- 1Department of Engineering, University of Cambridge, Cambridge, United Kingdom
- 2Aero-Thermo-Mechanics Department, Université Libre de Bruxelles, Brussels, Belgium
Emissions of nitrogen oxides (NOx) from combustion systems remain a lingering environmental issue, being these species either greenhouse gases or acid rain precursors. Moderate or Intense Low-oxygen Dilution (MILD) combustion can reduce the emissions of NOx thanks to its characteristic features (i.e., homogeneous reaction zones, reduced temperature peaks, diluted mixtures of reactants) that influence and change the main chemical pathways of NOx formation. A summary of the relevant routes of formation and destruction of NOx in MILD combustion is presented in this review, along with the identification of the sources of uncertainty that prevent reaching an overall consensus in the literature about the dominant NOx chemical pathway in MILD regime. Computational Fluid Dynamics (CFD) approaches are essential tools for investigating the critical phenomena occurring in MILD combustion and the design of pollutant-free turbulent combustion systems. This paper provides an outline of the modeling approaches employed in CFD simulations of turbulent combustion systems to predict NOx emissions in MILD conditions. An assessment of the performances of selected models in estimating NOx formation in a lab-scale MILD combustion burner is then presented, followed by a discussion about relevant modeling issues, perspectives and opportunities for future research.
1. Introduction
Moderate and Intense Low-Oxygen Dilution (MILD) combustion (Cavaliere and de Joannon, 2004) technologies have become of vigorous industrial interest since they are able to reduce the environmental concerns linked to the use of both conventional and alternative fuels. MILD combustion, otherwise indicated as Flameless (Wünning and Wünning, 1997) or High-Temperature Air Combustion (HiTAC) (Gupta, 2004), can limit the emissions of pollutants like carbon monoxide (CO), nitrogen oxides (NOx) and soot (Dally et al., 2004; Li et al., 2006; Szegö et al., 2008) and provide elevated combustion efficiency and fuel flexibility (Sabia et al., 2007; Medwell and Dally, 2012). The abatement of pollutants is achieved through a strong recirculation of exhaust gases in the reaction zone: the fresh gases are hence diluted and heated up above their self-ignition temperature, and the combustion process is homogeneously distributed across a broad domain rather than confined to a flame front (de Joannon et al., 2012). For industrial processes whose primary aspects are energy efficiency and homogeneous distribution of high temperatures inside the combustion chamber (e.g., steel, glass, and ceramic industry), MILD combustion is a suitable and favorable technology.
MILD combustion can significantly reduce NOx formation via diminished temperature peaks, which inhibit the NOx thermal route (Zeldovich, 1946). However, considerable NOx emissions can still be observed (Mancini et al., 2002; Parente et al., 2011; Li et al., 2013). Other NOx formation routes, namely prompt (Fenimore, 1971), N2O-intermediate (Malte and Pratt, 1975), and NNH-intermediate (Bozzelli and Dean, 1995), are held accountable for the not negligible emissions of NOx in MILD regime. This review provides a summary of the current knowledge about the NOx formation routes in homogeneous combustion systems working in MILD conditions.
The overall mechanism leading to NOx formation is one of the peculiar features that make MILD combustion substantially different from conventional combustion processes. Computational Fluid Dynamics (CFD) approaches have been successfully used to investigate these features and gain insights that are aiding the design of pollutant-free MILD combustion systems. Correct predictions of NOx emissions require an accurate description of the temperature field, along with main and radical species concentrations. Hence, reliable numerical models of the intertwined phenomena occurring in combustion, namely turbulence, chemistry, and heat transfer, are necessary. An overview of the different modeling approaches for NOx formation in CFD simulations of MILD combustion systems is presented, along with a discussion about their potential and limitations.
2. Chemistry of NOx Formation in MILD Combustion
The routes leading to the formation of NOx in combustion systems mostly involve the insertion of radicals such as O, CHx, and H, into the triple bond of molecular nitrogen present in combustion air (Miller and Bowman, 1989). This mechanism forms reactive nitrogen intermediates (cyanides, N2O, NNH, etc.) that are subsequently oxidized to produce NOx. Alternatively, NOx are produced through oxidation reactions of organic nitrogen chemically bound in the fuel. The thermal route and fuel-N route, when nitrogen is present in the fuel, are the relevant pathways of NOx formation in conventional combustion regimes, whereas in non-conventional regimes, such as MILD, the prompt, the N2O-intermediate, and the NNH-intermediate pathways must be considered. A graphical overview of the above-mentioned pathways leading to the formation of NO from molecular nitrogen is presented in Figure 1.
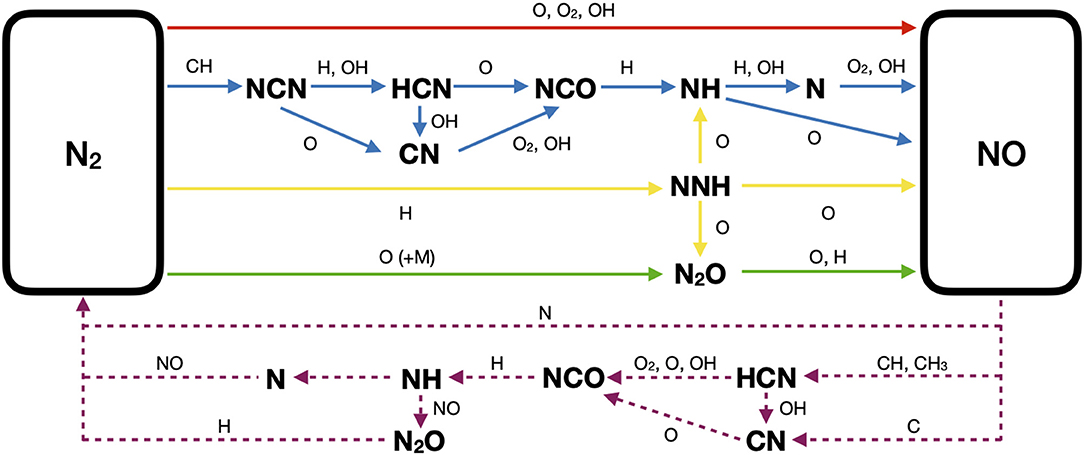
Figure 1. Simplified scheme of formation and destruction pathways of the NO species: thermal (red solid arrow), prompt (blue solid arrows), N2O-intermediate (green solid arrows), NNH-intermediate (yellow solid arrows), and reburning (purple dashed arrows).
The kinetic mechanism of the thermal route is quite well established in the literature (Glarborg et al., 2018). Thermal NOx emissions are prevalent in lean systems characterized by temperatures above 1800 K and high residence times. The prompt pathway has been revised in fairly recent studies that identified the formation of the NCN species as the most important initial step of the prompt route (Cui et al., 1999; Moskaleva and Lin, 2000). The kinetic parameters of the reaction of NCN formation, CH + N2⇌NCN + H, have been established within a factor of two by several experimental and modeling investigations (Vasudevan et al., 2007; Harding et al., 2008; Sutton et al., 2012; Klippenstein et al., 2018) and included in novel mechanisms (Lamoureux et al., 2016; Glarborg et al., 2018; Song et al., 2019). Since the CH species is produced by the oxidation of hydrocarbons and forms the NCN species by insertion into the triple bond of molecular nitrogen, the predictions of NOx emissions via the prompt route is also affected by the accuracy of the kinetic mechanisms of hydrocarbon oxidation (Santner et al., 2016). The prompt route is typical of fuel-rich systems and is dominant at temperatures below 1800 K. Li et al. (2014) showed that the prompt mechanism is the main source of NO in fuel-rich conditions in a laboratory-scale MILD combustion furnace. Prompt was also found to be the prevalent pathway in a numerical/experimental study in laminar co-flow diffusion flames on their transition to MILD combustion regime (Sepman et al., 2013). Preheating, dilution, and recirculation of burned gases are crucial for establishing MILD combustion and seem to favor the prompt mechanism over the other routes while reducing the overall emissions of NOx. Lee and Choi (2009) reported that the prompt route is predominant in high-temperature diluted-air combustion at low oxygen concentration in a coaxial jet flame. A numerical study (Abtahizadeh et al., 2012) investigating the effects of dilution and internal recirculation of burned gases on NOx formation also revealed that the prompt mechanism is the dominant reaction pathway. In the same study, it was further shown that the NO emissions are decreased by increasing the dilution ratio, as confirmed by other investigations performed in a lab-scale MILD combustion furnace (Szegö et al., 2008; Li et al., 2013).
The N2O-intermediate pathway plays an essential role in fuel-lean systems with temperatures below 1800 K and high pressures, and its mechanism is fairly well established (Glarborg et al., 2018). The NNH pathway is relevant when hydrogen-enriched fuel mixtures are oxidized, since H2 reacts with N2 to form the NNH intermediate species. The kinetics of this route is still under investigation: the uncertainty in the heat of formation of NNH may alter the NO predictions by a factor of two (Klippenstein et al., 2011), and the rate constants for the limiting reaction NNH + O ⇌ NH + NO reported in the literature (Bozzelli and Dean, 1995; Hayhurst and Hutchinson, 1998; Konnov et al., 2001; Haworth et al., 2003; Klippenstein et al., 2011) vary by more than an order of magnitude. However, recent kinetic models (Zhang et al., 2017; Glarborg et al., 2018; Song et al., 2019) have been updated with thermo-chemical and kinetic quantities estimated via ab initio transition state calculations (Klippenstein et al., 2011). The inclusion of the N2O and NNH routes has been crucial in obtaining accurate predictions of NO emissions for several lab-scale burners (Galletti et al., 2009; Mardani and Tabejamaat, 2012; Wang et al., 2015) and semi-industrial furnaces (Parente et al., 2011; Li et al., 2013) operating in MILD conditions. Li et al. (2014) showed that the N2O route dominates NO formation in lean conditions (equivalence ratio ϕ < 0.8) in a lab-scale MILD furnace, whereas the NNH pathway is predominant in the presence of H2-enriched mixtures, in accordance with other studies (Skottene and Rian, 2007; Parente et al., 2011; Wang et al., 2015). Although Cho and Chung (2009) showed that N2O-intermediate is negligible at lean conditions (ϕ < 0.8) in CH4/air counterflow premixed flames, Guo et al. (2005) found that the N2O and NNH routes are the main responsible of NO formation for ultra-lean (ϕ < 0.7) counterflow CH4-H2/air premixed flames. It was shown that the addition of hydrogen increased the emission of NO due to the enhancement in the rate of the NNH and N2O routes.
A reduced formation of NOx in MILD combustion is also attributed to the increasing relevance of the reburning route with respect to conventional combustion (Wünning and Wünning, 1997; Nicolle and Dagaut, 2006). In reburning, NOx are removed from the combustion products by reactions with hydrocarbon radicals. Reburning was found to be favored by the decrease in oxygen content and temperature of the coflow in the Adelaide Jet in Hot Coflow (JHC) flame (Wang et al., 2015), a jet that emulates MILD conditions via the injection of a heated and vitiated coflow (Dally et al., 2002). However, uncertainty still persists regarding the reactions that should be included in the reburning route to achieve accurate NOx predictions (Perpignan et al., 2018a). A simplified scheme of the reburning pathway is included in Figure 1.
As illustrated in this section, a significant number of studies have investigated the relative impact of the different pathways of NOx formation in MILD regime. Although qualitative formation trends can be identified, no agreement has been reached regarding an overall dominant source of NOx in MILD combustion. The influence of the burner operating conditions, such as temperature, composition of the reactants, and dilution levels, on the contribution of a certain route to the NOx emissions remains considerable. Moreover, uncertainty remains in the kinetics of prompt and NNH routes and in the methods of isolating the contribution of a single route with respect to the others, given their interdependence. Further research is desirable to quantify and reduce these sources of uncertainty.
3. CFD Modeling of NOx Emissions in MILD Conditions
In conventional combustion, it is recognized that the flame structure is dominated by the fast fuel-oxidizer reactions, other than the nitrogen pollutant chemistry. Thus, NOx formation models are often decoupled from the CFD main calculation and executed after the flame structure has developed. Poor effect of NOx chemistry on the overall temperature and flow fields have prompted the development of post-processing tools of CFD results. Most of these tools take the flow and temperature fields from the CFD simulation and carry out a calculation on a Chemical Reactor Network (CRN) built from the CFD grid using criteria based on the similarities of local temperature and species concentration.
The simplest post-processing tool considers a limited number of transport equations for pollutant species, such as NO, and intermediates, such as N2O, and global reacting steps for each NOx formation pathway. Equilibrium or quasi-steady-state assumptions are taken for the radical and intermediate species that are not transported but are involved in the NOx chemistry. The Arrhenius equation for the kinetic rates is integrated over a beta Probability Density Function (β-PDF) for the temperature (Peters, 2001), to account for the effect of temperature fluctuations on the mean reaction rates. This cost-effective approach has been used in several MILD combustion studies to model NOx emissions (Mancini et al., 2002; Galletti et al., 2009; Parente et al., 2011; Li et al., 2013).
A more sophisticated method consists in using rate of production analysis (ROPA) and sensitivity analysis to recognize the main reaction paths and develop reduced multi-step kinetic mechanisms of NOx formation. Two reduced-order mechanisms were developed specifically for MILD combustion (Galletti et al., 2015) starting from two detailed schemes (Ranzi et al., 1994; Glarborg et al., 1998). The most important reactions were identified via sensitivity analysis performed on a Perfectly Stirred Reactor (PSR) at wide ranges of temperatures and residence times typical of MILD combustion conditions. The quasi-steady-state assumption was made for some of the species involved in those reactions and a reduced expression for the NO formation rate including thermal, N2O, and NNH routes was obtained. The prompt NO route was accounted for via a global reaction rate (De Soete, 1975). The chemical source term in the post-processing transport equation for NO was obtained from the reduced mechanisms, and the predictions of NO showed adequate agreement with the experimental data of the Adelaide JHC flame (Dally et al., 2002). Hence, the reduced mechanisms performed reasonably well for the conditions on which they were tailored. However, errors may be introduced when extrapolating to different conditions than those used for fitting: as for the simplest post-processing tool mentioned above, the mechanism reduction relies on the assumptions of partial equilibrium and quasi-steady state that can fail under certain conditions and affect the predictivity of the reduced chemical schemes.
A CRN approach that employs a detailed mechanism with relatively low computational costs was presented by Frassoldati et al. (Frassoldati et al., 2010): the network was built on the CFD grid and a detailed kinetic mechanism was adopted to estimate the NOx emissions, whereas a simple chemical scheme was employed for the CFD simulation itself. The numerical algorithm of the CRN approach was refined (Cuoci et al., 2013) to allow the resolution of networks with a significant number of PSRs (~105), which are required in the presence of complex fluid dynamics. The formation of NOx was quite successfully modeled in laboratory flames (Bergmann et al., 1998; Barlow et al., 2000) and in a small-scale MILD combustor (Veŕıssimo et al., 2011). The joint CFD-CRN method was also employed to predict NOx emissions in a interturbine flameless combustor for gas turbines: the flamelet generate manifolds (FGM) method was used to model the combustion process in the CFD simulation, whereas a detailed mechanism was considered in the resolution of the network of PSRs (Perpignan et al., 2018b). Although the CRN approach can be used to evaluate the variation of NOx emissions under different operating conditions in a cost-effective way, the reliability of the CRN predictions is strongly dependent on the accuracy of the underlying CFD simulation. Moreover, network parameters such as number and type of reactors, mass flow rates and residence times, have to be tuned on the combustion conditions and a general effective method for the optimization of these parameters has not been validated yet.
Detailed kinetic mechanisms that include both hydrocarbons oxidation and NOx chemistry are necessary to improve predictivity and comprehensiveness of CFD simulations of turbulent MILD combustion systems. They include mutual NO-hydrocarbons interactions that are incorporated in the NOx chemistry, especially in prompt and reburning routes. The effects of turbulence-related fluctuations on the mean reaction rates become more prominent when detailed kinetic mechanisms are considered for NOx formation. Turbulent combustion models have been developed over the years following two main assumptions: infinitely fast chemistry (compared to the scale of turbulence) and finite-rate chemistry. The recognizing feature of MILD combustion is relatively low Damköhler numbers (Parente et al., 2008). Hence, models that account for the effects of turbulence interactions on finite-rate chemical reactions should be considered (Minamoto and Swaminathan, 2015). The Eddy Dissipation Concept (EDC) (Granm and Magnussen, 1996) is a finite-rate chemistry combustion model and has found wide application for the simulation of MILD combustion systems thanks to affordable, although not negligible, computational costs when compared to more sophisticated models such as the Transported Probability Density Function (TPDF) methods. Several numerical investigations (Mardani and Tabejamaat, 2012; Gao et al., 2013; Wang et al., 2015; Jiang et al., 2018; Shu et al., 2018) have analyzed the relative importance of NOx formation routes in the Adelaide JHC burner using EDC with detailed kinetic mechanisms, showing that an overall good agreement can be reached. Chomiak (1990) proposed the Partially Stirred Reactor (PaSR) model to represent a PSR with imperfect mixing. PaSR and EDC are conceptually similar since they both model combustion as a sequence of reaction and mixing steps in locally uniform regions. In EDC, the features of these regions are defined by two parameters, Cτ and Cγ, which are either supposed constant or calculated based on local turbulence parameters, as proposed in recent studies (Parente et al., 2016; Evans et al., 2019). In PaSR, a reacting region volume fraction κ is defined based on local estimations of chemical and mixing time scales. Better results in terms of predicted temperatures and main species concentrations in the Adelaide JHC burner have been achieved by both Reynolds Averaged Navier Stokes (RANS) and Large Eddy Simulation (LES) studies employing the PaSR model (Li et al., 2018; Ferrarotti et al., 2019; Iavarone et al., 2019) with respect to EDC. However, estimating the formation of NOx may require the resolution of chemical source terms with different characteristic time scales, since the reactions forming NO occur in a wider range of time scales compared to those of the main combustion process. Thus, a PaSR formulation that relates the source terms of the NOx species to their formation time scales, resulting in different values of κ for the NOx species, was presented (Iavarone et al., 2019).
Figure 2 provides a comparison of the capabilities of some of the CFD modeling approaches mentioned above to replicate the emission of NOx measured in the Adelaide JHC burner. The burner has been the target of numerous numerical studies in the context of NOx formation modeling. It consists of an insulated and cooled central fuel jet supplying an equimolar mixture of CH4 and H2. The fuel jet is surrounded coaxially by an annulus containing the hot co-flow flue gases produced by a secondary burner mounted upstream. The hot combustion products are further mixed with air and nitrogen to control the oxygen levels. The burner is placed inside a tunnel. Details of the geometry can be found in Dally et al. (2002). Figure 2 reports the radial profiles of mass fraction of the NO species measured at the axial locations z = 60/120/200 mm for the experimental case HM1 (Dally et al., 2002). Case HM1 is characterized by 3% oxygen content (as mass fraction) in the co-flow, inlet temperature of the co-flow Tcof = 1300 K, and fuel-jet Reynolds number Re = 10k. Measurements of NO were taken via the single-point Raman-Rayleigh-laser-induced fluorescence technique. The selected numerical results are provided by RANS studies that differs from one another in terms of employed chemical mechanism [either KEE, (Bilger et al., 1990) or GRI2.11, (Bowman et al., 1996)] and combustion model (either EDC or PaSR). The use of the KEE mechanism implies the use of a post-processing tool for the estimation of NO, whereas in the GRI2.11 scheme the NOx chemistry is included. Models M1 and M2 make use of the EDC model and the KEE mechanism. Model M1 employs global schemes for thermal, prompt, N2O and NNH formation routes. In contrast, model M2 considers the same global scheme for prompt formation as model M1 and multi-step mechanisms for the other routes. These mechanisms were reduced by ROPA for the Adelaide JHC flame conditions based on the POLIMI kinetic scheme (Galletti et al., 2009). Models M3 and M4 consider EDC with different values for the Cτ constant, i.e., Cτ = 0.82 (Shu et al., 2018) and Cτ = 1.5, respectively, and the GRI2.11 mechanism. Models M5 and M6 employ the PaSR model and the GRI2.11 scheme. Model M5 consider a single value of κ in the PaSR formulation for all the species, whereas model M6 uses additional specific values of κ for the NOx species, as put forth by Iavarone et al. (2019).
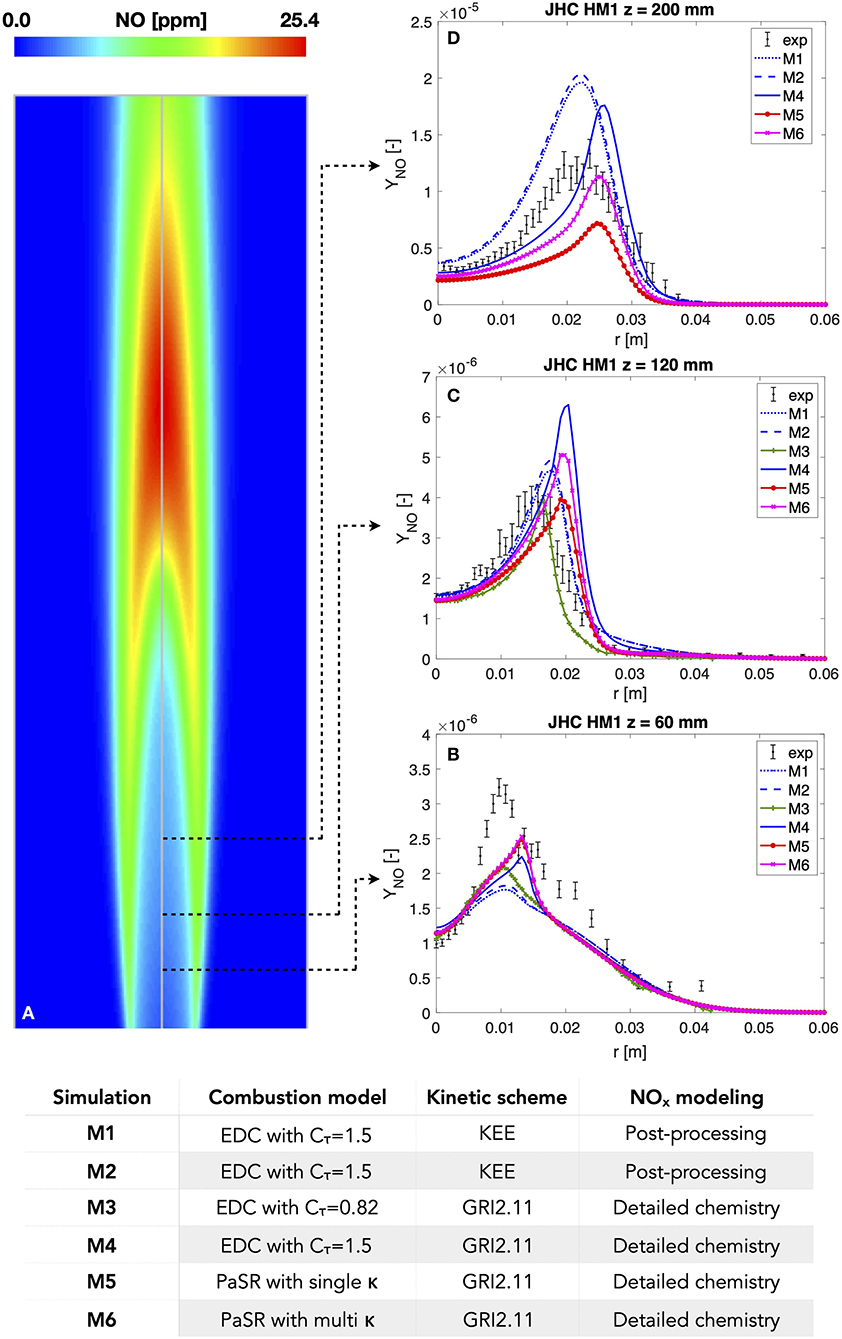
Figure 2. (A) Contour plot of NO mass fraction in the Adelaide Jet in Hot Coflow (JHC) burner for the experimental case HM1 (jet Reynolds number Re = 10 k, coflow inlet temperature Tcof = 1300 K, and YO2 = 0.03 in the coflow) (Dally et al., 2002). (B–D) Experimental and numerical radial profiles of NO mass fraction at axial locations z = 60/120/200 mm in the Adelaide JHC burner. The experimental profiles include both the mean values and the error bar with 99.5% confidence interval associated with a Student's distribution for the true mean value. The relevant settings of the numerical simulations are reported in the explanatory table at the bottom.
It can be noticed that model M6 provides improved predictions of NO at axial locations z = 60 mm and z = 200 mm. A slight overestimation persists at z = 120 mm, along with a displacement of the NO profile. The latter is due to the shift of the predicted profiles of relevant quantities for NOx formation, such as temperature and OH concentration, reported in Iavarone et al. (2019). The cause may be attributed not only to the kinetic mechanism and the combustion model employed, but also to the choice of the turbulence model, which must be able the replicate the spreading of the jet flame. At axial location z = 120 mm, the use of the post-processing tool (models M1 and M2) leads to better predictions than the ones from a detailed mechanism (model M4), given the same combustion model, namely EDC with constant Cτ = 1.5. The results of models M1 and M2 are also comparable with those of model M6 at z = 120 mm. However, these models provide an underestimation of NO emissions at z = 60 mm and a significant overestimation at z = 200 mm. This overestimation is likely due to the absence of the reburning route and the assumption of a global scheme for the prompt route, which was found to be the main contributor to NO formation in the corresponding study (Galletti et al., 2015). For models M5-M6, Iavarone et al. (2019) found that the contribution of each pathway to the peak of NO formation at z = 200 mm is as follows (in decreasing order): NNH>thermal>prompt>N2O. The analysis has been extended to the other two axial locations: at z = 60 mm the rank is prompt>NNH>N2O>thermal, whereas at z = 120 mm the NNH contribution overcomes the prompt one and the rank is thus NNH>prompt>N2O>thermal. Same results have been obtained for simulation M4, showing that the contribution rank is not affected by using a different combustion model. These trends can be related to the structure of the JHC flame. Three subregions can be identified: a fuel-rich region located inside the jet, a reaction region inside the shear layer, and a fuel-lean region. The results show that the NO emissions are produced through the prompt route in the fuel-rich region before z = 120 mm and through the NNH route further downstream, where the temperature increases in the reactive region and less fuel-rich conditions occur. Hence, the NNH route is proven to be a relevant mechanism of NOx formation in H2-enriched turbulent diffusion flames under MILD conditions. The thermal route is suppressed under the low temperature conditions, and the N2O mechanism is not important. This is in agreement with the results of Gao et al. (2013). Finally, it can also be noticed from Figure 2 that biases in the combustion closure can be as important as the level of the accuracy of the chemical scheme employed.
4. Discussion and Conclusion
MILD combustion represents a promising technology to comply with restrictive policies on pollutant emissions prompted by growing environmental issues. MILD combustion can provide low emissions of NOx thanks to the alteration of the corresponding chemistry. This alteration is caused by the peculiar features of MILD regime, namely homogeneous reaction zones, reduced temperature peaks, and changes in the composition of reactants and subsequent radical species pool. As a consequence, prompt, N2O-intermediate, NNH-intermediate, and reburning routes, which may be typically neglected in conventional combustion systems, end up playing a crucial role in the formation (and destruction) of NOx in MILD regime. Although several studies have analyzed the relative impact of the NOx chemical pathways on the emissions of NOx in MILD combustion, a dominant source has not been identified. The lack of consensus is given by several factors: (a) variability of the operating conditions at which the MILD regime is established; (b) considerable uncertainty still present in the kinetics of NNH and prompt routes; (c) strong interconnection between the hydrocarbon chemistry and the NOx chemistry, via prompt and reburning routes in particular; (d) ambiguity in the methods of isolating the contribution of a single route with respect to the others, given their interdependence; (e) role of closure sub-models, such as hydrocarbon oxidation kinetics and turbulence-chemistry interactions.
CFD tools remain crucial to aid the investigation and the design of turbulent MILD combustion systems. A summary of NOx formation modeling approaches employed in CFD simulations has been presented in this review. The approaches span from the estimation of the NOx emissions through CFD post-processing with simple kinetic mechanisms to the modeling of NOx formation with detailed kinetic schemes incorporated in turbulence-chemistry interaction models. Even though fairly good predictions of NOx emissions may be obtained by post-processing techniques, their use can be negatively affected by: (a) lack of necessary detail when simple kinetic mechanisms are used; (b) lack of generality, with risks when extrapolating, since important parameters must be adjusted on the combustion conditions and are thus case-dependent; (c) strong dependence on the accuracy of the underlying CFD simulation. On the other hand, the use of detailed mechanisms with reactor-based combustion models, desirable in terms of improved predictivity at still affordable computational costs, can be affected by the challenge of accounting for the gap of chemical time scales between nitrogen chemistry and fuel-oxidizer reactions. Therefore, models that bridge these different scales are necessary, since it has been shown that biases in the combustion closure are as important as the level of the accuracy of the chemical scheme employed.
To reduce the uncertainty related to the use of combustion models, one could leverage Direct Numerical Simulations (DNS), which solve the entire range of spatial and temporal scales of the turbulence avoiding the need for a combustion closure. DNS has been recently used to provide useful insights on the physics of MILD combustion and validate combustion models under this regime (Swaminathan, 2019). Indeed, a DNS study confirmed the flaws of the flamelet approaches in MILD combustion due to the presence of microscopic flame interactions that result into distributed reaction zones at the macroscopic level (Minamoto and Swaminathan, 2015). A-priori assessments of combustion models can be beneficial also for the prediction of NOx emission with detailed kinetic mechanisms incorporated in finite-rate chemistry combustion models. Moreover, DNS of igniting mixing layers have been performed at conditions similar to those of the Adelaide JHC experiments to study autoignition in MILD combustion (Göktolga et al., 2015), whereas the effect of turbulence intensity and level of premixing on NOx formation were studied via DNS in Karimkashi et al. (2016) and Luca et al. (2017), respectively. Thus, employing DNS data can be beneficial for NOx formation modeling in MILD combustion. So far, affordable DNS solvers can accommodate reduced kinetic schemes. However, in the next few years, the increasing computational resources can facilitate the inclusion of more detailed schemes, with the possibility to explore the range of time scales involved in the NOx chemistry and shed light on the importance of specific NOx pathways in MILD combustion. More validation studies of newly available kinetic mechanisms and combustion models are to be accomplished using data coming from either experiments or DNS of turbulent MILD burners to reach clear conclusions about the role and the modeling of NOx formation routes, fuel oxidation chemistry, and turbulence-chemistry interactions in the predictions of NOx emissions in MILD combustion.
Author Contributions
SI and AP have contributed to the research concept and design. They equally contributed to the manuscript through literature review and useful discussions. The work contains parts of their joint research activities. SI has assembled the data and written the first release of the manuscript. AP has critically revised the paper up to the final version.
Funding
This work has been financially supported by the Wiener Anspach Foundation and has received funding from the European Research Council (ERC) under the European Union's Horizon 2020 research and innovation programme under grant agreement No. 714605.
Conflict of Interest
The authors declare that the research was conducted in the absence of any commercial or financial relationships that could be construed as a potential conflict of interest.
References
Abtahizadeh, E., van Oijen, J., and de Goey, P. (2012). Numerical study of mild combustion with entrainment of burned gas into oxidizer and/or fuel streams. Combust. Flame 159, 2155–2165. doi: 10.1016/j.combustflame.2012.02.004
Barlow, R., Fiechtner, G., Carter, C., and Chen, J.-Y. (2000). Experiments on the scalar structure of turbulent CO/H2/N2 jet flames. Combust. Flame 120, 549–569. doi: 10.1016/S0010-2180(99)00126-1
Bergmann, V., Meier, W., Wolff, D., and Stricker, W. (1998). Application of spontaneous raman and rayleigh scattering and 2D lif for the characterization of a turbulent CH4/H2/N2 jet diffusion flame. Appl. Phys. B 66, 489–502. doi: 10.1007/s003400050424
Bilger, R., Stårner, S., and Kee, R. (1990). On reduced mechanisms for methane/air combustion in nonpremixed flames. Combust. Flame 80, 135–149. doi: 10.1016/0010-2180(90)90122-8
Bowman, C., Hanson, R., Davidson, D. Jr., Gardiner, W., Lissianski, V., Smith, G., et al. (1996). Gri-mech 2.11. Available online at: http://www.me.berkeley.edu/gri-mech
Bozzelli, J. W., and Dean, A. M. (1995). O + NNH: a possible new route for nox formation in flames. Int. J. Chem. Kinet. 27, 1097–1109. doi: 10.1002/kin.550271107
Cavaliere, A., and de Joannon, M. (2004). Mild combustion. Prog. Energy Combust. Sci. 30, 329–366. doi: 10.1016/j.pecs.2004.02.003
Cho, E.-S., and Chung, S. H. (2009). Numerical evaluation of NOx mechanisms in methane-air counterflow premixed flames. J. Mech. Sci. Technol. 23, 659–666. doi: 10.1007/s12206-008-1222-y
Chomiak, J. (1990). Combustion: A Study in Theory, Fact and Application. Abacus Press/Gorden and Breach Science Publishers.
Cui, Q., Morokuma, K., Bowman, J. M., and Klippenstein, S. J. (1999). The spin-forbidden reaction CH+N2 = HCN+N revisited. II. nonadiabatic transition state theory and application. J. Chem. Phys. 110, 9469–9482. doi: 10.1063/1.478949
Cuoci, A., Frassoldati, A., Stagni, A., Faravelli, T., Ranzi, E., and Buzzi-Ferraris, G. (2013). Numerical modeling of nox formation in turbulent flames using a kinetic post-processing technique. Energy Fuels 27, 1104–1122. doi: 10.1021/ef3016987
Dally, B., Karpetis, A., and Barlow, R. (2002). Structure of turbulent non-premixed jet flames in a diluted hot coflow. Proc. Combust. Inst. 29, 1147–1154. doi: 10.1016/S1540-7489(02)80145-6
Dally, B., Riesmeier, E., and Peters, N. (2004). Effect of fuel mixture on moderate and intense low oxygen dilution combustion. Combust. Flame 137, 418–431. doi: 10.1016/j.combustflame.2004.02.011
de Joannon, M., Sorrentino, G., and Cavaliere, A. (2012). Mild combustion in diffusion-controlled regimes of hot diluted fuel. Combust. Flame 159, 1832–1839. doi: 10.1016/j.combustflame.2012.01.013
De Soete, G. G. (1975). Overall reaction rates of no and N2 formation from fuel nitrogen. Symp. (Int.) Combust. 15, 1093–1102. doi: 10.1016/S0082-0784(75)80374-2
Evans, M., Petre, C., Medwell, P., and Parente, A. (2019). Generalisation of the eddy-dissipation concept for jet flames with low turbulence and low damköhler number. Proc. Combust. Inst. 37, 4497–4505. doi: 10.1016/j.proci.2018.06.017
Fenimore, C. (1971). Formation of nitric oxide in premixed hydrocarbon flames. Symp. (Int.) Combust. 13, 373–380. doi: 10.1016/S0082-0784(71)80040-1
Ferrarotti, M., Li, Z., and Parente, A. (2019). On the role of mixing models in the simulation of mild combustion using finite-rate chemistry combustion models. Proc. Combust. Inst 37, 4531–4538. doi: 10.1016/j.proci.2018.07.043
Frassoldati, A., Sharma, P., Cuoci, A., Faravelli, T., and Ranzi, E. (2010). Kinetic and fluid dynamics modeling of methane/hydrogen jet flames in diluted coflow. Appl. Ther. Eng. 30, 376–383. doi: 10.1016/j.applthermaleng.2009.10.001
Galletti, C., Ferrarotti, M., Parente, A., and Tognotti, L. (2015). Reduced no formation models for CFD simulations of mild combustion. Int. J. Hyd. Energy 40, 4884–4897. doi: 10.1016/j.ijhydene.2015.01.172
Galletti, C., Parente, A., Derudi, M., Rota, R., and Tognotti, L. (2009). Numerical and experimental analysis of no emissions from a lab-scale burner fed with hydrogen-enriched fuels and operating in mild combustion. Int. J. Hyd. Energy 34, 8339–8351. doi: 10.1016/j.ijhydene.2009.07.095
Gao, X., Duan, F., Lim, S. C., and Yip, M. S. (2013). Nox formation in hydrogen–methane turbulent diffusion flame under the moderate or intense low-oxygen dilution conditions. Energy 59, 559–569. doi: 10.1016/j.energy.2013.07.022
Glarborg, P., Alzueta, M. U., Dam-Johansen, K., and Miller, J. A. (1998). Kinetic modeling of hydrocarbon/nitric oxide interactions in a flow reactor. Combust. Flame 115, 1–27. doi: 10.1016/S0010-2180(97)00359-3
Glarborg, P., Miller, J. A., Ruscic, B., and Klippenstein, S. J. (2018). Modeling nitrogen chemistry in combustion. Prog. Energy Combust. Sci. 67, 31–68. doi: 10.1016/j.pecs.2018.01.002
Göktolga, M. U., van Oijen, J. A., and de Goey, L. P. H. (2015). 3D DNS of mild combustion: a detailed analysis of heat loss effects, preferential diffusion, and flame formation mechanisms. Fuel 159, 784–795. doi: 10.1016/j.fuel.2015.07.049
Granm, I., and Magnussen, B. (1996). A numerical study of a bluff-body stabilized diffusion flame. Part 2. Influence of combustion modeling and finite-rate chemistry. Combust. Sci. Technol. 119, 191–217.
Guo, H., Smallwood, G. J., Liu, F., Ju, Y., and Gülder, Ö. L. (2005). The effect of hydrogen addition on flammability limit and nox emission in ultra-lean counterflow CH4/air premixed flames. Proc. Combust. Inst. 30, 303–311. doi: 10.1080/00102209608951999
Gupta, A. K. (2004). Thermal characteristics of gaseous fuel flames using high temperature air. J. Eng. Gas Turb. Power 126, 9–19. doi: 10.1115/1.1610009
Harding, L. B., Klippenstein, S. J., and Miller, J. A. (2008). Kinetics of CH + N2 revisited with multireference methods. J. Phys. Chem. A 112, 522–532. doi: 10.1021/jp077526r
Haworth, N. L., Mackie, J. C., and Bacskay, G. B. (2003). An ab initio quantum chemical and kinetic study of the NNH + O reaction potential energy surface. How important is this route to no in combustion? J. Phys. Chem. A 107, 6792–6803. doi: 10.1021/jp034421p
Hayhurst, A., and Hutchinson, E. (1998). Evidence for a new way of producing no via nnh in fuel-rich flames at atmospheric pressure. Combust. Flame 114, 274–279. doi: 10.1016/S0010-2180(97)00328-3
Iavarone, S., Cafiero, M., Ferrarotti, M., Contino, F., and Parente, A. (2019). A multiscale combustion model formulation for nox predictions in hydrogen enriched jet flames. Int. J. Hyd. Energy 44, 23436–23457. doi: 10.1016/j.ijhydene.2019.07.019
Jiang, X., Li, P., Guo, J., Hu, F., Wang, F., Mi, J., et al. (2018). Detailed investigation of no mechanism in non-premixed oxy-fuel jet flames with CH4/H2 fuel blends. Int. J. Hyd. Energy 43, 8534–8557. doi: 10.1016/j.ijhydene.2018.03.100
Karimkashi, S., Bolla, M., Wang, H., and Hawkes, E. R. (2016). “A direct numerical simulation study of turbulence intensity effects on nox formation in freely propagating premixed flames,” in 20th Australasian Fluid Mechanics Conference (Perth, WA).
Klippenstein, S. J., Harding, L. B., Glarborg, P., and Miller, J. A. (2011). The role of nnh in no formation and control. Combust. Flame 158, 774–789. doi: 10.1016/j.combustflame.2010.12.013
Klippenstein, S. J., Pfeifle, M., Jasper, A. W., and Glarborg, P. (2018). Theory and modeling of relevance to prompt-no formation at high pressure. Combust. Flame 195, 3–17. doi: 10.1016/j.combustflame.2018.04.029
Konnov, A., Colson, G., and Ruyck, J. D. (2001). No formation rates for hydrogen combustion in stirred reactors. Fuel 80, 49–65. doi: 10.1016/S0016-2361(00)00060-0
Lamoureux, N., Merhubi, H. E., Pillier, L., de Persis, S., and Desgroux, P. (2016). Modeling of no formation in low pressure premixed flames. Combust. Flame 163, 557–575. doi: 10.1016/j.combustflame.2015.11.007
Lee, K., and Choi, D. (2009). Analysis of no formation in high temperature diluted air combustion in a coaxial jet flame using an unsteady flamelet model. Int. J. Heat Mass Transf. 52, 1412–1420. doi: 10.1016/j.ijheatmasstransfer.2008.08.015
Li, G., Stankovic, D., Overman, N., Cornwell, M., Gutmark, E., and Fuchs, L. (2006). “Experimental study of flameless combustion in gas turbine combustors,” in 44th AIAA Aerospace Sciences Meeting and Exhibit (Reno, NV). doi: 10.2514/6.2006-546
Li, P., Dally, B. B., Mi, J., and Wang, F. (2013). Mild oxy-combustion of gaseous fuels in a laboratory-scale furnace. Combust. Flame 160, 933–946. doi: 10.1016/j.combustflame.2013.01.024
Li, P., Wang, F., Mi, J., Dally, B., Mei, Z., Zhang, J., et al. (2014). Mechanisms of no formation in mild combustion of CH4/H2 fuel blends. Int. J. Hyd. Energy 39, 19187–19203. doi: 10.1016/j.ijhydene.2014.09.050
Li, Z., Ferrarotti, M., Cuoci, A., and Parente, A. (2018). Finite-rate chemistry modelling of non-conventional combustion regimes using a partially-stirred reactor closure: combustion model formulation and implementation details. Appl. Energy 225, 637–655. doi: 10.1016/j.apenergy.2018.04.085
Luca, S., Attili, A., and Bisetti, F. (2017). “Direct numerical simulations of NOx formation in spatially developing turbulent premixed bunsen flames with mixture inhomogeneity,” in 55th AIAA Aerospace Sciences Meeting (Grapevine, TX). doi: 10.2514/6.2017-0603
Malte, P., and Pratt, D. (1975). Measurement of atomic oxygen and nitrogen oxides in jet-stirred combustion. Symp. (Int.) Combust. 15, 1061–1070. doi: 10.1016/S0082-0784(75)80371-7
Mancini, M., Weber, R., and Bollettini, U. (2002). Predicting nox emissions of a burner operated in flameless oxidation mode. Proc. Combust. Inst. 29, 1155–1163. doi: 10.1016/S1540-7489(02)80146-8
Mardani, A., and Tabejamaat, S. (2012). Nox formation in H2-CH4 blended flame under mild conditions. Combust. Sci. Technol. 184, 995–1010. doi: 10.1080/00102202.2012.663991
Medwell, P. R., and Dally, B. B. (2012). Effect of fuel composition on jet flames in a heated and diluted oxidant stream. Combust. Flame 159, 3138–3145. doi: 10.1016/j.combustflame.2012.04.012
Miller, J. A., and Bowman, C. T. (1989). Mechanism and modeling of nitrogen chemistry in combustion. Prog. Energy Combust. Sci. 15, 287–338. doi: 10.1016/0360-1285(89)90017-8
Minamoto, Y., and Swaminathan, N. (2015). Subgrid scale modelling for mild combustion. Proc. Combust. Inst. 35, 3529–3536. doi: 10.1016/j.proci.2014.07.025
Moskaleva, L., and Lin, M. (2000). The spin-conserved reaction ch + n2 = h + ncn: a major pathway to prompt no studied by quantum/statistical theory calculations and kinetic modeling of rate constant. Proc. Combust. Inst. 28, 2393–2401. doi: 10.1016/S0082-0784(00)80652-9
Nicolle, A., and Dagaut, P. (2006). Occurrence of no-reburning in mild combustion evidenced via chemical kinetic modeling. Fuel 85, 2469–2478. doi: 10.1016/j.fuel.2006.05.021
Parente, A., Galletti, C., and Tognotti, L. (2008). Effect of the combustion model and kinetic mechanism on the mild combustion in an industrial burner fed with hydrogen enriched fuels. Int. J. Hyd. Energy 33, 7553–7564. doi: 10.1016/j.ijhydene.2008.09.058
Parente, A., Galletti, C., and Tognotti, L. (2011). A simplified approach for predicting no formation in mild combustion of CH4–H2 mixtures. Proc. Combust. Inst. 33, 3343–3350. doi: 10.1016/j.proci.2010.06.141
Parente, A., Malik, M. R., Contino, F., Cuoci, A., and Dally, B. B. (2016). Extension of the eddy dissipation concept for turbulence/chemistry interactions to mild combustion. Fuel 163, 98–111. doi: 10.1016/j.fuel.2015.09.020
Perpignan, A. A., Rao, A. G., and Roekaerts, D. J. (2018a). Flameless combustion and its potential towards gas turbines. Prog. Energy Combust. Sci. 69, 28–62. doi: 10.1016/j.pecs.2018.06.002
Perpignan, A. A. V., Talboom, M. G., Levy, Y., and Rao, A. G. (2018b). Emission modeling of an interturbine burner based on flameless combustion. Energy Fuels 32, 822–838. doi: 10.1021/acs.energyfuels.7b02473
Ranzi, E., Sogaro, A., Gaffuri, P., Pennati, G., and Faravelli, T. (1994). A wide range modeling study of methane oxidation. Combust. Sci. Technol. 96, 279–325. doi: 10.1080/00102209408935359
Sabia, P., de Joannon, M., Fierro, S., Tregrossi, A., and Cavaliere, A. (2007). Hydrogen-enriched methane mild combustion in a well stirred reactor. Exp. Therm. Fluid Sci. 31, 469–475. doi: 10.1016/j.expthermflusci.2006.04.016
Santner, J., Ahmed, S. F., Farouk, T., and Dryer, F. L. (2016). Computational study of nox formation at conditions relevant to gas turbine operation: part 1. Energy Fuels 30, 6745–6755. doi: 10.1021/acs.energyfuels.6b00420
Sepman, A., Abtahizadeh, S., Mokhov, A., van Oijen, J., Levinsky, H., and de Goey, L. (2013). Numerical and experimental studies of the no formation in laminar coflow diffusion flames on their transition to mild combustion regime. Combust. Flame 160, 1364–1372. doi: 10.1016/j.combustflame.2013.02.027
Shu, Z., Dai, C., Li, P., and Mi, J. (2018). Nitric oxide of mild combustion of a methane jet flame in hot oxidizer coflow: its formations and emissions under h2o, co2 and n2 dilutions. Fuel 234, 567–580. doi: 10.1016/j.fuel.2018.07.057
Skottene, M., and Rian, K. E. (2007). A study of nox formation in hydrogen flames. Int. J. Hyd. Energy 32, 3572–3585. doi: 10.1016/j.ijhydene.2007.02.038
Song, Y., Marrodán, L., Vin, N., Herbinet, O., Assaf, E., Fittschen, C., et al. (2019). The sensitizing effects of NO2 and no on methane low temperature oxidation in a jet stirred reactor. Proc. Combust. Inst. 37, 667–675. doi: 10.1016/j.proci.2018.06.115
Sutton, J. A., Williams, B. A., and Fleming, J. W. (2012). Investigation of ncn and prompt-no formation in low-pressure C1–C4 alkane flames. Combust. Flame 159, 562–576. doi: 10.1016/j.combustflame.2011.08.023
Swaminathan, N. (2019). Physical insights on mild combustion from DNS. Front. Mech. Eng. 5:59. doi: 10.3389/fmech.2019.00059
Szegö, G., Dally, B., and Nathan, G. (2008). Scaling of nox emissions from a laboratory-scale mild combustion furnace. Combust. Flame 154, 281–295. doi: 10.1016/j.combustflame.2008.02.001
Vasudevan, V., Hanson, R. K., Bowman, C. T., Golden, D. M., and Davidson, D. F. (2007). Shock tube study of the reaction of CH with N2: overall rate and branching ratio. J. Phys. Chem. A 111, 11818–11830. doi: 10.1021/jp075638c
Veríssimo, A. S., Rocha, A. M. A., and Costa, M. (2011). Operational, combustion, and emission characteristics of a small-scale combustor. Energy Fuels 25, 2469–2480. doi: 10.1021/ef200258t
Wang, F., Li, P., Zhang, J., Mei, Z., Mi, J., and Wang, J. (2015). Routes of formation and destruction of nitrogen oxides in CH4/H2 jet flames in a hot coflow. Int. J. Hyd. Energy 40, 6228–6242. doi: 10.1016/j.ijhydene.2015.03.047
Wünning, J., and Wünning, J. (1997). Flameless oxidation to reduce thermal no-formation. Prog. Energy Combust. Sci. 23, 81–94. doi: 10.1016/S0360-1285(97)00006-3
Zeldovich, Y. (1946). The oxidation of nitrogen in combustion explosions. Acta Physicochim. URSS 21, 577–628.
Keywords: MILD combustion, NOx formation, NOx chemistry, NOx post processing, chemical reactor network, detailed chemistry, reactor-based combustion models
Citation: Iavarone S and Parente A (2020) NOx Formation in MILD Combustion: Potential and Limitations of Existing Approaches in CFD. Front. Mech. Eng. 6:13. doi: 10.3389/fmech.2020.00013
Received: 23 November 2019; Accepted: 28 February 2020;
Published: 20 March 2020.
Edited by:
Dipankar Chatterjee, Central Mechanical Engineering Research Institute (CSIR), IndiaReviewed by:
Khanh Duc Cung, Southwest Research Institute (SwRI), United StatesMd Nurun Nabi, Central Queensland University, Australia
Copyright © 2020 Iavarone and Parente. This is an open-access article distributed under the terms of the Creative Commons Attribution License (CC BY). The use, distribution or reproduction in other forums is permitted, provided the original author(s) and the copyright owner(s) are credited and that the original publication in this journal is cited, in accordance with accepted academic practice. No use, distribution or reproduction is permitted which does not comply with these terms.
*Correspondence: Salvatore Iavarone, c2kzMzkmI3gwMDA0MDtjYW0uYWMudWs=