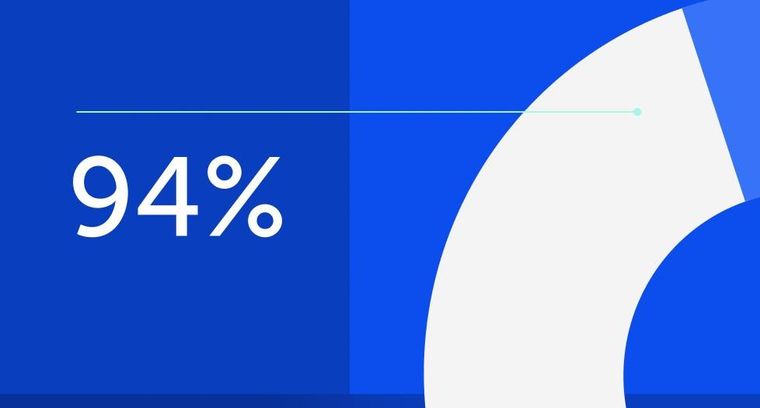
94% of researchers rate our articles as excellent or good
Learn more about the work of our research integrity team to safeguard the quality of each article we publish.
Find out more
REVIEW article
Front. Mech. Eng., 04 January 2023
Sec. Fluid Mechanics
Volume 8 - 2022 | https://doi.org/10.3389/fmech.2022.1072214
This article is part of the Research TopicHorizons in Mechanical Engineering 2022View all 7 articles
Large outdoor fires have become commonplace all over the world. The International Organization for Standardization (ISO) defines large outdoor fires as an urban fire, tsunami-generated fire, volcano-generated fire, wildland-urban interface (WUI) fire, wildland fire, or informal settlement fire, where the total burnout area is significant. Perhaps of all the large outdoor fires, it is wildland fires that spread into urban areas, simply called WUI fires that attract the most attention. A glance at the recent headlines in the summer of 2022 reveals numerous catastrophic WUI fires all over Europe. Across the Atlantic Ocean in the USA, there is yet another destructive WUI fire raging in the USA state of California. With the increasing risks from a changing climate, these large outdoor fire disasters are only going to become more and more commonplace all over the world. More homes will be lost and more lives will be lost. It is the authors opinion that a targeted, multi-disciplinary approach is needed to address the large outdoor fire problem. In this short, invited paper to Horizons in Mechanical Engineering, it is argued that large outdoor fire problem is a fascinating and challenging research area and that engineers have the necessary skills and training to impact a problem that influences millions upon millions of people all over the world. An important danger, present in all large outdoor fires, are firebrands. Firebrands are introduced for non-specialist readers, and the most recent literature is reviewed. Several challenges are discussed, in particular, areas where engineers may help move the needle forward on this globally important topic.
The International Organization for Standardization (ISO) defines large outdoor fires as an urban fire, tsunami-generated fire, volcano-generated fire, wildland-urban interface (WUI) fire, wildland fire, or informal settlement fire, where the total burnout area is significant (ISO 2022a). Throughout the world, large outdoor fires have been responsible for destruction of vast amounts of infrastructure and loss of human life over many years. From the Great Meireki fire of 1657 in Japan, to the Great Chicago Fire of 1872 in United States, to the very recent wildland/WUI fires in United States and Europe, to informal settlement fires in Africa and Asia, large outdoor fires continue to destroy infrastructure and affect people’s lives (see Figure 1).
FIGURE 1. Great Meireki Fire of 1657, Japan (A), Great Chicago Fire of 1872, United States (B), Lick Creek Fire of 2021, Oregon, United States (C), Black Saturday 2009, Australia (Attribution: Richmeister at English Wikipedia) (D), and Imizamo Yothu Informal Settlement Fires of 2017, South Africa (Credit: Aletta Harrison/GroundUp) (E).
Wildland fires that spread into urban areas, known as wildland-urban interface (WUI) fires are capable of enormous destruction. In the year 2018, WUI fires in the state of California destroyed more than 24,000 structures and caused scores of fatalities (CALFIRE, 2018). The Kincade Fire in Sonoma County, California, United States was the largest fire in the 2019 WUI fire season and mandatory evacuation orders affected about 190,000 people living in the area (Palinkas, 2020; Wong et al., 2020). In 2020, California experienced yet another more than 10,000 structure loss due to WUI fires (CALFIRE 2020). In 2021, California experienced an unusually early start of fire season. The Dixie fire alone consumed almost 404,000 ha of land and destroyed more than 1,000 structures (CALFIRE 2021). WUI fires continue to occur throughout the Americas, Australia, Europe, the Middle East and in Asia. The 2009 Black Saturday WUI fires in Victoria, Australia resulted in 173 lives lost, displacing more than 7,500 people (Teague, et al., 2010). In 2014, the Great Fire of Valpariso in Chile destroyed more than 2,500 homes and left 11,000 people homeless (Salinas-Silva, 2015). A series of WUI fires near Hafia, Israel in 2016 forced the evacuation of over 40,000 residents (15% of the city’s population) from 13 different neighborhoods (Marom and Toledo, 2021). Significant WUI fires were observed in South Korea in 2019 (Lee and Lee, 2020) and in Australia in 2019 and 2020 (Deb et al., 2020). The 2022 WUI fire season all across Europe was unprecedented, with so many uncontrolled fires at one time. Although Southern Europe is not new to the dangers of WUI fires, Northern Europe is now experiencing WUI fire disasters (Ganteaume et al. 2021; Plathner et al., 2023). Figure 2 shows examples of WUI areas in various locations around the world.
FIGURE 2. Examples of the wildland-urban interface across the world (Johnston et al., 2019) (Examples of interface WUI in (A) Fort McMurray, Alberta, Canada (Canadian Forest Service/Wiens B.); (B) Sydney, Australia (CSIRO/McArthur N.); (C) Australia (Google Maps); and (D) Slave Lake, Alberta, Canada (University of Alberta/Flannigan M.)). The copyright of this figure is owned by the third party.
It is important to distinguish WUI fires from wildland fires (Manzello and Suzuki, 2022); WUI fires include the combustion of both vegetative fuels and entire communities whereas wildland fires include the combustion of vegetative fuels and occur in uninhabited areas. Estimates place at least 70,000 communities, nearly 46 million structures at risk from WUI fires, which amounts to nearly 120 million people in the United States (Manzello et al., 2018). Studies have linked climate change to increased WUI fire hazards (Abatzoglou and Williams, 2016).
The rise of densely populated urban areas has also seen the development of large urban fires. In Asia, such fires have occurred for hundreds of years (see Figure 1). The most recent of these occurred in the winter of 2016 in Niigata, Japan (Suzuki and Manzello, 2018). In that urban fire, evacuation orders were issued to a total of 744 residents. Similarly, the United States has also experienced several major urban fires, such as the Great Chicago Fire in 1872 (see Figure 1) and the Baltimore Fire in 1904 (Petersen, 2010).
In addition, the rise of informal settlement communities in Southeast Asia and Africa continues to result in large outdoor fires capable of great destruction (Arup, 2018). An informal settlement fire in Imizamo Yethu, Cape Town, South Africa in 2017 displaced more than 9,700 residents and destroyed 2,194 dwellings (Kahanji et al., 2019).
To help alleviate fires that have occurred inside buildings, fire safety science research initially focused on how to best study and understand fire growth inside buildings. These types of fires have been often described as compartment fires, since the fire that is studied is contained to a given room or compartment of a building. Extensive research in this area lead to the development of fundamental understanding of compartment fire dynamics. Concerted research efforts led to the development of the concept of compartmentation, in where the basic design principle was to be able to manage and control an unwanted fire in a given compartment and design buildings to resist the spread of the unwanted fire to other rooms or compartments. In parallel, materials research was also undertaken to be sure that common furniture in homes and buildings could be more ignition resistant. As such, even if an unwanted fire occurs, the materials located inside buildings may be more ignition resistant. While these approaches have been very successful, it is important for readers to grasp that fires that spread outside of a building are not confined to well-defined boundaries, and these complexities render understanding large outdoor fires a very complex endeavor (Manzello et al., 2018). At the same time, vegetation and other human made fuels found outdoors may not be so easily be made more ignition resistant. For interested readers, the books of Drysdale (2011) and Quintiere (2016) provide an overview of compartment fire dynamics. An example of a typical schematic to understand fire growth in a compartment is shown in Figure 3.
FIGURE 3. A typical schematic focused on fires inside a confined room or compartment (Novozhilov 2001).
When fires occur in an outdoor setting, ignition of buildings could occur in three ways: direct flame contact, thermal radiation, and firebrands (and in combination; please see Figure 4). Direct flame contact refers to the situation where a structural component is in direct contact with flaming combustion from an adjacent combusting fuel source. In WUI fires, this could be ornamental vegetation, such as mulch, shrubs, or trees, or other fuel types, such as a burning vehicle or a neighboring structure.
FIGURE 4. Schematic of fire spread mechanisms in large outdoor fires (Top: Direct flame contact, middle: thermal radiation, bottom: firebrands).
Thermal radiation is a form of electromagnetic radiation that is emitted from any object whose temperature is above absolute zero. Due to the combustion of vegetative and structural fuels in WUI fires, any fuel type in proximity to these combustion processes will experience radiation. The probability of ignition is a function of the distance and depends on the time of exposure.
Firebrands are the production or generation of new, far smaller combustible fragments from the original fire source. Firebrands signify any hot object in flight that are capable to ignite other fuel types. Firebrands are produced or generated from the combustion of vegetative and structural fuels and are described in detail as a part of this review.
Naturally, combinations of any of the above are possible. Direct flame contact and thermal radiation act in combination as a flame exists and emits thermal radiation. Direct flame contact and firebrands may also act in combination while direct flame contact is likely dominant. Thermal radiation and firebrands may also act in combination as studied in (Suzuki and Manzello, 2021a).
Firebrands are the production or generation of new, far smaller combustible fragments from the original fire source. In the news media, firebrands are often called embers but this is not a precise terminology. According to ISO (2022a), firebrands are similar to embers but with a slight distinction: ember refers to any small, hot, carbonaceous particle and when embers have the capability of setting additional fires, they become firebrands. Firebrands are produced or generated from the combustion of vegetative and structural fuels. Figure 5 shows examples of firebrands collected from various fuel types.
FIGURE 5. Examples of firebrands collected from vegetative fuel combustion as opposed to structural fuel combustion (Suzuki, 2019). Left: firebrands from vegetative fuel (Manzello et al. 2007), Right: firebrands from structural fuel (Suzuki, 2017b). The rights in this content are owned by the third party.
For some WUI fires, it has been reported that a majority of home losses have been from firebrand attack (Blanchi et al., 2019). In the case of WUI fires, the production of firebrands occurs from the combustion dynamics of vegetative and human-made fuel elements, such as homes and other structures. For urban fires and informal settlement fires, firebrands are produced primarily from human-made fuel elements. Firebrand combustion has a series of important aspects: initial generation or formation from the combustion of both vegetative and structural fuel types, transport, deposition, and ignition of fuel sources generally far removed the original fire source (see Figure 6) (Manzello et al., 2020).
In the combustion process of vegetative fuels, pyrolysis of the fuel elements is an important mechanism. During the vegetative combustion process, wind flow around the fuel elements generates and impose aerodynamic forces. These forces produce moments and stresses along the fuel elements while pyrolysis simultaneously thermally degrades them and reduces their structural integrity. Firebrands are formed when a critical point is reached, and the fuel elements fracture into smaller pieces and are subsequently entrained in the flow (Barr and Ezekoye, 2013). Firebrand generation from structure combustion processes is also an important mechanism to generate firebrands in large outdoor fires.
By far the most often studied aspect of firebrand processes is the transport of firebrands. After the firebrands are generated, they may be initially lofted by the buoyant large outdoor fire plume and transported in the atmospheric boundary layer. Far less investigated, is the deposition process of firebrands after they are generated and transported.
Once the firebrands are generated and transported within the atmospheric boundary layer, firebrands are deposited and will come in contact with other fuel beds. Firebrands may initiate either a smoldering combustion reaction, or a flaming combustion reaction.
There have several reviews on firebrand processes over the past decade (Koo et al., 2010; Manzello 2014; Suzuki 2017a; Fernandez-Pello 2017). Most recently, a comprehensive review was published by Manzello and co-workers in Progress in Energy and Combustion Science (Manzello et al., 2020). Due to growing large outdoor fire problem, the firebrand literature has continued to expand. Recent literature is reviewed that has been published after 2019. The authors contacted several groups active in this area for recent literature as well as conducted an extensive google scholar search. Emphasis is given here on those studies published in the peer-reviewed archival literature (e.g. scientific journals).
In a broad sense, firebrand generation studies may be divided into those focused on vegetative fuels, such as trees or shrubs, and those studies focused on structural fuels, that is fuels present in buildings such as homes. As there are currently no validated computational models capable of simulating firebrand generation processes, the main thrust in this research area has been on experimental studies to attempt to gather the needed physical understanding of the firebrand generation processes at hand. Figure 7 demonstrates that firebrand generation is dependent on many factors.
To understand the complex firebrand generation processes, full-scale experiments that use real-scale vegetation, such as trees or shrubs, or real-scale buildings are the most realistic configurations. This type of experiment provides general understanding of the firebrand generation process and specific features that may only be captured at realistic scales. Yet, conducting these types of experiments is very challenging, requires experience handling combustion processes from real-scale vegetation and structures, and further requires necessary experimental facilities designed for both wind and combustion studies. Alternatively, gaining the information from actual outdoor fire events are always an option, yet due to the lack of any trustable measurement device for quick and easy use, this is not a possible option to date. For these reasons, researchers often turn to post-fire studies to provide some insights (Manzello and Foote, 2014; Suzuki and Manzello, 2018; Suzuki and Manzello, 2022a). Occasionally prescribed burning, that is the controlled application of fire to a defined vegetated area to maintain or modify a system to meet a predetermined objective or objectives (Duff et al., 2018), has been used as an alternate choice to study wildland fire behavior, including firebrand studies (Hiers et al., 2020; Thomas et al., 2021; Thompson et al, 2022). At the same time, there is an ongoing debate on the health of people that live near-by locations where prescribed fires are conducted. Both human and animal populations may be exposed to large amounts of particulates that may pose risk to health (Reisen et al., 2015; Suzuki and Manzello 2022b; Hill et al., 2022).
For structural fuels, an extensive research effort has been undertaken to first conduct a series of full-scale experiments under applied wind fields, and then, go to smaller and smaller-scales (Suzuki and Manzello, 2020a) to determine if simpler experiments are able to capture the complex physics of firebrand generation processes. Results were compared with available data from real fire events, and subsequent efforts at each stage to develop new, smaller, less expensive experimental methods able to capture important factors to study the firebrand generation process (Manzello et al., 2020). Mock-ups of wall and corner assemblies showed that the effect of wind on firebrand generation is significant, which is similar to larger-scale experiments (Suzuki and Manzello, 2019a).
Most recent studies specifically focused on the effect of siding applications, as cedar siding treatments are still popular in many countries, including the United States. Using small-scale experimental methods developed in (Suzuki and Manzello, 2019a; Suzuki and Manzello, 2020a), four kinds of cedar sidings (shakes/shingles, fire retardant treatment/no treatment) were applied to roofing assemblies. Due to the thickness of cedar siding and use of tar paper, the thickness of firebrands generated from roofing assemblies were diverse, as compared to thickness of those firebrands generated from only sheathing materials. For all cases, the firebrand co-efficient was found to increase as the wind speed increased. A fire-retardant treatment on cedar shingle siding applied on roofing assemblies reduced the firebrand production while a fire-retardant treatment on cedar shake siding applied on roofing assemblies did now show much change (Suzuki and Manzello, 2021b). There exists a need to develop, simple and inexpensive laboratory-scale test methods to quantify firebrand production from building materials and this work is providing the necessary scientific-basis for future test method development.
Firebrands could be also generated from small fragments of wood materials, such as wood chips which may be used for landscape purposes. In Hong Kong Polytech University in China, disk wood slices with typical size of firebrands were examined under irradiation to characterize the smoldering burning and the corresponding deformation behaviors (Wang et al., 2021a). Due to interactions between chemical reactions and thermomechanical stresses, four successive deformation stages were observed and hypothesized (I) drying shrinkage to ∪ shape (II) irradiation-driven thermal expansion to ∩ shape (III) pyrolysis shrinkage to ∪ shape, and (IV) oxidation-driven thermal expansion to ∩ shape. For these 5mm to 15 mm thick samples, the degree and occurrence of these deformation stages were sensitive to the aspect ratio. Increasing the slice thickness decreased the deformation in the first three stages but increased the deformation of the fourth stage. The authors indicated that these experimental observations were qualitatively reproduced by a 2-D finite-element numerical model, coupling 3-step heterogeneous kinetics with a thermomechanical solver.
In the case of vegetative fuels, experimental studies are much further behind as compared to those conducted for buildings. To date, there have been very limited studies of vegetative fuels under realistic-scales and even fewer studies undertaken using wind. Only recently have experimental methods been devised to understand these combustion processes under controlled wind fields. Figure 8 shows firebrand generation studies, from complex to simpler scales for vegetative fuels.
FIGURE 8. Firebrand generation from vegetation—actual-scale to simple, smaller-scales (image credit right to left, Rim fire (Wikipedia), Ken Meinhart (USFWS), Manzello et al., 2007, Suzuki and Manzello 2022c, and Hajilou et al., 2021).
For vegetative firebrand studies at realistic-scales, there has been some work done in prescribed fires to understand the size and density of firebrands (the number of firebrands per unit area). Past research focused more on visual approaches (these are reviewed in Manzello et al., 2020) using existing technologies. For example, recent work done in the New Jersey Pine Barrens, United States, compared firebrand generation behavior from two prescribed burns in different conditions. Fire behavior and firebrand generation was coupled, and it was found that fire intensities and fuel characteristics are related to firebrand generation (Thomas et al., 2021).
A tool to monitor firebrand fluxes (the number of firebrands entering the equipment), sizes, and thermal conditions was also developed by combing visual and infrared cameras (Zen et al., 2021). This application was tested in the laboratory and deployed in prescribed fires. According to the authors, the tool shows good measurement of the firebrands size and thermal conditions. The size of entire system is about 2 m (L) x 0.5 m (W) x 1 m (H). The size, structure, and cost of such as system is clearly for research purposes and isnot ready for rapid deployment in large outdoor fires (Manzello et al., 2010).
Recent advances in acoustic analysis, specifically pattern detection, have also enabled the quantification of the rate at which firebrands are observed in the audio recordings of in-fire cameras housed within fire-proof steel boxes that have been deployed on experimental fires (Thompson et al, 2022). The audio pattern being detected is the sound created by a flying firebrand hitting the steel box of the camera. This technique allows for the number of firebrands per second to be quantified and can be related to the fire’s location at that same time interval (using a detailed rate of spread reconstruction) in order to determine the firebrand travel distance. A proof of concept is given for an experimental crown fire that shows the viability of this technique. When related to the fire’s location, key areas of medium-distance spotting are observed that correspond to regions of peak fire intensity. The authors concluded that trends on the number of firebrands landing per square meter as the fire approaches were quantified using low-cost instrumentation.
Even though prescribed burning has the advantages of being conducted on realistic scales, it is evident that these types of events cannot be conducted under conditions typical of actual fires (e.g. high winds). As stated above, there is a concern that both human and animal populations may be exposed to large amounts of particulates that may pose risk to human health during prescribed burning (Suzuki and Manzello 2022b; Hill et al., 2022). For these reasons, researchers have tried to extract meaningful information at less dangerous scales.
Continuing to a simpler individual tree-scale, recent work at Oregon State University in the United States conducted investigations under no controlled wind fields in an outdoor setting (Hudson et al, 2020). The size of firebrands, number flux (defined by the authors as the number of firebrands per m2), and propensity to ignite spot fires (i.e., called char-mark flux of firebrands in their work, this aspect will be discussed later) are reported for several tree species under different combinations of number (one, three or five) and moisture content (11–193%). Douglas-fir, grand fir, western juniper and ponderosa pine trees were investigated. Firebrands were collected on an array of fire-resistant fabric panels and trays filled with water. It was reported that Douglas-fir trees generated the highest average firebrand flux (the number of firebrands per m2) per kilogram of mass loss during torching, whereas grand fir trees generated the highest char-marked firebrand flux (the number of firebrands per m2) per kilogram of mass loss. Western juniper produced the largest fraction of char-marked firebrands, with 30% of the firebrands generated being hot enough to leave char marks. In contrast, only 6% of the firebrands generated by ponderosa pine were hot enough to leave char marks. It was suggested the findings can be used to help understand the propensity of different species of tree to produce firebrands and the portion of firebrands that may be hot enough to start a spot fire.
Another 71 individual trees/shrubs of different heights (1.4 m—6.2 m) were investigated in their follow-up work (Adusumilli et al., 2021). Firebrands were collected using fire-resistant fabrics. Douglas-fir, ponderosa pine, and sagebrush were of their interest. Due to the usage of fire-resistant fabrics, the number of char-marked firebrands was measured. The total number of char-marked firebrands appear to increase as the height of tree/shrubs increase. Sagebrush produced the greatest number of char-marked firebrands and the greatest number of char-marked firebrands per kg of fuel consumed. No conclusive relationship was found between the char-marked firebrand production and moisture content of tree/shrub. Those findings were also supported by observations. Since all these experiments are not conducted in a controlled wind field and many dangerous large outdoor fires occur under windy conditions, care should must be considered in these findings.
In work at the University of Coimbra in Portugal, researchers have made use of particle image velocimetry (PIV), to investigate these generation processes under no wind (Almeida et al., 2021). In their work, the number and characteristics of the firebrands produced during the combustion of four different trees with the size between 1.0 m—1.8 m, were studied. The new methodology presented on PIV imaging proved to be useful in their analysis and the authors concluded future studies are required under wind.
No previous studies on firebrand generation from trees were performed under controlled wind fields. As stated, experiments were either performed in an indoor laboratory, under no wind conditions, or at an outdoor setting, with no controlled wind. As outdoor fires become dangerous in windy conditions, it is essential experiments be performed under controlled wind, to understand the wind effect precisely and also provide necessary data for modelling.
It is clear that conifer tree combustion experiments conducted under an applied wind field should result in new understanding of firebrand generation processes. In order to study firebrands produced from vegetative fuels under wind, new ignition strategies were needed and recently developed at the National Research Institute for Fire and Disaster (NRIFD) in Japan (Suzuki and Manzello, 2022c). Flame length, flame tilt angle, and mass loss rate of a Noble-tree combusting under 3 m/s wind were compared to tree combustion under no wind conditions. Under wind, the mass loss rate increased by at least a factor of 2, as compared to combustion without wind. On the other hand, the flame length under no wind was larger than that under 3 m/s wind. Some of the firebrands produced under 3 m/s wind were larger and heavier and sometimes partially burnt. Under no wind conditions, the firebrand yields from 1.5 m Noble-fir trees were less than 1%. The firebrand yield under 3 m/s increased significantly compared to those under no wind for a similar tree size. These results are due to the wind force. While the study advances the understanding of vegetative combustion under applied wind conditions, additional experiments will be needed in the future to consider other vegetation types, different wind speeds, and a broader range of vegetative fuel sizes.
As it is still difficult and requires a relatively large experimental setting to burn trees, some research performed smaller-scale experiments with branches in the wind tunnel to provide a quantitative means to generate firebrands at the source of a fire. One of these studies were performed at Oregon State University in the United States (Hudson and Blunck, 2019). Samples of four fuel species, Douglas fir, western juniper, ponderosa pine and white oak, were burned in a heated wind tunnel and the time required for the firebrands to form was measured using a DSLR camera. A factorial analysis of variance was used to determine the sensitivity of the time to generation to species, diameter, moisture content, fuel condition (i.e. dowel v. Natural sample), crossflow temperature and crossflow velocity. The diameter of the samples had the greatest effect on the time to generation and the fuel species had the second greatest effect. The small diameter samples were relatively insensitive to changes in other parameters. Natural samples required significantly longer time than dowels to generate a firebrand. According to the authors, fuel morphology is one of the most significant factors influencing firebrand generation.
Another study performed at the University of Maryland in the United States considered firebrand generation from branches of two conifers at a fixed wind speed of 4 m/s (Hajilou et al, 2021). This was a continuation to the initial work of Caton-Kerr et al., 2019 for wooden dowels. At the same time, major downstream gaseous species concentrations were measured. A carbon mass balance was utilized to analyze preliminary results and understand how much of the fuel mass transitions to firebrands vs gases. These results provide a description of the mass burning process and ultimately tie firebrand production to a time-dependent heat-release rate for initialization of firebrand transport in numerical simulations. An average firebrand yield ranging from 3 to 4% of initial dry mass is ultimately presented for lodgepole pine and Douglas fir. It was concluded that future work was required with larger fuel sizes pertaining to real outdoor fire scenarios. The complication here that these studies were conducted before any detailed understanding of the combustion processes were obtained in actual-scales, tree-scales under wind. As a result, the experimental findings, while very important, may be an artifact of the experimental protocols used, not reflecting phenomena in realistic-scales.
With limited number of experiments, the efforts to model firebrand generation from trees in detail have not advanced much since pioneering work conducted at the University of Texas at Austin in the United States (Barr and Ezekoye, 2013). Due to the lack of data to model, Victoria University in Australia conducted a series of physics-based simulations on a trial-and-error basis to reproduce the experimental collection data, which is called an inverse analysis (Wickramasinghe et al., 2022). Once the generation data was determined from the simulation, authors applied the interpolation technique to calibrate the effects of wind velocity, relative humidity, and vegetation species. First, the authors simulated Douglas-fir (Pseudotsuga menziesii) tree-burning and quantified firebrand generation against the tree burning experiment. Then, the authors applied the same technique to a prescribed forest fire experiment conducted in the Pinelands National Reserve (PNR) of New Jersey, the United States. The simulations were conducted with the experimental data of fuel load, humidity, temperature, and wind velocity to ensure that the field conditions are replicated in the experiments. The findings may be of limited value, since the calibration conditions are based on tree burn data under no wind. As discussed, the combustion of conifer trees in the presence of wind is far different.
The transport of firebrands is the most often aspect of firebrand processes investigated. The main reasons are due to the fact that transport processes are the simplest to potentially model, yet validation of these models has remained elusive. Figure 9 lists important factors that influence firebrand transport process.
The most recent studies have been greatly advanced by the use of experimental firebrand generators, a device that produces controlled and repeatable sets of firebrands, as well as increased computational power of computers. The development of experimental firebrand generator technology was pioneered by the authors (Manzello et al., 2008; Suzuki and Manzello, 2011; Manzello, 2014).
Following on the development of the firebrand generator by Manzello, researchers at Victoria University in Australia focused on conducting a set of benchmark experiments using a modified firebrand generator, and validating a numerical model for firebrand transport against this set of experiments (Wadhwani et al., 2022). The validation was conducted for the transport of non-burning and burning cubiform firebrand particles at two flow speeds. Four generic drag sub-models used to estimate drag coefficients that are suited for a wide variety of firebrand shapes were verified for their applicability to firebrand transport modelling. According to the authors, the four sub-models were found to be good in various degrees at predicting the transport of firebrand particles. Work in Japan has also used a modified firebrand generator to investigate firebrand transport (Himoto and Iwami, 2021).
Other studies have been undertaken to image firebrands using firebrand generators. For work done at Tomsk State University in Russia, a study was devoted to the development of new algorithms and their testing and, as such, several laboratory experiments were conducted (Prohanov et al., 2020). Wood pellets, bark, and twigs of pine were used to generate firebrands. An infrared camera was used to obtain the necessary thermal video files. The thermograms were then processed to create an annotated IR video database that was used to test both the detector and the tracker. Following these studies, the analysis showed that the Difference of Gaussian detection algorithm and the Hungarian tracking algorithm upheld the highest level of accuracy and were the easiest to implement. The study also indicated that further development of detection and tracking algorithms using the current approach will not significantly improve their accuracy. It was suggested by the authors that convolutional neural networks hold high potential to be used as an alternative approach.
Another study at North Carolina State in the United States, looked at three methods for quantifying the landing distribution of firebrands: Monte Carlo simulations, importance sampling, and large deviation theory (LDT) (Mendez and Farazmand, 2022). According to the authors, Monte Carlo and importance sampling methods are most efficient in quantifying the high probability landing distances near the mode of the distribution. However, these methods become computationally intractable for quantifying the tail of the distribution due to the large sample size required. It was argued that the most probable landing distance grew linearly with the mean characteristic velocity of the wind field. The authors defined a relative deposited mass as the proportion of mass deposited at a given distance from the main fire, thereby deriving an explicit formula which allowed for the computing of this quantity as a function of the landing distribution at a negligible computational cost.
Some other studies have also begun to look at firebrand deposition and accumulation processes using non-burning particles as surrogates for firebrands. For example, work at Clemson University in the United States have looked at firebrand deposition around a roofing assembly (Nguyen and Kaye, 2021) and an entire building, but the firebrands are not burning (Nguyen and Kaye, 2022a; Nguyen and Kaye, 2022b). The lack of firebrand combustion may be an in important missing piece in these studies, and future work should attempt to compare any results to those that include firebrand combustion. For example, during the combustion process, firebrands are often covered in sticky char formation, and this is not well represented by non-burning particles. In another study at the University of Alabama in the United States, firebrand combustion is considered but it was a modeling study with no experimental validation (Mankame and Shotorban, 2021). As the approach is very interesting, it is hoped future validation will occur.
An important simplifying assumption made in early research into firebrand transport is the terminal-velocity assumption, in which firebrands are assumed to fly at their terminal velocity relative to the wind field. With increases in computational power, researchers at the University of New South Wales in Australia worked to directly simulate the atmospheric conditions resulting from wildland fires, suggesting such simulations may resolve the larger of the turbulent processes involved (Thomas et al., 2020). The researchers used a large eddy simulation of a turbulent plume to examine the validity of the terminal-velocity assumption when modelling the long-range transport of non-combusting embers. The results indicated that the use of the terminal-velocity assumption significantly overestimates the density of firebrand landings at long range, particularly for firebrands with higher terminal fall speeds.
Additional studies from the University of New South Wales in Australia have looked at observational data to better grasp firebrand transport, such as the influence of wildfire area, topography, fuel, surface weather and upper-level weather conditions on long-distance spotting during wildfires (Storey et al., 2020b). The analysis was based on a large dataset of 338 observations, from aircraft-acquired optical line scans, of spotting wildland fires in south-east Australia between 2002 and 2018. Source fire area (a measure of fire activity) was the most important predictor of maximum spotting distance and the number of long-distance spot fires produced. Weather (surface and upper-level), vegetation and topographic variables had important secondary effects. Spotting distance and number of long-distance spot fires increased strongly with increasing source fire area, particularly under strong winds and in areas containing dense forest and steep slopes. General vegetation descriptors better predicted spotting compared with bark hazard and presence variables, suggesting systems that measure and map bark spotting potential need improvement. In another analysis, which was performed with the data from 251 wildfires, the number of spot fires, spotting distance distributions, and the number of “long distance” spotting (longer than 500 m) was investigated (Storey et al., 2020a). Many long distance spotting was associated with a multi-modal distribution type, and the multi-modal distribution suggests that current models of spotting distance, which typically follow an exponential-shaped distribution, could underestimate long-distance spotting.
A new research area, conducted in Japan, has been the investigation of the structure to structure separation distance on firebrand accumulation (Suzuki and Manzello, 2021c). In that study, the effect of structure to structure separation distance on firebrand accumulation was investigated by using a custom designed firebrand generator installed in a real scale wind tunnel. Firebrands accumulated at 4 and 6 m s−1, but no firebrand accumulation zone was observed at 8 and 10 m s−1, regardless of separation distance (SD). Experimental results were compared with a simple CFD flow simulation (no firebrands included). The size of firebrand accumulation zone as well as distance from the structure front was compared with SD in the cases of 4 and 6 m s−1 wind speeds. It was found that firebrands behave differently from SD = 1–2 m, to that of SD = 2–3 m. The results of this study are the first to explore these important interactions between firebrands and structure separation distances.
Once firebrand deposit, a key stage in the overall process is whether firebrands may ignite the fuels that they have landed upon. To best understand the various research approaches used to study firebrand ignition, it is important to consider Figure 10.
Figure 11 further shows a simple schematic that is useful to understand how recent research has been undertaken to better grasp the complex ignition processes induced by firebrands. Firebrand ignition research ranges from investigating an individual firebrand, a group of firebrands burning in the laboratory, to investigating the vulnerabilities of building features in a wind tunnel. Recent work has also tried to better understand the overall heat transfer processes that firebrands impart on fuel beds but it does not look at the details of the fuel bed and firebrand interactions (Bearinger et al., 2020).
In research at Case Western Reserve University in the United States, efforts were undertaken to investigate the effects of gap spacing on the burning behaviors of a group of wooden samples (Kwon and Liao, 2022). In these experiments, firebrands are simulated using nine wooden cubes, 19 mm on each side. The results show that the flame height and the sample mass loss rate have non-monotonic dependencies on the gap spacing. When the gap spacing reduces, the flame height and the mass loss rate first increase due to enhanced heat input from the adjacent flames to each sample. When spacing became less than 10 mm, flames from individual samples are observed to merge into a single large fire. The gaseous flame height was correlated to the solid burning rate. The correlation generally follows previous empirical equations for continuous fire sources. For smoldering combustion, compared to a single burning sample, the smoldering temperature and duration significantly increase due to the thermal interactions between adjacent burning samples.
In work at Hong Kong Polytechnic University in China, a controlled experiment was performed to investigate smoldering and flaming ignitions of stationary disc-shaped wood particles with different diameters (25mm–60 mm) and thicknesses (15 mm–25 mm) under varying radiant heat flux (Wang et al., 2021b). The ignition difficulty, in terms of the minimum heat flux, increased from smoldering ignition to piloted flaming ignition and then to flaming autoignition. As the sample thickness increases, the minimum heat flux, ignition temperature, and burning duration for flaming autoignition all increase, while the peak burning flux decreases, but they are insensitive to the sample diameter. During ignition and burning processes, the disc particle is deformed due to the interaction between chemical reactions and thermomechanical stresses, especially for smoldering. The characteristic thickness of the smoldering front on wood is also found to be 10 mm–15 mm. According the authors, this study sheds light on the size effect on the ignition of wood particles by wildfire radiation and helps understand the interaction between flaming and smoldering wildfires.
Understanding the parameters that affect firebrand burning conditions is needed to quantify and model heat transfer from firebrands to combustible surfaces. In research at Virginia Tech University in the United States, an experimental and analytical effort was conducted to determine the variable relationships that control firebrand burning (Lattimer et al., 2022). A series of experiments were performed to quantify the mass loss rate, temperature, and char diameter change with time for single and arrays of cylindrical firebrands. An analytical model was developed to predict the time dependent burning of firebrands including ash accumulation in forced flow conditions. Six different methods for predicting char oxidation were included in the model to identify the best approach for predicting firebrand burning. Based on the simulation results, the model with char oxidation determined using the heat and mass transfer Reynolds analogy provided the best results with predicted temperatures, char diameter, and mass loss rates within 5%, 4%, and 29% of the single firebrand test data, respectively. Higher differences were predicted with arrays of firebrands, which was attributed to the complex flow field that develops around the firebrands. Analysis of the analytical equations was used to identify the variable relationships affecting firebrand temperature, mass loss rate, char diameter, and burning duration.
In a study conducted at Tomsk State University in Russia (Matvienko et al., 2022), mathematical modeling and laboratory experiments were conducted to better understand the conditions of wood ignition by a single or group of firebrands with different geometry. Their model considered the heat exchange between the firebrands, wood layer and the gas phase, moisture evaporation in the firebrands, and the diffusion gases of water vapor in the pyrolysis zone. In order to test and verify the model, a series of experiments to determine probability and conditions for ignition of wood-based materials caused by wildland firebrands was undertaken. The results of experiments showed that an increase in wind speed lead an increase in the probability of wood ignition. Based on the findings, it was concluded that the ignition curve of wood samples by firebrands was nonlinear and dependent on the wind speed and firebrand size as well as their quantity. There was no ignition of wood samples in the range of wind speed of 0–1 m/s. Other work conducted in China further demonstrated the importance of wind on the ignition of fuel beds (Yang et al., 2022).
Researchers in Australia were interested in better understanding firebrand ignition of wet eucalypt forests from firebrands (Cawson et al., 2022). In their study, firebrands were simulated using cotton cylinders and a sawdust wax-lighter. While it not clear what types of firebrands these were intended to represent, it is well-known that showers of firebrands may ignite various fuel beds with very high moisture content, so future work should consider firebrand showers.
In research conducted in universities in Chile, experiments were carried out using a bench-scale apparatus specifically designed to test the ignition of forest fuel layers from a representative firebrand (Rivera et al., 2020). A cylindrical heater was used to model the firebrand, which allowed to control the incident radiative heat flux on the specimen, from the critical heat flux up to 25 kW/m2, for five different porosities of the fuel layer. Experimental ignition delay times were interpreted based on a theoretical model of the radiative heating of the fuel layer. In the limit of small ignition delay times an analytical expression was derived to correlate the inverse of the ignition time to the incident heat flux. This analytical expression is used to obtain the ignition temperature and effective properties for the forest fuel layers, namely the product of the fuel volume fraction by solid fuel density and solid heat capacity. Analytical solutions were found to be consistent with experimental data and a correlation relating the inverse of the non-dimensional time-to-ignition to the non-dimensional heat flux is provided. As seen in Figure 11, the cartridge heater approach is analogous to hot metal particles, and since firebrands are often reacting themselves, it is not clear how these simplifications represent the actual physical processes at hand.
In work by Oregon State University in the United States, a similar experimental setting with a cartridge heater (representing a firebrand) was used to investigate flaming ignition of five fuel beds: two kind of flat plates (Douglas-fir and cardboard) and three size classes of Douglas-fir shavings (Lc < 1 mm, 4 mm < Lc < 6 mm, and 6 mm < Lc < 12 mm) (Bean and Blunck, 2021). The smaller shavings resulted in quicker ignition than the larger shavings. Both conduction and radiation from a heater to fuel beds play a role in ignition of fuel beds, and larger shavings requires more time to flaming ignition due to the role of radiation. It was found that heat flux would be more significant indictor of ignition than heat temperature.
At the University of Texas in the United States, experiments were conducted to determine a quantitative ignition criterion (Weisses and Ezekoye, 2022). Firebrands were deposited on cellulose insulation fuel beds under an impinging air jet at two different velocities while thermocouple measurements were taken in the fuel bed and cameras recorded the tests in the visible and IR spectrum. The firebrand temperature and the temperatures within the fuel bed were insufficient to predict the ignition of the fuel bed. However, using the IR camera to monitor the growth of the reacting area in the fuel bed, a quantitative definition of ignition was found. Over the course of a test, different growth rates, representing different phases in the ignition process, were apparent in the non-dimensional reacting area. The time to the final growth rate matched well with the flaming ignition times recorded in the visible videos. The average error between the observed and predicted time to flaming ignition was 12%. According to the authors, this non-dimensional reacting area analysis provides a framework for determining ignition in a quantitative way.
While investigating individual ignition is of interest, it remains important to study realistic fuel configurations. As shown in past studies reviewed by Manzello et al., 2020, firebrand accumulation often leads to ignition, and the fuel configuration may only enhance the accumulation process. In recent full-scale experiments in wind tunnels in Japan that considered mulch beds installed in full-scale reentrant corners, the time to the ignition was compared with the ignition behavior and the number of firebrands required for sustained ignition (both smoldering and flaming) was also investigated (Suzuki and Manzello, 2020b). The results showed that accumulation of firebrands may be the key to ignite high fuel moisture content (FMC) fuel beds. Under dried conditions, a single firebrand was able to ignite fuel beds, which is the same result from legacy studies. With fuel moisture content increased, a single firebrand was not able to ignite; ignition requires more than one firebrand. This was explained as follows; the fuel bed with given mass was heated continuously by firebrands intermittently depositing and accumulating on the fuel beds. The number of firebrands required for ignition was larger as the wind speed decreased. A simple model was developed to understand these complex processes. Further efforts to validate this model require high fidelity measurements of important physical parameters needed to better understand firebrand combustion.
In further work at University of California, Berkeley in the United States, researchers studied the propensity of wooden materials in different geometries to ignition by a pile of smoldering wooden dowels, emulating a deposition of firebrands (Richter et al., 2022). In total, five geometries (a flat board and four crevice configurations emulating a sample of wood decking) and three wood types (oriented strand board (OSB), whitewood, and pressure-treated wood) under four windspeeds (0.5 m/s to 1.4 m/s). In all cases, the propensity for ignition increases with wind speed; however, a crevice geometry significantly increases the likelihood for ignition, receiving a significantly higher heat flux from a smoldering pile than a flat plate under the same conditions. All experiments were compared to measurements of heat transfer to an inert sample under the same firebrand loading and wind conditions. The orientation of the crevice with the wind also produced a substantial effect. Comparing inert vs. wood tests revealed the inert board configuration is a reasonable worst-case scenario to understand heating from firebrands to target materials. It was argued that these findings are explained by re-radiation within the crevice configuration, heat losses from the firebrand pile, and the material properties of the wood species, derived from a simple heat transfer analysis. Consequently, both geometry and wood species have a significant effect on the propensity of ignition. The study reinforced what was known in the literature for crevice ignition by firebrands (Manzello et al., 2009).
At the University of Corsica in France, work has been conducted to understand the ignition dangers to decking assemblies (Meerpoel-Pietri et al., 2021). This study investigated the ignition by flaming firebrands of two decking slabs used in French dwellings located in Wildland–Urban Interface. The first decking slab was made of pine and the second one was a thermoplastic composed of polypropylene and calcium carbonate. Flaming firebrands were produced by heating and igniting wood chips of different shapes (square, longitudinal and rectangular) with a cone calorimeter. The firebrands generated preserved their shape during their heating. Their projected area was between 0.07 and 12.00 cm2 and their mass ranged from 0.57 mg to 2.66 g. The location of flaming firebrands, the minimal mass and the minimal number of firebrands needed to ignite the slabs were analyzed in order to determine the critical conditions of ignition. The ignition of the decking slab only occurred when the firebrands were positioned at the interstices of the wooden slabs and against the leg of the thermoplastic slabs. No ignition occurred when the firebrands were located on the surface of the decking slab. A minimum mass of firebrands of 0.31 g and 0.80 g was necessary to ignite the wooden slabs and the thermoplastic ones, respectively. Less firebrands were needed to ignite wooden slabs than thermoplastic ones. More work is required for European building features to better understand firebrand dangers in European communities.
As part of recent building code change discussions in the United States, it has been suggested that by increasing the spacing of decking boards, it may be possible to mitigate ignition of wood decking assemblies from wind-driven firebrand showers. An experimental series was undertaken to vary the board spacing from 0 mm (no gaps), 5 mm, and 10 mm, to determine if it was possible to observe reduced ignition propensity of full-scale wood decking assemblies fitted to a reentrant corner wall assembly (Manzello and Suzuki, 2019). In these experiments, three common wood types were used and firebrand showers were directed at the wall/decking assemblies using wind speeds of 8 m/s generated using a realistic-scale wind tunnel. Based on the results of these experiments, it was observed that board spacing significantly influenced ignition propensity of these assemblies. Ignition events were observed for all board spacing considered and in particular, more ignition points were observed for a board spacing of 10 mm. A similar approach was taken to study deck assembly performance using firebrand generators in the Insurance Institute for Business and Home Safety in the United States (Hedayati et al., 2022).
Full-scale experiments that have employed firebrand generators have been used to study vulnerabilities of homes to firebrand showers for some time (Manzello et al., 2020), including even more recent studies mentioned above. While it is interesting to conduct full-scale experiments, this is very expensive and not always practical, so efforts are underway to devise far cheaper reduced-scale experiments to provide more in-depth scientific understanding of firebrand shower ignition of construction components. In particular, fencing assemblies, roof assemblies, and mulch beds were also studied with a bench-scale firebrand generator, comparing the results with larger-scale experiments (Suzuki and Manzello 2019b; Suzuki and Manzello, 2022d). Developing a methodology with a bench-scale firebrand generator shows a path to provide standard test methods in the future. As part of this process, ISO is currently developing the ISO standard firebrand generator (ISO, 2022b).
One of the missing studies on ignition by firebrands is the combined effect of firebrands and radiant heat. This could become prominent in the case of short-range firebrand spotting. A new experimental setting was developed to investigate the combined effect of firebrand showers and radiant heat (Suzuki and Manzello, 2021a). Experiments were performed under 6 m/s and 8 m/s, with different pre-heating by radiative heat source. The research showed that radiant heat indeed has an effect of ignition by firebrands, especially under 6 m/s, shortening the time to ignition. It was found that the sum of the total heat received at the fuel beds and heat from firebrands is constant. Follow-up study with different size and mass of firebrands confirmed those findings (Suzuki and Manzello, 2022e).
The suppression of firebrand showers in perhaps the newest area of research in this the firebrand arena. In work at Clemson University in the United States, an analytical model was developed to calculate the probability of inter-particle collisions within two intersecting streams of particles, and was then applied to a range of test cases involving firebrands and water droplets (Green and Kaye, 2019). Results from this simplified analysis indicated that water sprays could effectively protect buildings from ‘firebrand attack’ in this manner, but only when either: i) large water flow rates were used (in the order of 1 L s−1 per meter of building perimeter to be protected), or ii) the sprays were comprised of very small (∼0.1 mm) droplets at moderate water flow rates (∼0.1 L s−1 m−1). It is likely that the quantity of water required to satisfy 1) would not be available in many circumstances, and further investigation is required to determine whether sprays of ∼0.1 mm droplets could operate effectively in the conditions of a wildfire. According to the authors, the analysis presented would be a suitable basis for further investigation into these spray systems, and for quantitative comparison with other types of wildfire sprinkler systems.
In work at Hong Kong Polytechnic University in China, firebrands were represented by a dry wood ball with a diameter of 20 mm and a weight of 2.9 g, which carried a flame with the heat release rate of 250 W (Xiong et al., 2021). The firebrand was held by a pendulum system to adjust the velocity. Results showed that there is a minimum sound pressure to extinguish the firebrand flame, which increases slightly with the sound frequency. As the firebrand velocity increases from 0 m/s to 4.2 m/s, the minimum sound pressure for extinction decreases significantly from 114 dB to 90 dB. The cumulative effect of firebrand motion and acoustic oscillation was found to facilitate flame extinction. A characteristic Damköhler number (∼1), with the ratio of the fuel residence time to the flame chemical time, is used to quantify the extinction limit of the flaming firebrand. According to the authors, this work provides a potential technical solution to mitigate the hazard of firebrand flame and spotting ignition in WUI and helps understand the influence of acoustic waves on the flame stability on the solid fuel.
There are many unresolved questions related to firebrand processes in large outdoor fires. Even in light of the interesting recent studies reviewed here, the needs listed in Manzello et al., 2018 and Manzello et al., 2020, still apply today.
Of these, a general, fundamental understanding is still required for firebrand generation from both the combustion of buildings, other human-made fuels, and vegetation. There are existing studies that may assist in future understanding even though the research is not focused on firebrands. In particular, some work related to structural changes, that is the cracking and shrinkage of wood under heat loading may be applicable to understand firebrand generation. Depending on wood species, thermal radiation, and ambient pressure, wood may crack and shrink differently (Li et al., 2021). During the combustion of wood-based materials found in buildings, the materials may go through drying, cracking, and shrinkage, and in the present of wind or buoyancy forces would break pieces from the virgin fuel, generating firebrands.
With the lack of fundamental understanding of firebrand generation, modelling of firebrand generation remains also a challenge. After work with vegetation by Barr and Ezekoye 2013, there is no real advance in modelling generation of firebrands. The current focus is limited to more source terms for modelling purpose. This is a somewhat dangerous approach as there is not enough data nor deep understanding of the phenomena itself. For firebrand generation from vegetation, it has been shown that generation process and generated firebrands under wind greatly differ from those under no wind.
The overall lack of understanding also leads to experiments which do not represent actual phenomena nor advance understanding of firebrand generation. Careful experiments and detailed observations are needed to understand physics of firebrand generation, which is currently limited.
The measurement of firebrand flux, or measurement of temperature/size of firebrands, has been a topic of interest for a long time. These measurements remain elusive from actual fires. Existing technology has been applied to measure this information so far, with very limited success, from prescribed fires. As part of future work, the existing technology should be refined to make more accurate measurements (for research purposes) or consider to replace these methods with simpler methods that may be applied in the field, during actual fires. Work was started to develop the concept of rapid response instrumentation to deploy in actual fires; these approached should be re-visited (Manzello et al., 2010).
Temperature measurements of the generated firebrands would be desirable as this is also a long, recent discussion on “hot” and “cold” firebrands. While it may be possible that firebrands may not be burning by the time they deposit on the ground, the question on the needs to understand why this even important remains unclear. As indicated, studies that have tried to quantify hot or cold firebrands are done under no controlled wind or during prescribed fires, which can never replicate actual fires, for safety reasons.
While firebrand transport process has been studied for decades, the deposition process is mostly unsolved. From a risk assessment point of view, it is important to understand where firebrands land and eventually accumulate, as this leads to ignition. Without properly incorporating the combustion of firebrands into modelling, transport and deposition, eventual accumulation of firebrands cannot be properly predicated. Once firebrands deposit, the friction force between surface and firebrands become another issue, and how a firebrand with localized wind may move on the surface needs to be considered.
Another great challenge is the development of a comprehensive ignition theory to better understand firebrand ignition processes. Some recent work has begun in this direction, but similar to the firebrand generation topic, there remains still limited experimental data on the basic firebrand ignition results.
As shown in Figures 7, 9, and Figure 10, many parameters affect firebrand generation, transport and ignition process. Wind plays clearly an important role, as many firebrands are observed in real fires with strong wind. Yet, other than wind, it remains unknown what other key parameters influence all of these processes.
An area of great caution is the recent trends in using machine learning techniques to better understand large outdoor fire processes (Jain et al., 2020). In particular, firebrand processes, there still remains too few data sets to readily apply these methods. A recent review shows that the number of publications in this area is exploding but the authors also indicate the methods are tenuous for many aspects of wildland fires, due to an overall lack of reliable data (Jain et al., 2020).
The review closes with is the greatest need of all: mechanical engineers are needed. Fire safety science still remains a relatively esoteric topic that has not been embraced by the engineering disciplines. Mechanical engineering is a broad discipline that has been traditionally divided into subdisciplines. In particular, the thermal sciences branch of mechanical engineering is the area that may best prepare students to partake in research in fire safety science and address the large outdoor fire problem. The thermal sciences are broadly focused on the fundamentals of heat and mass transfer, thermodynamics, fluid mechanics, and combustion.
In the case of the firebrand problem, it is clear that all these subjects are directly applicable. In particular, combustion science is an area that has been well-developed over extensive years of research. Now that the world appears to be moving away from internal combustion engines (Ainger and Krukowska, 2022; Rott, 2022), it is believed that fire safety science is a new area where traditional mechanical engineering principles, such as combustion science may be applied, to garner improved understanding of firebrand processes.
With the increasing risks from a changing climate, these large outdoor fire disasters are only going to become more and more commonplace all over the world. It is the authors opinion that a targeted, multi-disciplinary approach is needed to address the large outdoor fire problem. Looking at engineering departments across the globe, rarely if ever are large outdoor fire problems engaged by the engineering community. Part of the problem is that engineering, and especially mechanical engineering departments, do not focus on fire safety in general. As stated, the large outdoor fire problem is emerging and becoming more complicated, it is argued that this topic is a fascinating and challenging research area and that engineers have the necessary skills and training to impact a problem that influences millions upon millions of people all over the world. Firebrands were introduced for non-specialist readers and most recent literature is reviewed. Several challenges were discussed, in particular, areas where engineers may help move the needle forward on this globally important topic.
SM conceptualization, writing initial draft; SS conceptualization, writing initial draft.
The authors greatly appreciate the kind invitation to prepare this paper for the new series Horizons in Mechanical Engineering, as part of the journal Frontiers in Mechanical Engineering. The authors also thank David Blunck, Jean-Louis Consalvi, Michael Gollner, Faraz Heydayati, Xinyan Huang, Nigel Kaye, Brian Lattimer, Ya-Ting Liao, Khalid Moinuddin, Paul-Antoine Santoni, and Jason Sharples, for sharing their recent studies, so that these may be included in this review. The authors greatly appreciate the effort of two reviewers to improve the quality of the manuscript.
SM are employed by the company Reax Engineering Inc.
The remaining author declares that the research was conducted in the absence of any commercial or financial relationships that could be construed as a potential conflict of interest.
All claims expressed in this article are solely those of the authors and do not necessarily represent those of their affiliated organizations, or those of the publisher, the editors and the reviewers. Any product that may be evaluated in this article, or claim that may be made by its manufacturer, is not guaranteed or endorsed by the publisher.
Abatzoglou, J. T., and Williams, A. P. (2016). Impact of anthropogenic climate change on wildfire across western US forests. Proc. Natl. Acad. Sci. U. S. A. 113, 11770–11775. doi:10.1073/pnas.1607171113
Adusumilli, S., Chaplen, J. E., and Blunck, D. L. (2021). Firebrand generation rates at the source for trees and a shrub. Front. Mech. Eng. 7, 655593. doi:10.3389/fmech.2021.655593
Ainger, J., and Krukowska, E. (2022). EU gets landmark deal to phase out combustion engine by 2035, bloomberg, june 29, 2022, EU countries uphold cars emissions phaseout in end to combustion engine - bloomberg. Available at https://www.bloomberg.com/news/articles/2022-06-29/eu-countries-uphold-phaseout-of-emissions-from-new-cars-by-2035#:∼:text=European%20Union%20countries%20endorsed%20a,of%20the%20internal%20combustion%20engine (accessed October 6, 2022).
Almeida, M., Porto, L., and Viegas, D. X. (2021). Characterization of firebrands released from different burning tree species. Front. Mech. Eng. 7, 651135. doi:10.3389/fmech.2021.651135
Arup, (2018). The framework for fire safety in informal settlements. Available at: https://www.arup.com/perspectives/publications/research/section/a-framework-for-fire-safety-in-informal-settlements
Barr, B. W., and Ezekoye, O. (2013). Thermo-mechanical modeling of firebrand breakage on a fractal tree. Proc. Combust. Inst. 34, 2649–2656. doi:10.1016/j.proci.2012.07.066
Bean, D., and Blunck, D. L. (2021). Sensitivities of porous beds and plates to ignition by firebrands. Front. Mech. Eng. 7, 653810. doi:10.3389/fmech.2021.653810
Bearinger, E. D., Hodges, J. F., Yang, F., Rippe, C. M., and Lattimer, B. Y. (2020). Localized heat transfer from firebrands to surfaces. Fire Saf. J. 120, 103037. doi:10.1016/j.firesaf.2020.103037
Blanchi, R., Maranghides, A., and England, J. R. (2019). “Lessons learnt from post-fire surveys and investigations,” in Encyclopedia of wildfires and wildland-urban interface (WUI) fires. Editor S. Manzello (Cham: Springer). doi:10.1007/978-3-319-51727-8_46-1
CALFIRE (2018). Incident archive. Available at https://www.fire.ca.gov/incidents/2018/.
CALFIRE (2020). Incident archive. Available at https://www.fire.ca.gov/incidents/2020/.
CALFIRE (2021). Incident archive. Available at https://www.fire.ca.gov/incidents/2021/.
Caton-Kerr, S. E., Tohidi, A., and Gollner, M. J. (2019). Firebrand generation from thermally-degraded cylindrical wooden dowels. Front. Mech. Eng. 5. doi:10.3389/fmech.2019.00032
Cawson, J., Pickering, B. J., Filkov, A., Burton, J., Kilinc, M., and Penman, T. (2022). Predicting ignitability from firebrands in mature wet eucalypt forests. For. Ecol. Manag. 519, 120315. doi:10.1016/j.foreco.2022.120315
Deb, P., Moradkhani, H., Abbaszadeh, P., Kiem, A. S., Engström, J., Keellings, D., et al. (2020). Causes of the widespread 2019–2020 Australian bushfire season. Earth's. Future 8 (11), e2020EF001671. doi:10.1029/2020ef001671
Duff, T. J., Cawson, J. G., and Penman, T. D. (2018). “Prescribed burning,” in Encyclopedia of wildfires and wildland-urban interface (WUI) fires. Editor S. Manzello (Cham: Springer). doi:10.1007/978-3-319-51727-8_120-1
Fernandez-Pello, A. C. (2017). Wildland fire spot ignition by sparks and firebrands. Fire Saf. J. 91, 2–10. doi:10.1016/j.firesaf.2017.04.040
Ganteaume, A., Barbero, R., Jappiot, M., and Maillé, E. (2021). Understanding future changes to fires in southern Europe and their impacts on the wildland-urban interface. J. Saf. Sci. Resil. 2, 20–29. doi:10.1016/j.jnlssr.2021.01.001
Green, A., and Kaye, N. B. (2019). On the use of sprays to intercept airborne embers during wildfires. Fire Saf. J. 108, 102842. doi:10.1016/j.firesaf.2019.102842
Hajilou, M., Hu, S., Roche, T., Garg, P., and Gollner, M. J. (2021). A methodology for experimental quantification of firebrand generation from WUI fuels. Fire Technol. 57, 2367–2385. doi:10.1007/s10694-021-01119-9
Hedayati, F., Quarles, S. L., and Standohar-Alfano, C. (2022). Evaluating deck fire performance—limitations of the test methods currently used in California’s building codes. Fire 5 (4), 107. doi:10.3390/fire5040107
Hiers, J. K., O’Brien, J. J., Varner, J. M., Butler, B., Dickinson, M., Furman, J., et al. (2020). Prescribed fire science: The case for a refined research agenda. fire Ecol. 16, 11. doi:10.1186/s42408-020-0070-8
Hill, L., Jaeger, J., and Smith, A. (2022). Can prescribed fires mitigate health harm?. A Review of Air Quality and Public Health Implications of Wildfire and Prescribed Fire. Available at: https://www.lung.org/getmedia/fd7ff728-56d9-4b33-82eb-abd06f01bc3b/pse_wildfire-and-prescribed-fire-brief_final_2022
Himoto, K., and Iwami, T. (2021). Generalization framework for varying characteristics of the firebrand generation and transport from structural fire source. Fire Saf. J. 125, 103418. doi:10.1016/j.firesaf.2021.103418
Hudson, T. R., and Blunck, D. L. (2019). Effects of fuel characteristics on ember generation characteristics at branch-scales. Int. J. Wildland Fire 28, 941–950. doi:10.1071/wf19075
Hudson, T. R., Bray, R. B., Blunck, D. L., Page, W., and Butler, B. (2020). Effects of fuel morphology on ember generation characteristics at the tree scale. Int. J. Wildland Fire 29, 1042–1051. doi:10.1071/wf19182
ISO (2022b). Firebrand generator. Available at https://www.iso.org/standard/81912.html?browse=tc (accessed October 12, 2022).
ISO (2022a). Large outdoor fires and the built environment — global overview of different approaches to standardization. Available at https://www.iso.org/obp/ui/#iso:std:iso:tr:24188:ed-1:v1:en (accessed October 14, 2022).
Jain, P., Coogan, S., Subramanian, S., Crowley, M., Taylor, S., and Flannigan, M. (2020). A review of machine learning applications in wildfire science and management. Environ. Rev. 28, 478–505. doi:10.1139/er-2020-0019
Johnston, L., Blanchi, R., and Jappiot, M. (2019). “Wildland-urban interface,” in Encyclopedia of wildfires and wildland-urban interface (WUI) fires. Editor S. Manzello (Cham: Springer). doi:10.1007/978-3-319-51727-8_130-1
Kahanji, C., Walls, R. S., and Cicione, A. (2019). Fire spread analysis for the 2017 Imizamo Yethu informal settlement conflagration in South Africa. Int. J. Disaster Risk Reduct. 39, 101146. doi:10.1016/j.ijdrr.2019.101146
Koo, E., Pagni, P. J., Weise, D. R., and Woycheese, J. P. (2010). Firebrands and spotting ignition in large-scale fires. Int. J. Wildland Fire 19, 818–843. doi:10.1071/wf07119
Kwon, B., and Liao, Y. T. T. (2022). Effects of spacing on flaming and smoldering firebrands in wildland-urban interface fires. J. Fire Sci. 40, 155–174. doi:10.1177/07349041221081998
Lattimer, B. Y., Bearinger, E., Wong, S., and Hodges, J. L. (2022). Evaluation of models and important parameters for firebrand burning. Combust. Flame 235, 111619. doi:10.1016/j.combustflame.2021.111619
Lee, S.-J., and Lee, Y.-W. (2020). Detection of wildfire-damaged areas using kompsat-3 image: A case of the 2019 unbong mountain fire in busan, South Korea. Korean J. Remote Sens. 36, 29–39.
Li, K., Zhou, Y., Bourbigot, S., Ji, J., and Chen, X. (2021). Pressure effects on morphology of isotropic char layer, shrinkage, cracking and reduced heat transfer of wooden material. Proc. Combust. Inst. 38 (3), 5063–5071. doi:10.1016/j.proci.2020.07.072
Mankame, A., and Shotorban, B. (2021). Deposition characteristics of firebrands on and around rectangular cubic structures. Front. Mech. Eng. 7, 640979. doi:10.3389/fmech.2021.640979
Manzello, S. L., Almand, K., Guillaume, E., Vallerent, S., Hameury, S., and Hakkarainen, T. (2018). FORUM position paper, the growing global wildland urban interface (WUI) fire dilemma: Priority needs for research. Fire Saf. J. 100, 64–66. doi:10.1016/j.firesaf.2018.07.003
Manzello, S. L. (2014). Enabling the investigation of structure vulnerabilities to wind- driven firebrand showers in wildland-urban interface (WUI) fires. Fire Saf. Sci. 11, 83–96. doi:10.3801/iafss.fss.11-83
Manzello, S. L., and Foote, E. I. D. (2014). Characterizing firebrand exposure from wildland-urban interface (WUI) fires: Results from the 2007 angora fire. Fire Technol. 50, 105–124. doi:10.1007/s10694-012-0295-4
Manzello, S. L., Maranghides, A., and Mell, W. E. (2007). Firebrand generation from burning vegetation. Int. J. Wildland Fire 16, 458–462. doi:10.1071/wf06079
Manzello, S. L., Park, S., and Cleary, T. G. (2010). Development of rapidly deployable instrumentation packages for data acquisition in wildland-urban interface (WUI) fires. Fire Saf. J. 45, 327–336. doi:10.1016/j.firesaf.2010.06.005
Manzello, S. L., Park, S., and Cleary, T. G. (2009). Investigation on the ability of glowing firebrands deposited within crevices to ignite common building materials. Fire Saf. J. 44, 894–900. doi:10.1016/j.firesaf.2009.05.001
Manzello, S. L., Shields, J. R., Cleary, T. G., Maranghides, A., Mell, W. E., Yang, J. C., et al. (2008). On the development and characterization of a firebrand generator. Fire Saf. J. 43, 258–268. doi:10.1016/j.firesaf.2007.10.001
Manzello, S. L., Suzuki, S., Gollner, M., and Fernandez-Pello, A. C. (2020). Role of firebrand combustion in large outdoor fire spread. Prog. Energy Combust. Sci. 76, 100801. doi:10.1016/j.pecs.2019.100801
Manzello, S. L., and Suzuki, S. (2019). Influence of board spacing on mitigating wood decking assembly ignition. Fire Saf. J. 110, 102913. doi:10.1016/j.firesaf.2019.102913
Manzello, S. L., and Suzuki, S. (2022). The importance of combustion science to unravel complex processes for informal settlement fires, urban fires, and wildland-urban interface (WUI) fires. Fuel 314, 122805. doi:10.1016/j.fuel.2021.122805
Marom, I., and Toledo, T. (2021). Activities and social interactions during disaster evacuation. Int. J. Disaster Risk Reduct. 61, 102370. doi:10.1016/j.ijdrr.2021.102370
Matvienko, O., Kasymov, D., Loboda, E., Lutsenko, A., and Daneyko, O. (2022). Modeling of wood surface ignition by wildland firebrands. Fire 5 (2), 38. doi:10.3390/fire5020038
Meerpoel-Pietri, K., Tihay-Felicelli, V., and Santoni, P. A. (2021). Determination of the critical conditions leading to the ignition of decking slabs by flaming firebrands. Fire Saf. J. 120, 103017. doi:10.1016/j.firesaf.2020.103017
Mendez, A., and Farazmand, M. (2022). Quantifying rare events in spotting: How far do wildfires spread? Fire Saf. J. 132, 103630. doi:10.1016/j.firesaf.2022.103630
Nguyen, D., and Kaye, N. B. (2021). Experimental investigation of rooftop hotspots during wildfire ember storms. Fire Saf. J. 125, 103445. doi:10.1016/j.firesaf.2021.103445
Nguyen, D., and Kaye, N. B. (2022a). Quantification of ember accumulation on the rooftops of isolated buildings in an ember storm. Fire Saf. J. 128, 103525. doi:10.1016/j.firesaf.2022.103525
Nguyen, D., and Kaye, N. B. (2022b). The role of surrounding buildings on the accumulation of embers on rooftops during an ember storm. Fire Saf. J. 131, 103624. doi:10.1016/j.firesaf.2022.103624
Novozhilov, V. (2001). Computational fluid dynamics modeling of compartment fires. Prog. Energy Combust. Sci. 27 (6), 611–666. doi:10.1016/s0360-1285(01)00005-3
Palinkas, L. A. (2020). “The California wildfires,” in Global climate change, population displacement, and public health (Cham: Springer).
Petersen, P. B. (2010). Crisis during recovery: Lessons from 1904 Baltimore fire. J. Manag. Hist. 16, 297–311. doi:10.1108/17511341011051216
Plathner, F., Sjostrom, J., and Granstrom, A. (2023). Garden structure is critical for building survival in northern forest fires – an analysis using large Swedish wildfires. Saf. Sci. 157, 105928. doi:10.1016/j.ssci.2022.105928
Prohanov, S., Filkov, A., Kasymov, D., Agafontsev, M., and Reyno, V. (2020). Determination of firebrand characteristics using thermal videos. Fire 3 (4), 68. doi:10.3390/fire3040068
Reisen, F., Duran, S. M., Flannigan, M., Elliott, C., and Rideout, K. (2015). Wildfire smoke and public health risk. Int. J. Wildland Fire 24, 1029–1044. doi:10.1071/wf15034
Richter, F., Bathras, B., Barbetta Duarte, J., and Gollner, M. J. (2022). The propensity of wooden crevices to smoldering ignition by firebrands. Fire Technol. 58, 2167–2188. doi:10.1007/s10694-022-01247-w
Rivera, J., Hernández, N., Consalvi., J. L., Reszka, P., Contreras, J., and Fuentes, A. (2020). Ignition of wildland fuels by idealized firebrands. Fire Saf. J. 120, 103036. doi:10.1016/j.firesaf.2020.103036
Rott, N. (2022). Largest U.S. Auto market is moving away from the internal combustion engine. Natl. Public Radio. Available at: https://www.npr.org/2022/08/25/1119291043/largest-u-s-auto-market-is-moving-away-from-the-internal-combustion-engine (Accessed October 6, 2022)
Salinas-Silva, V. (2015). The ‘GREAT FIRE’ of valparaiso 2014: Social class differences and people’ s vulnerability. A case study of wildland-urban fire. London, UK: UCL Hazard Centre Disaster Studies and Management Working.
Storey, M. A., Price, O. F., Bradstock, R. A., and Sharples, J. J. (2020a). Analysis of variation in distance, number, and distribution of spotting in Southeast Australian wildfires. Fire 3 (2), 10. doi:10.3390/fire3020010
Storey, M. A., Price, O. F., Sharples, J. J., and Bradstock, R. A. (2020b). Drivers of long-distance spotting during wildfires in south-eastern Australia. Int. J. Wildland Fire 29 (6), 459–472. doi:10.1071/wf19124
Suzuki, S., and Manzello, S. L. (2021c). Investigating the effect of structure to structure separation distance on firebrand accumulation. Front. Mech. Eng. 6, 628510. doi:10.3389/fmech.2020.628510
Suzuki, S. (2017b). Study on characteristics of firebrands collected from three different fires. NRIFD technical report 123 Tokyo, Japan: National Research Institute of Fire and Disaster, 1–9. doi:10.1016/j.ygcen.2017.02.011
Suzuki, S. (2017a). A review on structure ignitions by firebrands. Bull. Jpn. Assoc. Fire Sci. Eng. 67 (1), 49–55.
Suzuki, S., and Manzello, S. L. (2019a). Investigating effect of wind speeds on structural firebrand generation in laboratory scale experiments. Int. J. Heat. Mass Transf. 130, 135–140. doi:10.1016/j.ijheatmasstransfer.2018.10.045
Suzuki, S., and Manzello, S. L. (2019b). Understanding structure ignition vulnerabilities using mock-up sections of attached wood fencing assemblies. Fire Mater. 43, 675–684. doi:10.1002/fam.2716
Suzuki, S., and Manzello, S. L. (2018). Characteristics of firebrands collected from actual urban fires. Fire Technol. 54, 1533–1546. doi:10.1007/s10694-018-0751-x
Suzuki, S., and Manzello, S. L. (2022b). Comparing particulate morphology generated from human-made cellulosic fuels to natural vegetative fuels. Int. J. Wildland Fire. doi:10.1071/WF22093
Suzuki, S., and Manzello, S. L. (2022a). Firebrands generated in shurijo castle fire on october 30th, 2019. Fire Technol. 58, 777–791. doi:10.1007/s10694-021-01176-0
Suzuki, S., and Manzello, S. L. (2020a). Garnering understanding into complex firebrand generation processes from large outdoor fires using simplistic laboratory-scale experimental methodologies. Fuel 267, 117154. doi:10.1016/j.fuel.2020.117154
Suzuki, S., and Manzello, S. L. (2022c). Investigating conifer tree combustion in the presence of an applied wind field. Combust. Sci. Technol., 1–18. doi:10.1080/00102202.2022.2112034
Suzuki, S., and Manzello, S. L. (2021a). Investigating coupled effect of radiative heat flux and firebrand showers on ignition of fuel beds. Fire Technol. 57, 683–697. doi:10.1007/s10694-020-01018-5
Suzuki, S., and Manzello, S. L. (2011). On the development and characterization of a reduced scale continuous feed firebrand generator. Fire Saf. Sci. -. Proc. 10th. Int. Symposium. 10, 1437–1448. doi:10.3801/iafss.fss.10-1437
Suzuki, S., and Manzello, S. L. (2022e). On unraveling community ignition processes: Joint influences of firebrand showers and radiant heat applied to fuel beds. Combust. Sci. Technol., 1–14. doi:10.1080/00102202.2021.2019238
Suzuki, S., and Manzello, S. L. (2020b). Role of accumulation for ignition of fuel beds by firebrands. Appl. Energy Combust. Sci. 1-4, 100002. doi:10.1016/j.jaecs.2020.100002
Suzuki, S., and Manzello, S. L. (2022d). Toward understanding ignition vulnerabilities to firebrand showers using reduced-scale experiments. Fire Mater. 46 (5), 809–817. doi:10.1002/fam.3027
Suzuki, S., and Manzello, S. L. (2021b). Towards understanding the effect of cedar roof covering application on firebrand production in large outdoor fires. J. Clean. Prod. 278, 123243. doi:10.1016/j.jclepro.2020.123243
Suzuki, S. (2019). “Structure firebrand attack,” in Encyclopedia of wildfires and wildland-urban interface (WUI) fires. Editor S. Manzello (Cham: Springer). doi:10.1007/978-3-319-51727-8_5-2
Teague, B., McLeod, R., and Pascoe, S. (2010). 2009 victorian bushfires royal commission final report: Summary. Available at: http://www.royalcommission.vic.gov.au/finaldocuments/summary/HR/VBRC_Summary_HR.pdf.
Thomas, C. M., Sharples, J. J., and Evans, J. P. (2020). The terminal-velocity assumption in simulations of long-range ember transport. Math. Comput. Simul. 175, 96–107. doi:10.1016/j.matcom.2019.08.008
Thomas, J. C., Mueller, E. V., Gallagher, M. R., Clark, K., Skowronski, N., Simeoni, A., et al. (2021). Coupled assessment of fire behavior and firebrand dynamics. Front. Mech. Eng. 7, 650580. doi:10.3389/fmech.2021.650580
Thompson, D. K., Yip, D. A., Koo, E., Linn, R., Marshall, G., Refai, R., et al. (2022). Quantifying firebrand production and transport using the acoustic analysis of in-fire cameras. Fire Technol. 58, 1617–1638. doi:10.1007/s10694-021-01194-y
Wadhwani, R., Sutherland, D., Ooi, A., and Moinuddin, K. (2022). Firebrand transport from a novel firebrand generator: Numerical simulation of laboratory experiments. Int. J. Wildland Fire 31, 634–648. doi:10.1071/wf21088
Wang, S., Ding, P., Lin, S., Gong, J., and Huang, X. (2021b). Smoldering and flaming of disc wood particles under external radiation: Auto-ignition and size effect. Front. Mech. Eng. 7, 686638. doi:10.3389/fmech.2021.686638
Wang, S., Ding, P., Lin, S., Huang, X., and Usmani, A. (2021a). Deformation of wood slice in fire: Interactions between heterogeneous chemistry and thermomechanical stress. Proc. Combust. Inst. 38, 5081–5090. doi:10.1016/j.proci.2020.08.060
Weisses, S., and Ezekoye, D. K. (2022). A framework for determining the ignition signatures in a fuel bed due to firebrand deposition. Fire Technol. doi:10.1007/s10694-022-01316-0
Wickramasinghe, A., Khan, N., and Moinuddin, K. (2022). Determining firebrand generation rate using physics-based modelling from experimental studies through inverse analysis. Fire 5 (1), 6. doi:10.3390/fire5010006
Wong, S., Broader, J., and Shaheen, S. (2020). Review of California wildfire evacuations from 2017 to 2019. California: University of California Institute of Transportation Studies.
Xiong, C., Liu, Y., Xu, C., and Huang, X. (2021). Acoustical extinction of flame on moving firebrand for the fire protection in wildland–urban interface. Fire Technol. 57, 1365–1380. doi:10.1007/s10694-020-01059-w
Yang, G., Ning, J., Shu, L., Zhang, J., Yu, H., and Di, X. (2022). Spotting ignition of larch (Larix gmelinii) fuel bed by different firebrands. J. For. Res. 33, 171–181. doi:10.1007/s11676-020-01282-9
Keywords: climate, large outdoor fires, firebrands, mechanical engineering, combustion
Citation: Manzello SL and Suzuki S (2023) The world is burning: What exactly are firebrands and why should anyone care?. Front. Mech. Eng 8:1072214. doi: 10.3389/fmech.2022.1072214
Received: 17 October 2022; Accepted: 03 November 2022;
Published: 04 January 2023.
Edited by:
XJ Jing, City University of Hong Kong, Hong Kong SAR, ChinaReviewed by:
Xinyan Huang, Hong Kong Polytechnic University, Hong Kong SAR, ChinaCopyright © 2023 Manzello and Suzuki. This is an open-access article distributed under the terms of the Creative Commons Attribution License (CC BY). The use, distribution or reproduction in other forums is permitted, provided the original author(s) and the copyright owner(s) are credited and that the original publication in this journal is cited, in accordance with accepted academic practice. No use, distribution or reproduction is permitted which does not comply with these terms.
*Correspondence: Samuel L. Manzello, bWFuemVsbG9AcmVheGVuZ2luZWVyaW5nLmNvbQ==
Disclaimer: All claims expressed in this article are solely those of the authors and do not necessarily represent those of their affiliated organizations, or those of the publisher, the editors and the reviewers. Any product that may be evaluated in this article or claim that may be made by its manufacturer is not guaranteed or endorsed by the publisher.
Research integrity at Frontiers
Learn more about the work of our research integrity team to safeguard the quality of each article we publish.