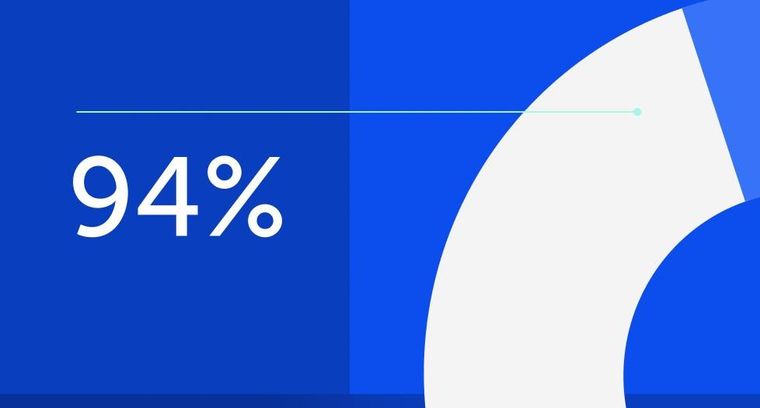
94% of researchers rate our articles as excellent or good
Learn more about the work of our research integrity team to safeguard the quality of each article we publish.
Find out more
REVIEW article
Front. Mater., 28 March 2025
Sec. Biomaterials and Bio-Inspired Materials
Volume 12 - 2025 | https://doi.org/10.3389/fmats.2025.1530524
This review discusses the current applications of hydrogels in the management of airway lung injury and ventilator-associated pneumonia (VAP), with a particular focus on their potential impact in clinical practice. Research indicates that hydrogel technology can not only enhance airway management and promote lung tissue repair but also significantly reduce the risk of infection. For instance, clinical trials have shown that hydrogel-coated endotracheal tubes are effective at reducing the incidence of VAP, with related data indicating a reduction of up to 30%. Furthermore, we analyze the limitations of current research and propose directions for the future development of hydrogel-based therapies in this important field of respiratory medicine.
Ventilator-associated pneumonia (VAP) refers to pneumonia that occurs 48 h or more after intubation and mechanical ventilation and represents a significant complication for patients receiving mechanical ventilation. According to recent epidemiological studies, the incidence of VAP in intensive care units has risen to approximately 27%, highlighting the urgent need for effective management strategies. VAP not only increases patient morbidity but also prolongs hospital stays and raises mortality rates, making it crucial to gain a deeper understanding of the mechanisms of airway lung injury. Epidemiological studies have reported that VAP affects 9%–27% of mechanically ventilated patients, with variations depending on the population and healthcare settings (Rouzé and Nseir, 2022). The incidence of VAP in intensive care units is particularly concerning, as these patients are often critically ill and more susceptible to infections (Guillamet and Kollef, 2024). Understanding the mechanisms responsible for airway lung injury is crucial, as these injuries can exacerbate the severity of pneumonia and complicate treatment strategies.
The pathophysiology of airway lung injury in the context of VAP is multifactorial. Mechanical ventilation can lead to barotrauma and volutrauma, resulting in damage to the alveolar-capillary membrane. Additionally, the presence of endotracheal tubes can disrupt normal mucociliary function, leading to impaired clearance of secretions and increased risk of bacterial colonization (Xu et al., 2019). The inflammatory response triggered by these injuries can further compromise lung function and promote the development of pneumonia. Clinically, this translates to worsened oxygenation, increased work of breathing, and a higher likelihood of prolonged mechanical ventilation (Motowski et al., 2023).
Considering these risks of adverse effects, the exploration of innovative strategies to prevent and treat VAP is essential. The application of biocompatible materials, such as hydrogels, in clinical settings presents a promising avenue for enhancing patient outcomes. Hydrogels are three-dimensional polymer networks that can retain large amounts of water, making them suitable for various biomedical applications, including drug delivery and tissue engineering. Their unique properties allow for the encapsulation of therapeutic agents, which can be released in a controlled manner to target specific tissues or conditions (Chen et al., 2020). In relation to the prevention and management of VPA, the development and application of hydrogel-based strategies could potentially mitigate airway injuries and improve lung function, highlighting the importance of ongoing research in this area (Tian et al., 2023).
VAP arises primarily due to the introduction of pathogens into the lower respiratory tract via the endotracheal tube, which allows them to bypass the natural airway defenses. This condition is particularly prevalent in critically ill patients who are mechanically ventilated, as they often exhibit diminished immune responses and impaired mucociliary clearance. The presence of biofilms on the endotracheal tube can harbor bacteria, facilitating their migration into the lungs. Studies have indicated that factors such as prolonged mechanical ventilation, extended sedation, and a longer duration of intubation significantly increase the risk of VAP. Pathogens commonly identified in VAP include multidrug-resistant organisms, which not only complicate treatment but also increase the morbidity and mortality rates associated with this condition (Rouzé and Nseir, 2022). Additionally, the microenvironment of the ventilated lung is characterized by changes in pH and oxygenation that can promote bacterial colonization and subsequent infection (Motowski et al., 2023).
The main physiological mechanisms of airway protection, including the mucociliary escalator, cough reflex, and airway epithelial integrity, are significantly compromised in patients receiving mechanical ventilation. Intubation disrupts the normal function of the cilia and alters the secretory properties of airway epithelial cells, leading to impaired mucus clearance. The inflammatory response triggered by mechanical ventilation further exacerbates epithelial damage, resulting in increased permeability and susceptibility to infection (Sunil and Skaria, 2022). Furthermore, artificial ventilation can lead to alterations in the respiratory microbiome, promoting dysbiosis and favoring the growth of pathogenic bacteria over commensal flora (Sommerstein et al., 2019). Such disruptions not only facilitate the colonization of harmful microorganisms but also diminish the host’s ability to mount an effective immune response, thereby increasing the risk of VAP (Fromentin et al., 2021).
The onset of VAP is closely linked to bacterial infection, which normally triggers a robust inflammatory response. Upon invasion of the alveolar space, pathogens are detected by immune cells, leading to activation of pro-inflammatory cytokines and chemokines. This inflammatory cascade is essential for recruiting additional immune cells to the site of infection; however, it can also result in collateral damage to lung tissue, contributing to the pathophysiology of VAP (Acharya et al., 2023). In critically ill patients, an imbalance between pro-inflammatory and anti-inflammatory responses commonly results in a hyper-inflammatory state that can worsen lung injury and impair gas exchange (Loftus et al., 2021). Additionally, the presence of biofilm-forming bacteria such as Pseudomonas aeruginosa complicates the inflammatory response by evading immune detection and contributing to chronic inflammation, making treatment more challenging (Sodhi et al., 2019). Understanding the interplay between bacterial infection and the host inflammatory response is crucial for developing effective strategies to prevent and treat VAP.
Hydrogels are three-dimensional polymer networks that can retain a large amount of water within their structure. Depending on their source, hydrogels can be divided into two main categories: natural and synthetic. Natural hydrogels, such as collagen, alginates, and gelatin, have good biocompatibility and biodegradability, while synthetic hydrogels are produced through chemical processes, allowing for precise adjustment of their chemical properties to achieve specific functions. In recent years, the development of smart hydrogels has enabled them to change their properties in response to environmental stimuli (such as pH, temperature, or specific biomolecules), which has broad application prospects in fields such as drug delivery and tissue engineering. They can be classified based on their source materials, structure, and response to environmental stimuli. Natural hydrogels, such as collagen, alginate, and gelatin, are derived from biological sources and offer good biocompatibility and biodegradability. In contrast, synthetic hydrogels are produced through chemical processes and offer the possibility of precise chemical tailoring to achieve specific properties and functions. Commonly used synthetic hydrogels include crosslinked networks of polyacrylamide, polyethylene glycol (PEG), and polyvinyl alcohol, and the ability to tune the properties, including mechanical strength, of these hydrogels is well-established. Smart hydrogels that can change their properties in response to environmental cues also have been developed. Accordingly, hydrogels can also be categorized based on their responsiveness to stimuli, such as pH, temperature, or specific biomolecules. This level of classification is relevant to their diverse applications in fields such as drug delivery, tissue engineering, and wound healing (Pepelanova, 2021; Trapani et al., 2023).
The biocompatibility and biodegradability of hydrogels are essential characteristics that determine their suitability for medical applications. Biocompatibility refers to the ability of a material to perform its intended function within the body with elicitation of an appropriate host response. Hydrogels derived from natural polymers often exhibit excellent biocompatibility, because they closely mimic the extracellular matrix (ECM) of tissues. Synthetic hydrogels can also be engineered to achieve good biocompatibility through surface modifications and the incorporation of bioactive molecules. Biodegradability is another critical factor in most applications, depending on the desired function of the hydrogel and the duration of that function. For example, in applications that use hydrogels for drug delivery and tissue scaffolding, the hydrogel material must degrade at a precise rate over time to achieve a specific rate of drug or release and allow for tissue regeneration, respectively. Recent studies have focused on developing hydrogels with adjustable biodegradability, to enable the controlled release of therapeutic agents while minimizing long-term foreign body reactions (An et al., 2021; Liu et al., 2023). As an example, pectin-based hydrogels have shown promise due to their self-healing and biodegradable properties, which make them suitable for enhanced synergistic anticancer therapies (An et al., 2021).
Hydrogels have been extensively investigated in medical research studies and ultimately employed in many clinical applications due to their unique properties, which make them suitable for a wide variety of applications. One of the most prominent uses of hydrogels to date is in drug delivery systems, in which their ability to swell and retain drugs allows for controlled drug release profiles. For example, injectable hydrogels have been developed for localized drug delivery and shown to provide sustained therapeutic effects while minimizing systemic side effects (Priddy-Arrington et al., 2023). Additionally, hydrogels are used in many wound dressings due to their moisture-retentive properties, which promote healing and prevent infection. Recent advancements have focused on the development of antimicrobial hydrogels that incorporate agents to combat infection at the wound site (Li et al., 2024). In tissue engineering applications, hydrogels serve as scaffolds that support cell growth and tissue regeneration, with research focusing on tuning their mechanical properties and bioactivity to best mimic native tissues and adjusting their biodegradability to coincidence with new tissue formation (Strecanska et al., 2022). Beyond these fields, hydrogels are being explored in innovative applications such as soft robotics and wearable sensors, emphasizing their versatility beyond traditional medical uses (Wang et al., 2024; Wang et al., 2022). Overall, the current landscape of hydrogel applications in medicine demonstrates their increasing importance in the development of advanced healthcare technologies.
Hydrogels have become a promising material in airway management technologies due to their unique properties, such as biocompatibility, high water content, and tunable mechanical characteristics. In airway repair, hydrogels can mimic the natural extracellular matrix, providing a favorable environment for cell adhesion and proliferation. Additionally, hydrogels can serve as drug delivery systems, directly delivering therapeutic agents to the site of injury, providing both structural support and targeted treatment functions. These properties make hydrogels suitable candidates for use as a scaffold material in tracheal repair, particularly in reconstructive surgery and the treatment of various airway injuries. The ability of hydrogels to mimic the natural ECM provides an environment conducive to cell adhesion and proliferation, which is essential for effective airway healing and regeneration. Recent studies exploring the application of 3D-bioprinted hydrogels in tracheal reconstruction have demonstrated their potential to support cellular growth and integration into host tissues, resulting in improved success rates for surgical interventions (Frejo and Grande, 2019). Furthermore, hydrogels can be engineered to deliver therapeutic agents directly to the site of injury (Bai et al., 2022), offering dual functions as both a structural support and a drug delivery system. This capability not only addresses the immediate mechanical needs of the airway but also facilitates targeted therapy, potentially improving outcomes in cases of airway trauma or disease.
Hydrogel-based strategies have also shown promise for improving airway patency and minimizing injury during various medical procedures. Hydrogels can be designed to provide a cushioning effect, reducing the risk of trauma to delicate airway tissues during intubation or extubation processes. Research has indicated that the use of hydrogel-coated endotracheal tubes can significantly decrease the incidence of pressure ulcers and trauma to the tracheal mucosa, thereby enhancing patient comfort and safety (San Miguel-Pagola et al., 2020). Additionally, hydrogel use can help maintain airway moisture, which is crucial for preventing mucosal damage and ensuring optimal respiratory function. By providing a protective barrier against mechanical stress and maintaining the integrity of the airway lining, hydrogels can reduce the incidence of complications associated with airway management (Zhao et al., 2020; Li et al., 2020), such as inflammation and infection. This protective role is particularly vital in patients with pre-existing airway conditions, where the risk of injury is heightened.
Hydrogel-based approaches have shown promise in promoting lung clearance and maintaining pulmonary hygiene (Suk et al., 2019; Patel and Jain, 2015), particularly in patients with conditions that predispose them to mucus obstruction, such as cystic fibrosis and chronic obstructive pulmonary disease. The viscoelastic properties of hydrogels can facilitate the mobilization and clearance of mucus from the airways, thereby supporting respiratory function and reducing the risk of infections. San Miguel-Pagola et al. demonstrated that incorporation of hypertonic saline within hydrogel formulations can significantly improve sputum expectoration in patients with cystic fibrosis, leading to better lung function and quality of life (San Miguel-Pagola et al., 2020). Moreover, as drug delivery systems, hydrogels can be used to administer mucolytic agents directly to the airways, to induce the breakdown of thick mucus and promote effective clearance. This dual action can not only provide immediate symptom relief but also promote long-term lung health by preventing accumulation of pathogens and inflammatory secretions, thus lowering the incidence of exacerbations and hospitalizations in at-risk populations (Na et al., 2021).
Hydrogels are also promising biomaterials in strategies for lung tissue repair. As in the airway, their unique properties, including high water content, biocompatibility, and tunable mechanical characteristics, again make them ideal candidate materials mimicking the ECM of lung tissue. The use of hydrogels in lung repair is being explored in various contexts, including idiopathic pulmonary fibrosis and other lung injuries. By providing a supportive environment for cellular activities, hydrogels can facilitate tissue regeneration and functional recovery of lung tissues, thereby improving outcomes for patients with pulmonary diseases.
A critical property of hydrogels in lung tissue repair is their ability to promote cell proliferation and migration. Hydrogels can be engineered to release growth factors and other bioactive molecules that stimulate the proliferation of lung epithelial and mesenchymal stem cells (Zhang et al., 2020; Chen et al., 2022). For example, Ulldemolins et al. discovered that ECM-derived hydrogels release bioactive molecules that boost fibroblast function and facilitate lung epithelial repair by creating a favorable microenvironment for cellular activities (Ulldemolins et al., 2022). Additionally, the mechanical properties of hydrogels can be tailored to match those of native lung tissue, which better supports cell adhesion and migration. Another study showed that soft hydrogels incorporating matrix metalloproteinase-cleavable sites and RGD-based adhesive sites could promote both cell proliferation and differentiation, leading to improved recruitment of cells to the site of injury (Wei et al., 2020). Such biological functions are particularly important in the context of lung diseases, in which a rapid cellular response is necessary to counteract tissue damage and initiate repair processes.
Hydrogels also have demonstrated the ability to promote angiogenesis, which is crucial for effective tissue regeneration. Vascularization of hydrogels can enhance the delivery of nutrients and oxygen to injured tissues, thereby supporting cell survival and function. For example, PEGylated fibrin hydrogels were shown to increase the proliferation of human-induced pluripotent stem cell-derived cardiomyocytes by promoting angiogenesis (Vanderslice et al., 2024). Furthermore, the incorporation of angiogenic factors, such as vascular endothelial growth factor (VEGF), into hydrogels was shown to stimulate endothelial cell migration and sprouting, promoting the formation of new blood vessels (Min and Tae, 2023). Angiogenesis is an essential process in the repair of lung tissue repair, as an adequate blood supply is necessary to restore normal tissue function and facilitate the regeneration of alveoli and other lung structures.
By supporting cellular activities and promoting angiogenesis, hydrogels can help restore the structural integrity and functional capacity of lung tissues. Accordingly, the use of hydrogels in lung tissue repair has been associated with improved lung function and better prognosis for patients suffering from pulmonary conditions. In one study, hydrogel use was shown to enhance the mechanical properties of repaired tissues, ultimately leading to improved respiratory function (Sun et al., 2023). Moreover, the use of a hydrogel material in combination with other therapeutic modalities, such as stem cell therapy, provided promising results in a preclinical model, suggesting that such approaches may enhance the overall efficacy of treatments for lung diseases (Shen et al., 2022). With continued research advances, hydrogels are expected to play an increasingly vital role in the development of innovative therapies to improve lung health and patient outcomes.
Current research reveals several limiting factors that hinder the development of hydrogels in biomedical applications. Among these, poor batch-to-batch consistency of hydrogel properties is a major challenge, which may lead to inconsistent performance in clinical applications. Variability in the synthesis processes, including the choice of monomers and crosslinking agents, can also lead to the production of hydrogels with differing mechanical and biological properties, complicating their standardization for medical use (Giordano et al., 2023). To address this issue, researchers are exploring the use of hybrid materials that combine the advantages of natural and synthetic polymers to achieve more consistent mechanical properties and degradation characteristics. Additionally, advancements in 4D printing technology have introduced new possibilities for the application of hydrogels, as this technology can allow the shape and properties of hydrogels to change in response to environmental stimuli, thereby enhancing their adaptability in dynamic clinical settings. Furthermore, unpredictable degradation patterns and rates pose challenges for the application of some hydrogel materials in controlled drug delivery systems and tissue engineering (Gan et al., 2024). An incomplete understanding of the interactions between hydrogels and biological environments also presents a barrier to their clinical application, as these interactions can significantly affect drug release and influence cell behavior (Babić Radić et al., 2021). Additionally, the limited scalability of hydrogel production techniques can restrict their availability for widespread clinical application. Addressing these limitations will require a concerted effort to refine synthesis methods, enhance material characterization, and develop standardized testing protocols to ensure the reliability and safety of hydrogel-based products.
The future of hydrogel research is promising, particularly with the ongoing development of novel hydrogel materials that provide advanced functionalities. Innovations such as 4D printing technology are being explored for the creation of hydrogels that can change shape or properties in response to environmental stimuli, offering new possibilities for drug delivery and tissue engineering applications (Tran et al., 2022). Additionally, the integration of bioactive compounds into hydrogel matrices continues to be investigated as a means to enhancing their therapeutic effectiveness through improved biocompatibility and to achieve targeted delivery of drugs (Jiang et al., 2022). Research is also investigating hybrid hydrogels consisting of both natural and synthetic polymers to leverage the advantages of both types of materials in order to achieve improved mechanical properties and degradation profiles (Lee et al., 2023). Furthermore, the exploration of smart hydrogels that respond to specific biological signals or stimuli continues to show these materials offer great potential for personalized medicine by allowing for tailored therapeutic strategies (Pierau and Versace, 2021). Continued interdisciplinary collaboration between materials scientists, biologists, and clinicians will be essential to further development of these innovations and their translation into clinical applications.
The clinical translation of hydrogel technologies presents both challenges and significant opportunities. While potential applications of hydrogels in drug delivery, wound healing, and tissue engineering are well-documented, efforts to apply them in clinical use have met many obstacles. These include regulatory challenges, requirements for extensive preclinical and clinical testing to establish safety and efficacy, and the need for cost-effective manufacturing processes (Gawel et al., 2021). However, continually increasing interest in regenerative medicine and personalized healthcare is creating a favorable environment for the adoption of hydrogel-based therapies. As researchers continue to address the limitations of existing hydrogel materials and develop novel applications, the prospects for clinical use are expanding. For example, the integration of hydrogels in combination therapies may enhance treatment outcomes in complex conditions such as cancer and chronic wounds, where conventional therapies fall short (Wang et al., 2019). Moreover, nanoparticle-infused hydrogels for targeted drug delivery have shown promise in improving therapeutic efficacy while minimizing side effects (Babić Radić et al., 2021). Eventual clinical application of hydrogel-based strategies will depend on ongoing research efforts and collaboration across disciplines, to overcome the current limitations in the field.
Due to their unique properties, including their biocompatibility, moisture retention, and ability to deliver therapeutic agents, hydrogels represent a potentially transformative solution for mitigating lung damage and enhancing recovery in critically ill patients. Several limiting factors still hinder the development of hydrogels in biomedical applications though, including poor batch-to-batch consistency of hydrogel properties, which may lead to inconsistent performance in clinical applications. Possible solutions currently under investigation include the use of hybrid materials that combine the advantages of natural and synthetic polymers to achieve more consistent mechanical properties and degradation characteristics. Another potential solution is the application of 4D printing technology for hydrogel production, as this technology can produce hydrogels capable of altering their shape and properties in response to environmental stimuli, thereby enhancing their adaptability in dynamic clinical settings.
It is also crucial to recognize the existing heterogeneity in research findings regarding the efficacy and safety of different hydrogel formulations. This variability underscores the need for systematic approaches to evaluate and optimize these materials for clinical application. Future research should prioritize understanding the interactions between hydrogel components and lung tissues, as well as the pharmacodynamics of incorporated drugs. Standardized protocols also must be developed to facilitate comparability across studies, which is necessary to establish robust evidence supporting the clinical use of hydrogels.
Interdisciplinary collaboration will be vital in bridging the gap between laboratory research and clinical practice. Integration of insights from materials science, pharmacology, and respiratory medicine will be critical to achieving the therapeutic potential of hydrogels and engineering them to meet the specific needs of patients suffering from airway injuries and VAP.
Ultimately, the prospect of hydrogels as a novel therapeutic strategy in respiratory medicine is both exciting and hopeful. As ongoing research continues to reveal their capabilities, significant opportunities to redefine treatment paradigms for airway lung injury and VAP are expected, potentially improving patient outcomes and reducing healthcare burdens.
YX: Conceptualization, Supervision, Visualization, Writing–original draft. BZ: Formal Analysis, Investigation, Resources, Writing–original draft.
The author(s) declare that financial support was received for the research, authorship, and/or publication of this article. This work was supported by the National Natural Science Foundation of Guangdong (No. 2024A1515012881).
The authors declare that the research was conducted in the absence of any commercial or financial relationships that could be construed as a potential conflict of interest.
The authors declare that no Generative AI was used in the creation of this manuscript.
All claims expressed in this article are solely those of the authors and do not necessarily represent those of their affiliated organizations, or those of the publisher, the editors and the reviewers. Any product that may be evaluated in this article, or claim that may be made by its manufacturer, is not guaranteed or endorsed by the publisher.
VAP, Ventilator-associated pneumonia; PEG, Polyethylene glycol; ECM, Extracellular matrix; VEGF, Vascular endothelial growth factor.
Acharya, Y., Taneja, K. K., and Haldar, J. (2023). Dual functional therapeutics: mitigating bacterial infection and associated inflammation. RSC Med. Chem. 14 (8), 1410–1428. doi:10.1039/d3md00166k
An, H., Yang, Y., Zhou, Z., Bo, Y., Wang, Y., He, Y., et al. (2021). Pectin-based injectable and biodegradable self-healing hydrogels for enhanced synergistic anticancer therapy. Acta biomater. 131, 149–161. doi:10.1016/j.actbio.2021.06.029
Babić Radić, M. M., Filipović, V. V., Vukomanović, M., Nikodinović Runić, J., and Tomić, S. L. (2021). Degradable 2-hydroxyethyl methacrylate/gelatin/alginate hydrogels infused by nanocolloidal graphene oxide as promising drug delivery and scaffolding biomaterials. Gels Basel, Switz. 8 (1), 22. doi:10.3390/gels8010022
Bai, M. H., Zhao, B., Liu, Z. Y., Zheng, Z. L., Wei, X., Li, L., et al. (2022). Mucosa-like conformal hydrogel coating for aqueous lubrication. Adv. Mater. Deerf. Beach, Fla 34 (46), e2108848. doi:10.1002/adma.202108848
Chen, S., Yang, M., and Liu, Y. (2022). Controlled release of growth factors from smart hydrogels for lung regeneration. Adv. Healthc. Mater.
Chen, Y., Zhang, R., Zheng, B., Cai, C., Chen, Z., Li, H., et al. (2020). A biocompatible, stimuli-responsive, and injectable hydrogel with triple dynamic bonds. Mol. Basel, Switz. 25 (13), 3050. doi:10.3390/molecules25133050
Frejo, L., and Grande, D. A. (2019). 3D-bioprinted tracheal reconstruction: an overview. Bioelectron. Med. 5, 15. doi:10.1186/s42234-019-0031-1
Fromentin, M., Ricard, J. D., and Roux, D. (2021). Respiratory microbiome in mechanically ventilated patients: a narrative review. Intensive care Med. 47 (3), 292–306. doi:10.1007/s00134-020-06338-2
Gan, X., Wang, X., Huang, Y., Li, G., and Kang, H. (2024). Applications of hydrogels in osteoarthritis treatment. Biomedicines 12 (4), 923. doi:10.3390/biomedicines12040923
Gawel, D., Bojner Horwitz, E., Sysoev, O., Jacobsson, B., Jönsson, J. I., Melén, E., et al. (2021). Clinical translation of genomic medicine. Lakartidningen, 118.
Giordano, S., Gallo, E., Diaferia, C., Rosa, E., Carrese, B., Borbone, N., et al. (2023). Multicomponent peptide-based hydrogels containing chemical functional groups as innovative platforms for biotechnological applications. Gels Basel, Switz. 9 (11), 903. doi:10.3390/gels9110903
Guillamet, C. V., and Kollef, M. H. (2024). Is zero ventilator-associated pneumonia achievable? Updated practical approaches to ventilator-associated pneumonia prevention. Infect. Dis. Clin. N. Am. 38 (1), 65–86. doi:10.1016/j.idc.2023.11.001
Jiang, T., Yang, T., Bao, Q., Sun, W., Yang, M., and Mao, C. (2022). Construction of tissue-customized hydrogels from cross-linkable materials for effective tissue regeneration. J. Mater. Chem. B 10 (25), 4741–4758. doi:10.1039/d1tb01935j
Lee, K. Z., Jeon, J., Jiang, B., Subramani, S. V., Li, J., and Zhang, F. (2023). Protein-based hydrogels and their biomedical applications. Mol. Basel, Switz. 28 (13), 4988. doi:10.3390/molecules28134988
Li, Y., Han, Y., Li, H., Niu, X., Zhang, D., and Wang, K. (2024). Antimicrobial hydrogels: potential materials for medical application. Small Weinheim der Bergstrasse, Ger. 20 (5), e2304047. doi:10.1002/smll.202304047
Li, Y. P., Liu, W., Liu, Y. H., Ren, Y., Wang, Z. G., Zhao, B., et al. (2020). Highly improved aqueous lubrication of polymer surface by noncovalently bonding hyaluronic acid-based hydration layer for endotracheal intubation. Biomaterials 262, 120336. doi:10.1016/j.biomaterials.2020.120336
Liu, S., Yu, Q., Guo, R., Chen, K., Xia, J., Guo, Z., et al. (2023). A biodegradable, adhesive, and stretchable hydrogel and potential applications for allergic rhinitis and epistaxis. Adv. Healthc. Mater. 12 (29), e2302059. doi:10.1002/adhm.202302059
Loftus, T. J., Ungaro, R., Dirain, M., Efron, P. A., Mazer, M. B., Remy, K. E., et al. (2021). Overlapping but disparate inflammatory and immunosuppressive responses to SARS-CoV-2 and bacterial sepsis: an immunological time course Analysis. Front. Immunol. 12, 792448. doi:10.3389/fimmu.2021.792448
Min, K., and Tae, G. (2023). Cellular infiltration in an injectable sulfated cellulose nanocrystal hydrogel and efficient angiogenesis by VEGF loading. Biomaterials Res. 27 (1), 28. doi:10.1186/s40824-023-00373-y
Motowski, H., Ilges, D., Hampton, N., Kollef, M. H., and Micek, S. T. (2023). Determinants of mortality for ventilated hospital-acquired pneumonia and ventilator-associated pneumonia. Crit. care Explor. 5 (3), e0867. doi:10.1097/cce.0000000000000867
Na, N., Guo, S. L., Zhang, Y. Y., Ye, M., Zhang, N., Wu, G. X., et al. (2021). Value of refined care in patients with acute exacerbation of chronic obstructive pulmonary disease. World J. Clin. cases 9 (21), 5840–5849. doi:10.12998/wjcc.v9.i21.5840
Patel, A. K., and Jain, S. K. (2015). S.S., A. Biodegradable polymeric nanoparticles for drug delivery to the lungs. Pharm. Res. doi:10.1007/s11095-014-1545-1
Pepelanova, I. (2021). Tunable hydrogels: introduction to the world of smart materials for biomedical applications. Adv. Biochem. engineering/biotechnology 178, 1–35. doi:10.1007/10_2021_168
Pierau, L., and Versace, D. L. (2021). Light and hydrogels: a new generation of antimicrobial materials. Mater. Basel, Switz. 14 (4), 787. doi:10.3390/ma14040787
Priddy-Arrington, T. R., Edwards, R. E., Colley, C. E., Nguyen, M. M., Hamilton-Adaire, T., and Caldorera-Moore, M. E. (2023). Characterization and optimization of injectable in situ crosslinked chitosan-genipin hydrogels. Macromol. Biosci. 23 (6), e2200505. doi:10.1002/mabi.202200505
Rouzé, A., and Nseir, S. (2022). Hospital-acquired pneumonia/ventilator-associated pneumonia and ventilator-associated tracheobronchitis in COVID-19. Seminars Respir. Crit. care Med. 43 (2), 243–247. doi:10.1055/s-0041-1740334
San Miguel-Pagola, M., Reychler, G., Cebrià, I. I. M. A., Gómez-Romero, M., Díaz-Gutiérrez, F., and Herrero-Cortina, B. (2020). Impact of hypertonic saline nebulisation combined with oscillatory positive expiratory pressure on sputum expectoration and related symptoms in cystic fibrosis: a randomised crossover trial. Physiotherapy 107, 243–251. doi:10.1016/j.physio.2019.11.001
Shen, C., Li, Y., and Meng, Q. (2022). Adhesive polyethylene glycol-based hydrogel patch for tissue repair. Colloids surfaces B, Biointerfaces 218, 112751. doi:10.1016/j.colsurfb.2022.112751
Sodhi, C. P., Nguyen, J., Yamaguchi, Y., Werts, A. D., Lu, P., Ladd, M. R., et al. (2019). A dynamic variation of pulmonary ACE2 is required to modulate neutrophilic inflammation in response to Pseudomonas aeruginosa lung infection in mice. J. Immunol. 203 (11), 3000–3012. doi:10.4049/jimmunol.1900579
Sommerstein, R., Merz, T. M., Berger, S., Kraemer, J. G., Marschall, J., and Hilty, M. (2019). Patterns in the longitudinal oropharyngeal microbiome evolution related to ventilator-associated pneumonia. Antimicrob. Resist. Infect. control 8, 81. doi:10.1186/s13756-019-0530-6
Strecanska, M., Danisovic, L., Ziaran, S., and Cehakova, M. (2022). The role of extracellular matrix and hydrogels in mesenchymal stem cell chondrogenesis and cartilage regeneration. Life Basel, Switz. 12 (12), 2066. doi:10.3390/life12122066
Suk, J. S., Xu, Q., Kim, N., Hanes, E., Ensign, M. L., Suh, H. D., et al. (2019). Mucus-penetrating nanoparticles for enhanced mucus clearance. Sci. Transl. Med. doi:10.1126/scitranslmed.aau8746
Sun, Z., Xiong, H., Lou, T., Liu, W., Xu, Y., Yu, S., et al. (2023). Multifunctional extracellular matrix hydrogel with self-healing properties and promoting angiogenesis as an immunoregulation platform for diabetic wound healing. Gels Basel, Switz. 9 (5), 381. doi:10.3390/gels9050381
Sunil, A. A., and Skaria, T. (2022). Novel regulators of airway epithelial barrier function during inflammation: potential targets for drug repurposing. Expert Opin. Ther. targets 26 (2), 119–132. doi:10.1080/14728222.2022.2035720
Tian, B., Liu, J., Guo, S., Li, A., and Wan, J. B. (2023). Macromolecule-based hydrogels nanoarchitectonics with mesenchymal stem cells for regenerative medicine: a review. Int. J. Biol. Macromol. 243, 125161. doi:10.1016/j.ijbiomac.2023.125161
Tran, T. S., Balu, R., Mettu, S., Roy Choudhury, N., and Dutta, N. K. (2022). 4D printing of hydrogels: innovation in material design and emerging smart systems for drug delivery. Pharm. Basel, Switz. 15 (10), 1282. doi:10.3390/ph15101282
Trapani, G., Weiß, M. S., and Trappmann, B. (2023). Tunable synthetic hydrogels to study angiogenic sprouting. Curr. Protoc. 3 (8), e859. doi:10.1002/cpz1.859
Ulldemolins, A., Jurado, A., Herranz-Diez, C., Gavara, N., Otero, J., Farré, R., et al. (2022). Lung extracellular matrix hydrogels-derived vesicles contribute to epithelial lung repair. Polymers 14 (22), 4907. doi:10.3390/polym14224907
Vanderslice, E. J., Golding, S. G. H., and Jacot, J. G. (2024). Vascularization of PEGylated fibrin hydrogels increases the proliferation of human iPSC-cardiomyocytes. J. Biomed. Mater. Res. Part A 112 (4), 625–634. doi:10.1002/jbm.a.37662
Wang, F., Qin, Z., Lu, H., He, S., Luo, J., Jin, C., et al. (2019). Clinical translation of gene medicine. J. gene Med. 21 (7), e3108. doi:10.1002/jgm.3108
Wang, S. J., Jing, X., Mi, H. Y., Chen, Z., Zou, J., Liu, Z. H., et al. (2022). Development and applications of hydrogel-based triboelectric nanogenerators: a mini-review. Polymers 14 (7), 1452. doi:10.3390/polym14071452
Wang, Y., Wang, Y., Mushtaq, R. T., and Wei, Q. (2024). Advancements in soft robotics: a comprehensive review on actuation methods, materials, and applications. Polymers 16 (8), 1087. doi:10.3390/polym16081087
Wei, Q., Young, J., Holle, A., Li, J., Bieback, K., Inman, G., et al. (2020). Soft hydrogels for balancing cell proliferation and differentiation. ACS biomaterials Sci. and Eng. 6 (8), 4687–4701. doi:10.1021/acsbiomaterials.0c00854
Xu, Y., Lai, C., Xu, G., Meng, W., Zhang, J., Hou, H., et al. (2019). Risk factors of ventilator-associated pneumonia in elderly patients receiving mechanical ventilation. Clin. interventions aging 14, 1027–1038. doi:10.2147/cia.S197146
Zhang, J., Wang, J., and Li, H. (2020). Hydrogel-based delivery of growth factors for tissue regeneration in the lung. Biomaterials Sci.
Keywords: biomaterials, hydrogels, airway lung injury, ventilator-associated, pneumonia
Citation: Xu Y and Zhao B (2025) Impact of hydrogel-based airway management strategies on ventilator-associated pneumonia: clinical applications and future directions. Front. Mater. 12:1530524. doi: 10.3389/fmats.2025.1530524
Received: 19 November 2024; Accepted: 17 February 2025;
Published: 28 March 2025.
Edited by:
Ceren Karaman, Akdeniz University, TürkiyeReviewed by:
Manisha Singh, Massachusetts Institute of Technology, United StatesCopyright © 2025 Xu and Zhao. This is an open-access article distributed under the terms of the Creative Commons Attribution License (CC BY). The use, distribution or reproduction in other forums is permitted, provided the original author(s) and the copyright owner(s) are credited and that the original publication in this journal is cited, in accordance with accepted academic practice. No use, distribution or reproduction is permitted which does not comply with these terms.
*Correspondence: Baisong Zhao, emhhb2JhaXNvbmc4MTlAc211LmVkdS5jbg==
Disclaimer: All claims expressed in this article are solely those of the authors and do not necessarily represent those of their affiliated organizations, or those of the publisher, the editors and the reviewers. Any product that may be evaluated in this article or claim that may be made by its manufacturer is not guaranteed or endorsed by the publisher.
Research integrity at Frontiers
Learn more about the work of our research integrity team to safeguard the quality of each article we publish.