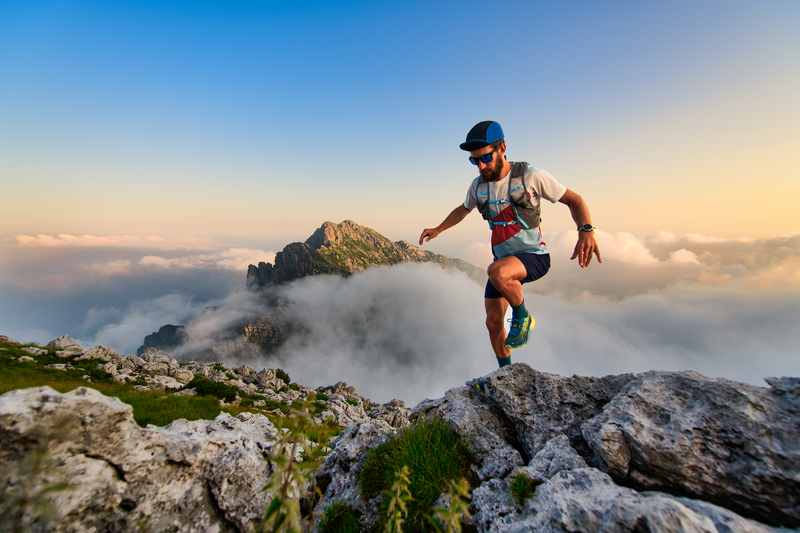
94% of researchers rate our articles as excellent or good
Learn more about the work of our research integrity team to safeguard the quality of each article we publish.
Find out more
ORIGINAL RESEARCH article
Front. Mater. , 12 February 2025
Sec. Structural Materials
Volume 11 - 2024 | https://doi.org/10.3389/fmats.2024.1450824
This article is part of the Research Topic Supplementary Cementitious Materials: Recent Developments, Performance Insights and Potential Applicability View all 5 articles
A detailed examination was carried out by substituting waste ceramic powder (WCP) for specific ratios of cement in concrete. To achieve this, five different WCP percentages (10%, 20%, 30%, 40%, and 50%) were used in manufacturing of concrete. First, the workability and slump values in the fresh state of concrete were determined by performing a slump test. Subsequently, several tests, including compressive strength (CS), splitting tensile strength (STS), and flexural strength (FS), were conducted on the specimens to assess the effectiveness of concrete fabricated using WCP. Variations in the strength were determined in terms of the various amounts of WCP. The findings demonstrated that by including WCP at levels of 10%, 20%, 30%, 40%, and 50%, there were corresponding reductions in CS of 5.8%, 21.8%, 47.1%, 63.2%, and 73.6%, respectively. The decreases in STS were 6.3%, 13.8%, 35.2%, 49.7%, and 65.4%, respectively, when a concrete STS value of 1.59 MPa was considered. Similarly, when the WCP content increased, FS was reduced by 15.3%, 21.4%, 31.6%, 44.9%, and 54.1%, respectively. This is very significant because it represents one of the key issues in calculating the optimal quantity of WCP in relation to both the strength and the amount of WCP utilized. Furthermore, taking into account our experimental research and previous studies on concrete produced utilizing WCP, straightforward equations were provided for practical use to predict CS, STS, and FS. In addition, scanning electron microscopy was done to validate the findings obtained from the experimental part of the study. The artificial neural network modeling technique was adopted to estimate the concrete properties with average coefficients of determination (R2) as 0.945 (CS), 0.901 (STS), and 0.856 (FS) with K-fold cross-validation.
Concrete is the main material used in building construction. Worldwide population growth and rural-to-urban migration have increased the global demand for concrete production. While the global rural population is currently over 3.4 billion, it is expected to decrease to 3.2 billion by 2050 (Leeson, 2018). Thus, the increasing demand for concrete necessitates improving its design and strength performance. In addition, considering the environmental effects of the production of concrete materials (i.e., cement and aggregate) (Jain et al., 2022), the use of demolished buildings and industrial or agricultural waste as alternatives to cement and concrete is very appealing (Osial et al., 2022). As the world population increases, accumulated waste can be categorized as biodegradable or non-biodegradable. While the type of waste may be important in concrete production, it is much more critical in terms of its environmental impact (Kishore and Gupta, 2020). Including waste materials in concrete production will prevent their disposal in the environment and preserve the natural resources of aggregate and cement.
In the literature, some of the industrial wastes employed instead of cement and aggregate are tire rubber (Al-Azzawi et al., 2019), glass waste (Bisht et al., 2020), ground granulated blast furnace slag (Tanwar et al., 2021), granite waste (Gupta et al., 2019), red mud (Putrevu et al., 2021), polymer-type waste (Sabău and Vargas, 2018), etc. One of the wastes with high potential for use as aggregate and cement in concrete is waste ceramic powder (WCP). Ceramics are exposed to very high temperatures (above 1,000°C), and their structural properties change; thus, WCPs cannot be recycled through ceramic production (Agrawal et al., 2021). These wastes are, therefore, generally dumped into the environment (Keshavarz and Mostofinejad, 2019). Hence, the use of WCPs in concrete can be beneficial from both environmental and economic perspectives.
Pacheco-Torgal and Jalali (2010) studied the influence of WCP on concrete performance. For this purpose, they considered four different types of WCPs: ceramic brick, once-fired stoneware, twice-fired stoneware, and sanitary ware. In their tests, 20% of cement was replaced with WCP. WCPs were also used as fine aggregate (FA) in mixtures. The compressive strength (CS) of concrete was reduced by a minimum of approximately 9% when WCPs were included. When WCPs were replaced with FA or coarse aggregate (CA), a 20% reduction in CS was observed. Keshavarz and Mostofinejad (2019) employed porcelain and red WCPs in the concrete samples with the substitution of CA at four different percentages (25%, 50%, 75%, and 100%). The concrete specimens containing porcelain wastes increased CS by up to 41%. In the case of red WCP involvement, CS increased by up to 29%. The splitting tensile strength (STS) and flexural strength (FS) of the concrete samples manufactured with partial porcelain WCP replacement increased by 41% and 67%, respectively.
In a study performed by Patel and Shah (2015), the impacts of using porcelain waste in concrete production on the mechanical, corrosion, capillary permeability, and rapid chloride penetration properties of concrete were studied. The porcelain WCP was substituted with 5%, 10%, 15%, or 20% of porcelain waste. In particular, when examining CS and FS, better values were obtained by using 15% porcelain WCP compared to a normal concrete mixture. Other mixing ratios do not lead to complete improvement, and even lower strength can be obtained. In terms of corrosion, capillary permeability and chloride permeability, the 15% porcelain-containing mixture performs well compared to normal porcelain waste percentages. Samadi et al. (2022) focused on the influence of using WCP as aggregate or cement in concrete on the characteristics of reinforced concrete beams (i.e., ultimate load at fracture, unit elongation in steel and concrete, crack development, and fracture mode). In addition to the reinforced concrete beams formed with the normal mixture (cement + aggregate), beams with 100% replacement of CA, 100% replacement of FA, 100% replacement of FA and CA, and 40% replacement of cement were tested. The results indicated that beams containing WCP exhibited lower displacement than beams produced from a normal concrete mix. The load at the time of the first crack and maximum load values were slightly less than or equal to the reference values. Likewise, in terms of the maximum moment-carrying capacity, the capacities of the reinforced concrete beams containing WCPs were the same or slightly lower than the reference value.
Sukesh et al. (2012) investigated the influence of 20% WCP on CS and durability of concrete. The results indicated a slight decrease in CS and an increase in durability. In the work by Raval et al. (2013a), cement was replaced with 10%, 20%, 30%, 40%, and 50% WCP. The findings showed that CS of concrete produced by utilizing up to 30% WCP instead of cement did not decrease much and could be considered optimum without affecting its fresh and hardened properties, while the strength of concrete samples produced with high amounts of WCP was lower. Tavakoli et al. (2013) studied the use of 10%, 20%, 30%, 40%, and 50% WCP instead of CA and 20%, 40%, 60%, 80%, and 100% WCP instead of FA in concrete production. A substantial improvement was observed in STS and CS of the concrete specimens when FA was replaced by WCP. Although replacing CA increased CS, no significant changes were observed in STS.
The impact of WCP on CS of concrete was studied by Fatima et al. (2013). Cement was substituted with up to 30% WCP in concrete. It was found that CS increased between 3.9% and 5.6% when 20% WCP was included. However, minor changes were observed in the FS and STS values when compared to the normal concrete mixture. Pacheco-Torgal and Jalali (2010) investigated the use of WCP for cement at different percentages. As a result of the experiments, when WCP was used instead of cement at a rate of 20%, an insignificant increase in CS and FS but a significant increase in durability were detected. Lopez et al. (2007) explored the use of WCP instead of FA in concrete and observed a noticeable increase in CS and FS.
In an experimental study by Pereira-de-Oliveira et al. (2012), red WCP was utilized instead of cement for concrete and mortar production. The results revealed that the addition of WCP had a positive effect on CS and STS of the concrete samples. Medina et al. (2011) studied the impact of using 20% and 25% of WCP instead of aggregate on concrete performance. It was found that the presence of WCP in concrete had a favorable effect on concrete performance as a result of the high pozzolanic reaction potential of the ceramic material. Another investigation was performed by Al-Tersawy et al. (2023). In this study, three types of industrial wastes were used to prepare concrete under standard manufacturing (cast-curing) conditions. A comprehensive examination of the chemical constituents and physical characteristics of the materials was accomplished. It was revealed that while the mechanical strength decreased, most samples produced using alkaline wastewater and 10% or 20% extra cementitious materials, or stone waste dust, achieved an acceptable concrete grade. Using 5%, 10%, 15%, and 20% sand as FA, Reda et al. (2023) examined the inclusion of granite powder (GP), iron powder (IP), brick powder (BP), and waste plastic particles (PP) in sustainable concrete production and evaluation. The increase in BP and GP proportions caused improvements in the mechanical properties of concrete. The highest value was achieved when the replacement ratio was 10%. Beyond this point, increasing the involvement of GP and BP led to a decline in the mechanical characteristics of concrete. Merwad et al. (2022) evaluated the lateral impact behavior of steel tubular columns filled with rubberized-fibrous concrete using both experimental and computational methods. The experimental program in the infilled columns included the use of four types of concrete: normal concrete (NC), rubberized concrete (RuC), steel fiber concrete (SFC), and hybrid RuC-SFC. The results revealed that RuC exhibited comparable lateral impact resistance to conventional concrete in the columns (Merwad et al., 2022). In another investigation performed by Mubaraki et al. (2019), FS and mode-I fracture toughness of flexible pavements were analyzed by considering various proportions of recycled reclaimed asphalt pavement. Two distinct percentages (20% and 40%) were analyzed. The experimental findings illustrated that FS and mode-I fracture toughness of flexible pavements, including 40% reclaimed asphalt pavement, surpassed those of flexible pavements without reclaimed asphalt pavement for both types of reclaimed asphalt pavement. Mubaraki et al. (2013) conducted experimental and computational investigations on the fracture behavior of recycled tire-rubber-filled concrete, specifically focusing on mixed-mode fracture. This study aimed to assess the impact of substituting 10% FA by volume with crumb rubber on fracture toughness, fracture initiation angle, and fracture course for several mode mixes. The fracture toughness was unaffected by the substitution of 10% sand with rubber particles, regardless of the mixity (Mubaraki et al., 2013).
As observed above, the analysis of the literature has displayed that most of the few experiments that have been conducted on concrete, including WCP, have focused on curing techniques. Few studies have explored the use of WCP as a constituent in concrete, specifically at a precise ratio, to determine the optimal ratio for this purpose. This study is noteworthy because it investigates the effects of different WCPs on concrete performance. For this purpose, cement was replaced by WCP at different percentages. The slump test was performed on fresh concrete. Subsequently, CS, STS, and FS tests were performed. Scanning electron microscopy (SEM) was also conducted. The results are presented with the strength values obtained from past studies, along with the best-fit curve and related empirical formulations involving the WCP content. The structure of this article consists of three main parts: materials and methods are presented first, followed by test results obtained from concrete samples. Finally, the proposed empirical models are discussed.
This investigation covers the impact of substituting cement with WCP (Figure 1) on the mechanical characteristics of concrete. In order to assess the mechanical properties of concrete containing WCP, several combinations were prepared and tested. Sustainable concrete production was studied by considering a CEM I 32.5 type of cement with certain FA and CA ratios obtained from WCP. The properties of the mixtures are listed in Table 1. Three categories of tests were conducted to assess the efficiency of concrete containing WCP. CS testing was carried out on 15 cm × 15 cm × 15 cm cubic samples, while STS tests were undertaken on cylindrical samples measuring 10 cm × 20 cm. In addition, FS tests were conducted on pieces measuring 10 cm × 10 cm × 40 cm. Three repetitions were applied to each test combination. ASTM C39/C39M (2014) was followed when performing the CS tests. The STS and FS tests were conducted according to ASTM (2017) and ASTM (2010). One of the main aims of this research work was to identify the optimum amount of WCP in terms of strength. Pursuant to this goal, 10%, 20%, 30%, 40%, and 50% of cement was substituted with WCP. The design of the mixture used in the experiments for reference samples was as follows: cement 580 kg/m3, water 270 kg/m3, FA 780 kg/m3, and CA 900 kg/m3.
The primary objective of this study was to conduct a thorough analysis of various cement production assemblies by utilizing different quantities of WCP as a replacement for cement. To achieve this objective, cement was replaced by WCP at rates of 10%, 20%, 30%, 40%, and 50%, as previously mentioned. Subsequently, FS, CS, and STS tests were performed. Moreover, analysis using SEM was carried out. A modeling technique called artificial neural networks (ANNs) was also used. The results obtained are described in the following subsections.
The slump test results are presented in Figure 2. Because of its large specific surface area compared to that of cement, the initial slump values tend to decrease as the degree of WCP incorporation increased (El-Dieb et al., 2019). The findings of this study are comparable to those of earlier studies. In addition, the incorporation of WCP resulted in an increase in the workability. One possible explanation for this is that WCP does not exhibit any hydraulic response, and its pozzolanic reaction is weak (El-Dieb et al., 2019).
To perform the CS test, the CS test specimens were not deteriorated. The purpose of this test was to determine the capability of the samples to fail under force. The CS testing of all the concrete samples was conducted in accordance with ASTM C39 provisions (ASTM, 2014). In order to achieve this aim, the test specimen’s dimensions were selected as 150 mm × 150 mm × 150 mm. The CS values for 28-day period mixes are depicted in Figure 3.
As presented in Figure 3, cement was altered by the use of 10%, 20%, 30%, 40%, and 50% WCP; this disposition was inverted as a graded replacement of cement by WCP, reducing CS. Detailed analysis of the test consequence demonstrated that a graded exchange of WCP for cement caused a sharp decrease in the CS values. As the WCP replacement ratio increased by 10%, 20%, 30%, 40%, and 50%, the 28-day CS was reduced by 5.7%, 21.8%, 47.2%, 63.2%, and 73.6%, respectively. When WCP was used instead of cement, a remarkable reduction in CS was observed compared to the reference sample. These results are in line with the strengths described by Lavat et al. (2009) and Sánchez de Rojas et al. (2018). From these results, it can be concluded that WCP exhibits pozzolanic reactivity. Furthermore, the main reason for the reduction in CS is the smooth surface texture and flaky nature of WCP, which probably led to poor bonding with the matrix (Ikponmwosa and Ehikhuenmen, 2017). The smooth surface of WCP results in poor mechanical adherence with the matrix and is probably the cause of the decay curve in CS (Canbaz, 2016). In addition, a higher WCP content in the concrete mix increases the amount of silica, and the formation of calcium hydroxide following cement hydration can also decrease CS (Hamad et al., 2020).
A comparative evaluation of STS of normal concrete and concrete obtained by substituting cement with WCP in various proportions is demonstrated in Figure 4. The trend of STS was almost similar to that of CS. Figure 4 shows that STS changed with the percentage increase of WCP. The strength of concrete decreased as the WCP content increased.
The general model in this study concluded that STS of concrete tended to decrease with increasing WCP. The maximum STS of the sample for the 28th day was concrete containing 10% WCP with a strength of 1.49 MPa, which was a difference of 6.3% compared to the reference sample, and the minimum STS value was the test specimen containing 50% WCP. The relationship between STS and CS is displayed in Figure 5. The model comprising CS and STS is flat. Moreover, the dashed line with an R2 value above 98% in Figure 5 indicates a strong relationship between CS and STS.
The FS results of the specimens are depicted in Figure 6. As observed from Figure 6, the strength values ranged from 4.5 to 9.8 MPa. Figure 6 exhibits that by adding WCP at 10%, 20%, 30%, 40%, and 50% of the cement weight, a decrease in FS was detected as 15.3%, 21.4%, 31.6%, 44.9%, and 54.1%, respectively, compared to the reference sample (9.8 MPa). After a 10% exchange, a significant decrease in FS of the samples was observed. This may be due to a decrease in the number of accessible cementing requirements. Figure 7 shows a straight-line link between FS of the test specimen and the percentage of WCP addition. At 10% WCP, the reduction in FS was 15.3%, compared to FS of the reference sample (9.8 MPa). The relationship between FS and CS is displayed in Figure 7. The dashed line with an R2 value above 95% indicates a strong relationship between CS and FS.
In this section of the work, the CS, STS, and FS properties of the concrete test specimens with the different percent admixtures of WCP replacing cement were investigated. Subsequently, median and best-fit curves consisting of three empirical equations are presented to represent the relationship between mechanical properties (CS, STS, and FS) and WCP amount. Because the mechanical properties of reference concrete samples (i.e., without WCP involvement in the concrete mixture) have different strength values, the absolute strength values cannot be directly evaluated. Therefore, all absolute strength values obtained from the literature and this study are normalized by their reference strength properties.
It is important to emphasize that the CS values utilized in Figure 8 were taken from the studies by Abdullah Anwar et al. (2015), Agil and Kumar (2017), Arthi (2016), Ay and Ünal (2000), Bhargav and Kansal (2020), El-Dieb et al. (2018), Fatima et al. (2013), Hilal et al. (2021), Kanchidurai et al. (2017), Kannan et al. (2017), Kumar and Reddy (2017), Lasseuguette et al. (2019), Manigandan and Saravanakumar (2017), Mohit and Sharifi (2019), Nasr et al. (2018), Patel and Shah (2015), Raval et al. (2013a), Tanwar et al. (2021), and Vejmelkova et al. (2012). The STS rates used in Figure 9 were adopted from the works by Agil and Kumar (2017), Fatima et al. (2013), and Kumar and Reddy (2017). Similarly, the FS values were obtained from the studies by Bhargav and Kansal (2020), Fatima et al. (2013), Kumar and Reddy (2017), Mohit and Sharifi (2019), Nasr et al. (2018), Patel and Shah (2015), and Vejmelkova et al. (2012). Data for FS are depicted in Figure 10.
Figure 8. Changes of normalized CS characteristics of concrete specimens treated with various amounts of WCP.
Figure 9. Changes of normalized STS characteristics of concrete specimens treated with various amounts of WCP.
Figure 10. Changes of normalized FS characteristics of concrete specimens treated with various amounts of WCP.
The best-fit curve is complemented by the median CS values of the present available associated data at 0%, 5%, 10%, 15%, 20%, 30%, 40%, and 50% of the WCP use, as shown in Figure 8. The corresponding polynomial equation describing the changes in the CS is given in Equation 1:
where fc is the CS value of concrete with WCP, fc′ is the CS value of the plain concrete, and WCP is the amount of WCP substituted for cement in weight percentage. Based on the data illustrated above, CS did not substantially change when up to 20% of WCP was involved, which is consistent with the literature results. The use of more than 20% WCP was found to lead to a significant decrease in CS. For instance, the use of 30% and 40% WCP resulted in 14% and 20% reductions in CS, compared to the reference value, respectively. The reduction became even more dramatic with 40% WCP.
The changes in STS as a function of WCP can be represented by a best-fit curve equation with an accuracy of approximately 92%, which is given in Equation 2.
where ft is the STS value of concrete with WCP and ft′ is the STS value of plain concrete. It can be concluded from the best-fit curve and the median values that WCP amounting to 10% of the cement replacement did not have a clear impact on the STS value. However, above that amount, there was a considerable reduction with increasing the WCP content. More clearly, the observed reductions were 22.5%, 20%, and 34% for the WCP contents of 15%, 20%, and 30%, respectively. The findings of this study are compatible with the trends obtained from the literature, as highlighted previously.
The normalized FS values from the literature and this study are plotted in Figure 10, along with their median and best-fit curves. The empirical formula (Equation 3) describing the FS characteristics of the WCP-blended concrete mixes can be written as:
where ff is the FS value of concrete with WCP and ff′ is the FS value of the reference concrete. Increasing the WCP content up to 20% in the concrete specimens did not cause a decrease in FS. In fact, FS increased by approximately 10% when 5% or 15% WCP was used. However, at 30% and 40% WCP contents, FS of the concrete specimens was reduced by 23% and 27%, respectively. A more drastic reduction (approximately 54%) was observed in the case of 50% WCP.
SEM analysis was conducted to analyze and enhance the final properties of WCP-blended concrete. Figure 11A reveals a well-integrated pore structure with crack-free and delamination-free surfaces. These findings are consistent with those reported in similar studies (Yu et al., 2019). The ettringite seen in Figure 11B is a mineral phase that typically forms during the early stages of the hydration process. It helps increase the initial strength of cement; however, excessive amounts and late formation can negatively impact the long-term durability of concrete. When Portland cement mixes with water, aluminates (tricalcium aluminate, C₃A) and calcium sulfate (usually gypsum) react with water. As a result of this reaction, crystalline structures called ettringite are formed. Tricalcium aluminate (C₃A) in the cement combines with dissolved calcium sulfate (CaSO₄) in a hydrated environment to form ettringite (3CaO•Al₂O₃•3CaSO₄•32H₂O) crystals. These crystals form fine needle-like structures in the microstructure of concrete. If there is an excess of C₃A in cement or if concrete is overly exposed to water, late-stage ettringite formation can occur. In this case, ettringite continues to grow and fills the voids in concrete. This can cause expansion and sometimes cracking. The cracks in Figure 11B could potentially be attributed to delayed ettringite formation (Lubej et al., 2016; Talero, 2005; Talero, 2007). The formation of the calcium silicate hydrate (C-S-H) structure is the primary reaction that occurs during the hydration process of concrete and provides a significant portion of the concrete strength. The formation of C-S-H in the microstructure of concrete results from the reaction between cement and water. Figures 11C, D illustrate the formation of C-S-H in the concrete microstructure. The images show how C-S-H fills the voids between cement particles and binds them together, thereby enhancing the strength of concrete.
ANNs are commonly used in different engineering applications because of their capacity to model complex nonlinear systems. An ANN consists of nodes or neurons interconnected by nodes, which may be studied to execute tasks by regulating the strengths of the connections.
An ANN was used in this section to predict engineering properties (CS, STS, and FS) (using concrete mix ratio) with the help of experimental data obtained from the literature. Data about 286 concrete test specimens were collected from Patel and Shah (2015), Raval et al. (2013a), El-Dieb et al. (2018), Kannan et al. (2017), Atkuri and Rao (2021), Raval et al. (2013b), and Abou Rachied et al. (2023) to estimate CS. Data about 143 concrete test specimens were collected from Atkuri and Rao (2021), Nalli and Vysyaraju (2022), Attaelmanan et al. (2021), and Subaşı et al. (2017) to quantify STS. Data about 138 concrete test specimens were collected from the literature to estimate FS. Six input parameters, namely, cement (kg/m3), WCP amount (kg/m3), FA (kg/m3), CA (kg/m3), water (kg/m3), and age of specimens (days), and one output parameter, CS (MPa), were collected. Most (70%) of the data were employed for training the data, and the remaining 30% were utilized for testing data lists (Attaelmanan et al., 2021; Subaşı et al., 2017). Table 2 explores the minimum, maximum, and mean values for CS, STS, and FS of WCP-blended concrete with partial replacement of cement by WCP.
The Levenberg–Marquardt algorithm (LMA), an effective implementation algorithm, can be used to train ANNs to predict the performance of concrete, such as CS, STS, and FS, as output variables, based upon input variables, for example, mix proportions and age of specimens. In order to optimize the mixture design of WCP-blended concrete, ANNs were applied to predict concrete properties and reduce the costs, environmental impact, and energy associated with concrete production. A normalization technique was adopted prior to pre-processing the collected data. The normalization method was utilized in this modeling, as displayed in Equation 4. Furthermore, a scaling technique has been recommended for this approach in the literature.
Two methods, the feedforward propagation method and the mean square performance error, were used in this study. The number of “epochs” that the learning algorithm cycles through the entire training sample is a hyperparameter.
The coefficient of determination (R2) is a statistical measure that indicates the proportion of variance in the dependent variable, which is explained by the independent variable(s). It is often employed in regression analysis to identify the strength of relationships between dependent and independent variables. R2 ranges from 0 to 1, with higher values demonstrating a superior relationship between variables.
A two-layer (one input layer and one output layer) network with seven neurons in the input layer was selected to construct the proposed ANN models. For both the input and output layers, a nonlinear (TANSIG) transfer function was used. In this study, the number of neurons was seven (six input variables and one output variable) with one layer. The performance model for CS of concrete using LMA by means of an ANN is illustrated in Figures 12, 13. The performance of the epoch model in predicting CS of WCP-blended concrete is shown in Figure 12, and the effectiveness of the ANN model in identifying CS is depicted in Figure 13.
K-fold cross-validation was employed to obtain the best model for identifying CS of WCP-blended concrete. K-fold cross-validation was utilized to obtain the best combination of predicted data, as displayed in Figure 14. The collected data were split into 10 sub-sets that were combined in different forms to obtain the best predicted CS.
where
Cn—Normalized cement content.
WCPn—Normalized WCP.
FAn—Normalized natural FA.
CAn—Normalized natural CA.
Wn—Normalized water.
An—Normalized age of specimen.
CSn—Normalized CS.
Figure 14. K-fold (K = 8) for predicting CS using ANN. Normalized CS value is determined by Equation 5. Terms A1 to A7 are constant, with normalized values for cement (C) content (kg/m3), WCP content (kg/m3), FA content (kg/m3), CA content (kg/m3), water (W) content (kg/m3), age of specimens (A) (days), and CS (N/mm2).
Figure 15 demonstrates the predicted CS values from the ANN model; the ANN equation remains the same.
A two-layer (one input layer and one output layer) network with seven neurons in the input layer and one neuron in the output layer was selected to construct the proposed ANN models. A nonlinear (TANSIG) transfer function was used for both the input and output layers. The number of neurons was seven (six input variables and one output variable) with one layer. The performance model for STS of concrete with partial replacement of cement by WCP utilizing LMA via ANN is presented in Figures 16, 17. The performance of the epoch model to predict STS in WCP-blended concrete is indicated in Figure 16, and the performance of the ANN model to identify STS is demonstrated in Figure 17.
K-fold cross-validation is required to assess the most appropriate dataset for the most appropriate combination of data to obtain the maximum R2. K-fold cross-validation is illustrated in Figure 18 for predicting STS using ANN.
K-fold cross-validation was utilized to obtain the best model for estimating STS of WCP-blended concrete. K-fold cross-validation was employed to obtain the best combination of predicted data. The collected data were split into 10 sub-sets that were combined in different forms to obtain the best predicted STS.
The normalized STS value is determined by Equation 6. Terms B1–B7 are constant, with normalized values for cement (C) content (kg/m3), WCP content (kg/m3), FA content (kg/m3), CA content (kg/m3), water (W) content (kg/m3), age of specimen (A) (days), and STS (N/mm2).
where
Cn—Normalized cement content.
WCPn—Normalized CEP.
FAn—Normalized natural FA.
CAn—Normalized natural CA.
Wn—Normalized water.
An—Normalized age of specimen.
STSn—Normalized STS.
Figure 19 display the predicted STS values from the ANN model, where the ANN equation remains the same.
A two-layer (one input layer and one output layer) network with seven neurons in the input layer and one neuron in the output layer was selected for building the developed ANN models to predict FS. A nonlinear (TANSIG) transfer function was employed for both the input and output layers. The number of neurons was seven (six input variables and one output variable) with one hidden layer. The performance model for FS of concrete with partial replacement of cement by WCP using LMA via ANN is illustrated in Figures 20, 21. The performance of the epoch model in predicting FS of WCP-blended concrete is demonstrated in Figure 20, and the performance of the ANN model in estimating FS is depicted in Figure 21.
K-fold cross-validation is required to assess the most appropriate dataset for the most appropriate combination of data to obtain the optimized R2. K-fold cross-validation is indicated in Figure 22 for predicting FS using ANN.
K-fold cross-validation was utilized to obtain the best model for estimating FS of WCP-blended concrete. K-fold cross-validation was employed to obtain the best combination of predicted data. The collected data were split into 10 sub-sets, which were combined in different forms to obtain the best predicted FS.
The normalized FS value is determined from Equation 7, where terms C1–C7 are constant with normalized value for cement (C) content (kg/m3), WCP content (kg/m3), FA content (kg/m3), CA content (kg/m3), water (W) content (kg/m3), age of specimen (A) (days), and FS (N/mm2).
where
Cn—Normalized cement content.
WCPn—Normalized WCP.
FAn—Normalized natural FA.
CAn—Normalized natural CA.
Wn—Normalized water.
An—Normalized age of specimen.
FSn—Normalized FS.
Figure 23 shows the predicted FS values from the ANN model, where the ANN equation remains the same. A similar prediction of concrete properties was reported in the literature for different kinds of blended concretes.
In this study, we focused on examining how the mechanical characteristics of concrete are affected when WCP is substituted with cement. Different amounts of WCP as 10%, 20%, 30%, 40%, and 50%, were used instead of cement. While the slump and workability properties of the test samples were evaluated in their fresh state, CS, STS, and FS tests were carried out in hardened conditions. Moreover, SEM analyses were also conducted to equate the strength implications obtained from the research. In the last stage of the study, simple equations were developed to estimate the CS, STS, and FS properties of the produced concrete, taking into account our experimental research and previous studies conducted by different research teams. The ANN modeling technique was also adopted to estimate the above-mentioned concrete properties. Considering this study, the following results can be identified:
• As cement was replaced by WCP, a proportional reduction in CS was observed. With the addition of 10%, 20%, 30%, 40%, and 50% WCP, a reduction in CS of 5.8%, 21.8%, 47.1%, 63.2%, and 73.6% was detected, respectively. Compared to similar results in the literature, the reason for this decrease was the smooth surface texture and flaky nature of WCP, which probably explains the weak bonding with the concrete matrix.
• The STS test results exhibited that the STS values had a similar trend to CS. At 10% implantation of WCP, STS was only 13.8% less than the reference concrete sample.
• The incorporation of WCP into the cement led to a decrease in the FS values to a certain extent. Using WCP at 10%, 20%, 30%, 40%, and 50% reduced the FS value by 15.3%, 21.4%, 31.6%, 44.9%, and 54.1%, respectively.
• The ANN modeling technique was adopted to estimate CS with K-fold cross-validation as K = 8 with an average R2 value of 0.945. A similar algorithm was employed to predict STS with K-fold cross-validation as K = 5, with an average R2 value of 0.901. The same algorithm and normalization techniques were utilized to estimate FS with K-fold cross-validation as K = 7 with an average R2 value of 0.856.
• ANN modeling techniques proposed equations to accurately estimate the CS, STS, and FS values of concrete.
The raw data supporting the conclusions of this article will be made available by the authors, without undue reservation.
YÖ: conceptualization, formal analysis, investigation, methodology, validation, writing–original draft, and writing–review and editing. AB: conceptualization, formal analysis, investigation, methodology, resources, validation, writing–original draft, and writing–review and editing. YG: conceptualization and writing–original draft. AS: methodology and writing–original draft. MK: investigation and writing–original draft. EA: validation and writing–original draft. AC: methodology and writing–original draft. ÖZ: conceptualization and writing–original draft. PJ: validation and writing–original draft.
The author(s) declare that financial support was received for the research, authorship, and/or publication of this article.
The authors extend their appreciation to the Deanship of Scientific Research at King Khalid University, Abha, Saudi Arabia, for providing support to this research work through Large Groups RGP2/447/45.
The authors declare that the research was conducted in the absence of any commercial or financial relationships that could be construed as a potential conflict of interest.
All claims expressed in this article are solely those of the authors and do not necessarily represent those of their affiliated organizations, or those of the publisher, the editors, and the reviewers. Any product that may be evaluated in this article, or claim that may be made by its manufacturer, is not guaranteed or endorsed by the publisher.
Abdullah Anwar, S. A., Mohd, S., Husain, A., and Ahmad, S. A. (2015). Replacement of cement by marble dust and ceramic waste in concrete for sustainable development. Int. J. Innovative Sci. Eng. Technol. (IJISET) 2, 496–503.
Abou Rachied, T., Dbouk, F., Hamad, B., and Assaad, J. J. (2023). Structural behavior of beams cast using normal and high strength concrete containing blends of ceramic waste powder and blast furnace slag. Clean. Mater. 7, 100179. doi:10.1016/j.clema.2023.100179
Agil, R., and Kumar, A. (2017). Experimental study on replacement of cement with ceramic tile powder in concrete. Int. J. Res. Appl. Sci. Eng. Technol. 5, 1814–1818.
Agrawal, A., Saravanan, T. J., Bisht, K., and Kabeer, KISA (2021). Synthesis of cement composites utilizing ceramic waste as a partial replacement for Portland cement: literature review. J. Hazard. Toxic, Radioact. Waste 25, 03121003. doi:10.1061/(asce)hz.2153-5515.0000637
Al-Azzawi, A. A., Saad, N., and Shakir, D. (2019). Behavior of hybrid concrete beams with waste rubber. Computers and Concrete. An Int. J. 23, 245–253.
Al-Tersawy, S. H., Zakey, S. E., El-Sadany, R. A., and Sallam, H. E.-D. M. (2023). Utilization of various industrial wastes in ordinary concrete under normal manufacturing conditions. Int. J. Concr. Struct. Mater. 17, 44. doi:10.1186/s40069-023-00603-6
Arthi, A. J. J. (2016). Effective replacement of cement by ceramic waste in concrete for sustainable development. Int. J. Res. Eng. Technol. 5.
ASTM (2010). Standard test method for flexural strength of concrete (using simple beam with center-point loading). ASTM international.
ASTM (2014). Standard test method for compressive strength of cylindrical concrete specimens. ASTM international.
ASTM (2017). Standard test method for splitting tensile strength of cylindrical concrete Specimens1. ASTM international.
Atkuri, V. K., and Rao, G. R. (2021). “Strength properties of ceramic waste concrete,” in IOP Conference Series: Materials Science and Engineering. IOP Publishing. 1025 (1), 012017.
Attaelmanan, M., Kambal, M. E. M., and Mansour, M. I. (2021). A study the effect of using ceramic waste powder as partial replacement for cement on concrete properties. Journal of Karary University for Engineering and Science.
Ay, N., and Ünal, M. (2000). The use of waste ceramic tile in cement production. Cem. Concr. Res. 30, 497–499. doi:10.1016/s0008-8846(00)00202-7
Bhargav, M., and Kansal, R. (2020). Experimental investigation to substitute of cement with ceramic tiles powder in concrete. Int. J. Res. Appl. Sci. Eng. Technol. 8, 302–307. doi:10.22214/ijraset.2020.31363
Bisht, K., Kabeer, KISA, and Ramana, P. V. (2020). Gainful utilization of waste glass for production of sulphuric acid resistance concrete. Constr. Build. Mater. 235, 117486. doi:10.1016/j.conbuildmat.2019.117486
Canbaz, M. (2016). The effect of high temperature on concrete with waste ceramic aggregate. Iran. J. Sci. Technol. Trans. Civ. Eng. 40, 41–48. doi:10.1007/s40996-016-0002-7
El-Dieb, A. S., Taha, M. R., and Abu-Eishah, S. I. (2019). The use of ceramic waste powder (CWP) in making eco-friendly concretes. Ceramic Materials: synthesis, Characterization. Appl. Recycl., 1–35.
El-Dieb, A. S., Taha, M. R., Kanaan, D., and Aly, S. T. (2018). Ceramic waste powder: from landfill to sustainable concretes. Proc. Institution Civ. Engineers-Construction Mater. 171, 109–116. doi:10.1680/jcoma.17.00019
Fatima, E., Jhamb, A., and Kumar, R. (2013). Ceramic dust as construction material in rigid pavement. Am. J. Civ. Eng. Archit. 1, 112–116. doi:10.12691/ajcea-1-5-5
Gupta, L. K., Kabeer, K. S. A., and Vyas, A. K. (2019). “Effect on physical and mechanical properties of cement mortar prepared with waste granite powder as secondary aggregate,” In UKIERI Concr Congress.
Hamad, A. J., Sldozian, R. J. A., and Mikhaleva Z, A. (2020). Effect of ceramic waste powder as partial fine aggregate replacement on properties of fiber-reinforced aerated concrete. Eng. Rep. 2, e12134. doi:10.1002/eng2.12134
Hilal, N., Saleh, R. D., Yakoob, N. B., and Banyhussan, Q. S. (2021). Utilization of ceramic waste powder in cement mortar exposed to elevated temperature. Innov. Infrastruct. Solutions 6, 35. doi:10.1007/s41062-020-00403-x
Ikponmwosa, E. E., and Ehikhuenmen, S. O. (2017). The effect of ceramic waste as coarse aggregate on strength properties of concrete. Niger. J. Technol. 36, 691–696. doi:10.4314/njt.v36i3.5
Jain, P., Gupta, R., and Chaudhary, S. (2022). A literature review on the effect of using ceramic waste as supplementary cementitious material in cement composites on workability and compressive strength. Mater. Today Proc. 65, 871–876. doi:10.1016/j.matpr.2022.03.453
Kanchidurai, S., Bharani, G., and Mohan, K. S. R. (2017). Strength and durability studies on concrete with partial replacement over burnt brick bat waste. In IOP Conference Series: Earth and Environmental Science 80 (1), 012018.
Kannan, D. M., Aboubakr, S. H., El-Dieb, A. S., and Reda, T. M. M. (2017). High performance concrete incorporating ceramic waste powder as large partial replacement of Portland cement. Constr. Build. Mater. 144, 35–41. doi:10.1016/j.conbuildmat.2017.03.115
Keshavarz, Z., and Mostofinejad, D. (2019). Porcelain and red ceramic wastes used as replacements for coarse aggregate in concrete. Constr. Build. Mater. 195, 218–230. doi:10.1016/j.conbuildmat.2018.11.033
Kishore, K., and Gupta, N. (2020). Application of domestic and industrial waste materials in concrete: a review. Mater. Today Proc. 26, 2926–2931. doi:10.1016/j.matpr.2020.02.604
Kumar, V. P., and Reddy, K. C. (2017). Durability aspects of concrete by partial replacement of cement by ceramic waste. Int. J. Civ. Eng. 8, 22–30.
Lasseuguette, E., Burns, S., Simmons, D., Francis, E., Chai, H. K., Koutsos, V., et al. (2019). Chemical, microstructural and mechanical properties of ceramic waste blended cementitious systems. J. Clean. Prod. 211, 1228–1238. doi:10.1016/j.jclepro.2018.11.240
Lavat, A. E., Trezza, M. A., and Poggi, M. (2009). Characterization of ceramic roof tile wastes as pozzolanic admixture. Waste Manag. 29, 1666–1674. doi:10.1016/j.wasman.2008.10.019
Leeson, G. W. (2018). The growth, ageing and urbanisation of our world. J. Popul. Ageing 11, 107–115. doi:10.1007/s12062-018-9225-7
Lopez, V., Llamas, B., Juan, A., Moran, J. M., and Guerra, I. (2007). Eco-efficient concretes: impact of the use of white ceramic powder on the mechanical properties of concrete. Biosyst. Eng. 96, 559–564. doi:10.1016/j.biosystemseng.2007.01.004
Lubej, S., Anžel, I., Jelušič, P., Kosec, L., and Ivanič, A. (2016). The effect of delayed ettringite formation on fine grained aerated concrete mechanical properties. Sci. Eng. Compos. Mater. 23, 325–334. doi:10.1515/secm-2012-0107
Manigandan, S., and Saravanakumar, G. S. (2017). Experimental study on partial replacement of cement by using ceramic waste powder. International Journal for Research in Applied Science and Engineering-Technology IJRASET.
Medina, C., de Rojas, M. I. S., Frías, M., and Juan, A. (2011). “Using ceramic materials in ecoefficient concrete and precast concrete products,” in Advances in ceramics-electric and magnetic ceramics, bioceramics, ceramics and environment. IntechOpen.
Merwad, A. M., El-Sisi, A. A., Mustafa, S. A., and Sallam, H. E.-D. M. (2022). Lateral impact response of rubberized-fibrous concrete-filled steel tubular columns: experiment and numerical study. Buildings 12, 1566. doi:10.3390/buildings12101566
Mohit, M., and Sharifi, Y. (2019). Thermal and microstructure properties of cement mortar containing ceramic waste powder as alternative cementitious materials. Constr. Build. Mater. 223, 643–656. doi:10.1016/j.conbuildmat.2019.07.029
Mubaraki, M., Abd-Elhady, A. A., and Sallam, H. (2013). Mixed mode fracture toughness of recycled tire rubber-filled concrete for airfield rigid pavements. Int. J. Pavement Res. Technol. 6, 8–14.
Mubaraki, M., Osman, S., and Sallam, H. (2019). Effect of RAP content on flexural behavior and fracture toughness of flexible pavement. Lat. Am. J. Solids Struct. 16. doi:10.1590/1679-78255516
Nalli, B. R., and Vysyaraju, P. (2022). “Utilization of ceramic waste powder and rice husk ash as a partial replacement of cement in concrete,” in IOP Conference Series: Earth and Environmental Science. IOP Publishing. 982 (1), 012003.
Nasr, M. S., Hussain, T. H., and Najim, W. N. (2018). Properties of cement mortar containing biomass bottom ash and sanitary ceramic wastes as a partial replacement of cement. Int. J. Civ. Eng. Technol. (IJCIET) 9, 153–165.
Osial, M., Pregowska, A., Wilczewski, S., Urbańska, W., and Giersig, M. (2022). Waste management for green concrete solutions: a concise critical review. Recycling 7, 37. doi:10.3390/recycling7030037
Pacheco-Torgal, F., and Jalali, S. (2010). Reusing ceramic wastes in concrete. Constr. Build. Mater. 24, 832–838. doi:10.1016/j.conbuildmat.2009.10.023
Patel, V., and Shah, N. (2015). Durability properties of porcelain waste based high-performance concrete. Mag. Concr. Res. 67, 187–196. doi:10.1680/macr.14.00189
Pereira-de-Oliveira, L. A., Castro-Gomes, J. P., and Santos, P. M. S. (2012). The potential pozzolanic activity of glass and red-clay ceramic waste as cement mortars components. Constr. Build. Mater. 31, 197–203. doi:10.1016/j.conbuildmat.2011.12.110
Putrevu, M., Thiyagarajan, J. S., Pasla, D., Kabeer, KISA, and Bisht, K. (2021). Valorization of red mud waste for cleaner production of construction materials. J. Hazard. Toxic, Radioact. Waste 25, 03121002. doi:10.1061/(asce)hz.2153-5515.0000629
Raval, A. D., Patel, I. N., and Pitroda, J. (2013a). Re-use of ceramic industry wastes for the elaboration of eco-efficient concrete. Int. J. Adv. Eng. Res. Stud. 2, 103–105.
Raval, A. D., Patel, I. N., and Pitroda, J. (2013b). Eco-efficient concretes: use of ceramic powder as a partial replacement of cement. Int. J. Innovative Technol. Explor. Eng. (IJITEE) 3, 1–4.
Reda, R. M., Mahmoud, H. S., Ahmad, S. S., and Sallam, H. E.-D. M. (2023). Mechanical properties of sustainable concrete comprising various wastes. Sci. Rep. 13, 13234. doi:10.1038/s41598-023-40392-2
Sabău, M., and Vargas, J. R. (2018). Use of e-plastic waste in concrete as a partial replacement of coarse mineral aggregate. Comput. Concr. 21, 377–384.
Samadi, M., Baghban, M. H., Kubba, Z., Faridmehr, I., Abdul Shukor Lim, N. H., Benjeddou, O., et al. (2022). Flexural behavior of reinforced concrete beams under instantaneous loading: effects of recycled ceramic as cement and aggregates replacement. Buildings 12, 439. doi:10.3390/buildings12040439
Sánchez de Rojas, M. I., Frías, M., Sabador, E., Asensio, E., Rivera, J., and Medina, C. (2018). Use of ceramic industry milling and glazing waste as an active addition in cement. J. Am. Ceram. Soc. 101, 2028–2037. doi:10.1111/jace.15355
Subaşı, S., Öztürk, H., and Emiroğlu, M. (2017). Utilizing of waste ceramic powders as filler material in self-consolidating concrete. Constr. Build. Mater. 149, 567–574. doi:10.1016/j.conbuildmat.2017.05.180
Sukesh, C., Katakam, B. K., Saha, P., and Chamberlin, K. S. (2012). “A study of sustainable industrial waste materials as partial replacement of cement,” in IACSIT Coimbatore Conferences 28.
Talero, R. (2005). Performance of metakaolin and Portland cements in ettringite formation as determined by ASTM C 452-68: kinetic and morphological differences. Cem. Concr. Res. 35, 1269–1284. doi:10.1016/j.cemconres.2004.10.002
Talero, R. (2007). Performance of metakaolin and portland cements in ettringite formation as determinated by le chatelier-ansttet test: kinetic and morphological differences and new specification. Silic. Ind., 191–204.
Tanwar, V., Bisht, K., Ahmed Kabeer, K. I. S., and Ramana, P. V. (2021). Experimental investigation of mechanical properties and resistance to acid and sulphate attack of GGBS based concrete mixes with beverage glass waste as fine aggregate. J. Build. Eng. 41, 102372. doi:10.1016/j.jobe.2021.102372
Tavakoli, D., Heidari, A., and Karimian, M. (2013). Properties of concretes produced with waste ceramic tile aggregate. Asian J. Civ. Eng. 14, 369–382.
Vejmelkova, E., Kulovana, T., Keppert, M., Konvalinka, P., Ondracek, M., and Sedlmajer, M. (2012). “Application of waste ceramics as active pozzolana in concrete production,” in IACSIT Coimbatore Conference. 28, 132–136.
Yu, C.-J., Ri, B.-H., Kim, C.-H., Hwang, U.-S., Ri, K.-C., Song, C.-J., et al. (2019). Formation and characterization of ceramic coating from alumino silicate mineral powders in the matrix of cement composite on the concrete wall. Mater. Chem. Phys. 227, 211–218. doi:10.1016/j.matchemphys.2019.02.012
Keywords: waste ceramic powder, concrete, strength, artificial neural network modeling, supplementary cementitious material
Citation: Özkılıç YO, Bahrami A, Güzel Y, Soğancı AS, Karalar M, Althaqafi E, Çelik Aİ, Zeybek Ö and Jagadesh P (2025) Waste ceramic powder for sustainable concrete production as supplementary cementitious material. Front. Mater. 11:1450824. doi: 10.3389/fmats.2024.1450824
Received: 18 June 2024; Accepted: 20 September 2024;
Published: 12 February 2025.
Edited by:
Cut Rahmawati, Universitas Abulyatama, IndonesiaReviewed by:
Sagar Paruthi, DPG Institute of Technology and Management, IndiaCopyright © 2025 Özkılıç, Bahrami, Güzel, Soğancı, Karalar, Althaqafi, Çelik, Zeybek and Jagadesh. This is an open-access article distributed under the terms of the Creative Commons Attribution License (CC BY). The use, distribution or reproduction in other forums is permitted, provided the original author(s) and the copyright owner(s) are credited and that the original publication in this journal is cited, in accordance with accepted academic practice. No use, distribution or reproduction is permitted which does not comply with these terms.
*Correspondence: Yasin Onuralp Özkılıç, eW96a2lsaWNAZXJiYWthbi5lZHUudHI=; Alireza Bahrami, YWxpcmV6YS5iYWhyYW1pQGhpZy5zZQ==
Disclaimer: All claims expressed in this article are solely those of the authors and do not necessarily represent those of their affiliated organizations, or those of the publisher, the editors and the reviewers. Any product that may be evaluated in this article or claim that may be made by its manufacturer is not guaranteed or endorsed by the publisher.
Research integrity at Frontiers
Learn more about the work of our research integrity team to safeguard the quality of each article we publish.