- 1School of Chemistry and Chemical Engineering, South China University of Technology, Guangzhou, Guangdong, China
- 2School of Earth and Environment, Anhui University of Science & Technology, Huainan, Anhui, China
- 3School of Earth Resources and Materials Engineering, RWTH Aachen University, Aachen, North Rhine-Westphalia, Germany
In modern society, Organophosphorus flame retardants, as a class of widely used materials, have been extensively studied and applied across various fields. The diversity and uniqueness of phosphorus flame retardant, as well as the important role of its mechanism of action in the gas phase and condensed phase, determine that it is a good flame retardant material. In addition, the article highlights the range of applications of phosphorus flame retardants in a variety of materials, including polymers, coatings, fibers, and more. At the same time, the importance and future prospects of phosphorus flame retardant research are pointed out, and the development idea of organophosphorus flame retardant is proposed through the development of phosphorus flame retardant with a new structure and the in-depth study of the properties of different aspects of phosphorus flame retardant, which can provide strong support for the development of flame retardant technology.
1 Introduction: The critical role of phosphorus-based flame retardants and research background
Fires can result in significant loss of life and property, profoundly impacting social stability and sustainable development. In this context, the development of flame retardant (FR) technology becomes particularly important, as it can effectively delay combustion and slow the spread of fire, thereby allowing more time for evacuation and rescue, ultimately reducing fire-related losses.
Flame retardants (FRs) are typically added as functional additives to various manufactured products to reduce material flammability, prevent fires, protect human lives, and minimize fire-related losses (Li et al., 2023). With the continuous development of the production economy, synthetic and natural polymer materials are now applied across almost every industrial sector, including electronics, home furnishings, and aerospace, with increasingly stringent requirements (He et al., 2019; Zhang Y. et al., 2017; Zhang S. et al., 2017; Wang et al., 2017a; Sang et al., 2016). However, due to the typical molecular structure of polymers (high hydrocarbon content), flammability becomes a significant drawback, limiting the further application of polymer materials. Therefore, developing flame-retardant, high-performance materials has become a critical research focus (Dasari et al., 2013), leading to the increasing interest in flame retardant research.
Until 2004, polybrominated diphenyl ethers (PBDEs) were the most widely used halogenated flame retardants. However, due to their persistence, bioaccumulation, and adverse effects on animal and human health, and with the growing awareness of environmental protection, the use of halogenated flame retardants has been restricted. These flame retardants generate toxic and corrosive gases that endanger life and harm human health (Chen et al., 2016a; Zhao et al., 2013; Chen et al., 2016b; Jian et al., 2014; Tang et al., 2012; Li et al., 2012; Pan Y. et al., 2014; Lin et al., 2018; Xiao et al., 2016). As a result, halogenated flame retardants are being gradually phased out due to their toxicity and environmental impact (Lyche et al., 2015). Phosphorus-based flame retardants have thus gained attention for their efficiency, halogen-free nature, and environmental friendliness (Lu and Hamerton, 2002; Amarnath et al., 2018; Wang et al., 2015a). They are considered the best alternatives to halogenated flame retardants in terms of performance and cost (Chen et al., 2016a; Chen et al., 2016b; Xiao et al., 2016; Zhou et al., 2015; Xiao et al., 2014; Shi et al., 2017). Compared to nitrogen-based flame retardants, phosphorus-based variants exhibit superior char-forming efficiency in the condensed phase. Unlike silicon-based flame retardants, which rely on inert gas release, phosphorus compounds act synergistically in both gas and condensed phases. However, challenges such as moisture sensitivity and processing compatibility persist for phosphorus-based systems.
Recently, organophosphorus flame retardants (OPFRs) have become a common substitute for polybrominated diphenyl ethers. OPFRs are now used in construction materials, furniture plasticizers, textiles, electronics, and rubber products (Horrocks et al., 2016; Li X. et al., 2022; Zeng et al., 2018). Among them, triphenyl phosphate (TPHP) is one of the most popular OPFRs due to its low cost and excellent flame retardant properties (van der Veen and de Boer, 2012). The mechanisms of phosphorus in flame retardancy, coupled with the performance advantages of phosphorus-based flame retardants, make them an important research direction in the field of fire-resistant materials. With the increasing recognition of the toxicity and environmental persistence of traditional flame retardants, the development of new organophosphorus alternatives has reached unprecedented levels (Velencoso et al., 2018).
Phosphorus has a long history of application in the flame retardant field, and its diverse compounds and structures provide a wide variety of phosphorus-based flame retardants. Organophosphorus flame retardants, such as phosphate esters, phosphonates, and phosphoramides, are widely used in various materials due to their highly tunable structure and performance. Inorganic phosphorus flame retardants, such as phosphorus oxides and phosphorus-nitrogen compounds, provide new pathways for enhancing flame retardancy through unique reaction mechanisms. The mechanism of action of phosphorus-based flame retardants encompasses two primary aspects: gas-phase action and condensed-phase action. This will be explained in more detail later in the section 4.
The application of phosphorus-based flame retardants is not limited to specific fields but encompasses a broad range of materials, including polymers, coatings, and fibers. Introducing phosphorus-based flame retardants into these materials can significantly enhance their fire resistance, thereby increasing safety. The varying fire resistance requirements and performance characteristics of different materials also offer diverse opportunities for the application of phosphorus-based flame retardants.
As society increasingly emphasizes fire safety, the development prospects for flame retardant technology are becoming broader. Phosphorus-based flame retardants, as an important class of flame-retardant materials, will provide more options and possibilities for improving fire resistance through continuous innovation and in-depth research. By thoroughly investigating the types of phosphorus-based flame retardants, synthesis methods, mechanisms of action, and their applications in various materials, researchers can provide strong support for breakthroughs and innovations in flame retardant technology.
This paper will focus on phosphorus-based flame retardants, exploring their applications in various materials and the post-use disposal methods. By gaining a deeper understanding of the characteristics and effects of phosphorus-based flame retardants, researchers can better grasp the development trends of flame retardant technology, provide solid scientific support for constructing safer and more sustainable material applications, and offer in-depth insights and references for the advancement of flame retardant technologies.
2 Diversity and uniqueness of phosphorus-based flame retardants
Phosphorus-based flame retardants can be broadly categorized into two main types based on their composition and structure: inorganic phosphorus flame retardants and organic phosphorus flame retardants. Inorganic phosphorus flame retardants include compounds such as red phosphorus, ammonium phosphates, and polyphosphoric ammonium salts. Organic phosphorus flame retardants consist of compounds like phosphates, phosphonates, phosphinates, and phosphonium salts (Zhou et al., 2009).
Inorganic phosphorus flame retardants have a long history of use and include red phosphorus, ammonium polyphosphate (APP), and ammonium dihydrogen phosphate, among others. These phosphates decompose under heat to release phosphoric acid and polyphosphoric acid, which promote char formation on the surface of the substrate, providing a flame-retardant barrier. These flame retardants are widely applied in materials such as polyvinyl chloride (PVC), nylon, epoxy resins, polyester, and polyamides, with particularly common usage in the latter two materials. As an established class of flame retardants, they hold a prominent position in inorganic flame retardants due to their advantages, including being halogen-free, low-toxicity, stable, and offering long-lasting effectiveness.
Since the introduction of red phosphorus flame retardants by Bayer AG in 1963, researchers have continuously explored methods to enhance the stability of red phosphorus as a plastic flame retardant (Hansheng, 2002). Organic phosphorus flame retardants, often used as substitutes for brominated flame retardants, are widely applied in industrial production and everyday life. These compounds function primarily as additive-type flame retardants by being incorporated into flammable materials to inhibit combustion.
As a critical class of fire-protection materials, phosphorus-based flame retardants hold a significant place in the field due to their diversity and unique properties. Phosphorus possesses a rich set of chemical characteristics, allowing phosphorus-based flame retardants to exhibit various forms depending on their structure and intended application, thereby providing excellent flame retardant properties.
The diversity of phosphorus-based flame retardants arises from the abundant forms of phosphorus compounds and the multiple chemical reaction pathways available. These diverse phosphorus compounds demonstrate unique characteristics in flame retardant mechanisms and application directions. Compared to halogenated flame retardants, phosphorus-based flame retardants offer several advantages, such as diverse structural design, high flame retardant efficiency, and better environmental friendliness. For example, the structural design flexibility of phosphorus-based flame retardants is exemplified by Dihydro-9-oxa-10-phosphaphenanthrene-10-oxide (DOPO) derivatives, where functional groups (e.g., −OH, −NH2) can be introduced to tailor compatibility with polymers. Environmentally, phosphorus flame retardants avoid persistent organic pollutants (POPs) associated with halogenated systems, as demonstrated by their lower bioaccumulation potential (BCF <100) in aquatic organisms. As such, they have garnered considerable attention in recent years as halogen-free flame retardants.
Inorganic phosphorus flame retardants include substances like ammonium polyphosphate (APP) and red phosphorus, while organic phosphorus flame retardants can be subdivided based on different substituents into alkyl compounds, chlorinated compounds, aryl compounds, and phosphonate diesters, among others (He et al., 2020; Wang et al., 2020; Feng et al., 2017; Li et al., 2016). Table 1 presents commonly studied inorganic phosphorus flame retardants, and Table 2 highlights frequently researched organic phosphorus flame retardants. In addition to fundamental information about flame retardants, their molecular weight and density are critical parameters for predicting dispersibility within polymer matrices and assessing flame-retardant efficiency. For instance, low-density additives, such as TPrP (density: 1.01 g/cm3), facilitate uniform mixing in low-viscosity resins due to their favorable physical properties. Consequently, Table 1, 2 also provide detailed data on the molecular weights and densities of common inorganic or organic phosphorus-based flame retardants, which are essential for optimizing material formulations and performance evaluations.
Due to the distinct chemical properties of phosphorus, phosphorus-based FRs can exert flame-retardant effects through various mechanisms, including gas-phase and condensed-phase actions. In gas-phase action, phosphorus flame retardants decompose and generate phosphorus oxoacids, which slow down the spread of flames. In condensed-phase action, phosphorus forms a dense char layer on the surface of the material, which isolates it from oxygen and heat, thus achieving flame retardancy.
Phosphorus flame retardants generally possess excellent thermal stability, enabling them to maintain their flame-retardant properties under high-temperature conditions. This makes them particularly valuable in high-temperature materials and electronic devices. Some organic phosphorus flame retardants, due to their structural characteristics, exhibit certain biodegradability, which helps to reduce environmental impact. While certain organic phosphorus flame retardants (e.g., phytic acid) exhibit biodegradability under aerobic conditions, others like triphenyl phosphate (TPHP) persist in anaerobic environments. For instance, the findings by Hong et al. (2021) indicate that both tris(1,3-dichloro-2-propyl) phosphate (TDCIPP) and TPHP treatments resulted in a reduction in body length and pectoral fin length in pufferfish (Takifugu spp.), accompanied by symptoms of black scattering light. Additionally, these chemicals caused deformities in the pectoral fins of first-generation larvae and induced spinal curvature in adult fish. These results suggest that TDCIPP and TPHP may pose potential risks to marine fish populations and broader marine ecosystems.
2.1 Inorganic phosphorus flame retardants
The advantages of inorganic phosphorus flame retardants include excellent thermal stability, non-volatility, non-corrosive gas production, good flame-retardant effects, electrical insulation properties, low toxicity, insolubility, and high melting points. These features make them highly effective in enhancing the flame-retardant performance of materials, especially in resins, while preserving the physical and chemical properties of the base materials. Moreover, inorganic phosphorus flame retardants are characterized by low toxicity (LD50 > 2000 mg/kg) and significantly reduce smoke generation compared to halogenated alternatives, which has led to their increasing application in flame-retardant materials.
2.1.1 Red phosphorus
Red phosphorus is characterized by high phosphorus content, low dosage, and good flame-retardant efficiency. Besides, red phosphorus is stable under ambient conditions but ignites at temperatures exceeding 260°C in oxygen-rich environments. Untreated red phosphorus exhibits moisture absorption rates of 2%–5%, whereas surface-modified variants (e.g., Al(OH)3-coated red phosphorus) reduce absorption to <1%. However, it may releases toxic gases during combustion, which limits its applications (Sang et al., 2014; Hong et al., 2012). Combustion of red phosphorus primarily releases phosphine (PH3), a toxic gas requiring stringent ventilation in industrial settings. Significant research has focused on the modification and pretreatment of red phosphorus, including aluminum hydroxide-encapsulated red phosphorus, melamine-coated red phosphorus (Li et al., 2013), and magnesium hydroxide-coated red phosphorus (Yuye, 2014).
Another advancement is microencapsulation of red phosphorus, where a layer of inorganic material or polymer resin film is coated on the surface of red phosphorus through physical or chemical methods. When the material burns, the microcapsule ruptures, releasing the phosphorus flame retardant (Nana et al., 2013). Microencapsulation techniques have significantly advanced flame retardancy, as demonstrated by recent studies. For instance, Li et al. (2025) reported in their research that microencapsulated red phosphorus enhances both the flame retardancy and acid-base resistance of polypropylene/aluminum hydroxide composites. Their findings elucidate the mechanism behind microencapsulated flame retardation, highlighting that red phosphorus primarily functions in the gas phase. During combustion, the generated PO· radicals effectively capture H· and HO· radicals, thereby terminating the chain reaction of combustion. This innovative approach not only improves material safety but also extends the applicability of such composites in various industrial contexts. The development of highly efficient microencapsulated red phosphorus flame retardants broadens the application scope of phosphorus-based flame retardants (Zhou et al., 2009).
Red phosphorus exhibits thermal stability up to 260°C in air, while microencapsulated variants (e.g., phenolic resin-coated red phosphorus) resist decomposition until 300°C. In contrast, ammonium polyphosphate (APP) degrades at 250°C–300°C, releasing ammonia and forming polyphosphoric acid.
Huaichun et al. (2011) utilized in situ polymerization to prepare ultrafine microencapsulated red phosphorus (MRP) flame retardants with an average particle size of 7.5 µm, using phenolic resin (PF) as the capsule wall and red phosphorus as the core. High-density polyethylene (PE-HD) modified with ethylene-vinyl acetate copolymer (EVAC) was employed as the base resin for cable materials, and the MRP was used along with carbon black to enhance the flame retardancy and smoke suppression of the cable compound (Zhou et al., 2009).
Chang et al. (2012) developed a novel double-layer microencapsulated red phosphorus (DMRP), using aluminum hydroxide and melamine resin as the encapsulation materials, and applied it to polylactic acid (PLA). This DMRP raised the limiting oxygen index (LOI) of PLA from 20.5% to 29.3%, improved the material’s ignition point, reduced its moisture absorption, and exhibited better flame-retardant effects than traditional MRP or uncoated red phosphorus, achieving UL94V-0 rating (Zhou et al., 2009).
He (2008) used aluminum hydroxide as the encapsulation material for red phosphorus microencapsulation and optimized the encapsulation conditions through orthogonal experiments. The treated red phosphorus exhibited enhanced oxidation resistance, reduced moisture absorption, slower thermal degradation, and improved flame-retardant performance (Zhou et al., 2009).
2.1.2 Black phosphorus
Although black phosphorus (BP) and red phosphorus are allotropes of phosphorus, they exhibit distinct differences in physical and chemical properties. Notably, due to its two-dimensional structure and characteristics as a nanoscale flame retardant, recent extensive research on BP has revealed and validated its unique structural features, gradually gaining recognition among scientists worldwide (Chen et al., 2020; Koenig et al., 2016; Wang Y. et al., 2019).
In experimental studies, BP is commonly synthesized from amorphous red phosphorus powder through various chemical methods. The primary preparation techniques for bulk BP include high-pressure synthesis (Antonatos et al., 2020), bismuth flux method (Maruyama et al., 1981), high-energy mechanical milling (Ferrara et al., 2019), chemical vapor transport (CVT) (Nilges et al., 2008), and microwave-assisted liquid-phase synthesis (He D. et al., 2018), among others. These methods enable the controlled production of BP with tailored properties for diverse applications.
Zhang et al. (2023) reported an isomer of red phosphorus used as an example of inorganic phosphorus flame retardants, specifically few-layer black phosphorus. They created model materials to compare the flame-retardant performance of few-layer black phosphorus, nano-red phosphorus, and triphenyl phosphate in cellulose and polyacrylonitrile. Few-layer black phosphorus exhibited superior flame-retardant performance compared to the other additives, with its flame-retardant efficiency being approximately 1.8 times that of nano-red phosphorus and about 4.4 times that of triphenyl phosphate. The enthalpy of combustion for few-layer black phosphorus (−127.1 kJ/mol) was only one-third that of red phosphorus nanoparticles (−381.3 kJ/mol). Through combined thermodynamic, spectroscopic, and microscopic analyses, they attributed the superior flame retardancy of few-layer black phosphorus to its two-dimensional structure and thermal properties, which effectively inhibited combustion reactions.
Recently, Duan et al. (2025) reported the synthesis of crystalline phosphorene nanosheets (Crystal-PNS) via a solvothermal method using amorphous red phosphorus and amines as raw materials. Through a one-pot approach, Crystal-PNS with an average lateral size of 200 nm and thickness of 15 nm was produced at a yield exceeding 100 g, achieving a remarkable production efficiency of over 95%. The study systematically investigated the mechanism by which amines promote structural transformation and the transition from amorphous to crystalline states, proposing a macroscopic active amine shielding model. Due to its weak surface hydrophilicity, Crystal-PNS exhibited superior stability under various environmental conditions, including ambient atmosphere, humid air, aqueous solutions, and light irradiation. Compared to emerging high-efficiency flame-retardant materials such as BPNS (black phosphorus nanosheets), Crystal-PNS demonstrated enhanced flame-retardant performance. This amine-driven structural transformation paves the way for low-cost and large-scale production of nanomaterials. Stable phosphorus allotropes like Crystal-PNS hold promising potential for broader applications across diverse fields.
2.1.3 Aluminum hypophosphite
Metal hypophosphite compounds are considered an emerging class of phosphorus-based flame retardants (Li et al., 2012; Xiao et al., 2016; Zhao et al., 2012). Aluminum hypophosphite (AlHP) is a novel flame-retardant filler that can enhance the flame-retardant performance of polymers. It is recognized as an environmentally friendly and cost-effective flame retardant (Chen et al., 2016a; Zhao et al., 2013; Chen et al., 2016b). Existing literature has investigated the flame-retardant effects of AlHP in various polymers, including polylactic acid (PLA), thermoplastic polyurethane (TPU), polyethylene (PE), polystyrene (PS), polybutylene terephthalate (PBT), polyethylene terephthalate (PET), and polyamide 6 (PA6) (Chen et al., 2016a; Zhao et al., 2013; Chen et al., 2016b; Jian et al., 2014; Tang et al., 2012; Li et al., 2012; Pan Y. et al., 2014; Lin et al., 2018; Xiao et al., 2016; Zhou et al., 2015; Xiao et al., 2014; Shi et al., 2017; Zhao et al., 2012).
Tang et al. (2012) studied the effects of different concentrations of AlHP (10, 20, and 30 wt%) on the flammability and mechanical properties of PLA. The results indicated that the incorporation of AlHP into PLA improved its flame-retardant performance. Specifically, when 20 wt% of AlHP was added to PLA, the Limiting Oxygen Index (LOI) value increased from 19.5% to 28.5%, and the composite material achieved a V0 rating. The tensile strength and elongation at break values of FR-PLA composites are illustrated in Figure 1 (Tang et al., 2012). Pure PLA exhibits a high tensile strength of 61.1 MPa and an elongation at break of 3.7%. Upon the addition of 10 wt% AHP, the tensile strength decreases to 42.8 MPa, representing a reduction of approximately 30% compared to pure PLA. Further increases in AHP loading result in slight declines in tensile strength, with PLA/20AHP and PLA/30AHP demonstrating tensile strengths of 40.6 MPa and 32.4 MPa, respectively. Additionally, as the AHP content rises, the elongation at break progressively diminishes due to the rigidity of AHP particles. Although the mechanical properties of FR-PLA composites decline with increasing AHP loading, these composites still retain favorable mechanical performance overall.
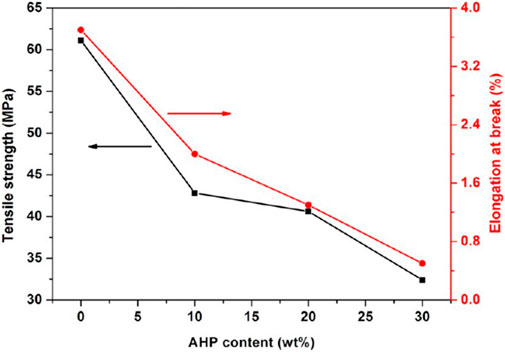
Figure 1. Effect of AHP loading on the mechanical properties of FR-PLA composites (Tang et al., 2012).
Liu and Gao (2018) investigated the synergistic flame-retardant effect of AlHP with a novel intumescent flame-retardant system in PLA. Using isocyanuric acid tri-glycerol ether and diethylenetriamine as raw materials, a hyperbranched carbonization agent (CT) was synthesized in water, forming a new intumescent flame-retardant (IFR) system in conjunction with ammonium polyphosphate (APP). The results revealed that when APP/CT (4:1) was set at 30% and IFR/AHP (3:1) at 20% (by mass), the oxygen index of PLA reached 41.2% and 43.5%, respectively, both achieving UL-94 V-0 level without dripping. The combination of IFR and AHP significantly reduced the heat release rate (HRR), maximum heat release rate (MHRR), and average mass loss rate, while considerably enhancing the thermal stability of PLA.
Xiao et al. (2014) examined the flammability of AlHP containing TPU at load ratios of 25–35 wt%. They concluded that a flame-retardant TPU with a V0 rating was achieved when 30 wt% of AlHP was added. The incorporation of AlHP affects the tensile strength of TPU. At a content of 25 wt%, the tensile strength reaches approximately 26 MPa. However, further increasing the AlHP content to 30 wt% does not significantly alter the tensile strength. The trend in elongation at break aligns with that of tensile strength: upon adding AlHP, the elongation at break for TPU/AP25 and TPU/AP30 slightly decreases to 818% and 779%, respectively. This minor decline in elongation at break indicates that TPU retains its elastomeric properties even with the inclusion of AlHP (Savas et al., 2020).
Li et al. (2012) investigated the flame-retardant and thermal degradation behavior of PA6 containing magnesium hypophosphite (MgHP) and 18–28 wt% of AlHP. They observed a significant difference in flame-retardant efficiency between MgHP and AlHP. Although PA6 with 18 wt% AlHP achieved the highest UL-94 V0 rating, the composites with MgHP did not reach V0 even at the maximum loading level of 28 wt%. Jian et al. (2014) explored the application of AlHP as a flame-retardant additive in acrylonitrile-butadiene-styrene (ABS). The LOI value increased from 18.5 to 24.1, and the UL-94 rating improved from N.R. (no rating) to V0 with the addition of 25 weight percent of AlHP.
2.1.4 Ammonium polyphosphate
As a common high-nitrogen phosphorus-based flame retardant (Lim et al., 2016), ammonium polyphosphate (APP) is frequently added as an additive (Cai et al., 2006; Camino et al., 1984; Ardanuy and Velasco, 2011) or utilized in the formation of intumescent flame retardants to enhance flame retardancy (Wang et al., 2015b; Shao et al., 2014; Pallmann et al., 2019; Chen et al., 2019; Feng et al., 2016; He W. et al., 2018; Liu et al., 2020; Wu et al., 2008; Dong et al., 2019; Enescu et al., 2013; Jiang and Liu, 2015; Sun et al., 2020). APP is a long-chain polyphosphate that was first synthesized in 1857 through the reaction of phosphorus and ammonia and was used as a forest firefighting agent. Due to its excellent flame-retardant capabilities, it is widely used for flame-retardant treatments in coatings, plastics, fabrics, and wood (Nguyen et al., 2021; Usri et al., 2021). Compared to halogenated flame retardants, APP offers advantages such as low cost and low toxicity. Researchers have utilized APP to replace metal hydroxide flame retardants in epoxy resins to prepare flame-retardant composites, which have resulted in a reduction in composite density and improved flame retardant efficacy (Liu Y. et al., 2022; Wan et al., 2020). The incorporation of APP into rubber materials such as natural rubber has also significantly enhanced flame retardancy (Wan et al., 2020). Currently, extensive research has been conducted on the flame-retardant behavior of APP in ethylene-propylene-diene monomer (EPDM) rubber. However, most studies on the flame-retardant mechanism of APP in EPDM have been conducted in oxygen-rich environments, with limited research under oxygen-deficient conditions. Given that the working environment of solid rocket motors (SRM) is almost always oxygen-deficient, understanding the flame-retardant mechanism of APP in EPDM under these conditions is crucial for developing composites that meet the performance requirements of thermal insulation layers in practical applications (Chen et al., 2024).
Chen et al. (2024) reported the preparation of a composite material using APP as a flame retardant, filled into a ternary ethylene-propylene rubber (p-EPDM) premix containing fumed silica and aluminum hydroxide. By establishing an oxygen-deficient environment, the flame-retardant performance of APP in EPDM was investigated. The results indicated that the composites made with APP as a flame retardant exhibited good flame retardant performance in terms of limiting oxygen index and UL-94 tests, with minimal impact on mechanical strength and density. In oxygen-deficient environments, APP undergoes thermal decomposition, producing ammonia, water vapor, and phosphoric acid-containing substances. These gases dilute combustible gases and reduce thermal conductivity. The phosphoric acid-containing substances accumulate on the surface of the pyrolysis layer, promoting the formation of a carbonaceous layer. This provides guidance for designing thermal insulation materials that better meet practical usage requirements.
Huang et al. (2021) reported the encapsulation of ammonium polyphosphate in melamine-formaldehyde resin and sodium silicate using in situ polymerization, creating a flame-retardant polypropylene containing a microcapsule core-shell structure. The composition of the ammonium polyphosphate, melamine-formaldehyde resin-encapsulated ammonium polyphosphate, and microcapsule flame retardant was characterized. The modified flame retardancy and thermal stability were studied through testing. The results demonstrated a significant improvement in flame-retardant performance after modification, with polyamide 6 exhibiting a good char-forming effect. Additionally, the water solubility of the flame retardant and its effects on the mechanical properties of polypropylene were investigated, showing that the flame retardant effectively enhanced the flame-retardant properties of polypropylene.
2.2 Organic phosphorus flame retardants
Currently, research on organic phosphorus flame retardants primarily focuses on phosphates, phosphonates, phosphine oxides, polyphosphate esters, hypophosphites, and organic phosphonates. Phosphate esters (R-O-PO(OR)2) act via gas-phase radical quenching, whereas phosphonate esters (R-PO(OR)2) form stable P-O-C bonds in the condensed phase, enhancing char integrity. Phosphate flame retardants are widely used due to their abundance and low cost. These include phosphate esters, nitrogen-containing phosphate esters, and halogenated phosphate esters, most of which are categorized as additive flame retardants, possessing both flame-retardant and plasticizing functions. Similar to the previous section, this section will highlight a few representative organic phosphorus flame retardants, including their current research status and recent improvements made by researchers.
It is essential to recognize that, unlike inorganic phosphorus flame retardants, the use of organic phosphorus flame retardants must consider their potential environmental pollution and toxicity to living organisms when added to certain materials. Some organic phosphorus flame retardants are difficult to degrade, leading to their accumulation in the environment, entering the food chain and ecological cycle, ultimately threatening both environmental and human health. Therefore, while evaluating the flame-retardant performance of organic phosphorus flame retardants, their toxicity and pollution must also be considered. A more detailed discussion on toxicity is provided in Chapter 5 of this paper.
2.2.1 Triphenyl phosphate
Triphenyl phosphate (TPP) is an organic phosphorus ester flame retardant and plasticizer that is added to various consumer and industrial products (Germain and Winn, 2024). TPP is mainly used as a flame-retardant plasticizer for engineering plastics and phenolic resin laminates, as a softener for synthetic rubber, and as a raw material for the production of trimethyl phosphate. It can also be used as a plasticizer for nitrocellulose and cellulose acetate, a flame-retardant plasticizer, a fire-resistant solvent, a nitrocellulose paint, synthetic resin, an impregnating agent for roofing paper, and a substitute for camphor in the manufacture of celluloid.
Zhang et al. (2016) studied the effects of triphenyl phosphate on the suspension polymerization process of styrene and the flame-retardant properties of polystyrene/TPP nanocomposites. They found that TPP could completely dissolve in the styrene monomer. TPP forms spherical nanoparticles that are evenly distributed within the polystyrene (PS) matrix. The article also explored the formation mechanism of TPP nanoparticles. Additionally, the influence of TPP on the styrene polymerization process was examined, revealing that TPP promotes styrene polymerization. The PS/TPP nanocomposite exhibited excellent flame-retardant properties due to the dispersion of nanometer-sized TPP particles in its matrix. In conclusion, they found that suspension polymerization provides a simple method for preparing PS/TPP nanocomposites with improved performance.
Wu et al. (2018a) developed a flame-retardant polymer composite filled with triphenyl phosphate sealed in natural microtubes. As mentioned earlier, polymer compounds, especially hydrocarbon polymers, exhibit excellent properties and are widely used across various industries. However, their application is limited by their inherent flammability. When exposed to external heat sources, these polymers absorb heat and undergo endothermic pyrolysis reactions, resulting in the production of combustible gases and liquids. The enormous heat released can initiate a continuous process that often leads to fires (Chen and Wang, 2010; Braun et al., 2006; Bar et al., 2015; Pan M. et al., 2014; Gu et al., 2013), necessitating the addition of suitable flame retardants to enhance their overall performance and utility. This study addressed the issue by filling and sealing triphenyl phosphate into natural microtubes approximately 100 µm long, with diameters of 10–20 µm and wall thicknesses of 0.5–1.0 µm. Morphological observations and X-ray photoelectron spectroscopy analyses were conducted to investigate the sealing mechanism. When the mass ratio of triphenyl phosphate to cellulose acetate was 97:3, the mixture exhibited good encapsulation effects, ensuring that triphenyl phosphate would not leak from the microtubes during the curing process, achieving an encapsulation rate of 56%. Incorporating the filled microtubes into epoxy resin demonstrated no significant damage to the hardness and strength of the matrix. During combustion, the microtubes effectively increased char formation and reduced smoke release. This self-sealing method could be applied to other applications, such as self-repairing composites.
2.2.2 Trimethyl phosphate
Bolshova et al. (2016) proposed a skeletal mechanism for the flame retardancy of trimethyl phosphate based on a multi-step kinetic mechanism involving over 200 reactions of organic phosphorus compounds. This mechanism consists of 22 irreversible elementary reactions involving nine phosphorus-containing species. By simulating the results and conducting experimental measurements, comparisons were made with H2/O2, CH4/O2, and synthetic gas/air flames doped with trimethyl phosphate to validate the proposed mechanism.
Knyazkov et al. (2021) investigated the laminar burning velocity and structure of near-stoichiometric premixed laminar flames of methyl methacrylate (MMA) with and without the addition of trimethyl phosphate (TMP) through experiments and numerical simulations. Using the MMA + TMP combustion system as a model, they simulated the gas-phase combustion process of polymethyl methacrylate (PMMA) containing phosphorus flame retardants. Flame sampling molecular beam mass spectrometry was employed to measure the spatial variation of molar fractions of H, OH, PO, PO2, HOPO, HOPO2, and various intermediate hydrocarbons in a stabilized one-dimensional burner flame. The influence of TMP on the intermediate products of hydrocarbons in the flame was studied. Based on new laminar burning velocities and chemical form data, the reaction mechanism of the MMA + TMP system was validated. The performance and limitations of the flame-retardant kinetics of MMA were discussed. Sensitivity analyses indicated that the predicted molar fractions of H, O, and OH were not sufficiently accurate, resulting in inconsistencies in the distribution of molar fractions of hydrocarbons in the flame. Comparisons were made between the suppressive effects of TMP on MMA flames and experimental data from other fuels, discussing the observed trends.
2.2.3 Phospho-nitrile
Phosphazene-based flame retardants represent a class of highly efficient and environmentally friendly nitrogen-phosphorus flame-retardant materials, distinguished by their unique characteristics and advantages. Firstly, they exhibit exceptional flame-retardant efficacy. During combustion, phosphazene compounds release active substances containing phosphorus and nitrogen, which significantly inhibit flame propagation and combustion processes. Secondly, phosphazene flame retardants demonstrate excellent thermal stability. These compounds maintain their flame-retardant properties even at elevated temperatures due to their inherent thermal resistance. Moreover, they are characterized by low smoke emission and low toxicity. When burned, phosphazene flame retardants produce minimal smoke and toxic gases, thereby reducing environmental and human health risks. Additionally, phosphazene flame retardants offer multifunctionality. Beyond their primary role as flame retardants, they can also function as plasticizers and stabilizers, thus providing a versatile range of applications. These attributes collectively render phosphazene-based flame retardants a promising and sustainable choice for various industrial and material science applications.
Recently, Pan et al. (2024) reported a novel phosphazene-based flame retardant for cotton fabric, synthesized by grafting dopamine (DA) onto hexachlorocyclotriphosphazene (HCCP) through an acylation reaction, resulting in the formation of a new phosphazene flame retardant, hexadopamine cyclotriphosphazene (HDCCP). The pyrolysis behavior of HDCCP was characterized using thermogravimetric analysis (TGA), which revealed its excellent thermal stability. The influence of HDCCP at varying mass concentrations on the flame-retardant properties of cotton fabric was investigated through techniques such as X-ray diffraction (XRD), scanning electron microscopy (SEM), limiting oxygen index (LOI) testing, and TGA. The results demonstrated that when the mass fraction of HDCCP was 6%, the flame retardant not only exhibited optimal flame-retardant performance but also maintained superior mechanical properties.
In earlier research, Zhou et al. (2020) comprehensively reviewed the current state of polyphosphazene-based flame retardants. Polyphosphazenes are organic-inorganic polymers characterized by an alternating sequence of single and double bonds between phosphorus and nitrogen atoms along their backbone, endowing them with excellent inherent flame-retardant synergism and thermal stability. Leveraging the designable molecular structure and outstanding flame-retardant properties of polyphosphazenes, a series of linear and cross-linked polyphosphazene flame retardants have been synthesized to enhance the comprehensive performance of materials, particularly in terms of flame retardancy, thermal stability, and mechanical properties. Specifically, the review systematically discussed the molecular design and structural modifications of polyphosphazenes, their flame-retardant mechanisms, and the performance of flame-retardant materials.
2.2.4 Multi-element synergistic organophosphorus flame retardants
In recent years, the research approach of enhancing flame retardant performance through the synergistic effects of multiple flame-retardant elements has been increasingly prominent. Beyond effectively improving flame retardancy, these synergistic interactions can also mitigate the adverse impacts that single-element flame retardants may have on other material properties, thereby meeting the progressively stringent requirements for flame-retardant materials (Cheema et al., 2013; Liu J. et al., 2022; Wang C. et al., 2019; Wu et al., 2018b). Although phosphorus-nitrogen-based flame retardants exhibit significantly reduced smoke emission and toxicity compared to halogenated counterparts, their environmental impact remains a concern. Consequently, developers are now integrating more environmentally benign and non-toxic flame-retardant elements into synergistic systems to reduce biological toxicity and smoke emissions.
Boron-based flame retardants, known for their efficiency, low toxicity, non-irritating nature, and minimal smoke production, were among the earliest used in the flame-retardant finishing of cotton fabrics (Cheng et al., 2020; Wang et al., 2018; Wang et al., 2022; Xu et al., 2019). A novel approach involves incorporating organic borates into ammonium phosphate-based flame retardants to achieve durable, efficient, low-smoke, and low-toxicity characteristics. This strategy represents an innovative attempt to enhance the overall safety and effectiveness of flame retardants while addressing environmental concerns (Zhang et al., 2024).
Zhang et al. (2024) synthesized a reactive P/N/B spirocyclic compound—dimethylammonium methylenephosphonate borate (DMAPS), which imparts excellent flame retardant properties to cotton fabrics at low concentrations. After treatment with 100 g/L DMAPS, the limiting oxygen index (LOI) of the fabric increased to 31.6%, and the char residue length decreased to 5.9 cm. Further increasing the concentration of DMAPS resulted in an oxygen index of around 42.9%. Cone calorimeter tests revealed that the peak heat release rate (PHRR) of the DMAPS-treated cotton fabric was only 15 kW/m2, representing a reduction of approximately 92% compared to the untreated fabric. The total heat release (THR) also decreased from 5.0 to 0.9 MJ/m2, an 82% reduction compared to the untreated cotton. Char residue analysis indicated that the polyphosphoric acid formed during the pyrolysis of DMAPS promoted char formation, while the carbon-boron structure remained in the char, stabilizing the char layer and preserving the original warp and weft structure of the fabric. Additionally, the treated samples maintained flame retardancy and self-extinguishing properties after 10 washing cycles. This study provides a potential approach for the preparation of durable flame-retardant cotton fabrics.
Recently, the research team led by Zhang et al. (2025) developed a novel environmentally friendly antibacterial flame retardant and applied it to the durable antibacterial and flame-retardant finishing of cotton fabrics. The synergistic effect of phosphorus, nitrogen, and boron in the flame retardant facilitated excellent flame retardancy in cotton fabrics, while the halamine structure imparted superior antibacterial properties. The cotton fabric treated with this antibacterial flame retardant exhibited self-extinguishing properties, with an average burn length of approximately 65 mm and an oxygen index exceeding 31%. After activation by sodium hypochlorite bleaching, the logarithmic reduction values for Staphylococcus aureus and Escherichia coli both exceeded 5, indicating outstanding bactericidal performance. XPS, CCT, and TG-IR analyses revealed that the flame-retardant mechanism of the APBN-treated cotton fiber was predominantly in the condensed phase, with some contribution from the gas phase. On the basis of a comprehensive evaluation of antibacterial, flame-retardant, and physical properties, a composite antibacterial flame retardant was developed, yielding cotton fabrics with excellent antibacterial and durable flame-retardant properties.
3 Mechanism of action of phosphorus flame retardants
Phosphorus flame retardants exhibit different flame-retardant mechanisms due to the differences in oxidation states of phosphorus and coordination bonds (Yang et al., 2016; Yang et al., 2019; Xue et al., 2020; Ran et al., 2019). The mechanisms of action of phosphorus flame retardants encompass two main aspects: gas-phase action and condensed-phase action. In the gas phase, phosphorus compounds generated from the decomposition of phosphorus flame retardants can neutralize free radicals in the flame, inhibiting the propagation rate of the flame. In the condensed phase, phosphorus can form a layer of phosphorus compounds on the material’s surface, effectively isolating oxygen and heat, thereby providing flame-retardant effects.
3.1 Condensed-phase flame retardant mechanism
Phosphorus-based flame retardants generate phosphoric anhydride during combustion, which promotes the dehydration and carbonization of combustible materials. The formation of char reduces thermal conduction between the flame and the condensed phase, thereby preventing or delaying the production of combustible gases. For instance, Kim et al. (Kim et al., 2023) reported two flame retardants containing both phosphorus and nitrogen: N-(2-methoxyethyl)-N-methyl-P,P-diphenylphosphoryl amide (PNOFR) and 2-(dimethylamino)ethyl diphenyl phosphonate (PONFR) (as shown in Figure 2), which were applied to polyvinyl chloride (PVC). The flame-retardant mechanisms of PNOFR and PONFR exhibit typical characteristics of phosphorus and nitrogen-containing flame retardants. The results indicated that the primary flame-retardant mechanisms of PNOFR and PONFR vary due to the elements bonded to phosphorus in their chemical structures. In the condensed phase, the cross-linked char layer of PNOFR is denser and contains a higher phosphorus content compared to the cross-linked char layer of PONFR.
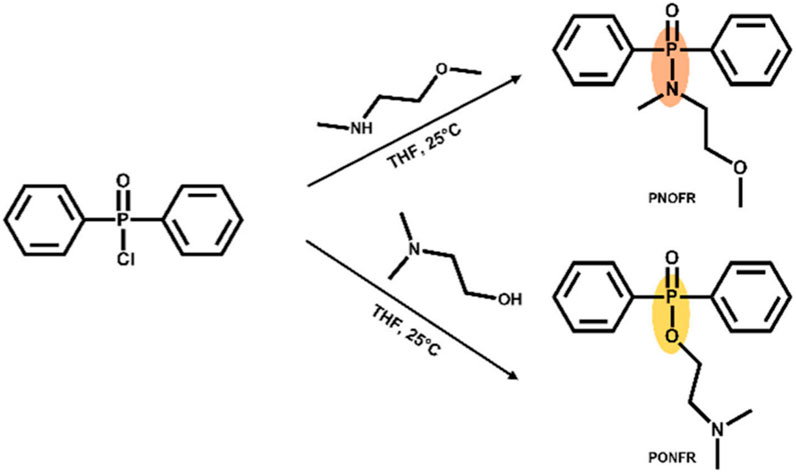
Figure 2. The structure of PNOFR and PONFR (Kim et al., 2023).
Phosphorus anhydride generated during the thermal decomposition forms a glassy-like molten material covering the surface of combustible materials, promoting their oxidation into carbon dioxide, thus contributing to flame retardancy. Additionally, phosphoric acid produced from phosphorus-based flame retardants can absorb heat, as it prevents the oxidation of carbon monoxide (CO) into carbon dioxide (CO2), thereby reducing the heating process (Zhang et al., 2015).
3.2 Gas-phase flame retardant mechanism
When flame retardants decompose upon heating, they release volatile phosphorous compounds (such as PO) that significantly reduce the concentration of hydrogen atoms. It has been demonstrated that PO can capture hydrogen, with the reaction PO + H=HPO, thus inhibiting or slowing down the combustion chain reaction. For example, in the work reported by Kim et al. (2023), PONFR released more PO2· and PO· free radicals in the gas phase compared to PNOFR. The decomposition of flame retardants generates non-flammable gases, which can reduce the concentration of combustible gases in the combustion zone below the lower flammability limit, while also diluting the oxygen concentration in that area, thereby preventing further combustion and achieving flame-retardant purposes (Zhang et al., 2015).
3.3 Synergistic flame retardant mechanism
When another flame-retardant element is introduced into phosphorus-containing flame retardants, the flame-retardant effect often exceeds that of phosphorus alone. Phosphorus primarily provides flame retardancy in the condensed phase, while the additional flame-retardant element acts in the gas phase. These different flame-retardant elements exhibit excellent synergistic effects across different phases. Examples include the synergy of phosphorus with halogens, nitrogen, and boron (Braun et al., 2006). Due to the presence of multiple flame-retardant elements within the same molecule, the synergistic effect of these elements enhances the flame-retardant performance of polymer materials. Commonly used flame retardants include tri (2-chloroethyl) phosphate, tri (2,3-dichloropropyl) phosphate, tri (2-bromopropyl) phosphate, tri (bromochloropropyl) phosphate, and halogenated polyphosphate esters. New research on these types of flame retardants has produced many candidates, notably brominated phosphate melamine salts, which contain 35% bromine, 6% phosphorus, and 15% nitrogen, with a thermal decomposition temperature above 250°C (Lv, 1998). Phosphoryl guanidine, which includes compounds such as dihydroguanosine phosphate, hydrogen dihydroguanosine phosphate, pyrophosphate, and condensed guanidine phosphate, is used for flame retardancy and fireproof coatings for cotton fabric, wood, paper, and polyolefins (Jieyu and Yongmei, 1999). Recently studied new flame retardants include monophosphate (1-chloro-3-hydroxypropyl) esters and their antimony compounds (Liu et al., 2000; Ma and Yang, 2016). Additionally, some carbon materials, such as fullerenes, carbon black, and carbon nanotubes, can capture highly reactive free radicals, interrupting the chain reaction during combustion (Peng et al., 2021; Ji et al., 2021; Sai et al., 2022).
3.4 Mechanism of action of organic phosphorus flame retardants
Organic phosphorus flame retardants are discussed separately due to their distinct decomposition pathways. Unlike inorganic variants, organophosphates undergo complex thermal degradation, releasing volatile phosphorus species (e.g., PO·) that interrupt gas-phase combustion. The mechanism of action of organic phosphorus flame retardants primarily involves the formation of more structurally stable cross-linked solids or char layers upon heating. This char formation not only prevents further pyrolysis of the polymer but also inhibits the volatile products generated from internal thermal decomposition from participating in the combustion process. Specifically, phosphorus-containing additives primarily act in the condensed phase, where their flame-retardant mechanisms include the formation of certain molecules that act as dehydrating agents, promoting char formation and reducing heat transfer from the flame to the condensed phase. Simultaneously, phosphoric acid can absorb heat and prevent the oxidation of carbon monoxide into carbon dioxide, thereby slowing the heating process. Furthermore, it may form a thin glassy or liquid protective layer over the condensed phase, reducing oxygen diffusion and heat transfer between the gas and solid phases, which suppresses the oxidation of char. For example, Li Jianzong et al. conducted thermal gravimetric analysis, differential scanning calorimetry, infrared spectroscopy, and elemental analysis to study the effects of TCPP (tri (2,3-dichloropropyl) phosphate) on the thermal degradation of epoxy resin. Their findings demonstrated that TCPP primarily operates through a condensed-phase mechanism, catalyzing decomposition and enhancing char formation, thus proposing a model for the condensed-phase phosphorus flame-retardant action of TCPP on epoxy resin (Jianzong et al., 1990).
Moreover, phosphorus-containing flame retardants undergo a series of transformations upon thermal decomposition, ultimately forming stable polyphosphoric acid, which is a non-volatile, stable compound with strong dehydrating properties that isolates the polymer surface from air. The water vapor released absorbs a significant amount of heat, reducing the surface temperature of the polymer and thus achieving flame-retardant effects.
4 Applications of phosphorus flame retardants in different materials
Phosphorus-based flame retardants are widely applied across various materials, including polymers, coatings, textiles, and electronic devices, due to their unique flame-retardant mechanisms and diverse chemical structures. These properties make phosphorus flame retardants an essential tool for enhancing the fire resistance of different materials.
4.1 Polymeric materials
Polymeric materials represent one of the key areas where phosphorus flame retardants are utilized. Organic phosphorus flame retardants can be blended with polymers to improve their flame-retardant properties. By incorporating phosphorus-based additives, the burning rate of polymeric materials decreases, limiting flame spread. Additionally, the char layer formed during combustion acts as a barrier to oxygen, enhancing the material’s fire resistance. Phosphorus flame retardants are not only used in general-purpose polymers but are also increasingly applied to high-performance polymers that require resistance to high temperatures.
Cotton fiber, as a natural polymeric material, is characterized by its high hygroscopicity, biodegradability, and skin-friendly properties. Owing to these attributes, it is extensively utilized in industrial fire-retardant apparel, military protective wear, and home furnishings, as well as in the construction sector (including architectural decoration materials, ornamental elements, and fencing) and the automotive industry (encompassing door panels and dashboards) (Shahidi and Ghoranneviss, 2013; Emam, 2019). Nevertheless, cotton fabric is inherently highly flammable, with a LOI approximating 17.0% (Liu X. et al., 2018), which facilitates rapid flame propagation. Li J. et al. (2022) synthesized a phosphorus-nitrogen flame retardant containing reactive -P-O-−amino groups, employing glycerol, phosphoric acid, and urea as precursors. Their findings revealed that cotton fabric treated with this P-N flame retardant exhibited commendable self-extinguishing properties. Thermogravimetric (TG) data further demonstrated that flame retardant (FR) treatment reduced the initial thermal degradation temperature of untreated cotton to 225.9°C and 221.8°C under nitrogen and air atmospheres, respectively, upon heating. Importantly, the whiteness and mechanical properties of the treated cotton remained within an acceptable range.
4.2 Carbon materials
In recent years, carbon materials have been widely used to prepare polymer composites. Compared to traditional flame retardants, the introduction of carbon materials can enhance the flame resistance, mechanical properties, thermal stability, and thermal conductivity of the materials, aiding in the development of high-performance flame-retardant polymer composites (Wang et al., 2017b). Carbon materials generally function through two flame-retardant mechanisms: (1) forming a continuous and dense carbon layer on the surface of the polymer, which suppresses mass and heat transfer during combustion, and (2) some carbon materials, such as fullerenes, carbon black, and carbon nanotubes, capture highly reactive free radicals, thereby interrupting the chain reactions that occur during combustion (Peng et al., 2021; Ji et al., 2021; Sait et al., 2022).
4.3 Resins and coating materials
In the field of coatings and resins, phosphorus flame retardants are extensively used to enhance the fire resistance of coatings. By introducing phosphorus-based flame retardants into coating systems, the flammability of the coatings is reduced, and the rate of flame spread is slowed, which is crucial for the fire safety of buildings, vehicles, and other applications. Moreover, phosphorus flame retardants improve the weather resistance and chemical resistance of the coatings.
However, in practical applications, these retardants tend to absorb moisture, oxidize, and emit highly toxic gases. Their powders are also prone to explosion, and their deep red color, along with poor compatibility with resins, makes them difficult to mix uniformly. This often results in reduced physical properties of the substrate (Luo and Ziqiang, 2005).
Recently, Zhang et al. (2021) reported a novel phosphorus-containing flame retardant and introduced its application in resins. Traditional flame retardants often contain highly toxic substances that pose significant risks to both human health and the environment. The new phosphorus-based flame retardant was synthesized using phenylphenol as a substrate. This intermediate was mixed with D4 Vi under specific conditions to create a new phosphorus-containing flame retardant, which was then added to epoxy resin to produce flame-retardant epoxy materials. Experimental results showed that the new flame retardant forms a more uniform and dense carbon layer during combustion, effectively protecting the underlying materials and significantly improving the flame-retardant properties of the epoxy resin. Furthermore, the toxicity of the new phosphorus-containing flame retardant is much lower than that of traditional flame retardants, making it more suitable for practical engineering applications.
4.4 Textile materials
The demand for flame-retardant textiles has become increasingly prominent in public transportation, home goods, and other sectors. Phosphorus flame retardants can be applied to fibers through impregnation, coating, or spinning processes, significantly improving the flame resistance of textiles. By slowing the combustion rate of fibers and reducing the heat release of flames, phosphorus flame retardants help mitigate fire hazards. As nylon products continue to expand in electronic, aerospace, and automotive applications, and with growing environmental awareness, there is increasing attention to eco-friendly products. Phosphorus flame retardant-enhanced nylon materials meet customer demands with balanced properties such as good processability, mechanical strength, and a high Comparative Tracking Index (CTI), making them widely applicable in products like low-voltage electronic capacitor housings, load-breaking switches, carbon brush brackets, and automotive seat components (Li et al., 2020).
In a study by Olvera-Gracia et al. (2012), 100% acrylic textile fabrics were treated with two types of flame retardants: one was a commercial flame retardant combining polymeric resin, phosphoric acid, and borax, while the other was sodium hexametaphosphate. Standard tests for flammability and mechanical properties revealed that fabrics treated with flame retardants demonstrated significantly improved or, at minimum, maintained mechanical properties compared to untreated fabrics. The use of flame retardants increased the fabric’s burn time from 8 s to 2 min.
Similarly, Xu et al. (2013) used a durable cyclic phosphonate flame retardant, Hifire T-886, on polyester fabrics, achieving flame resistance that met national standards for protective clothing. The flame-retardant treatment caused minimal damage to the physical and mechanical properties of the polyester fabric, with no significant changes in tensile strength, and exhibited excellent wash resistance. Halogenated diphosphonates have also been employed to modify various textiles, including cotton, linen, kapok, hemp, cellulose, and their derivatives, as well as nylon and other fibers, improving both flame resistance and processing performance. Cotton fibers or fabrics treated with halogenated diphosphonates not only gain enhanced flame retardancy but also show improvements in texture (Richard et al., 1964; Birum et al., 1965).
4.5 Other applications
Beyond the primary applications mentioned, phosphorus flame retardants are also utilized in construction materials, automotive interiors, and aerospace applications. With continuous advancements in technology, the application prospects of phosphorus flame retardants in even more material domains are expanding.
Phosphorus flame retardants have already achieved significant progress in various materials, contributing substantially to enhancing fire safety. Their diverse flame-retardant mechanisms and adaptability to different materials offer broad prospects for application across multiple fields. Further research and innovation will promote the wider use of phosphorus flame retardants, supporting improved material safety and sustainability.
5 Toxicity of organophosphorus flame retardants
5.1 Current status of toxicity assessment
Phytic acid, a naturally occurring form of phosphorus and widely found as a nutrient in many plant seeds, is used as a flame retardant (Mokhena et al., 2022). While phosphorus compounds are undeniably essential for the health of all living organisms, the toxicity of organophosphorus compounds varies significantly depending on their structure. These variations span a broad spectrum, from neurotoxins to pesticides, herbicides, disinfectants, and even food additives. According to Bob A. Howell, current research on the toxicity of organophosphorus flame retardants is still in its infancy, and the toxicity of many such compounds has not been comprehensively or effectively documented (Howell, 2023). In Table 3, we have compiled the toxicity data of several key phosphorus-based flame retardants that have been extensively studied to date (data primarily sourced from PubChem). The toxicity data for other phosphorus-based flame retardants can be obtained from PubChem or other relevant databases.
Some compounds with low-level oxidation at the phosphorus atom, particularly DOPO (9,10-dihydro-9-oxa-10-phosphaphenanthrene-10-oxide), have been observed to exhibit minimal toxicity (Hirsch et al., 2017; Liu M. et al., 2018). For example, Peng et al. (2023) investigated the toxicity of the organophosphorus flame retardant triphenyl phosphate (TPHP) on the intestines of mice. They observed symptoms similar to Crohn’s disease in the ileum and ulcerative colitis in the colon, including shortened colon length, structural damage to the ileum/colon, apoptosis of intestinal epithelial cells, an accumulation of pro-inflammatory cytokines and immune cells, and disruptions to tight junctions. Additionally, TPHP was found to induce oxidative stress and apoptosis in Caco-2 cells (intestinal epithelial cells), accompanied by the destruction of tight junctions. Their work also sought to explain the underlying mechanisms of these effects.
Cao et al. (2023) illustrated the splenotoxicity of the organophosphorus flame retardant tris(1,3-dichloro-2-propyl) phosphate (TDCPP) in mice, while Saquib et al. (2022) uncovered the genotoxicity and carcinogenic potential of TDCPP in human liver cells. These studies provided a genetic-level assessment of TDCPP’s toxic risks and offered directions for future research.
It is evident that global research on the toxicity of various organophosphorus flame retardants remains insufficient and incomplete. Many phosphorus-based flame retardants that are closely tied to everyday life have not yet had their toxicity fully explored, posing potential risks to human health. Therefore, the thorough evaluation of the toxicity of these compounds by the scientific community is both necessary and urgent.
5.2 Needs and directions for toxicity evaluation
There is a pressing need for careful and comprehensive assessments of the toxicity of alkyl phosphates (with the size and branching of alkyl groups as variables), aryl phosphates (with a focus on the nature of the aromatic ring and any substituents present), and, most importantly, halogenated organophosphates, which represent different classes of phosphorus compounds. Similarly, the toxicity of inorganic phosphorus flame retardants, such as phosphonates, hypophosphites, and phosphine oxides, should also be studied as a separate category. Moreover, reliable toxicity data based on the structure of organophosphorus compounds are essential as reference points for future research (Howell, 2023).
6 Prospects and outlook for research on phosphorus flame retardants
As societal attention to fire safety continues to grow, the development prospects for flame retardant technologies are becoming increasingly broad. Phosphorus flame retardants, as an important category of flame retardant materials, are continuously being innovated and researched, providing more options and possibilities for enhancing fire resistance in materials. In-depth studies on the types, synthesis methods, mechanisms of action, and applications of phosphorus flame retardants can offer robust support for ongoing breakthroughs and innovations in flame retardant technology.
In regions such as Yunnan, Guizhou, Sichuan, and Hubei in China, there are abundant phosphorus ore deposits, ranking among the top two or three globally (Ran, 2005). Recently, the Ningbo Institute of Materials Technology and Engineering, Chinese Academy of Sciences, signed a cooperative agreement with the National Engineering Technology Center for Composite Modified Polymer Materials, Guizhou Yuan Yi Mineral Group Co., Ltd., and the People’s Government of Weng’an County in Guizhou Province. This collaboration aims to jointly promote the development and industrialization of new high-end phosphorus-based flame retardants for engineering plastics that exhibit high thermal stability, chemical stability, good electrical properties, and high flame retardancy. The development and application of high-performance phosphorus-based flame retardants is a key research direction for the Ningbo Institute of Materials.
Recently, Liu and Yao (2017) designed and synthesized two efficient phosphorus flame retardants based on polycarbonate, the structures of which are illustrated in Figure 3. As shown in Table 2, it is evident that organic phosphorus flame retardants are often based on phosphoric acid, with various groups (such as phenyl or alkyl) connected to the hydroxyl oxygen or phosphorus atom itself. This structural commonality among organic phosphorus flame retardants can provide inspiration for future research. Specifically, when designing and synthesizing new organic phosphorus flame retardants, it may be advantageous to use phosphoric acid as a foundation and modify the groups at appropriate positions to achieve more favorable outcomes.
In summary, the development of bio-derived and recyclable flame retardants (FRs) has garnered significant attention due to their environmental friendliness and sustainability. However, despite notable progress, several unresolved challenges remain. Bio-based FRs often struggle to match the performance and cost-effectiveness of traditional petroleum-based counterparts, particularly in terms of flame retardant efficiency, thermal stability, and durability. Additionally, the recyclability and reprocessing efficiency of current recyclable FRs in polymer matrices are limited, hindering their large-scale application. Future research should focus on developing high-efficiency, low-cost bio-based materials with superior flame retardant properties, such as lignin, cellulose derivatives, and natural polymer-based composites, while exploring their potential in a circular economy framework.
Balancing flame retardancy with mechanical and thermal performance is a critical challenge in the development of FRs. The incorporation of traditional FRs often significantly compromises the mechanical strength, toughness, and thermal stability of materials, limiting their application in high-end fields. Research indicates that interfacial compatibility, dispersion, and synergistic effects between FRs and matrix materials are key factors influencing this balance. Future efforts should aim to design multifunctional FRs through molecular design and interface engineering to achieve a synergistic enhancement of flame retardancy, mechanical properties, and thermal stability. Moreover, microstructure regulation in composite materials and multiscale design of FRs will be essential directions for future research.
The standardization of toxicity evaluation frameworks for FRs is an urgent need to ensure environmental safety and human health. Currently, the lack of a unified standard for toxicity assessment leads to incomparable and often contradictory results across studies. Existing evaluation methods primarily focus on acute toxicity, while research on chronic toxicity, ecotoxicity, and bioaccumulation remains insufficient. Future studies should focus on establishing comprehensive and standardized toxicity evaluation frameworks, integrating in vitro experiments, computational modeling, and long-term exposure studies to elucidate the mechanisms of FR toxicity. Additionally, the principles of green chemistry should guide the design and development of FRs to minimize the generation and release of hazardous substances.
Nanoscale engineering offers promising opportunities for the development of multifunctional FRs. Leveraging the unique properties of nanomaterials, such as high surface area, excellent dispersibility, and versatile surface chemistry, can enable synergistic enhancements between FRs and matrix materials. However, challenges related to the production costs, scalability, and potential biological toxicity of nano-FRs continue to hinder their widespread application. Future research should prioritize the development of efficient, cost-effective, and environmentally friendly nano-FRs, such as systems based on two-dimensional materials (e.g., graphene, molybdenum disulfide) and metal-organic frameworks (MOFs). Furthermore, the performance stability of nano-FRs under extreme conditions and their interfacial interactions with matrix materials warrant further investigation.
Therefore, the development of bio-derived and recyclable FRs, the balance between flame retardancy and mechanical/thermal performance, the standardization of toxicity evaluation frameworks, and the application of nanoscale engineering in multifunctional FRs represent critical challenges in the field. Future research should emphasize interdisciplinary collaboration, integrating insights from materials science, chemistry, environmental science, and toxicology to drive innovation and sustainability in FR technology. By addressing these challenges, advances in FRs can achieve high-performance flame retardancy while ensuring environmental compatibility, economic viability, and multifunctionality, thereby contributing significantly to the future of materials science.
7 Conclusion
In summary, phosphorus-based flame retardants are characterized by their low halogen content, halogen-free composition, low smoke production, and low toxicity. Their low dosage and high efficiency have garnered significant attention in the field of flame retardants. However, certain limitations inherent to phosphorus-based flame retardants—such as poor compatibility, insufficient surface treatment technologies, their often liquid state, high volatility, substantial smoke generation, and relatively low thermal stability—restrict their application. Therefore, further research on phosphorus-based flame retardants is imperative (Zhou et al., 2009).
Continuing to strengthen research efforts is crucial, particularly in the area of toxicity assessment, where several aspects remain incomplete and insufficiently explored. Future research should encompass comprehensive and meticulous toxicity evaluations of various categories of organic phosphorus compounds, including alkyl phosphates, aryl phosphates, and halogenated organic phosphonates. Additionally, it is essential to investigate the toxicity of inorganic phosphorus flame retardants, such as phosphonates, phosphinates, and phosphine oxides, as separate entities.
Moreover, when synthesizing new organic phosphorus flame retardants, it is advisable to enhance performance and reduce toxicity by attaching functional groups to the phosphate backbone. As societal emphasis on fire safety continues to increase, ongoing innovation and research will facilitate the application of phosphorus-based flame retardants across diverse fields, including polymers, coatings, textiles, and other industrial materials, thereby contributing to the development of safer and more efficient materials. Finally, new strategies for synthesizing organic phosphorus flame retardants should also be explored, such as forming phosphates by attaching groups to the hydroxyl oxygen or directly linking specific atoms or groups to the phosphorus atom.
Author contributions
ZY: Writing – original draft, Writing – review and editing. ZJ: Writing – review and editing. TW: Writing – review and editing.
Funding
The author(s) declare that no financial support was received for the research and/or publication of this article.
Conflict of interest
The authors declare that the research was conducted in the absence of any commercial or financial relationships that could be construed as a potential conflict of interest.
Generative AI statement
The authors declare that no Generative AI was used in the creation of this manuscript.
Publisher’s note
All claims expressed in this article are solely those of the authors and do not necessarily represent those of their affiliated organizations, or those of the publisher, the editors and the reviewers. Any product that may be evaluated in this article, or claim that may be made by its manufacturer, is not guaranteed or endorsed by the publisher.
References
Amarnath, N., Appavoo, D., and Lochab, B. (2018). Eco-Friendly halogen-free flame retardant cardanol polyphosphazene polybenzoxazine networks. ACS Sustain. Chem. Eng. 6 (1), 389–402. doi:10.1021/acssuschemeng.7b02657
Antonatos, N., Bousa, D., Kovalska, E., Sedmidubský, D., Růzicka, K., Vrbka, P., et al. (2020). Large-scale production of nanocrystalline black phosphorus ceramics. ACS Appl. Mater. Interfaces 12 (6), 7381–7391. doi:10.1021/acsami.9b13362
Ardanuy, M., and Velasco, J. I. (2011). Mg–Al Layered double hydroxide nanoparticles. Appl. Clay Sci. 51, 341–347. doi:10.1016/j.clay.2010.12.024
Bar, M., Alagirusamy, R., and Das, A. (2015). Flame retardant polymer composites. Fibers Polym. 16 (4), 705–717. doi:10.1007/s12221-015-0705-6
Birum, G. H., and Gail, H. (1965). 2,2-Bis (halomethyl)- 1, 3-propylenebis (phosphorodihalidates) and halogenated diphosphate esters there of. U.S. Patent No. 3,192,242.
Bolshova, T. A., Shvartsberg, V. M., Korobeinichev, O. P., and Shmakov, A. G. (2016). A skeletal mechanism for flame inhibition by trimethylphosphate. Combust. Theory Model. 20 (2), 189–202. doi:10.1080/13647830.2015.1115556
Braun, U., Balabanovich, A. I., Schartel, B., Knoll, U., Artner, J., Ciesielski, M., et al. (2006). Influence of the oxidation state of phosphorus on the decomposition and fire behaviour of flame-retarded epoxy resin composites. Polymer 47 (26), 8495–8508. doi:10.1016/j.polymer.2006.10.022
Cai, Y., Hu, Y., Song, L., Lu, H., Chen, Z., and Fan, W. (2006). Preparation and characterizations of HDPE–EVA alloy/OMT nanocomposites/paraffin compounds as a shape stabilized phase change thermal energy storage material. Thermochim. Acta. 451, 44–51. doi:10.1016/j.tca.2006.08.015
Camino, G., Costa, L., and Trossarelli, L. (1984). Study of the mechanism of intumescence in fire retardant polymers: Part I—Thermal degradation of ammonium polyphosphate-pentaerythritol mixtures. Polym. Degrad. Stab. 6, 243–252. doi:10.1016/0141-3910(84)90004-1
Cao, L., Wei, L., Du, Q., Su, Y., Ye, S., and Liu, K. (2023). Spleen toxicity of organophosphorus flame retardant TDCPP in mice and the related mechanisms. Toxics 11 (3), 231. doi:10.3390/toxics11030231
Chang, S., Zeng, C., Yuan, W., and Ren, J. (2012). Preparation and characterization of double-layered microencapsulated red phosphorus and its flame retardance in poly(lactic acid). J. Appl. Polym. Sci. 125, 3014–3022. doi:10.1002/app.36449
Cheema, H. A., El-Shafei, A., and Hauser, P. J. (2013). Conferring flame retardancy on cotton using novel halogen-free flame retardant bifunctional monomers: synthesis, characterizations and applications. Carbohydr. Polym. 92, 885–893. doi:10.1016/j.carbpol.2012.09.081
Chen, L., and Wang, Y.-Z. (2010). A review on flame retardant technology in China. Part I: development of flame retardants. Polym. Adv. Technol. 21 (1), 1–26. doi:10.1002/pat.1550
Chen, X., Huang, C., Shi, Y., Yuan, B., Sun, Y., and Bai, Z. (2019). MoO3-ZrO2 solid acid for enhancement in the efficiency of intumescent flame retardant. Powder Technol. 344, 581–589. doi:10.1016/j.powtec.2018.12.056
Chen, X., Ma, C., and Jiao, C. (2016a). Synergistic effects between [Emim]PF6and aluminum hypophosphite on flame retardant thermoplastic polyurethane. RSC Adv. 6, 67409–67417. doi:10.1039/c6ra14094g
Chen, X., Ma, C., and Jiao, C. (2016b). Enhancement of flame-retardant performance of thermoplastic polyu-rethane with the incorporation of aluminum hypophosphite and iron-graphene. Polym. Degrad. Stab. 129, 275–285. doi:10.1016/j.polymdegradstab.2016.04.017
Chen, X., Ponraj, J. S., Fan, D., and Zhang, H. (2020). An overview of the optical properties and applications of black phosphorus. Nanoscale 12 (6), 3513–3534. doi:10.1039/c9nr09122j
Chen, Y., Yi, S., Zhang, X., Shi, D., Liu, C., Rao, P., et al. (2024). Study on flame retardancy of EPDM reinforced by ammonium polyphosphate. RSC Adv. 14 (13), 8684–8694. doi:10.1039/d4ra00733f
Cheng, X., Wu, Y., Huang, Y., Jiang, J., Xu, J., and Guan, J. (2020). Synthesis of a reactive boron-based flame retardant to enhance the flame retardancy of silk. React. Funct. Polym. 156, 104731. doi:10.1016/j.reactfunctpolym.2020.104731
Dasari, A., Yu, Z. Z., Cai, G. P., and Mai, Y. W. (2013). Recent developments in the fire retardancy of polymeric materials. Prog. Polym. Sci. 38 (9), 1357–1387. doi:10.1016/j.progpolymsci.2013.06.006
Dong, X., Qin, R., Nie, S., Yang, J., Zhang, C., and Wu, W. (2019). Fire hazard suppression of intumescent flame retardant polypropylene based on a novel Ni-containing char-forming agent. Polym. Adv. Technol. 30, 563–572. doi:10.1002/pat.4492
Duan, Z., Li, R., Bian, H., Wang, Y., Zhang, X., Cheng, Z., et al. (2025). Large-Scale synthesis of crystalline phosphorus nanosheets with superior air-water stability and flame-retardancy ability. Chem. Eng. J. 505, 159566. doi:10.1016/j.cej.2025.159566
Emam, H. E. (2019). Generic strategies for functionalization of cellulosic textiles with metal salts. Cellulose 26, 1431–1447. doi:10.1007/s10570-018-2185-5
Enescu, D., Frache, A., Lavaselli, M., Monticelli, O., and Marino, F. (2013). Novel phosphorous–nitrogen intumescent flame retardant system. Its effects on flame retardancy and thermal properties of polypropylene. Polym. Degrad. Stab. 98, 297–305. doi:10.1016/j.polymdegradstab.2012.09.012
Feng, C., Liang, M., Jiang, J., Zhang, Y., Huang, J., and Liu, H. (2016). An effective intumescent flame retardancy of LDPE induced by the combination of APP and CNCO-HA. J. Appl. Polym. Sci. 133, 43950. doi:10.1002/app.43950
Feng, J., Sun, Y., Song, P., Lei, W., Wu, Q., Liu, L., et al. (2017). Fire-resistant, strong, and green polymer nanocomposites based on poly(lactic acid) and core-shell nanofibrous flame retardants. ACS Sustain. Chem. Eng. 5, 7894–7904. doi:10.1021/acssuschemeng.7b01430
Ferrara, C., Vigo, E., Albini, B., Galinetto, P., Milanese, C., Tealdi, C., et al. (2019). Efficiency and quality issues in the production of black phosphorus by mechanochemical synthesis: a multi-technique approach. ACS Appl. Energy Mat. 2 (4), 2794–2802. doi:10.1021/acsaem.9b00132
Germain, L., and Winn, L. M. (2024). The flame retardant triphenyl phosphate alters the epigenome of embryonic cells in an aquatic in vitro model. J. Appl. Toxicol. 44, 965–977. doi:10.1002/jat.4589
Gu, H., Guo, J., He, Q., Tadakamalla, S., Zhang, X., Yan, X., et al. (2013). Flame-retardant epoxy resin nanocomposites reinforced with polyaniline-stabilized silica nanoparticles. Ind. Eng. Chem. Res. 52 (23), 7718–7728. doi:10.1021/ie400275n
Hansheng, H. (2002). Development trend of non-halogen flame retardant in Japan. Mod. Chem. Ind. 22 (12), 54–55.
He, D., Zhang, Z., Qu, J., Yuan, X., and Guan, J. (2018a). Facile one-step synthesis of black phosphorus via microwave irradiation with excellent photocatalytic activity. Syst. Char. 35 (11), 1800306. doi:10.1002/ppsc.201800306
He, L., Wang, J., Wang, B., Wang, X., Zhou, X., Cai, W., et al. (2019). Large-scale production of simultaneously exfoliated and Functionalized Mxenes as promising flame retardant for polyurethane. Compos B Eng. 179, 107486–107499. doi:10.1016/j.compositesb.2019.107486
He, W., Zhou, Y., Chen, X., Guo, J., Zhou, D., Chen, S., et al. (2018b). Novel intumescent flame retardant masterbatch prepared through different processes and its application in EPDM/PP thermoplastic elastomer: thermal stability, flame retardancy, and mechanical properties. Polymer 11, 50. doi:10.3390/polym11010050
He, Y. (2008). The Study and Development of Electrically Conductive Adhesive. Chem. Adhesion 30, 47–52.
He, W., Song, P., Yu, B., Fang, Z., and Wang, H. (2020). Flame retardant polymeric nanocomposites through the combination of nanomaterials and conventional flame retardants. Prog. Mater Sci. 114, 100687. doi:10.1016/j.pmatsci.2020.100687
Hirsch, C., Striegl, R., Mathes, S., Adlhart, C., Edelmann, M., Bono, E., et al. (2017). Multiparameter toxicity assessment of novel DOPO-derived organophosphorus flame retardants. Chemosphere 91, 407–425. doi:10.1007/s00204-016-1680-4
Hong, H., Zhao, Y., Huang, L., Zhong, D., and Shi, D. (2021). Bone developmental toxicity of organophosphorus flame retardants TDCIPP and TPhP in marine medaka Oryzias melastigma. Ecotoxicol. Environ. Saf. 223, 112605. doi:10.1016/j.ecoenv.2021.112605
Hong, X., Sun, C., Huang, J., Zhang, H., and Liang, B. (2012). Preparation of microcapsule red phosphorus and study on the properties of flame retardant epoxy resin application of engineering plastics. Engineering Plastics Application 40 (8), 86–89. doi:10.3969/j.issn.1001-3539.2012.08.020
Horrocks, A. R., Davies, P. J., Kandola, B. K., and Alderson, A. (2016). The potential for volatile phosphorus-containing flame retardants in textile back-coatings. J. Fire Sci. 25 (6), 523–540. doi:10.1177/0734904107083553
Howell, B. A. (2023). Toxicity of organophosphorus flame retardants. J. fire Sci. 41 (3), 102–104. doi:10.1177/07349041231161493
Huaichun, C., Tongjian, L., Yichao, G., and Qiuju, X. (2011). Preparation of microencapsulated red phosphorus with phenolic resin as capsule wall and its flame retardant application in cable materials. China Plastics Industry 39 (13), 89–92.
Huang, Z., Ruan, B., Wu, J., Ma, N., Jiang, T., and Tsai, F. C. (2021). High-efficiency ammonium polyphosphate intumescent encapsulated polypropylene flame retardant. J. Appl. Polym. Sci. 138 (20), 50413. doi:10.1002/app.50413
Ji, Y., Yang, Y., Yao, Y., Li, J., Shen, X., and Liu, S. (2021). Synthesis of carbon nanosphere-based nitrogen-phosphorus-sulfur compound flame retardant and flame retardancy of CNSs-H-D reinforced epoxy resin. Chin. J. Mater Res. 35 (12), 918–924. doi:10.11901/1005.3093.2021.393
Jian, R. K., Chen, L., Zhao, B., Yan, Y. W., Li, X. F., and Wang, Y. Z. (2014). Acrylonitrile–Butadiene–Styrene Terpolymer with metal hypophosphites: flame retardance and mechanism research. Ind. Eng. Chem. Res. 53, 2299–2307. doi:10.1021/ie403726m
Jiang, Z., and Liu, G. (2015). Microencapsulation of ammonium polyphosphate with melamine-formaldehyde-tris(2-hydroxyethyl)isocyanurate resin and its flame retardancy in polypropylene. RSC Adv. 5, 88445–88455. doi:10.1039/c5ra14586d
Jianzong, L., Shiyuan, C., and Xiaoming, X. (1990). Mechanism of flame-retardant action of tris (2, 3-dichloropropyl) phosphate on epoxy resin. J. Appl. Polym. Sci. 40 (3-4), 417–426. doi:10.1002/app.1990.070400310
Jieyu, L., and Yongmei, D. (1999). The current situation of nitrogen-containing flame retardants Flame retardant materials and technology (1), 5.
Kim, H. H., Sim, M. J., Lee, J. C., and Cha, S. H. (2023). The effects of chemical structure for phosphorus-nitrogen flame retardants on flame retardant mechanisms. J. Mater. Sci. 58 (15), 6850–6864. doi:10.1007/s10853-023-08414-6
Knyazkov, D. A., Bolshova, T. A., Shvartsberg, V. M., Chernov, A. A., and Korobeinichev, O. P. (2021). Inhibition of premixed flames of methyl methacrylate by trimethylphosphate. Proc. Combust. Inst. 38 (3), 4625–4633. doi:10.1016/j.proci.2020.06.048
Koenig, S. P., Doganov, R. A., Seixas, L., Carvalho, A., Tan, J. Y., Watanabe, K., et al. (2016). Electron doping of ultrathin black phosphorus with Cu adatoms. Nano Lett. 16 (4), 2145–2151. doi:10.1021/acs.nanolett.5b03278
Li, J., Jiang, W., and Liu, M. (2022b). Durable phosphorus/nitrogen flame retardant for cotton fabric. Cellulose 29 (8), 4725–4751. doi:10.1007/s10570-022-04558-x
Li, N. N., Jiang, G. W., Zhou, G. Y., et al. (2016). Synthesis and application progress of organic phosphorus-containing flame retardants. Chin. J. Appl. Chem. 33 (6), 611. doi:10.11944/j.issn.1000-0518.2016.06.150299
Li, Q., Li, B., Zhang, S., and Lin, M. (2012). Investigation on effects of aluminum and magnesium hypophosphites on flame retardancy and thermal degradation of polyamide 6. J. Appl. Polym. Sci. 125, 1782–1789. doi:10.1002/app.35678
Li, W., Li, J., Xing, H., Wang, K., Zhang, Y., and Liu, J. (2025). Simultaneously enhancing fire retardancy, acid and alkali resistance of polypropylene/aluminum trihydrate composite by microencapsulated red phosphorus. J. Vinyl Addit. Technol. 31 (1), 199–210. doi:10.1002/vnl.22165
Li, X., and Wang, Y. (2013). Preparation of two types of red phosphorus coatings and their flame retardancy. Modem Plastics Process. Appl. 25 (3), 35–38.
Li, X., Wang, L., Wang, Y., Yao, Y., Zhang, P., Zhao, H., et al. (2022a). Occupational exposure to organophosphate esters in e-waste dismantling workers: risk assessment and influencing factors screening. Ecotoxicol. Environ. Saf. 240, 113707. doi:10.1016/j.ecoenv.2022.113707
Li, Y., Wang, X., Zhu, Q., Xu, Y., Fu, Q., Wang, T., et al. (2023). Organophosphate flame retardants in pregnant women: sources, occurrence, and potential risks to pregnancy outcomes. Environ. Sci. Technol. 57 (18), 7109–7128. doi:10.1021/acs.est.2c06503
Li, Y. A. O., Xingliang, X. U., and Sun, Q. (2020). “Effect of flame retardants on the properties of nylon modified materials,” in Utilization of rubber and plastic resources, 23–38.
Lim, K.-S., Bee, S.-T., Sin, L. T., Tee, T.-T., Ratnam, C. T., Hui, D., et al. (2016). A review of application of ammonium polyphosphate as intumescent flame retardant in thermoplastic composites. Compos. Part B 84, 155–174. doi:10.1016/j.compositesb.2015.08.066
Lin, Y., Jiang, S., Hu, Y., Chen, G., Shi, X., and Peng, X. (2018). Hybrids of aluminum hypophosphite and ammonium polyphosphate: highly effective flame retardant system for unsaturated polyester resin. Polym. Compos 39, 1763–1770. doi:10.1002/pc.24128
Liu, C., and Yao, Q. (2017). Design and synthesis of efficient phosphorus flame retardant for polycarbonate. Industrial Eng. Chem. Res. 56 (31), 8789–8796. doi:10.1021/acs.iecr.7b01915
Liu, G., and Gao, S. (2018). Synergistic effect between aluminum hypophosphite and a new intumescent flame retardant system in poly (lactic acid). J. Appl. Polym. Sci. 135 (23), 46359. doi:10.1002/app.46359
Liu, J., Qi, P., Meng, D., Li, L., Sun, J., Li, H., et al. (2022b). Eco-friendly flame retardant and smoke suppression coating containing boron compounds and phytic acids for nylon/cotton blend fabrics. Ind. Crops Prod. 186, 115239. doi:10.1016/j.indcrop.2022.115239
Liu, L., Xu, Y., He, Y., Xu, M., Wang, W., and Li, B. (2020). A facile strategy for enhancing the fire safety of unsaturated polyester resins through introducing an efficient mono-component intumescent flame retardant. Polym. Adv. Technol. 31, 1218–1230. doi:10.1002/pat.4852
Liu, M., Yin, H., Chant, X., Yang, J., Liang, Y., Zhang, J., et al. (2018b). Preliminary ecotoxicity hazard evaluation of DOPO-HQ as a potential alternative to halogenated flame retardants. Chemosphere 193, 126–133. doi:10.1016/j.chemosphere.2017.10.142
Liu, X., Zhang, Y., Cheng, B., Ren, Y., Zhang, Q., Ding, C., et al. (2018a). Preparation of durable and flame retardant lyocell fibers by a one-pot chemical treatment. Cellulose 25, 6745–6758. doi:10.1007/s10570-018-2005-y
Liu, Y., Tang, Z., and Zhu, J. (2022a). Synergistic flame retardant effect of aluminum hydroxide and ammonium polyphosphate on epoxy resin. J. Appl. Polym. Sci. 139, e53168. doi:10.1002/app.53168
Liu, Y., Shu, W., Zeng, D., and Huang, K. (2000). Synthesis of mono(1-chloro-trihydroxypropyl) phosphate and its antimony compounds as a novel flame retardant. Mod. Chem. Ind. 20 (1), 29–31.
Lu, S.-Y., and Hamerton, I. (2002). Recent developments in the chemistry of halogen-free flame retardant polymers. Prog. Polym. Sci. 27 (8), 1661–1712. doi:10.1016/s0079-6700(02)00018-7
Luo, J., and Ziqiang, L. (2005). Application of inorganic flame retardant additives in halogen-free flame retardant. J. Gansu United Univ. Nat. Sci. Ed. 64.
Lv, Z. (1998). Synthetic flame retardant materials and technologies of high-efficiency flame retardant - bromophosphate melamine salt.
Lyche, J. L., Rosseland, C., Berge, G., and Polder, A. (2015). Human health risk associated with brominated flame-retardants (BFRs). Environ. Int. 74, 170–180. doi:10.1016/j.envint.2014.09.006
Ma, C., and Yang, C. (2016). Development trend of phosphorus-based flame retardants. Mod. Plast. Process. Appl. 13 (2), 38–41.
Maruyama, Y., Suzuki, S., Kobayashi, K., and Tanuma, S. (1981). Synthesis and some properties of black phosphorus single crystals. Phys. B+C 105 (1), 99–102. doi:10.1016/0378-4363(81)90223-0
Mokhena, T. C., Sadiku, E. R., Ray, S. S., Mochane, M. J., Matabola, K. P., and Motloung, M. (2022). Flame retardancy efficacy of phytic acid: an overview. J. Appl. Polym. Sci. 139, e52495. doi:10.1002/app.52495
Nana, Y., Dongfang, C., Bingjie, Q., Zhijin, G., Jianglan, Z., and Jing, L. (2013). Research progress on microencapsulation of red phosphorus flame retardants [J]. Fine and Specialty Chemicals 21 (1), 51–54. doi:10.3969/j.issn.1008-1100-20130.1.012
Nguyen, H. T., Nguyen, K. T. Q., Le, T. C., and Zhang, G. M. (2021). Review on the use of artificial intelligence to predict fire performance of construction materials and their flame retardancy. Molecules 26 (4), 1022. doi:10.3390/molecules26041022
Nilges, T., Kersting, M., and Pfeifer, T. (2008). A fast low-pressure transport route to large black phosphorus single crystals. J. Solid State Chem. 181 (8), 1707–1711. doi:10.1016/j.jssc.2008.03.008
Olvera-Gracia, M., Mercado-Velazquez, L., Paniagua-Mercado, A. M., et al. (2012). Increasing the Burned Time and Mechanical Properties with New Mix As Flame Retardant Based in Hexametaphosphate of Sodium and Borax in Textile 100% Acrylic Fabrics. Adv. Mater. Phys. Chem. 2, 99–101. doi:10.4236/ampc.2012.24B027
Pallmann, J., Ren, Y. L., Mahltig, B., and Huo, T. G. (2019). Phosphorylated sodium alginate/APP/DPER intumescent flame retardant used for polypropylene. J. Appl. Polym. Sci. 136, 47794. doi:10.1002/app.47794
Pan, M., Huang, R., Wang, T., Huang, D., Mu, J., and Zhang, C. (2014b). Preparation and properties of epoxy resin composites containing hexaphenoxycyclotriphosphazene. High. Perform. Polym. 26 (1), 114–121. doi:10.1177/0954008313501008
Pan, X., Liu, J., Guo, X., Yan, C., Hua, M., and Jiang, J. (2024). Synthesis and analysis of a novel phospho-nitrile flame retardant for cotton fabric. Mater. Today Commun. 40, 109350. doi:10.1016/j.mtcomm.2024.109350
Pan, Y., Hong, N., Zhan, J., Wang, B., Song, L., and Hu, Y. (2014a). Effect of graphene on the fire and mechanical performances of glass fiber-reinforced polyamide 6 composites containing aluminum hypophos-phite. Polym Plast Technol Eng 53, 1467–1475. doi:10.1080/03602559.2014.909483
Peng, F., Chen, X., Zhang, A., and Zhou, H. (2021). Controllable preparation of hyperbranched polyphosphoramide coated carbonnanotubes and its application for flame retardancy. China Plast. 35 (9), 55. doi:10.19491/j.issn.1001-9278.2021.09.009
Peng, C., Zhang, X., Chen, Y., and Wang, L. (2023). Toxicity assessment of organophosphate flame retardant triphenyl phosphate (TPHP) on intestines in mice. Ecotoxicol. Environ. Saf. 268, 115685. doi:10.1016/j.ecoenv.2023.115685
Ran, S., Fang, F., Guo, Z., Song, P., Cai, Y., Fang, Z., et al. (2019). Synthesis of decorated graphene with P, N-containing compounds and its flame retardancy and smoke suppression effects on polylactic acid. Compos B Eng. 170, 41–50. doi:10.1016/j.compositesb.2019.04.037
Richard, M. A., and Birum, G. H. (1964). Flame resistant composition containing an organic polymer and a halogenated diphosphate. U.S. Patent No. 3,157,613. 17.
Sai, T., Ran, S., Guo, Z., Song, P., and Fang, Z. (2022). Recent advances in fire-retardant carbon-based polymeric nanocomposites through fighting free radicals. SusMat 2, 411–434. doi:10.1002/sus2.73
Sang, B., Li, Z.-W., Li, X.-H., Yu, L.-G., and Zhang, Z.-J. (2016). Graphene-based flame retardants: a review. J. Mater Sci. 51 (18), 8271–8295. doi:10.1007/s10853-016-0124-0
Sang, J., Liu, Y., Hua, Q.x., Wang, B.-m., Liu, L., and Tang, J.-w. (2014). School of chemical engineering and energy, Zhengzhou University. Eco-industry Science & Phosphorus Fluorine Engineering (6), 4–8.
Saquib, Q., Al-Salem, A. M., Siddiqui, M. A., Ansari, S. M., Zhang, X., and Al-Khedhairy, A. A. (2022). Organophosphorus flame retardant TDCPP displays genotoxic and carcinogenic risks in human liver cells. Cells 11 (2), 195. doi:10.3390/cells11020195
Savas, L. A., Hacioglu, F., Hancer, M., and Dogan, M. (2020). Flame retardant effect of aluminum hypophosphite in heteroatom-containing polymers. Polym. Bull. 77, 291–306. doi:10.1007/s00289-019-02746-7
Shahidi, S., and Ghoranneviss, M. (2013). Effect of plasma pretreatment followed by nanoclay loading on flame retardant properties of cotton fabric. J. Fusion Energy 33, 88–95. doi:10.1007/s10894-013-9645-6
Shao, Z. B., Deng, C., Tan, Y., Chen, M. J., Chen, L., and Wang, Y. Z. (2014). An efficient mono-component polymeric intumescent flame retardant for polypropylene: preparation and application. ACS Appl. Mat. Interfaces 6, 7363–7370. doi:10.1021/am500789q
Shi, Y., Fu, L., Chen, X., Guo, J., Yang, F., Wang, J., et al. (2017). Hypophosphite/graphitic carbon nitride hybrids: preparation and flame-retardant application in thermoplastic polyurethane. Nanomaterials 7, 259–272. doi:10.3390/nano7090259
Sun, Q., Ding, Y., Stoliarov, S. I., Sun, J., Fontaine, G., and Bourbigot, S. (2020). Development of a pyrolysis model for an intumescent flame retardant system: poly(lactic acid) blended with melamine and ammonium polyphosphate. Compos. Part B 194, 108055. doi:10.1016/j.compositesb.2020.108055
Tang, G., Wang, X., Xing, W., Zhang, P., Wang, B., Hong, N., et al. (2012). Thermal degradation and flame retardance of biobased polylactide composites based on aluminum hypophos-phite. Ind. Eng. Chem. Res. 51, 12009–12016. doi:10.1021/ie3008133
Usri, S. N. K., Jamain, Z., and Makmud, M. Z. H. (2021). A Review on Synthesis, Structural, Flame retardancy and dielectric properties of hexasubstituted cyclotriphosphazene. Polymers 13 (17), 2916. doi:10.3390/polym13172916
van der Veen, I., and de Boer, J. (2012). Phosphorus flame retardants: properties, production, environmental occurrence, toxicity and analysis. Chemosphere 88 (10), 1119–1153. doi:10.1016/j.chemosphere.2012.03.067
Velencoso, M. N., Battig, A., Markwart, J., Schartel, B., and Wurm, F. R. (2018). Molecular firefighting—how modern phosphorus chemistry can help solve the challenge of flame retardancy. Angew. Chem. Int. Ed. Engl. 57, 10450–10467. doi:10.1002/anie.201711735
Wan, L., Deng, C., Zhao, Z., Chen, H., and Wang, Y. Z. (2020). Flame retardation of natural rubber: strategy and recent progress. Polymer 12 (2), 429. doi:10.3390/polym12020429
Wang, C., Ge, X., and Jiang, Y. (2019b). Synergistic effect of graphene oxide/montmorillonite-sodium carboxymethycellulose ternary mimic-nacre nanocomposites prepared via a facile evaporation and hot-pressing technique. Carbohydr. Polym. 222, 115026. doi:10.1016/j.carbpol.2019.115026
Wang, Q., Xiong, L., Liang, H., Chen, L., and Huang, S. (2018). Synthesis of a novel polysiloxane containing phosphorus, and boron and its effect on flame retardancy, mechanical, and thermal properties of epoxy resin. Polym. Compos 39, 807–814. doi:10.1002/pc.24002
Wang, S., Kong, D., Chen, H., Wang, Z., and Lu, Z. (2022). Construction of a novel B/N/Si synergistic flame retardant system and its application on cotton fabric. Ind. Crops Prod. 178, 114574. doi:10.1016/j.indcrop.2022.114574
Wang, X., Kalali, E. N., Wan, J. T., and Wang, D. Y. (2017a). Carbon-family materials for flame retardant polymeric materials. Prog. Polym. Sci. 69, 22–46. doi:10.1016/j.progpolymsci.2017.02.001
Wang, X., Kalali, E. N., and Wang, D.-Y. (2015a). Renewable cardanol-based surfactant modified layered double hydroxide as a flame retardant for epoxy resin. ACS Sustain. Chem. Eng. 3 (12), 3281–3290. doi:10.1021/acssuschemeng.5b00871
Wang, Y., Slassi, A., Cornil, J., Beljonne, D., and Samorì, P. (2019a). Black phosphorus: tuning the optical and electrical properties of few-layer black phosphorus via physisorption of small solvent molecules (Small 47/2019). Small 15 (47), 1970252. doi:10.1002/smll.201970252
Wang, P., Chen, L., Xiao, H., and Zhan, T. (2020). Nitrogen/sulfur-containing DOPO based oligomer for highly efficient flame-retardant epoxy resin. Polym. Degrad. Stab. 171, 109023. doi:10.1016/j.polymdegradstab.2019.109023
Wang, X., Kalali, E. N., Wan, J. T., and Wang, D. Y. (2017b). Carbon-family materials for flame retardant polymeric materials. Prog. Polym. Sci. 69, 22–46. doi:10.1016/j.progpolymsci.2017.02.001
Wang, W., Peng, Y., Zhang, W., and Li, J. (2015b). Effect of pentaerythritol on the properties of wood-flour/polypropylene/ammonium polyphosphate composite system. BioResources 10, 6917. doi:10.15376/biores.10.4.6917-6927
Wu, H., Araby, S., Xu, J., Kuan, H. C., Wang, C. H., Mouritz, A., et al. (2018a). Filling natural microtubules with triphenyl phosphate for flame-retarding polymer composites. Compos. Part A Appl. Sci. Manuf. 115, 247–254. doi:10.1016/j.compositesa.2018.09.030
Wu, H., Li, Y., Zeng, B., Chen, G., Wu, Y., Chen, T., et al. (2018b). A high synergistic P/N/Si-containing additive with dandelion-shaped structure deriving from self-assembly for enhancing thermal and flame retardant property of epoxy resins. React. Funct. Polym. 131, 89–99. doi:10.1016/j.reactfunctpolym.2018.07.009
Wu, K., Song, L., Wang, Z., and Hu, Y. (2008). Microencapsulation of ammonium polyphosphate with PVA–melamine–formaldehyde resin and its flame retardance in polypropylene. Polym. Adv. Technol. 19, 1118. doi:10.1002/pat.1231
Xiao, S., Chen, M., Dong, L., Deng, C., Chen, L., and Wang, Y. (2014). Thermal degradation, flame retardance and mechanical properties of thermoplastic polyurethane composites based on aluminum hypophos-phite. Chin. J. Polym. Sci. 32, 98–107. doi:10.1007/s10118-014-1378-0
Xiao, X., Hu, S., Zhai, J., Chen, T., and Mai, Y. (2016). Thermal properties and combustion behaviors of flame-retarded glass fiber-reinforced polyamide 6 with piperazine pyrophosphate and aluminum hypophosphite. J. Therm. Anal. Calorim. 125, 175–185. doi:10.1007/s10973-016-5391-0
Xu, C., and Jun, G. (2013). Phosphorus based flame retardant t-886 flame retardant finishing process for polyester fabrics Shanghai Textile Technology. Shanghai Textile Science Technology 41 (12), 3.
Xu, F., Zhong, L., Zhang, C., Wang, P., Zhang, F., and Zhang, G. (2019). Novel high-efficiency casein-based P–N-containing flame retardants with multiple reactive groups for cotton fabrics. ACS Sustain Chem. Eng. 7, 13999–14008. doi:10.1021/acssuschemeng.9b02474
Xue, Y., Shen, M., Zheng, Y., Tao, W., Han, Y., Li, W., et al. (2020). One-pot scalable fabrication of an oligomeric phosphoramide towards high performance flame retardant polylactic acid with a submicron-grained structure. Compos B Eng. 183, 107695. doi:10.1016/j.compositesb.2019.107695
Yang, H., Yu, B., Song, P., Maluk, C., and Wang, H. (2019). Surface-coating engineering for flame retardant flexible polyurethane foams: a critical review. Compos BEng 176, 107185. doi:10.1016/j.compositesb.2019.107185
Yang, S., Wang, J., Huo, S., Wang, M., and Zhang, B. (2016). Synergistic flame-retardant effect of expandable graphite and phosphorus-containing compounds for epoxy resin: strong bonding of different carbon residues. Polym. Degrad. Stab. 128, 89–98. doi:10.1016/j.polymdegradstab.2016.03.017
Yuye, Z. (2014). Preparation of inorganic-organic double encapsulated red phosphorus and its application research. Hefei: Hefei University of Technology, 1–52.
Zeng, X., Wu, Y., Liu, Z., Gao, S., and Yu, Z. (2018). Occurrence and distribution of organophosphate ester flame retardants in indoor dust and their potential health exposure risk. Environ. Toxicol. Chem. 37 (2), 345–352. doi:10.1002/etc.3996
Zhang, C., Li, X., Yang, R., and Lan, Y. (2016). Effects of triphenyl phosphate on styrene suspension polymerization process and flame retardance properties of polystyrene/triphenyl phosphate nanocomposite. Colloid Polym. Sci. 294, 1153–1163. doi:10.1007/s00396-016-3872-0
Zhang, H., Gong, X., Li, Z., and Wang, Y. (2021). Synthesis of new phosphorous-containing flame retardant and the properties of flame retardant epoxy resins. Pigment. Resin Technol. 50 (6), 554–562. doi:10.1108/prt-09-2020-0101
Zhang, S., Ma, H., Zhang, X., Liu, W., Zhang, L., Wu, D., et al. (2017b). Influence of various side groups on thermostability and flame retardant of polyphosphazene. N. Chem. Mat. 45 (12), 114–117.
Zhang, T., Xie, H., Xie, S., Hu, A., Liu, J., Kang, J., et al. (2023). A superior two-dimensional phosphorus flame retardant: few-layer black phosphorus. Molecules 28, 5062. doi:10.3390/molecules28135062
Zhang, X., Zhang, Y., Ye, F., Yang, Y., and Qian, Y. H. (2015). Development and application of phosphorus-based flame retardants. Eng. Plast. Appl. 43 (11), 112–117. doi:10.3969/j.issn.1001-3539.2015.11.026
Zhang, Y., Luo, R., and Tian, X. (2025). A phosphorus–nitrogen–boron cooperative flame retardant and antibacterial reagent for cotton fabric. ChemistrySelect 10 (8), e202406055. doi:10.1002/slct.202406055
Zhang, Y., Yu, B., Wang, B., Liew, K. M., Song, L., Wang, C., et al. (2017a). Highly effective P–P synergy of a novel DOPO-based flame retardant for epoxy resin. Ind. Eng. Chem. Res. 56 (5), 1245–1255. doi:10.1021/acs.iecr.6b04292
Zhang, Z., Wang, S., Jin, X., Li, Y., Yang, C., Zhu, P., et al. (2024). Preparation and application of durable and efficient flame retardants: phosphorus–nitrogen–boron synergistic effect, flame retardant properties, combustion behavior and mechanical properties. Cellulose 31 (3), 1891–1907. doi:10.1007/s10570-023-05667-x
Zhao, B., Chen, L., Long, J., Jian, R., and Wang, Y. (2013). Synergistic effect between aluminum hypophos-phite and alkyl-substituted phosphinate in flame-retarded polyamide 6. Ind. Eng. Chem. Res. 52, 17162–17170. doi:10.1021/ie4009056
Zhao, B., Chen, L., and Wang, Y. (2012). “Thermal degradation and fire behaviors of glass fiber reinforced PA6 flame retarded by combination of aluminum hypophosphite with melamine derivatives,” in Fire and polymers VI: new advances in flame retardant chemistry and science. Editors A. B. Morgan, C. A. Wilkie, and G. L. Nelson (Washington: American Chemical Society), 168–182.
Zhou, X., Li, J., and Wu, Y. (2015). Synergistic effect of aluminum hypophosphite and intumescent flame retardants in polylactide. Polym. Adv. Technol. 26, 255–265. doi:10.1002/pat.3451
Zhou, X., Qiu, S., Mu, X., Zhou, M., Cai, W., Song, L., et al. (2020). Polyphosphazenes-based flame retardants: a review. Compos. Part B Eng. 202, 108397. doi:10.1016/j.compositesb.2020.108397
Keywords: phosphorus flame retardant, gas phase, condensed phase, fiber, carbon material (CM)
Citation: Yin Z, Jiang Z and Wu T (2025) The development and application of contemporary phosphorus flame retardants: a review. Front. Mater. 12:1508000. doi: 10.3389/fmats.2025.1508000
Received: 08 October 2024; Accepted: 17 March 2025;
Published: 03 April 2025.
Edited by:
Shouxun Ji, Brunel University London, United KingdomCopyright © 2025 Yin, Jiang and Wu. This is an open-access article distributed under the terms of the Creative Commons Attribution License (CC BY). The use, distribution or reproduction in other forums is permitted, provided the original author(s) and the copyright owner(s) are credited and that the original publication in this journal is cited, in accordance with accepted academic practice. No use, distribution or reproduction is permitted which does not comply with these terms.
*Correspondence: Zheng Yin, MjAyMjMwMDMwMTEwQG1haWwuc2N1dC5lZHUuY24=