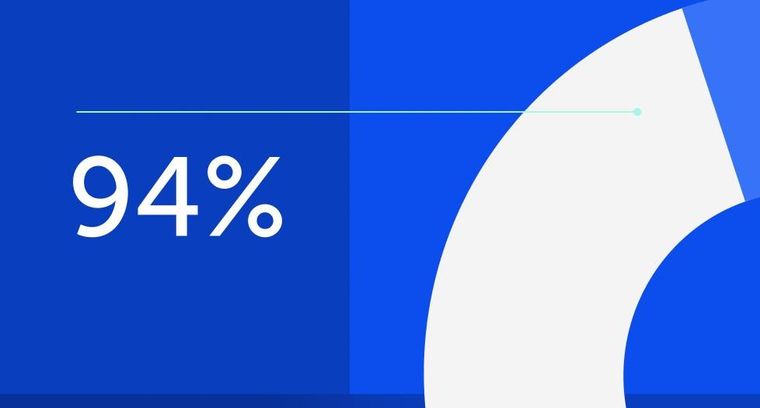
94% of researchers rate our articles as excellent or good
Learn more about the work of our research integrity team to safeguard the quality of each article we publish.
Find out more
ORIGINAL RESEARCH article
Front. Mater., 27 March 2025
Sec. Environmental Degradation of Materials
Volume 12 - 2025 | https://doi.org/10.3389/fmats.2025.1566151
Freshwater supply systems are considered as an important component within urban water systems. Although the development of freshwater supply systems may have significant impact on the environment, there have been only a few studies examining its environmental effects. This paper assesses the environmental impact of four pipeline materials in freshwater supply system using life cycle assessment following ISO 14040–14044 standards. The SimaPro 9.6.0.1 software was used for life cycle analysis. The results indicated that steel has a greater environmental impact in most impact categories during the pipe manufacturing phase than other pipeline materials. During the installation phase, two types of trenches were considered for plastic pipelines and steel pipelines installation and found that the plastic pipe trench experiences its greatest impact during installation phase. To showcase the practicality of the suggested approach, a segment of the Seri Iskandar freshwater supply system was chosen as a case study. The findings revealed that by substituting a portion of the pipes with environmentally sustainable materials, the environmental impact during manufacturing and materials phase of pipelines used for construction of FWSS can be reduced by 14% in fossil resource scarcity, 19% in ozone layer depletion, 20% in ionization radiation, 22% in climate change, and 25% in marine ecotoxicity potential.
UNESCO has clearly declared that water is among the most fundamental necessities of human beings (UNESCO, 2021). In contrast, the present worldwide water crisis is attributable to a variety of elements, such as growing populations (Fida et al., 2023), excessive use (Ismanto et al., 2023), and the effects of climate change (Greve et al., 2018). The global population is expected to reach 8.5 billion by 2030 (Bahar et al., 2020), causing significant shortages of water in regions where it is already prevalent (Brown et al., 2019). Consequently, the demand for fresh water increases significantly (Albert et al., 2021; Boretti and Rosa, 2019; Chen et al., 2018). The current increase in demand requires the improvement and expansion of freshwater networks. Apart from meeting the increasing demand, it is crucial to prioritize the maintenance of current fresh water supply networks, recognizing the economic and environmental implications that must be considered throughout the entire lifecycle of fresh water supply units (FWSU), from production to installation and upkeep (Bheel et al., 2024a). It is essential to strike a balance between these factors to guarantee sustainable access to fresh water amid new global challenges (Abdullah et al., 2024; Chohan et al., 2024).
It is imperative to incorporate environmental value into the macro-level planning of infrastructure initiatives. Neglecting the significance of the environment has resulted in an increase in hazardous gas emissions and numerous categories of environmental pollution (Baleta et al., 2019; Bheel et al., 2024b; Satterthwaite, 2021). As a result, engineers have directed their focus towards examining the ecological consequences of infrastructure, including urban water supply system (UWSS) (Chohan et al., 2023). A freshwater supply network (FWSN), a water treatment process (FWTP), a wastewater discharge network (WWDN), and a wastewater treatment process (WWTP) are the constituent elements of a UWSN, as illustrated in Figure 1. FWSN is an essential component of UWSS and is fundamental to the delivery of public service (Krueger et al., 2019).
In recent years, the majority of FWSS studies have primarily examined the mechanical, qualitative, and financial aspects of schemes. However, the rise of pollution in the environment has prompted a reconsideration of their environmental consequences (Jin, 2019; Xiong et al., 2020). Through life cycle assessment (LCA), the environmental impact of FWSN is investigated in this study. LCA, as defined by the International Organization for Standardization (ISO) (Lewandowska et al., 2011), is a technique that evaluates the environmental impact (EI) of a service, activity, or product throughout its lifespan (Chohan et al., 2023; MIR et al., 2017; Tunio et al., 2017). Since more than 2 decades ago, LCA has been utilized to assess the EI of water systems, establishing its importance (Alsadi, 2019; Hajibabaei et al., 2017; Lemos et al., 2013). Specific components of a UWSS have been the subject of some LCA-based research. Certain scientists have examined wastewater treatment plants (WWTPs) and methods for treating sediment from wastewater (Loubet et al., 2014; Loubet et al., 2016; Tidåker et al., 2006). Whereas some scientist conducted the LCA based study of FWSN and WWDN (Piratla et al., 2012; Vahidi et al., 2015; Vahidi et al., 2016). Several studies also examine UWSs in the whole (Duan et al., 2020). Other researchers regarded water users (both industrial and residential) as components of the system in addition to the UWSS, because both categories can affect the environment (Pokhrel et al., 2022; Venkatesh and Bratteb, 2011; Venkatesh and Brattebø, 2011) assessed environmental impact, energy consumption, and maintenance costs of UWSS during operation and repair phase. In accordance with their findings, WWTP accounted for 88% of the total climate change (CC), whereas FWTP accounted for a mere 5%. According to Loubet et al. (2016) the maximum EI of the UWSS in the Paris sub-urban area has been credited to its WWTP. In 2013, Amores et al. (2013) identified FWSN as the most consequential element of the UWSS due to its substantial energy consumption, as classified under the impact category such as climate change. Various studies have utilized LCA to estimate the environmental implications of various UWSS components during the development, operation, and repair/maintenance phases. Such as, Stokes and Horvvath (2006), Stokes and Horvath (2006) conducted an evaluation of the energy cycles of an FWTP, a WWTP, and an FWSN. Their findings indicated that the operation phase (including water circulation) accounted for 51%–90%. The findings indicate that the construction phase accounts for a small 3%–6% of the overall EI. An analysis of the existing scholarly works indicates that the findings are limited to the specific case study and cannot be applied to regions that have distinct specifications, as the EI of each phase of the system is primarily determined by its own characteristics. Table 1 provides an overview of research on the environmental impacts of various pipe materials in investigations of FWSNs.
Pipes are an important part of the fresh water supply system and ensure the smooth flow of fresh water. Previously, steel pipes were mainly used due to their strength and durability. However, modern advances have introduced plastic pipes such as HDPE and PVC, which offer significant advantages. Due to their lightweight, these plastics are easy to install and have corrosion resistance properties, but these types of pipes could not sustain the high pressure due to low mechanical properties as compared to steel pipes. To encounter these issues, glass fiber reinforced thermo polymers (GF-RTP) pipes were introduced. These pipes have low weight and corrosion-resistant properties like PVC and HDPE pipes and additionally have high mechanical strength like steel pipes. Prior research on FWSN neglected to evaluate the environmental consequences of GF-RTP and steel pipelines, as well as the effects of various trench configurations. In the current investigation, the EI of PVC and HDPE pipelines are assessed alongside those of GF-RTP and steel pipes. Furthermore, to compare the EI of various trenches, a distinct trench type was taken into account for every pipe. To compare the environmental impact of various pipe types during the stages of production, transportation, and installation, 7.8-inch diameters have been selected. To demonstrate the feasibility of reducing environmental impact in a practical scenario, a section of the Seri Iskandar, Perak FWSN was chosen as the research site. In addition to the conventional approach of excavating trenches, a trenchless method known as pipe bursting was implemented to underscore the significance of installation techniques for environmental impact in this study. In this study, an analytical approach such as life cycle assessment (LCA) is used to study the EI of different pipeline materials. LCA is a systematic approach in identifying the environmental consequences of resource consumption across various stages of the system’s life cycle. In urban areas, the findings of this paper can serve as an overview for determining the environmental impact of various FWSNs. Additionally, it is worth noting that architects and engineers can utilize the findings of this study to determine which pipelines material and trenches type result the minimum amount of damage to environment. This could be advantageous since pipeline materials and processes may substantially influence the EI of FWSNs.
The life-cycle assessment method is employed in this research to evaluate the environmental footprints of distinct pipeline materials, following ISO 14040 and 14,044 (ISO-14040 1997; ISO-14044 2000). ISO 14040 and 14,044 provide a complete framework for LCA studies and categorized in four more steps (see Figure 2).
Figure 2. The LCIA pipeline network methodology (Chohan et al., 2023).
This step consists of identifying the purpose of study, the boundary of study, and the functional unit for the study to have a better comparison among different product systems. The scope of this research work is to identify the most sustainable materials for FWSS in Seri Iskandar, Malaysia. This region is selected because the pipelines of FWSS in this location are 30 years old and because of their greater failure rate, these are assigned top priority for rehabilitation. In the future, PWB plans to replace approximately 80% of the 7.8-inch steel pipelines with alternative materials which has less environmental impact. It should be clear that the findings of this study for materials selection are useful for only this region and may not be used as global prospective because each country have their own cutoff values for power generation. Additionally, the scope of this study is to assess the only environmental impacts of different material and technical performance of different materials are not included. The main objectives of this study as.
• To perform comparatively LCA of various trenches, focusing on the specific for each pipeline.
• To identify the contribution of each pipeline on environmental during, manufacturing, transportation, and installation phase.
• To perform LCA on case study to identify the hotspot for better options.
The system boundaries, which may be established with the assistance of assumptions and cost limitations, indicate the steps and tasks that are encompassed within the LCA study (Laurant et al., 2020). A sequence of operations performed during the manufacturing, transport, and installation stages of FWSN piping constitute the system boundaries outlined in this article. The included phases and system boundaries are outlined in Figure 3, which depicts each of the phases of pipelines in FWSN. Environmental impact during the functioning and dismantling portions of FWSN pipelines is disregarded in accordance with these system boundaries. Aside from site-specific variables such as pump efficiency, topography, and nodal pressure, the operational characteristics of each FWSN lead to a variety of environmental consequences that are affected by these factors (Hsien et al., 2019). Conversely, the primary objective of this research is to conduct a comparative analysis of the environmental repercussions associated with various pipe materials throughout the stages of manufacturing, transportation, and installation. Impacts on the environment resulting from the material used in pipelines during functional, repair/maintenance, and decommissioning phases are undoubtedly present in previous literature (García-Sánchez and Güereca, 2019; Hsien et al., 2019). However, these impacts have not been examined in the study due to their exclusion from the defined system boundaries.
When comparing the environmental impacts of various products or services, distinct studies employ distinct functional units (FUs). The FU must correspond to the concept of the research and the parameters of its system (Albertí et al., 2019; Peñacoba-Antona et al., 2021). To illustrate, “supplying 1 m3 of water” has been used as the FU in some studies, which pertains to system efficacy (Amores et al., 2013; García-Sánchez and Güereca, 2019). As an indicator of user behavior, environmental impacts have been evaluated by others using a “1 capita/year” FU, which signifies the adequate provision of water services to a user within the urban water system (UWS) (Capodaglio and Olsson, 2020; Jeong et al., 2018). Furthermore, investigations that compare pipe types in FWSN have established the FU as an upper limit of 10 bars for unit length (Chohan et al., 2023). The selected FU for this research is “1 m of pipeline,” with a specific emphasis on the manufacturing, shipping, and installation stages. This FU was chosen to assess the EI of different pipeline types in FWSN and exclude system efficacy and user behavior from its parameters.
This phase, an essential component of Life Cycle Assessment (LCA), entails the collection of data for the purpose of quantifying the system’s inputs and outputs (Ingrao et al., 2021). This encompasses data related to the emissions and resource consumption that are correlated with the activities and processes conducted within the system (Khan et al., 2019; Nabavi-Pelesaraei et al., 2018). Pipe fabrication information was obtained from manufacturing facilities situated in Malaysia and the complete information of steel, HDPE, PVC and GF-RTP pipes is shown in Figure 4 (Shuaib et al., 2021). In addition, data about the installation phase was acquired from the Perak water board (PWB) (Author anonymous, 2024), including excavation specifications, consumption of diesel (MJ/h), and machinery times of operation (h). Table 2 details, for the purpose of illuminating the LCA inventory, the components that were included and excluded during the phases of manufacturing, transportation/shipping, and installation. To quantify the EI of consumption of resources and emissions from various processes on soil, air, and water, reputable databases like ecoinvent 3.3 and industry data 2.0 are used (Colosimo et al., 2024; Werner et al., 2016). ecoinvent 3.3 and industry data 2.0 provides a comprehensive repository of product and service information across various sectors (Author anonymous, 2024). To assess the EI of pipes and piping processes, SimaPro 9.6.0.1, a widely used Life Cycle Assessment (LCA) software tool, was employed (Barghash et al., 2022). The data collected from the ecoinvent 3.0 database and industry data 2.0 was inputted into SimaPro 9.6.0.1, with 7.8-inch and 9.0-inch pipe diameters selected for a comprehensive analysis. The LCA methodology remained consistent across all pipe diameters used in the Fresh Water Supply Network (FWSN) of the study area, aiming to identify potential strategies for reducing their environmental impact based on available data. The life-cycle inventory data for all types of pipelines considered in the study and provided in Table 3. The manufacturing phase, which includes processes and materials utilized in the fabrication of all types of pipelines, is outlined in Table 3. Internal and external corrosion resistant coating materials and processes are considered for steel pipes during the manufacturing phase. While the energy consumption for different pipeline materials during materials and manufacturing phase is detailed in Table 4. Considerable effort was dedicated to guaranteeing thorough data acquisition during this phase of the investigation. For example, this study considers processes and materials utilized for external and internal coatings on steel pipelines, in addition to the primary processes and materials. This aspect has frequently been neglected in prior research. Table 3 provides complete information regarding the sections of transportation phase included in this study. Since the distance between the manufacturing facility and the location of the installation, a transportation distance of 30 km was allocated for the pipelines and trench materials, while 15 km was designated for the excess sediment from the trench excavation to the dump land. Even though these distances would be identical for all pipe types, transportation would continue to be a significant factor when comparing the ecological consequences of various periods. Long-distance transport of pipelines and piping materials may have a significantly greater environmental impact than the preceding phases (Huang et al., 2021). In prior research, the installation phase of trench materials received inadequate consideration (Rubio et al., 2019). For all pipeline types, the studies evaluating the piping procedure examined a trench that was adapted to the characteristics of the area under investigation (Kaushal et al., 2020). This indicates that numerous proposed trench specifications fail to comply with international regulations. This paper looks at two trenches suggested by PWB that are in line with the types of pipelines. The materials and features of the trenches in this area match the requirements for the suggested trenches. In accordance with the American Water Works Association (AWWA) (McKinney, 2010), the American Society of Testing and Materials (ASTM, 2019; ASTM, 2019), and case study data (Dato et al., 2021), an assessment of the environmental impact associated with the installation of the two trench types has been carried out. The dimensions of trenches for steel pipe, plastic pipe, and GF-RTP pipes are shown in Figure 5. Furthermore, materials such as sand and gravel for plastic and steel pipelines based on the above-mentioned standards are obtained from ecoinvent 3.3 database. The trench excavation considered the hydraulic digger and diesel machine was considered as per working hours for compaction. These values for hydraulic digger and machine working hours are mentioned in Table 3.
Table 3. Inventory dataset for manufacturing, transportation, and installation of one meter steel pipe having 7.8-inch diameter.
Figure 5. Trenches specifications and dimentions (McKinney, 2010).
During this stage, the environmental impacts are assessed using the results obtained from the inventory. This is accomplished through the process of linking inventory data to impacts, in accordance with ISO standards (Laurant et al., 2020). Numerous studies evaluating the environmental influence of different types of pipelines have frequently utilized the CML two baseline 2000 as their LCA method (see Table 1). This approach priorities midpoint methodologies, classifying impacts into various categories including acidification potential, climate change, and ozone layer depletion (Acero et al., 2016). On the contrary, alternative approaches such as ReCiPe 2016 integrate midpoint levels with endpoint ICs). The ReCiPe 2016 divides environmental impacts into eighteen groups of midpoint ICs, which are subsequently converted into 3 endpoint ICs: resource availability, harm to human health, and ecosystem quality (Hardaker et al., 2022). The DALY index is employed by World Health Organization (WHO) to quantify harm inflicted on human health (Hassan et al., 2021). In contrast, degradation of ecosystem quality is gauged by the species extinction count within a designated timeframe, and resource damage is assessed by the additional energy demand related with forthcoming extraction of minerals and fossil fuels (Hassan et al., 2021). This article concentrates on Six midpoint impact categories (climate change, ionizing radiation, marine ecotoxicity, fresh water ecotoxicity, Stratospheric Ozone depletion, and fossil resource scarcity) derived from the ReCiPe 2016 to facilitate comparisons among various options for FWSNs. Based on ReCiPe 2016 method, the impact score for all impact categories can be calculated be following below Equation 1.
An assessment of the manufacturing phase’s environmental impacts was conducted utilizing the midpoint ICs of the ReCiPe 2016 method. Table 5 presents the results during the manufacturing phase. Furthermore, the finding of the environmental consequences related to the manufacturing of 7.8-inch diameter pipelines is presented in Figure 6. In Figure 6 for each impact category, the maximum result is set to 100% and the results of the other categories are displayed in relation to that result. As shown in Table 4, PVC pipelines have the minimal midpoint impact across most ICs. Although PVC and HDPE pipes generate comparable impacts, HDPE pipelines exhibit marginally greater impacts than PVC pipes. In the CC category, HDPE pipe has an environmental impact of 28.0 kg CO2 eq/meter, which is approximately 37% greater than that of PVC pipe. The main reason behind the HDPE pipe needs more materials than PVC pipe. The overall weight of HDPE pipe is 14.3 kg/m, whereas the weight of PVC is 9 kg/m. These current findings related to CC are aligned with the results of Sanjuan et al.‘s and Hajibabaei et al.‘s research (Hajibabaei et al., 2017; Sanjuan-Delmás et al., 2014). Their findings revealed that the impact of HDPE pipe was approximately 10%–15% greater than that of PVC pipe on CC. Steel pipe exhibits the most pronounced environmental impact across all six impact categories. As illustrated in Figure 6, the CC impact of steel during the manufacturing phase is six to seven times that of PVC pipelines. Due to the increased material requirements during the pipe manufacturing process, steel pipe exhibits a significantly greater environmental impact when compared to alternative pipe materials. To illustrate, in order to produce a steel pipe measuring meter in length and having a diameter of 7.8 inch, an estimated 35.3 kg of materials are needed. Conversely, the material requirements for PVC pipe of identical dimension and length are just around 9 kg. Additionally, Table 4 illustrates that the fossil resource scarcity (FRS) of steel pipe during production is greater than that of other pipelines; thus, the FRS increases in direct proportion to the amount of fuel used to generate energy for steel pipe manufacture. In a previous study, Sanjuan-Delmás et al. (2014) established that the cumulative energy demand (CED) to produce steel pipes was greater than that of plastic pipes. This finding confirms the importance of the present research in relation to the scarcity of fossil resources. Additionally, it was noted by Hajibabaei et al. (2017) that the CED of steel pipes is greater in magnitude compared to that of plastic pipes. In contrast, the findings of Piratla et al. (2012), Piratla et al. (2012), who assessed CO2 emissions from a fresh water pipeline, indicated that the embedded energy (EE) of steel pipelines was greater than that of PVC and HDPE. This discrepancy was attributed to the fact that they solely considered the EE of materials, whereas the present study incorporates pipe-manufacturing energy (including energy consumed by equipment and activities such as pipe coating, metal working, and extrusion).
The EI results related to the transportation phase for 7.8-inch pipelines are shown in Table 6. Additionally, the comparison among all categories of environmental impact is shown in Figure 7. The GF-RTP pipes have the most EI categories during the transportation phase, and the impact of HDPE and PVC is greater than that of steel pipe. This is due to the materials required in transportation in GF-RTP and plastic pipe trenches being heavier than steel pipelines. For example, the CC impact for material transportation of GF-RTP and HDPE is 4.14 and 4.10 kg CO2 eq, respectively. Furthermore, the material used for steel pipe trenches has a minimum weight than other tranches, and they have the negligible EI during transportation phase. Even though HDPE pipe and PVC pipe need fewer materials than steel pipes in the manufacturing phase, they have a greater EI during transportation phase due to the materials (gravel, sand, and crush) required for trench development. Similar type of pattern is also available in previous literature for examples in 2015 Vahidi et al. studied the environmental consequences of PVC pipe and ductile iron pipes. Their finding revealed that transportation of gravel and crushes for plastic pipe installation had more environmental impact than that of ductile iron pipe. Additionally in 2018 Hajibabaei et al. (2017) conducted LCA study of drainage system pipeline. They found that the CC of PVC was 3.25 kg CO2 eq whereas the CC of steel pipe was around 3.76 kg CO2 eq.
A consideration is given to the diesel required during the excavating processes and compacting, as well as the materials required in the excavations (gravel and sand), during the installation phase. As illustrated in Figure 5, an evaluation was conducted to compare the EI of the 2 trench varieties during the installation phase. Based on the FU, Figure 8 depicts the EI of various trench types during the installation phase for 7.8-inch pipelines. Additionally, the environmental implications of each IC are detailed in Table 7. The plastic pipe trench exhibits the most significant impact across all midpoint categories of impact when compared to the remaining trenches. Furthermore, the environmental impact of all plastic pipes is almost identical; steel pipes have a substantially lower environmental impact than plastic pipes due to the significantly lower material requirements for trenching and installation. As shown in Table 7, the climate change associated with the installation of steel pipes and plastic pipes are 7.07 kg CO2 eq and 11.7 kg CO2 eq, respectively. This indicates that the climate change of a plastic trench is about 15% greater than that of other trenches, as shown in Figure 8. In the category of CC impact, sand contributes between 76% and 79% as gravel material. In addition, the compaction in both trenches accounts for an approximate 20%–23.5% of CC. The EI of fresh water supply construction was assessed by Sanjuan et al. (2014), Sanjuan-Delmás et al. (2014), who determined that sand, when used as gravel, has a significant ecological footprint throughout the installation process. Thus, the selection of materials may have substantial implications for the environmental impact of the installation phase, as indicated in these results.
In Figure 9, which shows a detailed breakdown of the roles of the different stages, the harmful effects on the environment caused by 7.8-inch-diameter pipelines are looked at in detail. It is worth mentioning that the manufacturing process of steel pipes constitutes the predominant source of influence, accounting for approximately 90%–95% in various impact categories. In contrast, 50%–70% impacts are attributable to the manufacturing processes of plastic pipes, such as HDPE, GF-RTP, and PVC. Steel pipelines have a minimal environmental impact during transportation, as their effects are considered negligible. Conversely, the environmental ramifications of plastic pipelines are predominantly attributable to transportation, which accounts for approximately 10% across all categories. The installation phase ranks second in terms of environmental impact. Steel pipelines emit between 2% and 3% fresh water and marine ecotoxicity, 6% of their CC, and 20% of their ionizing radiation emissions. These results demonstrate, in general, that manufacturing processes have a substantial impact on environmental impacts, specifically regarding steel pipelines. Understanding these contributions can provide valuable insights for formulating approaches to mitigate the environmental impact of pipeline infrastructure throughout its lifespan.
As the subject of our investigation, we chose a segment of the Seri Iskandar FWSS that comprises pipelines constructed from widely available materials in Malaysia. The locality in question is defined by its 14,827 inhabitants and 12.14 km2 area. It is located 40 km southwest of Ipoh, the capital of the state of Perak. Approximately 126.7 L per day per person of water were consumed per capita in this region in 2023. The percentage distribution of pipe lengths by type in the Seri Iskandar region is presented in Table 8. The data utilized in this study was obtained from Perak water Board (PWB) (Dato et al., 2021). As shown in Table 8, steel comprises 90.5% of FWSS and is the predominant material for water supply pipes. One-foot sections of each pipe have been evaluated for their environmental impacts. The EI of the infrastructure has been determined by combining the overall pipe length in the FWSS (refer to Table 8) with the calculated EI. The data presented in this Table 9 indicates that steel pipelines contribute to more than 50% of the total environmental impacts throughout every category of impact. The reasoning behind this is that the infrastructure is generally constructed using steel pipelines, which, as illustrated in Figure 9, have the greatest ecological footprint in every stage.
Diverse methodologies have been suggested and examined by researchers to assess the feasibility of mitigating environmental impact. Several studies have computed the environmental impact reduction that could be achieved by substituting pipelines with lower impact for those that generate the greatest impact in a network (Hajibabaei et al., 2018; Sanjuan-Delmás et al., 2014; Venkatesh and Brattebø, 2011). Conversely, pipe replacement is contingent upon a multitude of factors, including cost, pipe type, soil composition, and pipe lifespan (Baird, 2018). Moreover, pipe materials that are prevalent in various nations may differ. For example, steel pipes usage is extremely prevalent in Malaysia. Based on the data collected, the pipelines in the case study’s FWSS are 30 years old and because of their greater failure rate, are assigned top priority for rehabilitation. In the future, PWB plans to replace approximately 80% of the 7.8-inch steel pipelines (Author anonymous, 2024). With respect to the prospective endeavours of PWB, we have evaluated two scenarios to approximate the capacity for mitigating environmental damage if network reconstruction is deemed feasible. Given the recent integration of PVC into this network, it is postulated that 7.8 mm PVC pipelines, which have a reduced ecological footprint, could serve as an alternative to steel pipes in the initial scenario. The second scenario is identical to the first, with the difference that during the installation phase, a trenchless approach would be utilised rather than open-cut trench digging. Manufacturing, transportation, and installation stages of PVC are considered in both scenarios.
For various applications, trenchless technology employs a variety of techniques, including slip lining, which allows one to choose between pipe split and cured-in-place. Cases vary regarding variables such as pipes depth, soil condition, and project objective. All these techniques have been recently implemented in Malaysia, with pipe bursting in the vicinity of this case study being a prevalent method based on the PWB recommendation. As a result, “pipe bursting” the trenchless techniques, was chosen for the replacement of old steel pipelines in this investigation. In contrast to conventional trench method, the pipe-bursting is a more expedient, cost-effective, and efficient technique that causes a reduced number of traffic disruptions. The acquisition of the requisite data from TERRA-trench less technologies was facilitated by the case study’s attributes (TERRA, 2023). To accomplish this, the TERRA-EXTRACTOR X 400 cable buster, which has an overall 40 tonnes pulling force, was chosen to replace steel pipelines with PVC pipes. The X400 has a stated capacity of 1,086 kJ/min, 1. and an operational speed of 1.2 m/min. Based on collected data and the properties of the FWSS, the hypothesise was made that a one-foot substitution of PVC for steel will consume 301.54 kJ of energy. Additionally, 100-meter-apart trenches measuring 2 × 1.8 × 1 m3 are considered when positioning X400 (TERRA, 2023). The percentage of environmental impact reduction and actual environmental effects for each of the scenarios under consideration are detailed in Table 10. The findings suggest that the second scenario exhibits greater efficacy in mitigating the environmental impact of the FWSS. 12%–25% less environmental impact can be produced by substituting PVC for steel. Pipe installation techniques, such as pipe bursting, are more effective than conventional methods at minimising EI. Selecting environmentally friendly pipeline materials can substantially mitigate environmental implications.
As the manufacturing phase has a significant impact on all impact categories, the uncertainty analysis was conducted of all type of pipeline materials in terms of climate change (CC). Figure 10 shows the mean values as well as the standard deviation of overall CC in the manufacturing phase. The mean values for GF-RPT, HDPE, PVC, and steel were approximately 36.5 kg CO2 eq, 33.5 kg CO2 eq, 20.9 kg CO2 eq, and 148 kg CO2 eq/meter, respectively. While the standard deviation was recorded around 8.58, 4.91, 7.91, and 34.75 per characterization factor for GF-RPT, HDPE, PVC, and steel, respectively. As shown in Figure 10, the steel had greater standard deviation than other materials. This higher value of standard deviation is because steel pipe required more material during manufacturing phase and have relatively higher CC. Furthermore, Appendix A. shows the supplementary data of uncertainty analysis of all impact categories. The uncertainty for all impact categories were between 9.5% and 9.96% with confidence interval of 95%.
Figure 10. Uncertainty analysis of manufacturing phase in term of CC with respect to different materials.
The EI of five frequently used pipe materials in FWSSs—steel pipe, high-density polyethylene (HDPE), polyvinyl chloride (PVC), and glass fibre-reinforced thermoplastic—is assessed in this paper using the LCA method. The system boundaries comprise the tasks and procedures performed throughout the pipe manufacturing, transportation, and installation phases. As a case study, FWSS, located in Seri Iskandar, Malaysia, has been examined in this research. From this investigation, the following conclusions are drawn.
• The steel pipe has greatest EI across all impact categories during manufacturing of material for construction that is 7 time greater than other materials.
• During transportation phase the Plastic pipes have little higher impact than steel pipes due to transportation of gravel material for trenches.
• Two types of trenches have been studied during installation phase and the trench for plastic pipe has more EI than that of steel pipe and that is 4–6 times greater because of materials and energy requirement for compaction.
• For a case study it is observed that 12%–25% reduction in EI can be achieved by replacing alternative pipe materials during manufacturing of materials for FWSS construction.
• Pipeline’s lifetime has not been considered in the functional unit.
• Operational phase and technical performance in not considered in this research work.
• The impact of the territory’s morphology on the distribution phase is not considered in this study.
• The Impact pf production and maintenance of equipment’s required for pipeline installation is not considered in this work.
Future directions for the entire life cycle (cradle to grave) of FWSS are uncertain. In addition, recycled plastic pipelines are utilised, and the EI of the manufacturing phase can be reduced through the application of recycled materials. Subsequently, an LCA study may be conducted to examine the sustainability of recycled materials utilised in pipe manufacturing to mitigate the EI. Furthermore, EI of machines and equipment required for manufacturing, transportation and installing the FWSS should be considered during LCA study to draw the complete picture and to find out the hotspot in the FWSS projects.
The raw data supporting the conclusions of this article will be made available by the authors, without undue reservation.
IMC: Investigation, Methodology, Software, Validation, Writing–original draft, Writing–review and editing. AA: Conceptualization, Formal Analysis, Resources, Supervision, Writing–review and editing. NB: Writing–original draft, Writing–review and editing, Conceptualization, Software. TN: Formal Analysis, Funding acquisition, Software, Visualization, Writing–review and editing. AHA: Formal Analysis, Funding acquisition, Visualization, Writing–review and editing.
The author(s) declare that financial support was received for the research and/or publication of this article. The authors would like to extend their sincere appreciation to Universiti Teknologi PETRONAS for their substantial financial assistance provided for this project via YUTP Cost Centre 015LC0-388 (ABMMG-IHA). This research was funded by Taif University, Saudi Arabia, for supporting this work through project number: TU-DSPP-2024-173.
The authors would like to extend their sincere appreciation to Universiti Teknologi PETRONAS for their substantial financial assistance provided for this project via YUTP Cost Centre 015LC0-388 (ABMMG-IHA). The authors extend their appreciation to Taif University, Saudi Arabia, for supporting this work through project number: TU-DSPP-2024-173.
The authors declare that the research was conducted in the absence of any commercial or financial relationships that could be construed as a potential conflict of interest.
All claims expressed in this article are solely those of the authors and do not necessarily represent those of their affiliated organizations, or those of the publisher, the editors and the reviewers. Any product that may be evaluated in this article, or claim that may be made by its manufacturer, is not guaranteed or endorsed by the publisher.
The Supplementary Material for this article can be found online at: https://www.frontiersin.org/articles/10.3389/fmats.2025.1566151/full#supplementary-material
Abdullah, G. M. S., Chohan, I. M., Ali, M., Bheel, N., Ahmad, M., Najeh, T., et al. (2024). Effect of Titanium Dioxide as nanomaterials on mechanical and durability properties of Rubberised Concrete by Applying RSM Modelling and Optimizations. Front. Mater. 11. doi:10.3389/fmats.2024.1357094
Acero, A. P., and Rodríguez, C.CirothAndreas (2016). LCIA methods: impact assessment methods in life cycle assessment and their impact categories, 1–23. GreenDelta (February 2014.
Albert, J. S., Destouni, G., Duke-Sylvester, S. M., Magurran, A. E., Oberdorff, T., Reis, R. E., et al. (2021). Scientists’ Warning to Humanity on the freshwater Biodiversity crisis. Ambio 50 (1), 85–94. doi:10.1007/s13280-020-01318-8
Albertí, J., Brodhag, C., and Fullana-i-Palmer, P. (2019). First steps in life cycle assessments of Cities with a sustainability Perspective: a proposal for Goal, function, functional Unit, and reference flow. Sci. Total Environ. 646, 1516–1527. doi:10.1016/j.scitotenv.2018.07.377
Alsadi, A. (2019). Evaluation of Carbon footprint during the life-cycle of four different pipe materials. Dr. Diss. Available online at: https://digitalcommons.latech.edu/dissertations/37.
Amores, M. J., Meneses, M., Pasqualino, J., Antón, A., and Castells, F. (2013). Environmental assessment of urban water cycle on Mediterranean conditions by LCA approach. J. Clean. Prod. 43, 84–92. doi:10.1016/j.jclepro.2012.12.033
ASTM (2019). “American Society of Testing and materials: Annual Book of standards.” doi:10.1520/D0664-18E02
Bahar, N. H. A., Lo, M., Sanjaya, M., Van Vianen, J., Alexander, P., Ickowitz, A., et al. (2020). Meeting the Food Security challenge for nine billion People in 2050: what impact on Forests? Glob. Environ. Change 62, 102056. doi:10.1016/j.gloenvcha.2020.102056
Baird, G. M. (2018). “Fixing the O&M Budget with Asset Management to create more capital Debt capacity for pipe projects,” in Pipelines 2018: Utility engineering, Surveying, and Multidisciplinary Topics - Proceedings of Sessions of the pipelines 2018 Conference, Canada, 15-18 July 2018, 43–52. doi:10.1061/9780784481660.006
Baleta, J., Mikulčić, H., Klemeš, J. J., Urbaniec, K., and Duić, N. (2019). Integration of energy, water and environmental systems for a sustainable development. J. Clean. Prod. 215, 1424–1436. doi:10.1016/j.jclepro.2019.01.035
Barghash, H., Arwa, Al F., Okedu, K. E., and Al-Wahaibi, B. M. (2022). Cost Benefit analysis for green Hydrogen production from treated Effluent: the case study of Oman. Front. Bioeng. Biotechnol. 10, 1046556. doi:10.3389/fbioe.2022.1046556
Bheel, N., Chohan, I. M., Ali Ghoto, A., Ahmed Abbasi, S., Tag-eldin, E. M., Almujibah, H. R., et al. (2024a). Synergistic effect of recycling Waste Coconut Shell Ash, Metakaolin, and Calcined Clay as supplementary Cementitious material on Hardened properties and Embodied Carbon of high strength Concrete. Case Stud. Constr. Mater. 20, e02980. doi:10.1016/j.cscm.2024.e02980
Bheel, N., Mir Chohan, I., Alwetaishi, M., Waheeb, S. A., and Alkhattabi, L. (2024b). Sustainability assessment and mechanical characteristics of high strength Concrete Blended with Marble Dust Powder and Wheat Straw Ash as Cementitious materials by using RSM Modelling. Sustain. Chem. Pharm. 39, 101606. doi:10.1016/j.scp.2024.101606
Boretti, A., and Rosa, L. (2019). Reassessing the Projections of the World water development Report. npj Clean. Water 2 (15), 15. doi:10.1038/s41545-019-0039-9
Brown, T. C., Mahat, V., and Ramirez, J. A. (2019). Adaptation to future water shortages in the United States caused by population Growth and climate change. Earth’s Future 7 (3), 219–234. doi:10.1029/2018EF001091
Capodaglio, A. G., and Gustaf, O. (2020). Energy issues in sustainable urban wastewater Management: use, demand reduction and Recovery in the urban water cycle. Sustain. Switz. 12 (1). doi:10.3390/su12010266
Chen, B., Han, M. Y., Peng, K., Zhou, S. L., Shao, L., Wu, X. F., et al. (2018). Global Land-water Nexus: Agricultural Land and freshwater Use Embodied in worldwide supply Chains. Sci. Total Environ. 613 (614), 931–943. doi:10.1016/j.scitotenv.2017.09.138
Chohan, I. M., Ahmad, A., Sallih, N., Bheel, N., Ali, M., and Ahmed, F. D. (2023). A review on life cycle assessment of different pipeline materials. Results Eng. 19, 101325. doi:10.1016/j.rineng.2023.101325
Chohan, I. M., Ahmad, A., Sallih, N., Bheel, N., Salilew, W. M., and Almaliki, A. H. (2024). Effect of Seawater Salinity, PH, and Temperature on external corrosion behavior and Microhardness of Offshore Oil and gas pipeline: RSM Modelling and Optimization. Sci. Rep. 14 (1), 16543. doi:10.1038/s41598-024-67463-2
Colosimo, B. M., Allison Jones-Farmer, L., Megahed, F. M., Paynabar, K., Ranjan, C., and Woodall, W. H. (2024). Statistical process Monitoring from industry 2.0 to industry 4.0: insights into research and Practice. Technometrics 66, 507–530. doi:10.1080/00401706.2024.2327341
Dato, Y. B., Yusof, M., and Abd, I. B. (2021). Perak water board. Available online at: http://www.lap.com.my/bi/.
Du, F., Woods, G. J., Kang, D., Lansey, K. E., and Arnold, R. G. (2013). Life cycle analysis for water and wastewater pipe materials. J. Environ. Eng. 139 (5), 703–711. doi:10.1061/(asce)ee.1943-7870.0000638
Duan, H. F., Pan, B., Wang, M., Chen, Lu, Zheng, F., and Zhang, Y. (2020). State-of-the-Art review on the Transient flow Modeling and Utilization for urban water supply system (UWSS) Management. J. Water Supply Res. Technol. - AQUA 69 (8), 858–893. doi:10.2166/aqua.2020.048
Fida, M., Li, P., Wang, Y., Khorshed Alam, S. M., and Nsabimana, A. (2023). Water Contamination and human health Risks in Pakistan: a review. Expo. Health 15 (3), 619–639. doi:10.1007/s12403-022-00512-1
García-Sánchez, M., and Güereca, L. P. (2019). Environmental and Social life cycle assessment of urban water systems: the case of Mexico city. Sci. Total Environ. 693, 133464. doi:10.1016/j.scitotenv.2019.07.270
Greve, P., Kahil, T., Mochizuki, J., Schinko, T., Satoh, Y., Burek, P., et al. (2018). Global assessment of water challenges under uncertainty in water scarcity Projections. Nat. Sustain. 1 (9), 486–494. doi:10.1038/s41893-018-0134-9
Hajibabaei, M., Nazif, S., and Sereshgi, F. T. (2018). Life cycle assessment of pipes and piping process in Drinking water distribution networks to Reduce environmental impact. Sustain. Cities Soc. 43, 538–549. doi:10.1016/j.scs.2018.09.014
Hajibabaei, M., Nazif, S., and Vahedizade, S. (2017). Comparing the environmental impacts caused by construction and Implementation of water distribution network pipes using life cycle assessment (LCA) method. J. Water Wastewater Sci. Eng. 1 (1), 37–48. doi:10.22112/jwwse.2017.51057
Hardaker, A., Styles, D., Williams, P., Chadwick, D., and Dandy, N. (2022). A framework for integrating ecosystem services as endpoint impacts in life cycle assessment. J. Clean. Prod. 370, 133450. doi:10.1016/j.jclepro.2022.133450
Hassan, A., Ilyas, S. Z., Jalil, A., and Ullah, Z. (2021). Monetization of the environmental damage caused by fossil fuels. Environ. Sci. Pollut. Res. 28 (17), 21204–21211. doi:10.1007/s11356-020-12205-w
Hsien, C., Low, J. S. C., Chan Fuchen, S., and Han, T. W. (2019). Life cycle assessment of water supply in Singapore — a water-Scarce urban city with Multiple water sources. Resour. Conservation Recycl. 151, 104476. doi:10.1016/j.resconrec.2019.104476
Huang, L., Qi, L., Yan, J., Liang, Y., and Zhang, H. (2021). Carbon footprint of Oil products pipeline transportation. Sci. Total Environ. 783, 146906. doi:10.1016/j.scitotenv.2021.146906
Ingrao, C., Stella Evola, R., Cantore, P., De Bernardi, P., Del Borghi, A., Vesce, E., et al. (2021). The contribution of Sensor-based equipment to life cycle assessment through improvement of data collection in the industry. Environ. Impact Assess. Rev. 88, 106569. doi:10.1016/j.eiar.2021.106569
Ismanto, A., Hadibarata, T., Widada, S., Indrayanti, E., Ismunarti, D. H., Safinatunnajah, N., et al. (2023). Groundwater Contamination Status in Malaysia: level of Heavy metal, source, health impact, and Remediation technologies. Bioprocess Biosyst. Eng. 46 (3), 467–482. doi:10.1007/s00449-022-02826-5
Jeong, H., Broesicke, O. A., Drew, B., and Crittenden, J. C. (2018). Life cycle assessment of small-Scale Greywater Reclamation systems combined with conventional Centralized water systems for the city of Atlanta, Georgia. J. Clean. Prod. 174, 333–342. doi:10.1016/j.jclepro.2017.10.193
Jin, Y. (2019). Estimating life cycle emissions in Managing practical Sewer pipeline projects. J. Environ. Manag. 231, 605–611. doi:10.1016/j.jenvman.2018.10.055
Kaushal, V., Najafi, M., and Serajiantehrani, R. (2020). Environmental impacts of conventional open-cut pipeline installation and trenchless technology methods: state-of-the-Art review. J. Pipeline Syst. Eng. Pract. 11 (2). doi:10.1061/(asce)ps.1949-1204.0000459
Khan, S. A. R., Chen, J., Zhang, Yu, Golpîra, H., Kumar, A., and Sharif, A. (2019). Environmental, Social and economic Growth indicators Spur Logistics performance: from the Perspective of South Asian association for regional Cooperation Countries. J. Clean. Prod. 214, 1011–1023. doi:10.1016/j.jclepro.2018.12.322
Krueger, E., Rao, P. S. C., and Borchardt, D. (2019). Quantifying urban water supply Security under global change. Glob. Environ. Change 56, 66–74. doi:10.1016/j.gloenvcha.2019.03.009
Laurant, A., Weidema, Bo P., Bare, J., Liao, X., Maia de Souza, D., Pizzol, M., et al. (2020). Methodological review and detailed Guidance for the life cycle Interpretation phase. J. Industrial Ecol. 24 (5), 986–1003. doi:10.1111/jiec.13012
Lemos, D., Dias, A. C., Gabarrell, X., and Arroja, L. (2013). Environmental assessment of an urban water system. J. Clean. Prod. 54, 157–165. doi:10.1016/j.jclepro.2013.04.029
Lewandowska, A., Matuszak-Flejszman, A., Joachimiak, K., and Ciroth, A. (2011). Environmental life cycle assessment (LCA) as a tool for Identification and assessment of environmental aspects in environmental Management systems (EMS) Part 2: case studies. Int. J. Life Cycle Assess. 16 (3), 247–257. doi:10.1007/s11367-011-0252-3
Loubet, P., Roux, P., Guérin-Schneider, L., and Bellon-Maurel, V. (2016). Life cycle assessment of Forecasting scenarios for urban water Management: a first Implementation of the WaLA Model on Paris Suburban area. Water Res. 90, 128–140. doi:10.1016/j.watres.2015.12.008
Loubet, P., Roux, P., Loiseau, E., and Bellon-Maurel, V. (2014). Life cycle assessments of urban water systems: a comparative analysis of selected Peer-Reviewed literature. Water Res. 67, 187–202. doi:10.1016/j.watres.2014.08.048
McKinney, C. (2010). “American water works association,” in American water works association Annual Conference and Exposition 2010. China, June 25, 2010, ACE 2010, Papers.
Mir, I., Samo, S., Hussain, T., Ali, I., and Durani, H. A. K. (2017). Influence of Convergent section length and Angle on performance of Supersonic Nozzle. Sindh Univ. Res. J. -Science Ser. 49 (004), 727–732. doi:10.26692/surj/2017.12.48
Nabavi-Pelesaraei, A., Rafiee, S., Saeid Mohtasebi, S., Hosseinzadeh-Bandbafha, H., and Chau, K. wing (2018). Integration of Artificial Intelligence methods and life cycle assessment to Predict energy output and environmental impacts of Paddy production. Sci. Total Environ. 631 (632), 1279–1294. doi:10.1016/j.scitotenv.2018.03.088
Peñacoba-Antona, L., Senán-Salinas, J., Aguirre-Sierra, A., Letón, P., Salas, J. J., García-Calvo, E., et al. (2021). Assessing METland® Design and performance through LCA: Techno-environmental study with Multifunctional Unit Perspective. Front. Microbiol. 12, 652173. doi:10.3389/fmicb.2021.652173
Author anonymous (2024). Perak water board. Available online at: http://www.lap.com.my/bi/.
Piratla, K. R., Ariaratnam, S. T., and Cohen, A. (2012). Estimation of CO2 emissions from the life cycle of a potable water pipeline project. J. Manag. Eng. 28 (1), 22–30. doi:10.1061/(asce)me.1943-5479.0000069
Pokhrel, S. R., Chhipi-Shrestha, G., Hewage, K., and Sadiq, R. (2022). Sustainable, Resilient, and Reliable urban water systems: Making the case for a ‘one water’ approach. Environ. Rev. 30 (1), 10–29. doi:10.1139/er-2020-0090
Rubio, C., Mar, M. del, Pujadas, P., Pardo-Bosch, F., Blanco, A., and Aguado, A. (2019). Sustainability assessment of trenches including the new Eco-trench: a Multi-Criteria Decision-Making tool. J. Clean. Prod. 238, 117957. doi:10.1016/j.jclepro.2019.117957
Sanjuan-Delmás, D., Petit-Boix, A., Gasol, C. M., Villalba, G., Suárez-Ojeda, M. E., Gabarrell, X., et al. (2014). Environmental assessment of different pipelines for Drinking water transport and distribution network in small to Medium Cities: a case from Betanzos, Spain. J. Clean. Prod. 66, 588–598. doi:10.1016/j.jclepro.2013.10.055
Satterthwaite, D. (2021). Sustainable Cities or Cities that contribute to sustainable development? Earthscan Read. Sustain. Cities, 80–106. doi:10.4324/9781315800462-7
Shuaib, N. A., Syazwan Osman, M., Shaharil, H., Wai, L. T., Fauzi, M. F. M., Jui, T. H., et al. (2021). Risk assessment in a pipe manufacturing industry: analysis from a case study. AIP Conf. Proc. 2339, 020210. doi:10.1063/5.0044247
Stokes, J., and Horvath, A. (2006). Life cycle energy assessment of alternative water supply systems (9 pp). Int. J. Life Cycle Assess. 11 (5), 335–343. doi:10.1065/lca2005.06.214
TERRA (2023). Terra. Available online at: http://en.terra-eu.eu/trenchless-pipelaying/2-0-terraag.
Tidåker, P., Kärrman, E., Baky, A., and Jönsson, H. (2006). Wastewater Management integrated with Farming -an environmental systems analysis of a Swedish country Town. Resour. Conservation Recycl. 47 (4), 295–315. doi:10.1016/j.resconrec.2005.12.003
Tunio, I., Kumar, D., and Chohan, I. M. (2017). “Dynamic Structural and modal analysis of Tyre Coupling on,” in AUSTRALIAN JOURNAL OF ENGINEERING and technology research AJMFR. Available online at: http://www.australianresearchjournals.com.au/.
UNESCO (2021). “Cultural values of water,” in UN World water development Report 2021. Available online at: https://www.unesco.org/reports/wwdr/2021/en/cultural-values-water.
Vahidi, E., Jin, E., Das, M., Singh, M., and Fu, Z. (2015). Comparative life cycle analysis of materials in wastewater piping systems. Procedia Eng. 118, 1177–1188. doi:10.1016/j.proeng.2015.08.461
Vahidi, E., Jin, E., Das, M., Singh, M., and Fu, Z. (2016). Environmental life cycle analysis of pipe materials for Sewer systems. Sustain. Cities Soc. 27, 167–174. doi:10.1016/j.scs.2016.06.028
Venkatesh, G., and Brattebø, H. (2011). Energy consumption, costs and environmental impacts for urban water cycle services: case study of Oslo (Norway). Energy 36 (2), 792–800. doi:10.1016/j.energy.2010.12.040
Werner, P., Bauer, C. J., Steubing, B., Reinhard, J., Moreno-Ruiz, E., and Weidema, B. (2016). The ecoinvent database Version 3 (Part I): overview and methodology. Int. J. Life Cycle Assess. 21, 1218–1230. doi:10.1007/s11367-016-1087-8
Keywords: pipeline materials, life cycle sustainability, fresh water supply system, environmental impacts, sustainability
Citation: Chohan IM, Ahmad A, Bheel N, Najeh T and Almaliki AH (2025) Sustainability assessment of different pipeline materials in freshwater supply systems. Front. Mater. 12:1566151. doi: 10.3389/fmats.2025.1566151
Received: 24 January 2025; Accepted: 27 February 2025;
Published: 27 March 2025.
Edited by:
Tadeusz Hryniewicz, Koszalin University of Technology, PolandReviewed by:
Pavlo Maruschak, Ternopil Ivan Pului National Technical University, UkraineCopyright © 2025 Chohan, Ahmad, Bheel, Najeh and Almaliki. This is an open-access article distributed under the terms of the Creative Commons Attribution License (CC BY). The use, distribution or reproduction in other forums is permitted, provided the original author(s) and the copyright owner(s) are credited and that the original publication in this journal is cited, in accordance with accepted academic practice. No use, distribution or reproduction is permitted which does not comply with these terms.
*Correspondence: Imran Mir Chohan, aW1yYW5fMjIwMDY3OTlAdXRwLmVkdS5teQ==; Taoufik Najeh, dGFvdWZpay5uYWplaEBsdHUuc2U=
Disclaimer: All claims expressed in this article are solely those of the authors and do not necessarily represent those of their affiliated organizations, or those of the publisher, the editors and the reviewers. Any product that may be evaluated in this article or claim that may be made by its manufacturer is not guaranteed or endorsed by the publisher.
Research integrity at Frontiers
Learn more about the work of our research integrity team to safeguard the quality of each article we publish.