Environ. 80, 102257. doi:10.1016/j.trd.2020.102257
Durbin, D., and Jugroot, C. (2013). Review of hydrogen storage techniques for on board vehicle applications. Int. J. Hydrogen Energy 38, 14595–14617. doi:10.1016/j.ijhydene.2013.07.058
Eason, J., and Biegler, L. (2015). Reduced model trust region methods for embedding complex simulations in optimization problems. Comput. Aided Chem. Eng., 773–778.
El-Emam, R., and Özcan, H. (2019). Comprehensive review on the techno-economics of sustainable large-scale clean hydrogen production. J. Clean. Prod. 220, 593–609. doi:10.1016/j.jclepro.2019.01.309
Elgas, L. Energy content LNG. [Online][Not Available in External Pubmed]Available at: http://lng.elgas.com.au/energy-content-lng.
Elmoe, T., Sorensen, R. Z., Quaade, U., Christensen, C. H., Norskov, J. K., and Johannessen, T. (2006). A high-density ammonia storage/delivery system based on Mg(NH3)6Cl2 forSCR–DeNOxin vehicles. Chem. Eng. Sci. 61, 2618–2625. doi:10.1016/j.ces.2005.11.038
Eyring, V., Isaksen, A. S.A., Berntsen, T, Collins, W. J., Corbett, J. J., Endersen, O., et al. (2010). Transport impacts on atmosphere and climate: Shipping. Atmospheric Environment, 4735–4771.
Gebremariam, S., and Marchetti, J. (2018). Economics of biodiesel production: Review. Energy Conversion and Management, 74–84.
Gilbert, P., Walsh, C., Traut, M., Kesieme, U., Pazouki, K., and Murphy, A. (2018). Assessment of full life-cycle air emissions of alternative shipping fuels. J. Clean. Prod. 172, 855–866. doi:10.1016/j.jclepro.2017.10.165
Gill, S., Chatha, G., Tsolakis, A., Golunski, S., and York, A. (2012). Assessing the effects of partially decarbonising a diesel engine by co-fuelling with dissociated ammonia. Int. J. Hydrogen Energy 7, 6074–6083. doi:10.1016/j.ijhydene.2011.12.137
Gkerekos, C., Lazakis, I., and Theotokatos, G. (2019). Machine learning models for predicting ship main engine fuel oil consumption: A comparative study. Ocean Engineering, 106282.
Gray, J., Dimitroff, E., Meckel, N., and Quillian, R. (1966). Ammonia fuel - engine compatibility and combustion. SAE Technical.
Guo, Z., Li, T., Dong, J., Chen, R., Xue, P., Wei, X., et al. (2011). Combustion and emission characteristics of blends of diesel fuel and methanol-to-diesel. Fuel, 1305–1308.
Gürel, S., and Shadmand, A. (2019). A heterogeneous fleet liner ship scheduling problem with port time uncertainty. Central European Journal Operation Resources, 1153–1175.
Holmgren, K., Berntsson, T., Andersson, E., and Rydberg, T. (2012). System aspects of biomass gasification with methanol synthesis – process concepts and energy analysis. Energy, 817–828.
Hwang, S., Gil, S. J., Lee, G. N., Lee, J. W., Park, H., Jung, K. H., et al. (2020). Life cycle assessment of alternative ship fuels for coastal ferry operating in republic of Korea. J. Mar. Sci. Eng. 8, 660. doi:10.3390/jmse8090660
Ji, M., and Wang, J. (2021). Review and comparison of various hydrogen production methods based on costs and life cycle impact assessment indicators. Int. J. Hydrogen Energy 46, 38612–38635. doi:10.1016/j.ijhydene.2021.09.142
Johnson, H., Johansson, M., Andersson, K., and Södahl, B. (2013). Will the ship energy efficiency management plan reduce CO2 emissions? A comparison with ISO 50001 and the ism code. Maritime Policy & Management, 177–190.
Kaliszewski, A., Kozlowski, A., Dabrowski, J., and Klimek, H. (2020). Key factors of container port competitiveness: A global shipping lines perspective. Mar. Policy 117, 103896. doi:10.1016/j.marpol.2020.103896
Kanna, I., Arulprakasajothi, M., and Eliyas, S. (2019). A detailed study of IC engines and a novel discussion with comprehensive view of alternative fuels used in petrol and diesel engines. Int. J. Ambient Energy 42, 1794–1802. doi:10.1080/01430750.2019.1614994
Karim, G. (2003). Hydrogen as a spark ignition engine fuel. Int. J. Hydrogen Energy 28, 569–577. doi:10.1016/s0360-3199(02)00150-7
Karmee, S., Patria, R., and Lin, C. (2015). Techno-economic evaluation of biodiesel production from waste cooking oil—A case study of Hong Kong. Int. J. Mol. Sci. 16, 4362–4371. doi:10.3390/ijms16034362
Karvounis, P., and Blunt, M. (2021). Unconstrained extraction of fossil fuels and implication for carbon budgets under climate change scenarios. J. Fluid Flow. Heat. Mass Transf. doi:10.11159/jffhmt.2021.009
Kavtaradze, R., Natriashvili, T., and Gladyshev, S. (2019). Hydrogen-diesel engine: Problems and prospects of improving the working process. SAE Technical Paper.
Kikuchi, K., Hori, T., and Akamatsu, F. (2022). Fundamental study on hydrogen low-NOx combustion using exhaust gas self-recirculation. Processes, 130.
Kim, K., Roh, G., Kim, W., and Chun, K. (2020). A preliminary study on an alternative ship propulsion system fueled by ammonia: Environmental and economic assessments. J. Mar. Sci. Eng. 8, 183. doi:10.3390/jmse8030183
Kong, S.-C. (2007). Combustion efficiency and exhaust emissions of ammonia combustion in diesel engines. San Francisco: 4th annual conference on ammonia.
Korberg, A., Brynolf, S., Grahn, M., and Skov, I. (2021). Techno-economic assessment of advanced fuels and propulsion systems in future fossil-free ships. Renewable and Sustainable Energy Reviews, 110861.
Kumar, S., and Kumar, M., (2013). Numerical modeling of compression-ignition engine: A review. Renewable and Sustainable Energy Reviews, 517–530.
Kurien, C., and Mittal, M. (2019). Review on the production and utilization of green ammonia as an alternate fuel in dual-fuel compression-ignition engines. Energy Conversion and Management, 114990.
Kurien, C., and Mittal, M. (2022). Review on the production and utilization of green ammonia as an alternate fuel in dual-fuel compression-ignition engines. Energy Conversion and Management, 114990.
Kyriakou, V., Garagounis, I., Vourros, A., Vasileiou, E., and Stoukides, M. (2020). An electrochemical haber-bosch process. Joule, 142–158.
Lamas Galdo, M., Santos, L., and Vidal, C. (2020). Numerical analysis of NOx reduction using ammonia injection and comparison with water injection. J. Mar. Sci. Eng. 8, 109. doi:10.3390/jmse8020109
Lamas, M., and Rodriguez, C. (2017). Numerical model to analyze NOx reduction by ammonia injection in diesel-hydrogen engines. Int. J. Hydrogen Energy 42, 26132–26141. doi:10.1016/j.ijhydene.2017.08.090
Lazakis, I., Dikis, K., Michala, A., and Theotokatos, G. (2016). Advanced ship systems condition monitoring for enhanced inspection, maintenance and decision making in ship operations. Transportation Research Procedia, 1679–1688.
Lewis, A. (2021). Optimising air quality co-benefits in a hydrogen economy: A case for hydrogen-specific standards for NOx emissions. Environ. Sci. Atmos. 1, 201–207. doi:10.1039/d1ea00037c
Li, J., Lai, S., Chen, D., Wu, R., Kobayashi, N., Deng, L., et al. (2021). A review on combustion characteristics of ammonia as a carbon-free fuel. Front. Energy Res.
Li, J., Huang, H., Kobayashi, N., He, Z., Osaka, Y., and Zeng, T. (2015). Numerical study on effect of oxygen content in combustion air on ammonia combustion. Energy 93, 2053–2068. doi:10.1016/j.energy.2015.10.060
Li, J., Huang, H., Kobayashi, N., He, Z., Osaka, Y., and Zeng, T. (2016). Research on combustion and emission characteristics of ammonia under preheating conditions. J. Chem. Eng. Jpn./. JCEJ 49, 641–648. doi:10.1252/jcej.15we075
Li, J., Wu, B., and Mao, G. (2015). Research on the performance and emission characteristics of the LNG-diesel marine engine. J. Nat. Gas Sci. Eng. 27, 945–954. doi:10.1016/j.jngse.2015.09.036
Lilik, G., Zhang, H., Herreros, J. M., Haworth, D. C., and Boehman, A. L. (2010). Hydrogen assisted diesel combustion. Int. J. Hydrogen Energy 35, 4382–4398. doi:10.1016/j.ijhydene.2010.01.105
Liu, J., Liu, Z., Wang, L., Wang, P., Sun, P., Ma, H., et al. (2022). Numerical simulation and experimental investigation on pollutant emissions characteristics of PODE/methanol dual-fuel combustion. Fuel Process. Technol. 231, 107228. doi:10.1016/j.fuproc.2022.107228
Livanos, G., Theotokatos, G., and Pagonis, D. (2014). Techno-economic investigation of alternative propulsion plants for Ferries and RoRo ships. Energy Conversion and Management, 640–651.
M Ikegami, K. M. M. S., Miwa, K., and Shioji, M. (1982). A study of hydrogen fuelled compression-ignition engines. Int. J. Hydrogen Energy 7, 341–353. doi:10.1016/0360-3199(82)90127-6
Man, , 2018. ME-LGI applications. [Online] Available at: https://marine.man-es.com/two-stroke/2-stroke-engines/me-lgi-engines.
Markowski, J., and Pielecha, I. (2019). The potential of fuel cells as a drive source of maritime transport. Earth Environmental Sciences.
McKinlay, J., Turnock, C., and Hudson, D. (2021). Route to zero emission shipping: Hydrogen, ammonia or methanol? Int. J. Hydrogen Energy 46, 28282–28297. doi:10.1016/j.ijhydene.2021.06.066
McTaggart-Cowan, G. M. K. H. J. S. A., Mann, K., Huang, J., Singh, A., Patychuk, B., Zheng, Z. X., et al. (2015). Direct injection of natural gas at up to 600 bar in a pilot-ignited heavy-duty engine. SAE Int. J. Engines 8, 981–996. doi:10.4271/2015-01-0865
Methanol, I., 2020. [Online] Available at: https://www.methanol.org/methanol-price-supply-demand/.
Miola, A., and Ciuffo, B. (2011). Estimating air emissions from ships: Meta-analysis of modelling approaches and available data sources. Atmospheric Environment, 2242–2251.
Miyamoto, T., Hasegawa, H., Mikami, M., Kojima, N., Kabashima, H., and Urata, Y. (2011). Effect of hydrogen addition to intake gas on combustion and exhaust emission characteristics of a diesel engine. Int. J. Hydrogen Energy 36, 13138–13149. doi:10.1016/j.ijhydene.2011.06.144
Muscat, A., de Olde, E., de Boer, I., and Ripoll-Bosch, R. (2020). The battle for biomass: A systematic review of food-feed-fuel competition. Glob. Food Secur., 100330.
Myhre, G., Shindell, D., Breon, F. M., Collins, W., Fuglestvedt, J., Huang, J., et al. (2013). “Anthropogenic and natural radiative forcing,” in Climate change 2013 (New York: Cambridge University Press).
Mylonopoulos, F., Boulougouris, E., Trivyza, N., Priftis, A., Cheliotis, M., Wang, H., et al. (2022). Hydrogen vs. Batteries: Comparative Safety Assessments for a High-Speed Passenger Ferry, 12. Applied Sciences, 2919.
Naber, J., and Siebers, D. (1998). Hydrogen combustion under diesel engine conditions. Int. J. Hydrogen Energy 23, 363–371. doi:10.1016/s0360-3199(97)00083-9
Nabi, M., and Hustad, J. (2010). Experimental investigation of engine emissions with marine gas oil-oxygenate blends. Science of The Total Environment, 3231–3239.
Nagarajan, N. S. a. G. (2009). Experimental investigation in optimizing the hydrogen fuel on a hydrogen diesel dual-fuel engine. Energy & Fuels, 2646–2657.
Nayak-Luke, R., and Alcántara, R. (2020). Techno-economic viability of islanded green ammonia as a carbon-free energy vector and as a substitute for conventional production. Energy & Environmental Science, 2957–2966.
Niki, Y., Yoo, D., Hirata, K., and Sekiguchi, H. (2016). ASME 2016 internal combustion engine division fall technical conference.Effects of ammonia gas mixed into intake air on combustion and emissions characteristics in diesel engine
Nikolaidis, P., and Poullikkas, A. (2017). A comparative overview of hydrogen production processes. Renew. Sustain. Energy Rev. 67, 597–611. doi:10.1016/j.rser.2016.09.044
Petzold, A., Hasselbach, J., Lauer, P., Baumann, R., Franke, K., Gurk, C., et al. (2008). Experimental studies on particle emissions from cruising ship, their characteristic properties, transformation and atmospheric lifetime in the marine boundary layer. Atmos. Chem. Phys. 8, 2387–2403. doi:10.5194/acp-8-2387-2008
Pirola, C., Bozzano, G., and Manenti, F. (2018). Chapter 3 - Fossil or renewable Sources for methanol production. s.l. Elsevier.
Pochet, M., Jeanmart, H., and Contino, F. (2020). A 22:1 compression ratio ammonia-hydrogen HCCI engine: Combustion, load, and emission performances. Front. Mech. Eng., 1–16.
Pomonis, T., Jeong, B., and Kuo, C. (2022). Engine room fire safety evaluation of ammonia as marine fuel. J. Int. Marit. Saf. Environ. Aff. Shipp. 6, 67–90. doi:10.1080/25725084.2021.2015867
Reiter, A., and Kong, S. (2008). Demonstration of compression-ignition engine combustion using ammonia in reducing greenhouse gas emissions. Energy fuels. 22, 2963–2971. doi:10.1021/ef800140f
Romano, A., and Yang, Z. (2021). Decarbonisation of shipping: A state of the art survey for 2000–2020. Ocean. Coast. Manag. 214, 105936. doi:10.1016/j.ocecoaman.2021.105936
Ronney, P. D. (1988). Effect of chemistry and transport properties on near-limit flames at microgravity. Combust. Sci. Technol. 122, 123–141. doi:10.1080/00102208808947092
Rujub, M. (2020). Simulation of a unit injector for ammonia injection in a compression-ignition engine. s.n: s.l.
Sakai, T., Kawashima, A., and Koshikawa, T. (2009). Economic assessment of batch biodiesel production processes using homogeneous and heterogeneous alkali catalysts. Bioresour. Technol., 3268–3276.
Saravanan, N., and Nagarajan, G. (2010). Performance and emission studies on port injection of hydrogen with varied flow rates with Diesel as an ignition source. Appl. Energy, 2218–2229.
Saxena, M., Maurya, R., and Mishra, P. (2021). Assessment of performance, combustion and emissions characteristics of methanol-diesel dual-fuel compression-ignition engine: A review. J. Traffic Transp. Eng. 8, 638–680. doi:10.1016/j.jtte.2021.02.003
Sayin, C., Ozsezen, A., and Canakci, M. (2010). The influence of operating parameters on the performance and emissions of a DI diesel engine using methanol-blended-diesel fuel. Fuel, 1407–1414.
Smith, T. W. P., Jalkanen, J. P., Anderson, B. A., Corbett, J. J., Faber, J., Hanayama, S., et al. (2014). Third IMO GHG study. London, UK: International Maritime Organization.
Speirs, J., et al. (2019). Can natural gas reduce emissions from transport? London: Sustainable Gas Institute.
Streibel, T., Schnelle-Kreis, J., Czech, H., and al, e. (2017). Recent advances in chemistry and the environment, 10976–10991.Aerosol emissions of a ship diesel engine operated with diesel fuel or heavy fuel oil
Svanberg, M., Ellis, J., Lundgren, J., and Landälv, I. (2018). Renewable methanol as a fuel for the shipping industry. Renew. Sustain. Energy Rev. 94, 1217–1228. doi:10.1016/j.rser.2018.06.058
Taccani, R., Maggiore, G., and Micheli, D. (2020). Development of a process simulation model for the analysis of the loading and unloading system of a CNG carrier equipped with novel lightweight pressure cylinders. Appl. Sci. (Basel) 10, 7555. doi:10.3390/app10217555
Torregrosa, A. J., (2011). Suitability analysis of advanced diesel combustion concepts for emissions and noise control. Energy, 825–838.
Trivyza, N., Rentizelas, A., and Theotokatos, G. (2018). A novel multi-objective decision support method for ship energy systems synthesis to enhance sustainability. Energy Conversion and Management, 128–149.
Wan, Z., Zhu, M., Chen, S., and Sperling, D. (2016). Pollution: Three steps to a green shipping industry. Nature 530 (7590), 275–277. doi:10.1038/530275a
Wang, Z., Wang, Y., Afshan, S., and Hjalmarsson, J. (2021). A review of metallic tanks for H2 storage with a view to application in future green shipping. Int. J. Hydrogen Energy 46, 6151–6179. doi:10.1016/j.ijhydene.2020.11.168
Wei, L., Yao, C., Han, G., and Pan, W. (2016). Effects of methanol to diesel ratio and diesel injection timing on combustion, performance and emissions of a methanol port premixed diesel engine. Energy 95, 223–232. doi:10.1016/j.energy.2015.12.020
Züttel, A. (2003). Materials for hydrogen storage. Mat. TodayKidlingt. 6, 24–33. doi:10.1016/s1369-7021(03)00922-2
Appendix
Table A1 provides summarised information of the key publications pertinent to compression-ignition engines using hydrogen, ammonia, and methanol as fuels (along with diesel).
Keywords: alternative fuels, marine engine, combusion, hydrogen, ammonia, methanol, susta inability, safety
Citation: Karvounis P, Tsoumpris C, Boulougouris E and Theotokatos G (2022) Recent advances in sustainable and safe marine engine operation with alternative fuels. Front. Mech. Eng 8:994942. doi: 10.3389/fmech.2022.994942
Received: 15 July 2022; Accepted: 31 October 2022;
Published: 28 November 2022.
Edited by:
Evangelos G. Giakoumis, National Technical University of Athens, GreeceReviewed by:
Maxim A. Dulebenets, Florida Agricultural and Mechanical University, United StatesIvica Ančić, University of Rijeka, Croatia
Andrea Coraddu, Delft University of Technology, Netherlands
Copyright © 2022 Karvounis, Tsoumpris, Boulougouris and Theotokatos. This is an open-access article distributed under the terms of the Creative Commons Attribution License (CC BY). The use, distribution or reproduction in other forums is permitted, provided the original author(s) and the copyright owner(s) are credited and that the original publication in this journal is cited, in accordance with accepted academic practice. No use, distribution or reproduction is permitted which does not comply with these terms.
*Correspondence: Gerasimos Theotokatos, Z2VyYXNpbW9zLnRoZW90b2thdG9zQHN0cmF0aC5hYy51aw==