- 1Max Planck Institute for Chemical Physics of Solids, Dresden, Germany
- 2University of St Andrews, Fife, United Kingdom
- 3Institute of Low Temperature and Structure Research, Polish Academy of Sciences, Wrocław, Poland
- 4Ivan Franko Lviv National University, Lviv, Ukraine
A large variety of chemical and physical properties are exhibited by mercurides and amalgams. In this work, we have successfully examined seven strontium mercurides: SrHg11, SrHg8, Sr10Hg55, SrHg2, SrHg, Sr3Hg2, and Sr3Hg. The interest in the mercury-rich region is motivated by the large number of mercury-based superconductors that have high mercury content. At the same time, the preparation on the mercury-rich side of the binary phase diagram is experimentally non-trivial, due to the high vapor pressure of mercury and extreme air-sensitivity of mercury-rich compounds. By employing a set of specialized techniques, we were able to discover superconductivity in three mercury-strontium compounds – SrHg11 (
1 Introduction
Mercury-based compounds (mercurides) and mercury-based alloys (amalgams) have long been of significant interest in chemistry and physics (Ferro, 1954; Bruzzone and Merlo, 1973; Bruzzone and Merlo, 1974; Bruzzone and Merlo, 1975). From their everyday applications (Bjørklund, 1989; Sappl et al., 2017) to their unique superconducting and topological states (Pelloquin et al., 1993; König et al., 2007), a plethora of exciting phenomena emerge in these systems. Many mercury-based materials have unique and complex crystal structures (Tkachuk and Mar, 2008), and it is suggested that the complexity of these structures is directly related to their intriguing physical properties (Steurer and Dshemuchadse, 2016; Dubois and Belin-Ferré, 2010; Urban and Feuerbacher, 2004; Conrad et al., 2009; Samson, 1962; Weber et al., 2009; Svanidze et al., 2019; Prots et al., 2022b). Among the alkali- and alkali-earth-based mercurides, a large variety of crystallographic motifs was observed with almost 40 structure types present, ranging in complexity from simple CsCl structure type (2 atoms per unit cell, Pearson symbol
The first analysis of mercury-strontium compounds and amalgams was carried out in the end of 19th century (Kerp, 1898; Kerp et al., 1900; Guntz and Roederer, 1906; Devoto and Recchia, 1930), with the first study of crystal structure dating back to 1954 (Ferro, 1954). The binary phase diagram was first unified 20 years later (Bruzzone and Merlo, 1974), and re-evaluated in 2005 (Gumiński, 2005), resulting in reformulation of multiple phases (Kerp et al., 1900; Guntz and Roederer, 1906; Devoto and Recchia, 1930). Both mercurides as well as isostructural families of compounds have been studied after that: for example, Sr3Hg2 (Druska et al., 1996),
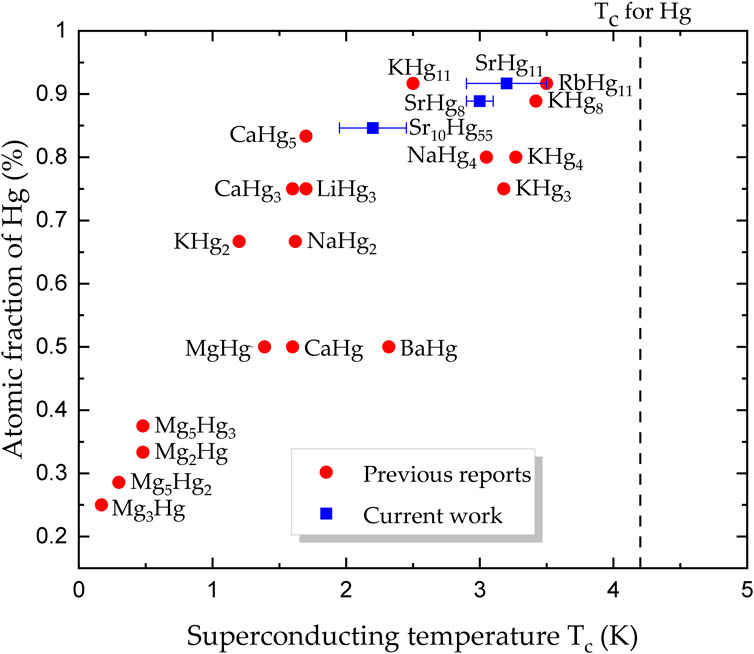
Figure 1. Binary superconductors containing mercury and alkali or alkali-earth elements. Superconductors reported as part of this work are marked with blue squares, while those reported previously are shown as red circles (Claeson and Luo, 1966; Roberts, 1976; Biehl and Deiseroth, 1999; Claeson and Luo, 1966; Hohl et al., 2023; Hänisch et al., 2023). The maximum value for the superconducting temperature
2 Materials and methods
Several issues complicate experimental investigations of mercury-containing materials – toxicity, high vapor pressure, high chemical reactivity, and extreme air sensitivity – call for a specialized laboratory environment. As we have previously shown (Witthaut et al., 2023; Prots et al., 2022a; Prots et al., 2022b; Svanidze et al., 2019), it is possible to conclusively establish chemical and physical properties of mercurides and amalgams by utilizing a set of dedicated experimental techniques (Leithe-Jasper et al., 2006).
All of the samples were synthesized by combining Hg (droplet, Alfa Aesar, 99.999%) and Sr (pieces, Alfa Aesar, 99.95%), with the Sr:Hg ratio varied from 2:98 to 79:21 (Table 1). Elements were sealed in tantalum tubes, in order to preserve stoichiometry. To protect samples from air and moisture, all syntheses were performed in an argon filled glove box system. The tubes were heated up to 500°C over 24 h and held there for a further 50 h before cooling back to room temperature over 96 h, with the slow cooling ensuring that the samples were able to crystallize efficiently. Mercury-rich samples were then placed into specialized crucibles (Canfield and Svanidze, 2024) (Figure 2) and centrifuged at room temperature to remove excess mercury. While most of the mercury can be eliminated this way, the small remainder can usually be removed by allowing the crystals to sit on gold foil for several days. Resultant single crystals of SrHg11, SrHg8, and Sr10Hg55 are shown in Figure 2 (bottom). The rest of the compounds were synthesized in polycrystalline form. It is important to note, that secondary phases were present in all samples (Table 1).
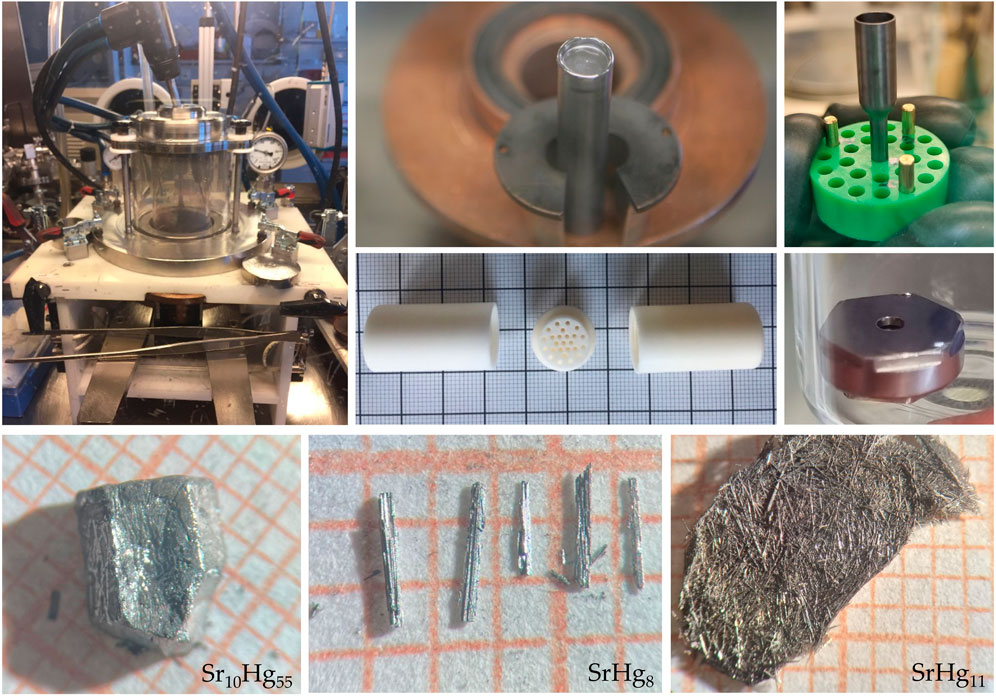
Figure 2. Top: Experimental solutions used for synthesis and characterization of mercury-strontium compounds. Left: arc melter used for sealing tantalum tubes under argon. Center, top: sealed tantalum tube. Center bottom: Canfield-Svanidze crucible set used for centrifugation of samples. Right, top: tantalum ampoule used for DTA measurements. Right, bottom: modified stainless steel high-pressure capsule used for DSC as an alternative to DTA on mercury-rich samples. Bottom: Single crystals of superconducting strontium mercurides–SrHg11 (sample 1), SrHg8 (sample 4), and Sr10Hg55 (sample 4, Table 1).
Powder X-ray diffraction was performed on a Huber G670 Image plate Guinier camera with a Ge-monochromator (Cu
Differential thermal analysis (DTA) was performed using STA 449 F3 Jupiter (NETZSCH) and DCS 404 Pegasus (NETZSCH) set-ups with a 10 K/min heating and cooling rate. The samples were measured in the temperature range from 25°C to 875°C, with the exact ranges based on expected transitions. The previously published mercury-strontium binary phase diagram defined by (Gumiński, 2005) was used as a guide. Samples were sealed in tantalum ampoules, under argon. The particularly challenging part was to evaluate the mercury-rich samples, given their high vapor pressure – this region of the binary phase diagram is also the least explored one. For this, modified stainless steel high-pressure capsules with a volume of 30
Magnetic properties were examined using a Quantum Design (QD) Magnetic Property Measurement System in the temperature range of 1.8–300 K and under various applied magnetic fields. Samples were sealed in glass tubes to prevent reaction with air. The specific heat data were collected on a QD Physical Property Measurement System from 0.4 K to 10 K and under various applied magnetic fields. Given the high air-sensitivity of the mercury-rich phases, samples were covered with Apiezon N vacuum grease, which resulted in a rather large background (Figure 3, panel a) – and while the transition to the superconducting state was observed, it was unfortunately not possible to carry out an in-depth analysis of the superconducting state. The corresponding
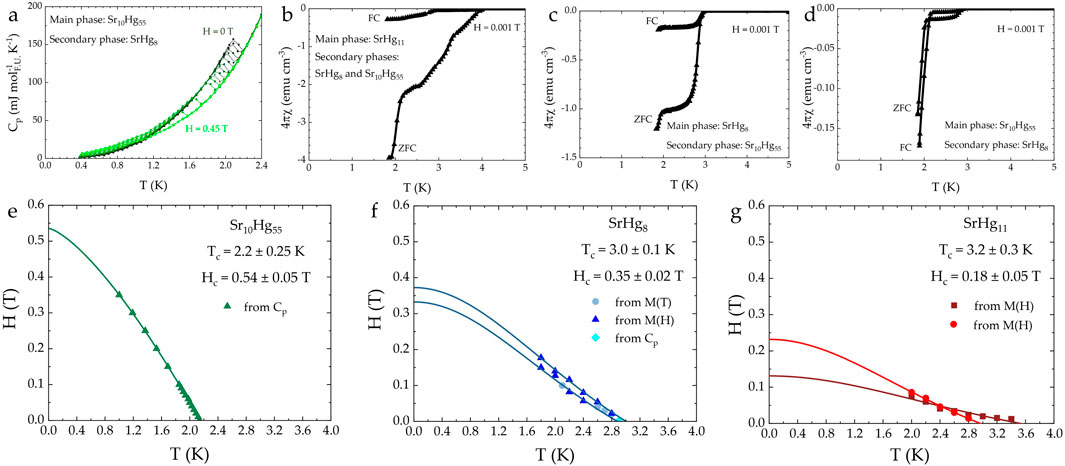
Figure 3. Superconducting properties of strontium mercurides Sr10Hg55, SrHg8, and SrHg11. (A) Specific heat for Sr10Hg55 (B–D) Magnetic susceptibility for the three superconducting samples, each panel shows one sample which has multiple superconducting transitions. Both ZFC (zero-field-cooled) and FC (field-cooled) curves are shown. (E–G)
3 Results and discussion
As mentioned above, the goal of this work was to revisit the mercury-strontium phases and explore their physical properties. In order to clarify the phases and their evolution with temperature, a complementary analysis of the powder X-ray diffraction and differential thermal analysis was implemented. The following phases were examined: SrHg11, SrHg8, Sr10Hg55, SrHg2, SrHg, Sr3Hg2, and Sr3Hg. No evidence of SrHg3 (Wendorff and Röhr, 2018) or Sr2Hg (Bruzzone and Merlo, 1974; Gumiński, 2005) was found. The difficulty in stabilizing the former can perhaps be explained by a relatively short liquidus line (Wendorff and Röhr, 2018).
Motivated by the lack of publications addressing physical properties of strontium mercurides, we have performed a thorough analysis of these materials. Much like in our previous work (Witthaut et al., 2023; Prots et al., 2022a; Prots et al., 2022b; Svanidze et al., 2019), we have taken considerable care to protect our samples from decomposition in order to study their intrinsic properties. All of the phases from 25 at.% strontium and up, were found to be either dia- or paramagnetic down to the lowest measured temperature
It is important to note that the values of the upper critical fields
4 Conclusion
The mercury-strontium compounds were revisited with the emphasis on the mercury-rich phases for two reasons: (i) conflicting reports regarding existing phases and (ii) a possibility of finding new superconductors. The reformulation of the system was made possible by implementing differential thermal analysis coupled with powder X-ray diffraction experiments. Existence of SrHg11, SrHg8, Sr10Hg55, SrHg2, SrHg, Sr3Hg2, and Sr3Hg was confirmed.
The physical properties of all phases were probed down to
Data availability statement
The original contributions presented in the study are included in the article/supplementary material, further inquiries can be directed to the corresponding author.
Author contributions
RN: Conceptualization, Data curation, Methodology, Visualization, Writing–original draft, Writing–review and editing. YP: Data curation, Investigation, Methodology, Writing–review and editing. MK: Data curation, Investigation, Methodology, Visualization, Writing–review and editing. NZ: Data curation, Investigation, Methodology, Writing–review and editing. OP: Data curation, Writing–review and editing. MS: Data curation, Investigation, Methodology, Writing–review and editing. LA: Data curation, Investigation, Writing–review and editing. YG: Data curation, Investigation, Methodology, Writing–review and editing. ES: Conceptualization, Data curation, Funding acquisition, Investigation, Methodology, Project administration, Supervision, Validation, Writing–original draft, Writing–review and editing.
Funding
The author(s) declare that financial support was received for the research, authorship, and/or publication of this article. Open access funded by Max Planck Society. RN and ES acknowledge the funding from the International Max Planck Research School for Chemistry and Physics of Quantum Materials and the funding provided by the German Research Foundation (DFG-528628333 “Complex compounds based on mercury”). OP acknowledges financial support from the Polish National Agency for Academic Exchange (NAWA) under the Bekker programme (BPN/BEK/2022/1/00190/U/00001).
Conflict of interest
The authors declare that the research was conducted in the absence of any commercial or financial relationships that could be construed as a potential conflict of interest.
Publisher’s note
All claims expressed in this article are solely those of the authors and do not necessarily represent those of their affiliated organizations, or those of the publisher, the editors and the reviewers. Any product that may be evaluated in this article, or claim that may be made by its manufacturer, is not guaranteed or endorsed by the publisher.
References
Akselrud, L., and Grin, Y. (2014). WinCSD: software package for crystallographic calculations (Version 4). J. Appl. Crystallogr. 47, 803–805. doi:10.1107/s1600576714001058
Amon, A., Svanidze, E., Cardoso-Gil, R., Wilson, M. N., Rosner, H., Bobnar, M., et al. (2018). Noncentrosymmetric superconductor BeAu. Phys. Rev. B 97, 014501. doi:10.1103/PhysRevB.97.014501
Beare, J., Nugent, M., Wilson, M., Cai, Y., Munsie, T., Amon, A., et al. (2019). muSR and magnetometry study of the type-I superconductor BeAu. Phys. Rev. B 99, 134510. doi:10.1103/physrevb.99.134510
Biehl, E., and Deiseroth, H. J. (1999). Preparation, structural relations, and magnetism of amalgams MHg11 (M: K, Rb, Ba, Sr). Z. fur Anorg. Allg. Chem. 625, 1073–1080. doi:10.1002/CHIN.199938008
Bjørklund, G. (1989). The history of dental amalgam. Tidsskr. Nor. Laegeforen 109 (34-36), 3582–3585.
Bjørklund, G., Dadar, M., Mutter, J., and Aaseth, J. (2017). The toxicology of mercury: current research and emerging trends. Environ. Res. 159, 545–554. doi:10.1016/j.envres.2017.08.051
Bruzzone, G., and Merlo, F. (1973). The calcium-mercury system. J. Less-Common Metals 32, 237–241. doi:10.1016/0022-5088(73)90091-X
Bruzzone, G., and Merlo, F. (1974). The strontium-mercury system. J. Less Common Metals 35, 153–157. doi:10.1016/0022-5088(74)90154-4
Bruzzone, G., and Merlo, F. (1975). The barium-mercury system. J. Less-Common Metals 39, 271–276. doi:10.1016/0022-5088(75)90201-5
Canfield, P., and Svanidze, E. (2024). Canfield-Svanidze crucible sets. LSP Industrial Ceramics, Inc. Available at: https://www.lspceramics.com/canfield-svanidze-crucibles/.
Chandrasekhar, B. S. (1962). A note on the maximum critical field of high-field superconductors. Appl. Phys. Lett. 1, 7–8.
Chirolli, L., Mercaldo, M. T., Guarcello, C., Giazotto, F., and Cuoco, M. (2022). Colossal orbital edelstein effect in noncentrosymmetric superconductors. Phys. Rev. Lett. 128, 217703. doi:10.1103/physrevlett.128.217703
Claeson, T., and Luo, H. L. (1966). Superconducting transition temperatures of mercury-alkaline earth metal compounds. J. Phys. Chem. solids 27, 1081–1085. doi:10.1016/0022-3697(66)90083-7
Clogston, A. M. (1962). Upper limit for the critical field in hard superconductors. Phys. Rev. Lett. 9, 266–267.
Conrad, M., Harbrecht, B., Weber, T., Jung, D. Y., and Steurer, W. (2009). Large, larger, largest–a family of cluster-based tantalum copper aluminides with giant unit cells. ii. the cluster structure. Acta Crystallogr. Sect. B Struct. Sci. 65, 318–325. doi:10.1107/s0108768109014013
Druska, C., Doert, T., and Böttcher, P. (1996). Refinement of the crystal structure of Sr3Hg2. Z. fur Anorg. Allg. Chem. 622, 401–404. doi:10.1002/zaac.19966220304
Ferro, R. (1954). The crystal structures of SrCd, BaCd, SrHg and BaHg. Acta Cryst. 7, 781. doi:10.1107/s0365110x5400240x
Finnemore, D., Mapother, D., and Shaw, R. (1960). Critical field curve of superconducting mercury. Phys. Rev. 118, 127–129. doi:10.1103/physrev.118.127
Gumiński, C. (2005). The Hg-Sr (mercury-strontium) system. J. Phase Equilibria Diffusion 26, 81–86. doi:10.1361/15477030522590
Guntz, A., and Roederer, G. (1906). Sur les amalgames de strontium. Bull. Soc. Chim. Fr. 35, 494–503.
Hänisch, J., Wimbush, S. C., Berger, L. I., and Roberts, B. W. (2023). “Properties of superconductors,” in CRC handbook of chemistry and physics. Editor J. R. Rumble (Boca Raton, FL: CRC Press/Taylor and Francis).
Hoch, C. (2019). Mein lieblingselement: quecksilber. Nachrichten aus Chem. 67, 54–60. doi:10.1002/nadc.20194090184
Hohl, T., Kremer, R. K., Ebbinghaus, S. G., Khan, S. A., Minár, J., and Hoch, C. (2023). Influence of disorder on the bad metal behavior in polar amalgams. Inorg. Chem. 62, 3965–3975. doi:10.1021/acs.inorgchem.2c04430
Kerp, W. (1898). Zur kenntnis der amalgame. Z. Anorg. Chem. 17, 284–309. doi:10.1002/zaac.18980170129
Kerp, W., Böttger, W., and Iggena, H. (1900). On the knowledge of amalgams. Z. Anorg. Chem. 25, 1–71. doi:10.1002/zaac.19000250102
Khasanov, R., Gupta, R., Das, D., Amon, A., Leithe-Jasper, A., and Svanidze, E. (2020a). Multiple-gap response of type-I noncentrosymmetric BeAu superconductor. Phys. Rev. Res. 2, 023142. doi:10.1103/physrevresearch.2.023142
Khasanov, R., Gupta, R., Das, D., Leithe-Jasper, A., and Svanidze, E. (2020b). Single-gap versus two-gap scenario: specific heat and thermodynamic critical field of the noncentrosymmetric superconductor BeAu. Phys. Rev. B 102, 014514. doi:10.1103/physrevb.102.014514
König, M., Wiedmann, S., Brüne, C., Roth, A., Buhmann, H., Molenkamp, L. W., et al. (2007). Quantum spin hall insulator state in HgTe quantum wells. Sci. 45, 766–770. doi:10.1126/science.1148047
Leithe-Jasper, A., Borrmann, H., and Hönle, W. (2006). Max Planck Institute for chemical Physics of solids, scientific report. Dresden: Max-planck-gesellschaft.
Naskar, M., Mishra, P., Ash, S., and Ganguli, A. (2021). Superconductors with noncentrosymmetric crystal structures. Bull. Mater. Sci. 44, 278. doi:10.1007/s12034-021-02587-z
Pelloquin, D., Hervieu, M., Michel, C., Tendeloo, G. V., Maignan, A., and Raveau, B. (1993). A 94 K Hg-based superconductor with a “1212” structure Hg0.5Bi0.5Sr2Ca1-xRxCu2O6+δ (R=Nd, Y, Pr). Phys. C Supercond. its Appl. 216, 257–263. doi:10.1016/0921-4534(93)90069-3
Prots, Y., Krnel, M., Grin, Y., and Svanidze, E. (2022a). Superconductivity in crystallographically disordered LaHg6.4. Inorg. Chem. 61, 15444–15451. doi:10.1021/acs.inorgchem.2c01987
Prots, Y., Krnel, M., Schmidt, M., Grin, Y., and Svanidze, E. (2022b). Uranium-mercury complex antiferromagnet: UHg6.4. Phys. Rev. B 106, L060412. doi:10.1103/PhysRevB.106.L060412
Roberts, B. W. (1976). Survey of superconductive materials and critical evaluation of selected properties. J. Phys. Chem. reference data 5, 581–822. doi:10.1063/1.555540
Sappl, J., Freund, R., and Hoch, C. (2017). Stuck in our teeth? crystal structure of a new copper amalgam, Cu3Hg. Crystals 7, 352. doi:10.3390/cryst7120352
Smith, M., Andreev, A., and Spivak, B. (2021). Giant magnetoconductivity in noncentrosymmetric superconductors. Phys. Rev. B 104, L220504. doi:10.1103/physrevb.104.l220504
Steurer, W., and Dshemuchadse, J. (2016). Intermetallics: structures, properties, and statistics, vol. 26. Oxford University Press.
Svanidze, E., Amon, A., Borth, R., Prots, Y., Schmidt, M., Nicklas, M., et al. (2019). Empirical way for finding new uranium-based heavy-fermion materials. Phys. Rev. B 99, 220403R. doi:10.1103/physrevb.99.220403
Tambornino, F., and Hoch, C. (2015). The mercury-richest europium amalgam Eu10Hg55. Z. fur Anorg. Allg. Chem. 641, 537–542. doi:10.1002/zaac.201400561
Tambornino, F., Sappl, J., and Hoch, C. (2015). The Gd14Ag51 structure type and its relation to some complex amalgam structures. J. Alloys Compd. 618, 326–335. doi:10.1016/j.jallcom.2014.08.017
Tanaka, H., Watanabe, H., and Yanase, Y. (2023). Nonlinear optical responses in noncentrosymmetric superconductors. Phys. Rev. B 107, 024513. doi:10.1103/physrevb.107.024513
Tkachuk, A. V., and Mar, A. (2008). Alkaline-earth metal mercury intermetallics A11-xHg54+x (A = Ca, Sr). Inorg. Chem. 47, 1313–1318. doi:10.1021/ic7015148
Tkachuk, A. V., and Mar, A. (2010). In search of the elusive amalgam SrHg8: a mercury-rich intermetallic compound with augmented pentagonal prisms. Dalton Trans. 39, 7132–7135. doi:10.1039/c0dt00304b
Urban, K., and Feuerbacher, M. (2004). Structurally complex alloy phases. J. non-crystalline solids 334, 143–150. doi:10.1016/j.jnoncrysol.2003.11.029
Weber, T., Dshemuchadse, J., Kobas, M., Conrad, M., Harbrecht, B., and Steurer, W. (2009). Large, larger, largest–a family of cluster-based tantalum copper aluminides with giant unit cells. i. structure solution and refinement. Acta Crystallogr. Sect. B Struct. Sci. 65, 308–317. doi:10.1107/s0108768109014001
Wendorff, M., and Röhr, C. (2018). Alkaline-earth tri-mercurides AIIHg3 (AII=Ca, Sr, Ba): binary intermetallic compounds with a common and a new structure type. Z. fur Kristallogr. - Cryst. Mater. 233, 515–529. doi:10.1515/zkri-2018-2054
Witthaut, K., Prots, Y., Zaremba, N., Krnel, M., Leithe-Jasper, A., Grin, Y., et al. (2023). Chemical and physical properties of YHg3 and LuHg3. ACS Org. Inorg. Au 3, 143–150. doi:10.1021/acsorginorgau.2c00048
Keywords: amalgams, mercurides, superconductivity, mercury, strontium, phase diagram, noncentrosymmetry
Citation: Nixon R, Prots Y, Krnel M, Zaremba N, Pavlosiuk O, Schmidt M, Akselrud L, Grin Y and Svanidze E (2024) Superconductivity in mercurides of strontium. Front. Mater. 11:1470878. doi: 10.3389/fmats.2024.1470878
Received: 26 July 2024; Accepted: 03 October 2024;
Published: 30 October 2024.
Edited by:
Alberto De La Torre, Northeastern University, United StatesReviewed by:
John Harter, University of California, Santa Barbara, United StatesBadih A. Assaf, University of Notre Dame, United States
Copyright © 2024 Nixon, Prots, Krnel, Zaremba, Pavlosiuk, Schmidt, Akselrud, Grin and Svanidze. This is an open-access article distributed under the terms of the Creative Commons Attribution License (CC BY). The use, distribution or reproduction in other forums is permitted, provided the original author(s) and the copyright owner(s) are credited and that the original publication in this journal is cited, in accordance with accepted academic practice. No use, distribution or reproduction is permitted which does not comply with these terms.
*Correspondence: Eteri Svanidze, ZXRlcmkuc3ZhbmlkemVAY3Bmcy5tcGcuZGU=