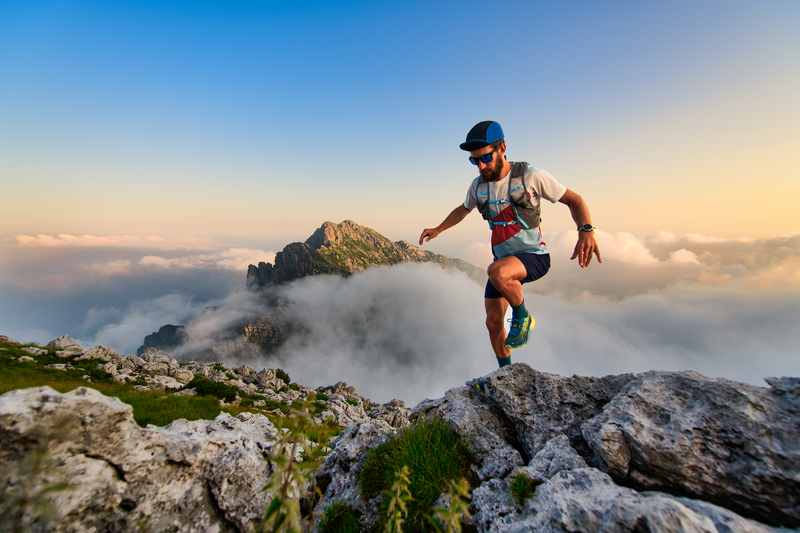
95% of researchers rate our articles as excellent or good
Learn more about the work of our research integrity team to safeguard the quality of each article we publish.
Find out more
REVIEW article
Front. Mater. , 03 June 2024
Sec. Polymeric and Composite Materials
Volume 11 - 2024 | https://doi.org/10.3389/fmats.2024.1405483
Global concern about plastic pollution is forcing new policies and modifications of human consumption as well as promoting new research lines aiming at the replacement of non-degradable plastics with other polymers more environmentally friendly. Addressing food waste and promoting circular economy strategies, among other approaches, are crucial in reducing environmental impacts and fostering sustainability in several sectors like the agri-food industry. The European Union’s Circular Economy Action Plan is a significant initiative in this direction. Biotechnological processes, especially the valorisation of agri-food waste to produce highly marketed biomolecules like poly (3-hydroxybutyrate-co-3-hydroxyvalerate) (PHBV) using microorganisms as cellular factories, offer promising avenues for achieving these goals. PHBV is a biodegradable polymer firstly characterised as an isolated biopolymer from bacterial biomass. This biopolymer shows interesting physicochemical properties making possible immense potential in various applications due to its biocompatibility and sustainability, thus revealing it as a good candidate to replace plastics produced by chemical synthesis from petroleum (which are highly recalcitrant and consequently pollutants). This review critically analyses the PHBV synthesis and end-of-life scenarios from their synthesis using chemical and biological pathways, through the forms of biotechnological operation and production, to the forms described until the moment of recycling.
The plastic era began in the mid-1800s when John Hyatt created the first synthetic polymer derived from cellulose nitrate as a substitute for ivory (Rasmussen, 2021). In the 1940s the massive production of plastics increased exponentially (Adjani Diankristanti et al., 2024; Liwarska-Bizukojc, 2021), reaching a global amount of 400.3 million tons (Mt) in 2022 (Plastics Europe, 2022). Among them, the use of petroleum-derived plastics has become a problem worldwide, due to the well-known impact on human health and the environment (González-Rojo and Díez-Antolínez, 2023; Pinho et al., 2022; Simó-Cabrera et al., 2021). In fact, these materials are widely distributed around the world, contaminating diverse ecosystems, also including the deepest marine ecosystems (de Mello et al., 2024) and one of the most critical problems are their long persistence in these environments (Amabile et al., 2024; Binelli et al., 2022; Pinho et al., 2022). The scientific community has focused on finding sustainable alternatives, including bioplastics, whose properties are similar to conventional plastic and whose production comes from renewable sources or biological matter (Atiwesh et al., 2021; Ciftcioglu-Gozuacik et al., 2023). Indeed, bioplastic production has been growing these last years from 1.792 Mt in 2021 to 2.217 Mt in 2022; in only 1 year, the production has increased by 123.72% globally. Additionally, global bioplastics production capacity is expected to increase significantly from around 2.18 million tons in 2023 to approximately 7.43 million tons in 2028 (European Bioplastics, 2022). However, it should be noted that not all bioplastics have biodegradable properties even though they all come from renewable sources, as in the case of some types of polyethylene (PE), polyethylene terephthalate (PET), polyamide (PA) and polytrimethylene terephthalate (PTT) (Atiwesh et al., 2021; Di Bartolo et al., 2021; Venâncio et al., 2022; Arif et al., 2023). In this context, the group of biopolymers known as polyhydroxyalkanoates (PHAs) stand out for its biodegradability, biocompatibility, sustainability, and thermoplastic properties, making them valuable in various industries (Simó-Cabrera et al., 2021; Zhang et al., 2021). PHAs, discovered by Lemoigne in 1925 inside Bacillus megaterium cells (Doudoroff and Stanier, 1959), are linear polyesters of (R)-hydroxyl fatty acid monomers (Tan et al., 2014b; Kumar et al., 2017). They accumulated in the cytoplasm of various microorganisms in the form of granules, serving as a source of energy and carbon, particularly in the presence of excess carbon and limited essential nutrients such as nitrogen, phosphorus, or oxygen (Cánovas et al., 2021; Koller et al., 2007; Kumar et al., 2017; Obruca et al., 2018; Rivera-Briso and Serrano-Aroca, 2018). It is documented that granules are composed of 97.5% PHA, 2% proteins (PHA synthases, PHA depolymerases, phasins, and regulatory proteins) and 0.5% lipids (Cai et al., 2012; Cai et al., 2015; Amabile et al., 2024). More than 150 different monomers have been described as building blocks in the structure of PHAs, which can be classified according to them and the length of the radical group (Tan et al., 2014b) as short-chain- length PHAs (scl-PHAs), medium-chain-length PHA (mcl-PHA) and long-chain- length PHA (lcl-PHA) (Obruca et al., 2018; Neoh et al., 2022). Among the various types, polyhydroxybutyrate (PHB) stands out as the most widely studied PHA due to its production by numerous microorganisms, including Gram-negative and Gram-positive bacteria. However, one of the main drawbacks associated with the use of PHB is the narrow working range, as the difference between its melting point and degradation temperature is 20°C (180°C and 200°C) (Priya et al., 2022). However, to improve its physicochemical properties for potential uses in different industrial and medical sectors, it is often necessary to incorporate secondary monomers to form copolymers. Although several types of PHB derivatives exist, one of the most promising for various applications is poly (3-hydroxybutyrate-co-3-hydroxyvalerate) (PHBV) (Rivera-Briso and Serrano-Aroca, 2018).
PHBV is formed by the incorporation of 3-hydroxyvalerate (3HV) monomers into the PHB structure (Figure 1). PHBV is distinguished from other PHAs by several important characteristics such as zero toxicity, total biodegradability, proven in soils, water and compost, biocompatibility with many cell types, resistance to UV radiation, and better mechanical characteristics, such as lower degree of crystallinity, higher flexibility, and strength (Rivera-Briso and Serrano-Aroca, 2018; Rodríguez-Cendal et al., 2023). These improvements directly depend on the amount of 3HV incorporated in its structure. It has even been observed that the higher the concentration of 3HV, the higher the degradation rate and the lower the melting temperature, making it easier to process (Rivera-Briso and Serrano-Aroca, 2018; Policastro et al., 2021; Rodríguez-Cendal et al., 2023).
Figure 1. Structure of poly (3-hydroxybutyrate-co-3-hydroxyvalerate) (adapted from Rivera-Briso and Serrano-Aroca, 2018).
Although PHBV was first commercialised in 1980 by Imperial Chemical Industries under the name “Biopol”, the cost of production is still high compared to the conventional production of plastic from petroleum for instance, making it very difficult to compete with the petrochemical industry (Obulisamy and Mehariya, 2021; Koller and Rittmann, 2022; Rodríguez-Cendal et al., 2023). The high cost associated with PHBV production is attributed to several factors related to the initial carbon source, fermentation costs, extraction and purification if it is necessary. These latter processes, account for approximately half of the total production cost (Zhang et al., 2024). Nevertheless, due to the growing concern about global pollution due to non-biodegradable plastics as well as growing interest in PHBV application in different biomedical areas and as a substitute for conventional plastic products, multiple research projects are being carried out to look for microorganisms able to produce PHBV efficiently and/or develop processes aiming at the reduction of the cost of their production (Hammami et al., 2022).
PHBV is produced by different Gram-negative and Gram-positive bacteria, however, the use of bacteria for the production has certain disadvantages. Gram-negative bacteria, such as Cupriavidus necator, which serves as a model for the study of PHA production, produce the well-known lipopolysaccharide, which is an endotoxin that copurifies with PHA extraction and can cause immunological reaction and limit its applications in the medical sector. Therefore, to remove toxins, the downstream process becomes more expensive (Tan et al., 2014b; Tebaldi et al., 2019; Kumar et al., 2020; Diankristanti et al., 2023). Gram-positive bacteria such as those belonging to the genera Nocardia or Rhodococcus are of limited interest due to their low production of the polymer compared to Gram-negative bacteria and their difficult purification (Guo et al., 2013; Tan et al., 2014b).
Therefore, scientists are currently focusing their attention on the use of extremophilic archaea, such as haloarchaea, to produce PHBV. The advantages of their use are numerous, such as easy lysis, no need for media sterilisation and the possibility of using a wide range of inexpensive raw materials for growth (Koller and Rittmann, 2022; Obruca et al., 2018).
Because of that, for an effective and competitive production, many efforts are being made such as the selection of efficient producer microorganisms, as well as the development of different strategies for the modification of PHBV to improve its properties. Despite all the technical difficulties that medium and large-scale PHBV production still has, the relevant benefits of the use of PHBV in biomedicine make the polymer of high interest to the global scientific community and companies aiming at the synthesis of bioplastics. Thus, its beneficial use has been reported in different fields of medicine, such as in tissue regeneration (bone, muscle, epithelial, among others) (Ren et al., 2023), in the controlled release of drugs using PHBV nanoparticles (tested in different treatments for various types of cancer, such as hepatocellular carcinoma, colon cancer, breast cancer, etc.) (Rodríguez-Cendal et al., 2023), in its use for sutures and in the manufacture of prostheses and pericardial patches, as well as its use for new vaccines against COVID-19. Other potential applications in different industries are mainly related to the packaging of food (Policastro et al., 2021; Koller and Rittmann, 2022; Urtuvia et al., 2022; Ren et al., 2023; Rodríguez-Cendal et al., 2023).
Considering the innovation and the current impact of the potential uses of PHBV, this review summarises insights into PHBV production by using different strategies (use of different carbon sources, microorganisms, and various industrial-scale production approaches), along with insights into its recyclability.
In the last decades, several chemocatalytic pathways have been investigated to synthesise different copolymers of PHAs. These pathways have attracted great attention since the thermal and mechanical properties of PHAs can be modulated by chemical approaches, manipulating to a greater extent the polymer stereomicrostructure. In this sense, several advantages can be reached, which include: i) precision in synthesis, considering both chain length control and comonomer sequence; ii) tunability of microstructure, which entails tacticities and R or S configurations; iii) ease scalability of the process, due to the fast reaction kinetics associated with ring-opening-polymerization (ROP) procedures. Nevertheless, the preparation of the cyclic monomers needed to conduct ROP mechanisms is not straightforward, and complex steps are required. Hence, the main constraint that it presents is caused by the need to develop a cost-effective synthesis of monomers to efficiently establish a “monomer-polymer-monomer” closed-loop lifecycle (Westlie et al., 2022). Considering their relevance, two different chemocatalytic pathways have been identified in this review as the most representative in chemical synthesis: i) direct route and ii) ring-opening polymerization (Gabirondo et al., 2020).
The direct route, also known as polycondensation, is considered the most industrially widespread relevant strategy to produce polyesters. In this case, the availability of the monomers does not imply an obstacle. Herein, two different mechanisms are recognised: step growth polymerization¸ which mainly consists of the polymerization between dicarboxylic acids and diols, and self-condensation polymerization, which is focused on hydroxy acids, since they have, at least, one nucleophile (alcohol group) and one electrophile (carboxylic acid group) in their structure. The conditions required for polycondensation of (R)-3-hydroxybutyric acid make it prone to produce side reactions, such as elimination/termination reaction, giving rise to crotonization which entails the formation of crotonate end groups (Kobayashi et al., 1993; Ono et al., 2023). Self-polycondensation presents an important advantage in comparison with conventional condensation (from dicarboxylic acids and diols) since it does not confer the need to strictly control the stoichiometry and what is more, it allows obtaining high Mw polymers.
ROP, which is considered a simple method to obtain polyesters, is of utmost interest since its paramount advantage is related to its ability to obtain polymers with not only narrow polydispersity, but also both high number and weight-average molecular values. Regarding the availability of target monomers, ROP of 4-membered β-butyrolactone (β-BL) is the most convenient and promising approach toward PHB synthesis. The ROP mechanism of β-BL results in a retention of stereochemistry by scission of the O-acyl bond and chiral conversion or racemization by scission of the O-alkyl bond. Nevertheless, the use of enantiopure monomers for producing isotactic PHB is not cost-efficient hampering its industrial scale (Tang and Chen, 2018; Tang et al., 2019). A study conducted by Bruckmoser and coworkers shows the stereocontrol of the catalytic system in situ generated consisting of Ys [N(SiHMe2)2]3 (THF)2(Y) and a salan-type pro-ligands, which allows the production of PHB with high productivity and isoselectivity (Figure 2) (Bruckmoser et al., 2023).
Figure 2. ROP of 4-membered butyrolactone (adapted from Dhaini et al., 2023).
Moreover, the ROP of the racemic BL has been studied using aluminoxane (AlR3/H2O) catalyst, generating a mixture of different tacticities (Zintl et al., 2008). Other catalysts, such as salan-ligand rare-earth metal amide complexes and lanthanum aminobisphenolate, have been reported as initiators for the ROP of rac-BL, which results in switching from iso-tactic to syndio-tactic polymerization (Zhuo et al., 2018; Dong and Robinson, 2020). The production of an isotactic PHB via ROP of 8-membered diolide monomers has also been reported including the description of how by varying starting ratios of chiral racemic and achiral meso diastereomers enable the direct polymerization into stereosequenced crystalline PHA with isotactic and syndiotactic stereodiblock microstructures which enhance ductility and toughness. Besides, it has also been described how the design of unsymmetrical disubstituted eight-membered diolides (rac-8DLR1-R2) and their stereoselective ROP with discrete chiral catalysts enable the synthesis of alternating isotactic PHA (Figure 3), specifically poly-(3-hydroxybutyrate-alt-3-hydroxyvalerate) (alt-P3HBV) and poly-(3-hydroxybutyrate-alt-hydroxyheptanoate) (alt-P3HBHp) (Tang et al., 2019).
Figure 3. Alternating isotactic PHAs using the same diolide, rac-8DL (adapted from Zhang et al., 2022).
More recently, the synthesis of enantiomeric (R)-alt-P3HBV and (S)-alt-P3HBV by ROP of rac-8DLMe-Et with an enhanced Tm has been described (Zhang et al., 2022). What is more, a recent report exhibits the potential of synthesizing unexplored triblock copolymers (tri-BCPs) of PHA. Since hard-soft-hard ABA tri-blocks combine the elasticity of elastomers and the thermal processability of plastics, the resulting all-PHA tri-block shows different thermal properties, polydispersity, and crystallinity. Based on the composition of the soft B block, which is associated with R substituents of the ring, these properties are tuned (Westlie et al., 2023).
Although the chemical synthesis in vitro of PHAs is currently possible and efficient, those biopolymers were first discovered and described as biomolecules produced by several microbial species. Specifically, it was first described from B. megaterium cells (Doudoroff and Stanier, 1959). Since that date, the number and diversity of microorganisms (belonging to the three domains of life) reported as PHAs-producers is significant. Most of them accumulate PHAs in their cytoplasm as granules at a high productivity rate. The cells produce and accumulate PHAs within the cells as a subcellular energy source and these biopolymers are usually produced when the microorganisms showing this metabolic capability are cultured with nutrient-limiting concentrations of nitrogen, phosphorus, sulphur, or oxygen and excess carbon sources (Cánovas et al., 2021; Simó-Cabrera et al., 2021). The most produced PHAs by microbes are polyhydroxybutyrate (PHB) although the most marketed is PHBV due to its physicochemical characteristics.
In the Bacteria domain, C. necator (Aramvash et al., 2016; Aramvash et al., 2018; Flores-Sánchez et al., 2017; Dalsasso et al., 2019; Novackova et al., 2019; Zhang et al., 2015) and B. megaterium are the better characterised bacterial species in terms of PHAs production (Blunt et al., 2023; Patil et al., 2024; Rivas-Castillo et al., 2024). Regarding yeast, it is worth mentioning that this is a group of organisms from which more literature has been reported related to PHAs production apart from bacteria (Kurian and Das, 2021). For example, Yarrowia lipolytica is a yeast able to accumulate until 25% (w/w) of mcl-PHA (Rigouin et al., 2019), while Wickerhamomyces anomalus cells could reach a production of 19.5 g/L. However, yeasts are not as efficient as bacteria in the yield of PHA production (Kurian and Das, 2021). In the case of algae, several cyanobacteria and green unicellular algae are highlighted as PHA producers. Spirulina plantesis can produce up to 10% (w/w) of PHA under the stress experienced by the presence of acetate and CO2 in the medium (Toh et al., 2008). In the case of Chrollella sp. PHAs production of around 27% (w/w) has also been reported. Finally, in the Archaea domain, haloarchaea are the most promising microorganisms showing metabolic capabilities for PHA. There are advantages to unnecessary strict sterile conditions because of the high salt concentrations of the media where these species are grown (thus avoiding the growth of other microbial strains) (Koller, 2019; Pacholak et al., 2021; Simó-Cabrera et al., 2021). Their metabolism is versatile thanks to molecular adaptations to extreme conditions in terms of environmental parameters or nutrient availability. Additionally, they produce other biomolecules with high biotechnological interest, like carotenoids (Flemming, 2016; Giani et al., 2019; Giani et al., 2022; Giani et al., 2021; Pfeifer et al., 2021) and exopolysaccharides (Flemming, 2016; Costa et al., 2018; Blackburn and Green, 2022). Besides, some haloarchaea can be used in the bioremediation processes to remove heavy metals and inorganic anions from brines and salty water (Oren, 2010; Nájera-Fernández et al., 2012; Martínez-Espinosa et al., 2015; Hou and Cui, 2018; Zuo et al., 2018; Pacholak et al., 2021; Pfeifer et al., 2021; Martínez et al., 2022; Priya et al., 2022; Moopantakath et al., 2023). Within haloarchaea, the most promising microorganism is Haloferax mediterranei (Pfeifer et al., 2021; Hagagy et al., 2022; Costa et al., 2023; Diankristanti et al., 2023), because it can synthesize PHBV without using any HV precursor (as required by other microorganisms) and can use different carbon sources to accomplish it (Tan et al., 2014b; Han et al., 2015; Parroquin-Gonzalez and Winterburn, 2023).
The metabolic pathways that allow the production of PHA are diverse if they are analyzed and compared in all groups of microorganisms, but essentially, all of them share the following reactions. The main building block required for their synthesis is acetyl-CoA. In principle, all the reactions and molecules involved in the production of acetyl-CoA can be considered the starting reactions required to produce PHAs. Thus, the Wood-Ljungdal pathway, Calvin–Benson–Bassham (CBB) cycle, Serine cycle, Ribulose monophosphate (RuMP) cycle, carbohydrates and fatty acids are involved in the PHA synthesis (Goswami et al., 2023; Banu et al., 2021; Salem et al., 2021). Once the microorganism has enough free acetyl-CoA within the cytoplasm, the enzyme 3-Ketothiolase (PhaA) bonds two units of acetyl-CoA, making acetoacetyl-CoA (AcAc-CoA)). Then, NADPH-linked acetoacetyl-CoA reductase (PhaB) reduces AcAc-CoA into 3-hydroxybutyryl-CoA (3HB-CoA). Finally, PHA synthase (PhaC) polymerises the molecules of 3HB-CoA into short-chain length PHA (Aghaali and Naghavi, 2023; Rodriguez-Perez et al., 2018; Banu et al., 2021; Han et al., 2010; Salem et al., 2021; Shahid et al., 2021; Zher Neoh et al., 2022). PhaC is also the enzyme needed to produce the medium-chain length, although the metabolic pathways promoting this reaction are the fatty acids β-oxidation and de novo fatty acids synthesis (Aghaali and Naghavi, 2023; Rodriguez-Perez et al., 2018; Banu et al., 2021; Salem et al., 2021; Shahid et al., 2021; Zhang et al., 2015; Zher Neoh et al., 2022).
In the last years, several studies focused on enhancing PHBV production while decreasing its economic costs (Tebaldi et al., 2019), since the high sale price of this copolymer entails a substantial limitation (Yu et al., 2006). It is necessary to address different strategies to reduce the competitiveness gap between PHBV and traditional plastics given the expenses derived from production costs regarding equipment, raw materials and substrates purchase, waste treatment or disposal, and operational conditions, among others (Policastro et al., 2021). Approximately estimated production costs of PHBV range from 1.50 to 10 $/KgPHA strongly depending on production factors, as well as location and general capital operating costs (Garcia et al., 2011; Hermann-Krauss et al., 2013; Bhattacharyya et al., 2015). The addition of 3HV precursors significantly increases the hydroxyvalerate fraction in the copolymer and the PHBV production by several species, but due to its high costs, wild-producing species can be considered. Metabolic engineering also involves an interesting approach when improving PHBV production, as well as the use of mixed cultures, extremophile species, the utilization of organic waste as a substrate or the modification of abiotic factors (Policastro et al., 2021).
As previously mentioned, running out of an essential nutrient, such as nitrogen or phosphorous, with an excess of carbon are the nutritionally unbalanced conditions that induce the synthesis of PHBV. The biological production of this polymer is also affected by several operational parameters, such as temperature, pH, dissolved oxygen concentration and process regime. When Gram-negative bacteria produce these polymers, they can contain high levels of endotoxins that need to be removed in the purification process (Rivera-Briso et al., 2018). On the other hand, PHBV production by Gram-positive bacteria entails a higher economic cost due to difficulties in the purifying steps (Guo et al., 2013).
Nowadays the main goal is to identify microorganisms able to produce PHBV avoiding the use of precursors, and showing high growth rates, metabolic versatility (to use different sources of nutrients including wastes of different industrial processes), and genetic stability. Some extremophilic microbial strains have been recently revealed as good candidates to be used as cellular factories to produce PHAs, because they fit all these requirements. In the case of halophilic bacteria and archaea, the high salt concentrations favouring their growth avoid the growth of other microorganisms in non-sterile conditions, thus significantly reducing process costs. It is possible to design a production process operating at low energy requirements, modifying the culture composition deriving the carbon flux towards PHBV accumulation, supplementing with nitrogen and phosphate sources, and using optimal temperatures (Koller et al., 2015). For example, the haloarchaeon H. mediterranei has been used for the conversion of different organic waste materials, as follows: whey (Koller et al., 2008; Pais et al., 2016); raw glycerol (Hermann-Krauss et al., 2013); cornstarch (Chen et al., 2006; Huang et al., 2006); vinasse and stillage (Bhattacharyya et al., 2012; Bhattacharyya et al., 2014); rice bran (Huang et al., 2006); crop waste (Alsafadi et al., 2020); silkworm excrement (Cai et al., 2022); or ricotta cheese exhausted whey (Raho et al., 2020). High PHBV amount can be produced even without substrate pre-treatment reducing the production costs (Hermann-Klaus et al., 2013; Bhattacharyya et al., 2014; Alsafadi and Al-Mashaqbeh, 2017). H. mediterranei can also produce high molecular weight PHBV from volatile fatty acids (VFA) (Ferre-Guel et al., 2018). Similarly, the purple non-sulphur bacteria (PNSB) Rhodospirillum rubrum can also synthesize PHBV when fed with sugars and wastes without the need for a precursor supply (Smith et al., 2008; Liu et al., 2019). Godoy and coworkers boosted this species of PHBV producer by performing an aerobic-anaerobic transition (Godoy et al., 2023). Other species such as B. cereus FA11 and Bacillus flexus can produce PHBV from glucose (Masood et al., 2012; Wagle et al., 2019). B. cereus can also produce PHBV using wheat starch wastewater (Sinaei et al., 2021). Pure cultures of obligate methanotrophs can convert the two primary components of natural gas (methane and ethane) into PHBV, mitigating methane greenhouse gas impact (Myung et al., 2024). The bacterium Pseudomonas oleovorans was found able to use Jatropha curcas seed oil as the only carbon source to synthesize PHBV, a renewable substrate that can be cheaply produced at large scale (Allen et al., 2010). Activated sludge also contains a versatile microbiome capable of converting waste into valuable products such as PHBV. Employing a hydrolysate derived from rice straw as substrate, Corynebacteriaceae and Bacillaceae were found to be the main microorganisms in this microbial population to produce PHBV thriving in repeated feast and famine phases (Morya et al., 2023). The marine bacterium Rhodovulum sulfidophilum DSM-1374 can be used as cellular factories enhancing PHBV production under a light-dark cycle using lactate as substrate and with the limitation of N-P nutrients (Carlozzi et al., 2022).
Apart from the natural metabolic capabilities, metabolic engineering is a strategy used to produce PHBV in recombinant strains or to increase its production. Since the supplementation of precursors is associated with high costs, this strategy can be considered to biosynthesize this copolymer. Escherichia coli-engineered strains can produce PHBV from unrelated carbon sources such as glucose and glycerol using enzymes involved in the 3HV biosynthetic pathway (Miscevic et al., 2019). The recombinant strain of Corynebacterium glutamicum in which the phaCAB gene cluster was inserted, produced high PHBV concentrations from glucose with high 3HV fraction (Ma et al., 2018). A recombinant Halomonas TD01 species was generated by overexpressing the threonine synthesis pathway and threonine dehydrogenase, producing PHBV using carbohydrates as the sole carbon source (Tan et al., 2014a). Choi and coworkers studied a threonine overproducing mutant of Alcaligenes sp. SH-69 able to increase the production of PHBV up to 6 times compared with the wild type, using glucose as substrate (Choi et al., 2023). Halomonas bluephagensis tricarboxylic acid (TCA) cycle was engineered via CRISPR/Cas9 producing PHBV with different 3HV fractions (0–25 mol%) using glucose as a carbon source (Chen et al., 2019). Yang and coworkers demonstrated that the mutant strain H. mediterranei Δ123 with a replication origin deletion produced a higher amount of PHBV, providing an advance in the production of bio-based chemicals by modulating chromosome replication (Yang et al., 2022). This biopolymer production was also optimised in H. mediterranei via CRISPRi-mediated redirection of the carbon flux. Specifically, plasmid-mediated CRISPRi downregulation of the citrate synthase genes (citZ and lgtA) improved the PHBV by 76.4% (Lin et al., 2021). The chromosomal expression system also showed advantages in PHBV production by Escherichia coli and the mutant Halomonas TD08 compared to the plasmid expression system (Yin et al., 2015).
As mentioned before, the addition of 3HV precursors is a strategy that implies an economical cost, but several species are only capable of producing PHBV when specific precursors are available. These compounds are also used to modify the polymer properties adjusting the 3HV fraction. Valeric acid and propionic acid have been the most widely studied precursors. Photobacterium sp. TLY01, a novel strain in this genus, could effectively utilize a series of sustainable substrates including plant oils, glycerol, and volatile fatty acids when propionate or valerate were added as the secondary carbon sources to produce PHBV (Tian et al., 2022). Bacillus megaterium could synthesize PHBV using cheese whey permeate, a processed by-product of the dairy industry, adding propionic acid and optimizing other parameters such as time or C/N ratio (Suhazsini et al., 2020). The addition of propionic acid and valeric acid was also studied in a thermophilic mixed methane-utilizing culture resulting in the production of PHBV with higher 3HV values (Luangthongkam et al., 2019). The addition of valerate with various combinations of methane was also tested in a methanotrophic consortium showing the same positive results (Myung et al., 2015). Caldimonas taiwanensis was also able to produce PHBV in a sugar-rich medium supplemented with different concentrations of valerate increasing the 3HV fraction up to 90% (Sheu et al., 2009). Choi and coworkers performed a comparison between the utilisation of propionic acid, valeric acid, and levulinic acid using the bacterium Alcaligens SH 69 with glucose as substrate, showing an increase of 3HV in all cases, with levulinic acid producing the highest value (Choi et al., 2023). The modulation of 3HV monomer is not only important for the quality of PHBV produced but also for enhancing the quality of the final product (Koller et al., 2008; Zinn et al., 2023). For this purpose, the addition of precursors can be combined with engineered strains, as well as other strategies, to obtain optimal results. phaCp and phaABp genes from Propylenella binnzhouense have been cloned in E. coli to produce PHBV, improving its synthesis activity and polymer performance at higher processing temperatures (Meng et al., 2022). Rhodoligotrophos defluvii was proved to be able to synthesize PHBV in the presence of propionate through the successful construction of a PHA synthesis pathway in recombinant E. coli (Miao et al., 2021).
PHA extraction is also a crucial aspect in terms of economic approach. The whole PHA production life cycle requires high energy consumption, especially during the downstream operations, which can represent half of the total cost (Del Oso et al., 2021). Isolation and purification of the polymers must be carried out by efficient methods in order to reduce not only costs, but also the environmental impact of the process. These methods can be mechanical, chemical, or biological (Zhang et al., 2024).
Mechanical methods minimize environmental pollution since they do not involve any chemicals (Jacquel et al., 2008). Among them, bead mill disruption requires less power supply when compared to other techniques and is easy to escalate but involves a large number of steps (Tamer et al., 1998); high pressure homogenization (HPH) is also easily scalable, less contaminant and does not require pre-treatment, but the energy consumption is high and PHA can be micronized; sonication presents low costs and is environmentally friendly (Divyashree et al., 2009), but must be combined with other methods as well as gamma irradiation, which can improve polymer properties (Zhang et al., 2024).
Chemical methods include solvent extraction, the most extended one due to its simplicity and rapidity, offers high purity levels and low levels of endotoxin content, but it is not suitable for large scale processing, presents high costs and most of the used solvents are harmful to the environment and human health (Kunasundari and Sudesh, 2011). Both halogenated and non-halogenated solvents offer a high recovery yield and purity, but the first one is toxic to the environment, while the latter has an elevated cost (Zhang et al., 2024). Aqueous two phase systems (ATPS) is a technique in which two immiscible phases (polymers and/or inorganic salts) coexist (Yang et al., 2008), presenting low toxicity, energy consumption and material cost, as well as good resolution and large operating capacity, but it has low reproducibility and the mechanism is not well known; surfactants are also widely used for PHA recovery as they allow a direct extraction of the polymer but the cost is elevated and the removal of SDS from the isolated polymer is challenging (Zhang et al., 2024); supercritical fluids (SCF) emerged as a technique for PHA recovery due to its advantages such as low toxicity, when compared to others, and low cost (Khosravi-Darani and Mozafari, 2009), but the process requires strict parameters, extraction of polar analytes and frequent cleaning up (Kunasundari and Sudesh, 2011); sodium hypochlorite is used as a method to extract PHA based on the solubilization of non-PHA cellular mass (NPCM) for which cell drying is not necessary, presenting strong oxidizing properties although the molecular weight of the extracted polymer is reduced (Yu and Chen, 2006). NPCM solubilization can also be carried out using acids, also resulting in a low molecular weight (Zhang et al., 2024).
Biological extraction methods are being studied focusing on the economic aspect of these technologies, as well as their environmental impact, in order to reach scalability goals of industrial biotechnology (Mannina et al., 2019). Enzymatic digestion is a method in which NPCM is solubilized with a high PHA recovery yield, purity and low toxicity, but it involves a complex procedure, and enzymes entail a high cost (Kapritchkoff et al., 2006); cell fragility provides an efficient polymer release obtaining a high yield and purity without harming the environment, but genetically engineered strains are required (Zhang et al., 2024); predatory bacteria can also be used to extract the biopolymers, as they can attack Gram-negative bacteria by using their cytoplasmic contents as nutrients. This method is economical and requires almost no chemical reagents, but PHA may be degraded (Im et al., 2018); the construction of genetically engineered strains with a PHA extracellular secretion system is another method that, despite the complexity of the process, needs no chemicals, does not harm the environment and offers a continuous production of the polymer (Zhang et al., 2024).
Despite all the environmental advantages of the PHBV copolymer, its wide production and utilization are limited by the high production and downstream process costs, as well as the low productivity rate. Therein lies the importance of implementing new efficient, low-cost PHBV production processes considering techno-economic factors. The evaluation of all the different mentioned strategies, along with their possible combinations and operational conditions are crucial to enhance the economic competitiveness of PHBV compared to petroleum-based plastics.
In the European Union (EU), between 118 and 138 million tonnes of biowaste are generated annually, of which over 58 million tonnes are food waste (131 kg/inhabitant) with associated costs estimated at €143 billion (Santiago et al., 2023). Within the food value chain, it is estimated that consumption generates 52% of food waste, followed by production (23%), handling and storage (11%), distribution and sale (9%) and processing (5%). Of the total food waste generated globally, the beverage industry accounts for 26%, followed by the dairy and ice cream industry (21.3%), fruit and vegetable production and preservation (14.8%), the manufacture of grain and starch products (12.9%), and meat production, processing, and conservation (8%) (Capanoglu, et al., 2022; Santiago et al., 2023).
Most of the wastes generated are seeds, leaves, roots, tubers, fruits, and pomace, among others. Unfortunately, despite retaining a high nutrient content in carbohydrates (30%–60%), lipids (15%–40%) and proteins (5%–20%), most food waste is considered a disposal problem and about 40% is currently being landfilled or incinerated. Intending to reduce the environmental impact of food production and reducing food waste, the EU has adopted The Circular Economy Action Plan (European Commission, 2020), which promotes the circularity of food waste/by-products by adopting strategies to close the loop in industrial production systems. In this sense, the biotechnological processes play a fundamental role in implementing circular economy strategies in key industries, such as the agri-food industry, through the valorisation of organic waste or by-products to obtain value-added products that can be later launched on the market.
Agri-food wastes are rich in lignocellulose, which can be hydrolysed to obtain fermentable sugars from the cellulose and hemicellulose fractions. Therefore, these fermentable sugars can replace the pure derived sugars as substrates in the fermentation process, such as the PHBV production, promoting the sustainability and circularity of this waste stream (González-Rojo and Díez-Antolínez, 2023). The carbon substrate in PHBV production contributes to about 40%–50% (Pérez et al., 2020) of the total cost of the process, increasing the price of PHAs by six times (Del Oso et al., 2021) compared to fossil-based plastics. The high cost of PHA production reduces the market applications to those with high-added value, such as biodegradable scaffolds for biomedical applications. Therefore, substituting commercial carbon sources with agri-food wastes would reduce the overall cost of PHBV production, which is the primary constraint to industrial scale-up, and the production will be affordable for many different applications, such as packaging. In this way, the process will be moved towards a biorefinery scenario using abundant and inexpensive materials, also considering the utilisation of waste-derived hydroxyvalerate precursors.
Life cycle assessment (LCA) is a systematic methodology to quantify the potential environmental impacts of a product system throughout its life cycle. LCA encompasses the complete life cycle of a product, process, or activity, comprising: the extraction and refinement of raw materials; manufacturing, transportation, and distribution; utilization, reutilization, and preservation; and recycling and disposal (Banerjee and Ray, 2022). They can be performed with different boundaries, which outcome in dissimilar calculations (Pawelzik et al., 2013). International LCA standards, like ISO 14040 and EB 16760, help guide the structure, conduct, limitations and assumptions for the general LCAs, although they are heterogeneous because of the different approaches and assumptions (Yates and Barlow, 2013; Spierling et al., 2018; Walker and Rothman, 2020).
The majority of LCA research has identified PHA downstream processing as the primary focus area. Studies often used either a cradle-to-gate or gate-to-gate methodology, overlooking the final stages of the bioplastic life cycle, including shaping, compounding, usage, and end-of-life (EoL) (Del Oso et al., 2023). Although PHA usage in food packaging typically imposes minimal burdens on life cycle impacts, given its limited energy consumption and environmental emissions (Nessi et al., 2021), disregarding the EoL phase fails to recognize the benefits associated with PHA’s biodegradability and overlooks the lasting environmental consequences of traditional plastic pollution in marine ecosystems (Roibás-Rozas et al., 2022).
In a 2010 study, Tabone et al. (2010) compared twelve polymers of fossil and biological origin, including PHA derived from corn stalks and corn grain. This study stands out for its use of a wide range of ecological indicators, such as acidification, carcinogens, eutrophication, eco-toxicity, GWP, ozone depletion, and fossil fuel depletion. While bio-based materials generally outperform fossil-based ones in GWP, they exhibit similar or even worse performance in other impact categories. PHA from corn grain, for instance, ranks highest in acidification and ozone depletion potential, comparable to or worse than polyethylene, polycarbonates, and polypropylene in eco-toxicity. However, many of these impacts are attributed to the agricultural production of raw materials. Similarly, a study by Kim and Dale (2008), analyzing the energy and GWP profiles of polyhydroxybutyrate (PHB) from corn grain, found PHB to have lower ecological impacts than fossil-based polymers. They suggest that implementing sustainable practices in corn cultivation, such as no-tillage and winter cover, could further reduce PHB’s production impact by up to 72% (Kim and Dale, 2008).
PHA’s recent LCA studies have been compared to harmonized product environmental footprint (PEF) (Cristóbal et al., 2016). The amount of kg CO2/kg PHA in these studies ranged from 2.3–6.9 which compared to 2.72 with sugarcane feedstock and to 4.26 for corn starch in the PEF. In the cases where the value of kg CO2/kg PHA is under 0.49 carbon storage in the polymer was counted as carbon sequestration from the atmospheric CO2. In the cases in which the polymer temporarily captures atmospheric CO2, forming its chains, kg CO2-eq/kg PHA values lower than 0.49 were obtained (Dietrich et al., 2017).
Many researchers have investigated the valorisation of agri-food waste for PHBV production using C. necator, which was the first microorganism used for industrial PHA production by Imperial Chemical Industries (Wang et al., 2019). C. necator is a well-known PHB producer from a wide variety of carbon sources. In addition, with short-chain volatile fatty acids obtained from the degradation of organic waste, such as acetic acid, valeric acid, propionic acid or butyric acid, C. necator is also able to produce PHBV. In this way, Hathi and coworkers studied the production of PHBV by C. necator valorising food waste hydrolysate and VFAs as PHBV inducers (Hathi et al., 2022). They showed that the fermentations combining food waste hydrolysate with valeric acid produced the highest yield of PHBV (34.12%) per total amount of biomass and the highest molar 3HV content (50%) (Hathi et al., 2022). The use of food waste derived VFAs as the sole carbon source for PHBV batch production was also analysed reporting a yield of 1.02 g/L PHAs (Vu et al., 2022). C. necator has also been tested for PHBV production through the valorisation of waste rapeseed oil adding selected precursors of 3-HV, such as propanol, propionate and valerate, allowing the production of PHA (g/L) of 11.7, 7.3 and 6.5, respectively, with a 3-HV (%) content of 9, 13 and 18 (Obruca et al., 2010).
C. taiwanensis has also been reported as a PHBV producer. It is a thermophilic microorganism capable of accumulating PHBV granules directly from starch by modulating the 3-HV content with the addition of valerate as a mixed carbon source (Sheu et al., 2009). The authors tested different starches from cassava, corn, potato, sweet potato, and wheat, combined with 0.05% valerate, and the highest PHBV accumulation was found in the corn and cassava starch experiments, which gave a yield of 2.145 and 1.876 g/L PHBV with a 3-HV content of 10% and 13%, respectively. Koller and coworkers studied the use of whey permeate from the dairy industry for PHB synthesis combined with levulinic acid and sodium valerate from renewable resources for 3-HV synthesis in Hydrogenophaga pseudoflava fermentation, reporting production of 4.4 g/L with molar 3-HV content of 55% using levulinic acid and valerate as precursors and 2.2 g/L PHBV with 45% of 3-HV only using levulinic acid (Koller et al., 2017).
However, the use of microorganisms which do not require 3-HV precursors is advisable to reduce production costs and control to keep their concentration in non-inhibiting thresholds. Within this group, it is worth mentioning some halophilic microorganisms, which can produce PHBV from waste and without the contribution of external precursors and have been widely studied in the literature. For example, starch and cassava waste were tested for PHBV production by Halogeometricum borinquense fermentation reaching a maximum yield of 4.6 g/L and 1.52 g/L with 13.11% and 19.65% 3-HV units, respectively (Salgaonkar et al., 2018). The potential of Halomonas alkaliantarctica to produce PHAs from bioproducts generated by cheese manufacturing has also been described finding that this microorganism could produce PHBV and PHB polymers utilising cheese whey and cheese whey mother liquor as the only carbon sources, obtaining the highest yield of 0.42 g/L using cheese whey mother liquors (Mozejko-Ciesielska et al., 2023).
In this context, H. mediterranei is the better-studied and most promising halophilic microorganism to produce PHBV. Table 1 summarises the most relevant works on the production of PHBV by H. mediterranei using wastes from different industries. By comparing these works, the main conclusion is that the batch cultivation mode is the most used. In this type of process, all media are added to the bioreactor at the beginning of the process and there are no inlet or outlet streams. The fermentation process is running until the desired cell stage or product concentration is reached (Policastro et al., 2021). The highest production described in the literature is 77.8 g PHBV/L with a fed-batch fermentation, thus indicating that this methodology for PHBV production usually produces more PHBV than batch fermentations (Huang et al., 2006). This comparison has been described by Alsafadi and coworkers: PHBV production was monitored using the same carbon waste (date waste extract) in a fed-batch fermentation against a batch fermentation (Alsafadi et al., 2020). In the first one, the production reached 4.5 g PHBV/L, while with the batch fermentation a final production of 3.20 ± 0.07 g PHBV/L was quantified (Alsafadi et al., 2020). Regardless, the batch fermentation type also has high production rates, reaching 19.7 g PHBV/L, 17.4 g PHBV/L (Bhattacharyya et al., 2012), 16.42 ± 0.02 g PHBV/L (Bhattacharyya et al., 2015) and 13.12 ± 0.05 g PHBV/L (Bhattacharyya et al., 2014). Consequently, a fed-batch fermentation increases the PHBV production potential of a carbon source with already potential, increasing its yields.
As can be seen in Table 1, none of the PHBVs obtained present an HV concentration higher than 20%, which provides properties similar to polypropylene (one of the most marketed polymers) (Lee et al., 2023). Even if the polymer is produced with an HV precursor, H. mediterranei cannot achieve this copolymer concentration based on the information reported in the bibliography. However, in some cases, the concentration of 20% HV is almost attained (Bhattacharyya et al., 2014; Bhattacharyya et al., 2015; Alsafadi et al., 2020; Priya et al., 2022; Wang et al., 2022).
Unquestionably, the disposal of biodegradable bioplastics must be considered, setting the basis for developing an optimal end-of-life pathway in terms of maximizing the circular economy. An ideal strategy to address the end-of-life key challenges of plastics is to develop next-generation polymers with closed-loop life cycles. Currently, there are no recycling strategies commercially implemented to give biodegradable bioplastics a second life, since only biodegradation is seen as a suitable end-on-life option for these bioplastics (Fredi and Dorigato, 2021; Renard et al., 2004). Nevertheless, the fact that bioplastics are biodegradable does not necessarily imply that they can degrade in all environments (domestic and industrial compost, soil, marine and freshwater). Moreover, existing standards (Silva et al., 2023) that regulate certifications of biodegradation in open environments exhibit an obvious weakness related to their poor reproducibility since several factors (such as biodiversity, soil, pH, water content and temperature) can affect the reproducibility of the results, causing the alteration of biodegradation. Most commercial biodegradable bioplastics comprise other substances, such as additives, adhesives, and coatings, which makes biodegradation challenging since initially its definition only considers the organic components of the plastics. Therefore, all the above-mentioned brings to light the need to develop real recycling routes, including for biodegradable bioplastics, to close the loop and thus fostering the main principles of Plastics Strategy regulation (Plastics Strategy, 2022).
Regarding bioplastics, selective collection has not yet been implemented in Europe, so it comes as no surprise that, at the end-of-life of biobased biodegradable plastics, it usually ends up landfilled together with organic residues, leading to the loss of high-quality materials. Hence, to efficiently involve these materials in the Circular Economy, it is crucial to develop feasible recycling routes. Up to now, very few papers have been reported to evaluate the performance of different recycling pathways of PHAs, but the main strategies involved can be summarised as follows: i) primary recycling, considered as mechanical recycling to obtain similar properties compared to initial products; ii) secondary recycling, or mechanical recycling thereby products of a lower quality are obtained; iii) tertiary recycling, chemical recycling or raw material recovery, that commonly requires depolymerisation to the constituent monomers; iv) quaternary recycling or energy recovery (Hahladakis et al., 2018).
Mechanical recycling is the most extended recycling pathway, considering its use for post-consumer and post-industrial plastics. It consists mainly of several cycles of extrusion or injection moulding, where the main aim is to preserve the original value of mechanical properties without modifying chemical structure. Indeed, it is the most developed alternative for plastics recycling, independently of its origin. Typical mechanical recycling process involves several steps such as cleaning, sorting, melting, shredding and finally, the re-extrusion process into recycled plastic pellets. If the final output is sensitive to be used in food-contact grade applications, an additional step of decontamination is required (Das et al., 2021). To be treated by mechanical recycling, the following conditions must be accomplished: i) a low degree of degradation; ii) efficient and suitable previous separation of PHAs from other types of plastics (such as fossil-based ones), for which selective collection is essential; iii) free from impurities, materials or particles that may interfere with the process or the final characteristics of the quality product and collection of sufficient quantities to achieve industrial, and iv) economic viability of the process.
Due to the high production costs and the poor mechanical properties, PHB is usually blended with other polymers to enhance their properties, which explains the few reported publications associated with pure PHAs reprocessing. In 2017, the recyclability of PHB was evaluated through multiple processing cycles, and after each cycle, mechanical and thermal properties were assessed. These properties were drastically decreased after two processing cycles, including the increase in the degree of crystallinity owing to the chemical crystallisation by chain scission (Rivas et al., 2017). Some authors (El-Taweel et al., 2004) noticed the interdependence between crystallization degree of PHB and tensile strength values so it is no wonder that the more cycles the worse mechanical properties. Four commercial grades of PHAs from different manufacturers were investigated via injection moulding (Corre et al., 2012) where the results showed a decrease in the molecular weight and dispersity value for all the PHAs studied. As a consequence, the degradation mechanism that could be expected is driving by a random process such as free radical mechanism. Several studies (Moraczewski et al., 2015) (Main et al., 2023) support the fact that the melt flow index (MFI) of virgin PHB increases with each cycle of reprocessing, resulting in a molecular weight decrease. Regarding mechanical properties, it was noticed that after five cycles of extrusion, virgin PHB becomes progressively brittle with a reduction of 50% in impact strength. Even if the results were not promising, other studies pointed out that mechanically recycled and degraded PHB could be used as a plasticizer for PLA (Yang et al., 2015). On the other hand, Zaverl evaluated the mechanical recycling of PHBV, which results in tensile and flexural strength decreased after five cycles (Zaverl et al., 2012). Moreover, the recyclability of blends of PHBV and PLA has also been investigated and, in comparison with the pure PHBV, the mixture shows higher mechanical properties (tensile strength, elongation at break and impact strength) stability after 6 cycles of processing (Zembouai et al., 2014).
Nevertheless, mechanical recycling presents the following limitations: i) those plastic materials that are excessively degraded, are not sensitive to be mechanically recycled, because of their poor mechanical and thermal properties; ii) plastic materials mixed or contaminated with other substances are not mechanically recyclable and iii) plastic colour, that hampers recycling since over pigmented plastics materials have a lower value than those which are not pigmented as well as the difficulty to treat pigmented materials which need to be sorted using optical methods.
The implementation of enzymes for the controlled degradation of plastics is emerging strongly in the research for new environmentally friendly technologies (Roohi and Kuddus, 2018). The natural biodegradation procedure for bioplastics is as follows: i) depolymerisation, cleavage of the polymer chain to small pieces occurs for better assimilation by microorganisms when polymer chains reach sufficiently low molecular weight (Webb et al., 2000); ii) microorganisms assimilate these small pieces to subsequently transform them in biomass and CO2. Microbial biodegradation can occur via aerobic or anaerobic; aerobic degradation, in the absence of oxygen, takes place to produce CO2 and H2O while in anaerobic degradation, the cleavage happens to obtain CH4, CO2 and H2O (Eubeler et al., 2010). The enzymatic degradation via hydrolysis is a two-step process, where firstly enzyme is tied up to the polymer substrate by catalysing the hydrolytic cleavage so the adherence, as a limiting step, comes before the colonization of the exposed surface (Amobonye et al., 2021; Shan et al., 2008).
PHA degradation has been investigated with different biopolymer blends and different enzymes. Even if numerous investigations have demonstrated its degradation through catalysed basic media, their degradation products have not been yet uniformly reported (Estelle et al., 2004). For instance, the viability of PHB depolymerization by PHA enzyme depolymerase from different sources at 37°C was investigated, obtaining dimers and monomers of 3-hydroxybutyric acid. Depending on the source of PHA depolymerase, such as P. picketti, greater yields are achieved for 3HB monomers (Mukrai et al., 1993). Furthermore, the degradation of P (3HB-co-4HB) to obtain its pertinent low molecular weight oligomers (5,000–10,000 Da) is also demonstrated through sustainable controlled enzymatic depolymerization using commercial triglyceride lipases, considering that the driving factor of the degradation is the enzymatic activity (Rodríguez-Contreras et al., 2012). Various microorganisms have found to be sensitive to produce depolymerase enzymes to degrade PHB and PHBV, among which are include: Pseudomonas lemoignei (Jendrossek et al., 1996), Streptomyces venezuelae (Santos et al., 2013), B. megaterium (Sanchez et al., 2000), Penicillium oxalicum strain (Li et al., 2012).
Normally, enzymatic recycling provides a wide range of degradation products with different molecular weights, which makes its subsequent repolymerization difficult since it requires a separation and purification process that would not make the recycling process viable.
It is urgent to develop a recycling closed loop to boost the circular economy, which can overcome current constraints in mechanical recycling. Chemical recycling tends to depolymerize into intermediate products that can be used as building blocks to synthesize the same material or to transform them into new high (upcycling)/low (downcycling) value products (Dhaini et al., 2023).
Most widespread chemical recycling methods consist of i) solvolysis and ii) the application of heat approach. The expression solvolysis includes total and partial depolymerization, which can be achieved by a wide range of solvents to depolymerize up to the pertinent monomers or other degradation products (such as oligomers). Solvolysis can be carried out using different approaches: hydrolysis (water-based), alcoholysis (alcohol-based), glycolysis (glycol-based) and lastly, aminolysis (amine-based). Most papers disclosed in this review are focused on hydrolysis, both acid and basic-based (Figure 4).
Figure 4. Hydrolysis of PHA in different conditions (adapted from Bruckmoser et al., 2023).
Concerning basic-based PHB hydrolysis, it is demonstrated that depolymerization rates increased up to 70% when NaOH concentration reached to 4.0 M, leading to crotonate acid (25%) and 3-hydroxybutyric acid (45%) as major degradation products. Nonetheless, concerning acid-based hydrolysis, the exposure of PHB to acid solutions (up to 90wt% H2SO4) mainly produces crotonate acid (90%) and, as the minor degradation product, 3-hydroxybutyric acid (2%) (Yu and Marchessault, 2000). Furthermore, recent work confirms the direct dependence of degradation kinetics with temperature as the higher the temperature, the greater the extent of weight loss (Yu et al., 2005). The main advantage that catalytic processes present in comparison with other recycled methods is the notable selectivity towards a specific product (Westhues et al., 2018). Initially, transformations of chemical recycling explored and boosted by catalysis can be classified as follows: (1) ester group hydrolysis and (2) ester group reductive rupture over metallic catalysts. Homogeneous catalysis has been addressed using acid catalysts as well as metallic complexes. It has been reported the depolymerization via a one-pot catalytic hydrogenolysis process of PHB, using Pd/C as catalyst at mild conditions where solvents are not needed (solid-solid reaction), leading to the formation of butyric acid, butanol, butyrate, and gaseous products, whereas a Cu/Zn/Al catalyst showed more selectivity to butyric acid (70%) (Zhang et al., 2021). More recently, the production of 3-hydroxybutyric and crotonate acid has been reported as degradation products when PHB is subject to hydrolysis in acids, bases and carboxylic acid salts conditions and followed by the dehydration and decarboxylation to propylene and CO2 (Li and Strathmann, 2019). Moreover, Melchiors and coworkers described how depolymerised PHB into its corresponding cyclic trimer [(R,R,R)-4,8,12-trimethyl-1,5,9-trioxacyclododeca-2,6,10-trione, TBL] at 80% yield, in solution using p-toluenesulfonic acid (PTSA) acting as catalyst, and thus the polymerization of TBL through dibutyltin dimethoxide to produce high-quality PHB (Melchiors et al., 1996). Various catalysts (Ru or Re) have been screened for the heterogeneous degradation of PHB, where it was demonstrated that Ru deposited on ceria (CeO2) exhibits promising yield (up to 79%) to achieve 3-HBA monomer (Lehnertz et al., 2022). Recently, the efficacy of ZnCl2 and polyethylene glycol (PEG) has been demonstrated, as a mixture to catalyse polyesters and polycarbonates depolymerization reactions towards their corresponding rings formation, under vacuum conditions and high ceiling temperature (Tc) (Gallin et al., 2023). What is more, the catalytic system can selectively depolymerize polyesters in the presence of other plastics, thus it shows the effectiveness of different plastic mixture separations.
When it comes to the application of heat, two different techniques can be well defined: i) thermochemical gasification, which mainly produces H2, CO, H2O, CH4 y CO2, which are accompanied by high-boiling polycyclic aromatic hydrocarbons, and ii) pyrolysis, which describes the thermal decomposition of solid material in absence of oxygen which gives rise to CO, H2, H2O, CO2 and simple hydrocarbons.
Ariffinet proposed, from a biorefinery point of view, a thermal catalyst degradation, where CaO and Mg(OH)2 were used as catalysts to selectively depolymerized towards crotonic and 2-pentenoic acid at lower degradation temperatures (Ariffin et al., 2010). In 1996, thermodynamics was investigated to satisfy the viability of different degradation products obtained (Melchiors et al., 1996). Two different pathways were examined: i) solution-based and ii) heat-applied. Heat depolymerization reached higher yields to obtain crotonic acid and linear oligomers from 3-hydroxybutyric acid, aligned with the expected results of esters pyrolysis reactions, independently of the catalyst used.
Interestingly, through solution-based depolymerization, cyclic oligomers are obtained mainly by back-biting mechanism-based reactions. Several parameters, such as catalyst/substrate rates, solvent nature, reaction time and temperature range were proven for both heat and solvent-based depolymerization. In any case, it required aggressive conditions as well as high reaction times and non-secure catalysts (such as p-TsOH or CF3SO3Me) leading to unsustainable processes which are against the circular economy principles.
The current low recycling rates for plastics have produced an increased interest in developing different recycling strategies. Regarding plastics recycling, independently of its origin, several recycling technologies have been implemented during the last years to deal with the pollution concern. The most developed technologies have been mechanical, chemical and enzymatic techniques, intending to substitute current plastics end-of-life options, which are focused on landfill and energy recovery. In terms of bio-based biodegradable plastics, in particular, for the PHA family, recycling technologies are still at a very early stage, just like it can be seen on the previous sections.
The following table (Table 2) shows a narrow comparison between the different techniques developed for PHAs recycling so far. It must be considered that the technology maturity (Technology Readiness Level, TRL) is poor, which makes the comparison sparse on details.
Table 2. Comparison between the different techniques developed for PHAs recycling reported nowadays in the literature.
Various studies have demonstrated the feasibility of using different waste streams and microorganisms like C. necator, C. taiwanensis, H. pseudoflava, H. borinquense, H. alkaliantarctica, and H. mediterranei for PHBV production from diverse carbon sources ranging from food waste to industrial byproducts. Of these microorganisms, the ones which stand out the most are C. necator because of their high yields and H. mediterranei because of their high 3-HV production. What makes H. mediterranei the most interesting microorganism for PHBV production is its capability to grow in highly salty mediums, where the contaminations are more unlikely to happen, reducing the restrictions for sterile conditions. Also, H. mediterranei metabolism is so versatile, making them suitable to produce PHBV using wastes of a wide range of chemical compositions.
Concerning production methods, fed-batch fermentation processes have enhanced microorganisms’ PHBV production potential, emphasizing the importance of process optimization. While challenges remain in achieving higher 3-HV copolymer concentrations comparable to commercial standards, ongoing research and optimization efforts continue to show promising results, making PHBV a viable and sustainable alternative in various applications, such as packaging and biomedical materials.
Regarding PHAs end-of-life, different applications and end-of-life scenarios must be considered to settle on the proper pathway to follow. As PHAs are still at an early stage of investigation and development, it is not surprising that different end-of-life options are not sufficiently described. Despite that, different routes including mechanical, enzymatic, and chemical recycling have been reported. Indeed, chemical recycling exhibits a promising environmental solution due to its ability to recover the constituent monomers and put them back into the PHAs life cycle. Refinement of LCA methodologies and standardization efforts are crucial for accurate environmental assessment. Continued research is essential to fully understand PHA’s environmental impact throughout its lifecycle. Therefore, to have an accurate LCA, it is essential to consider all the processes around the PHA, from the feedstock generation to the PHA EoL.
Overall, this is an innovative field for research and industrial applications due to global concerns about plastic pollution and the advantages of using PHBV in specific fields like biomedicine due to their physicochemical properties. The advancements described here underscore the potential of biotechnological approaches in transforming waste streams into valuable resources, contributing to a more sustainable and circular economy in the agri-food sector.
SC: Investigation, Writing–original draft, Writing–review and editing. TM: Investigation, Writing–original draft, Writing–review and editing. MN: Investigation, Writing–original draft. FM: Conceptualization, Project administration, Writing–review and editing. AS: Investigation, Writing–original draft. LC: Investigation, Writing–original draft. RM-E: Conceptualization, Investigation, Project administration, Writing–original draft, Writing–review and editing.
The author(s) declare that financial support was received for the research, authorship, and/or publication of this article. This study has received funding from the European Union’s Horizon 2020 research and innovation programme (Agro2circular; Grant agreement ID: 101036838; upPE-T: Upcycling of PE and PET wastes to generate biodegradable bioplastic for food and drink packaging. Grant agreement ID: 953214), Generalitat Valencia (PROMETEO/2021/055), and Universidad de Alicante (VIGROB-309).
Authors SC and MN were employed by company Cetec Biotechnology S. L.
The remaining authors declare that the research was conducted in the absence of any commercial or financial relationships that could be construed as a potential conflict of interest.
All claims expressed in this article are solely those of the authors and do not necessarily represent those of their affiliated organizations, or those of the publisher, the editors and the reviewers. Any product that may be evaluated in this article, or claim that may be made by its manufacturer, is not guaranteed or endorsed by the publisher.
Adjani Diankristanti, P., Lin, Y.-C., Yi, Y.-C., and Ng, I.-S. (2024). Polyhydroxyalkanoates bioproduction from bench to industry: thirty years of development towards sustainability. Bioresour. Technol. 393, 130149. doi:10.1016/j.biortech.2023.130149
Aghaali, Z., and Naghavi, M. R. (2023). Biotechnological approaches for enhancing polyhydroxyalkanoates (PHAs) production: current and future perspectives. Curr. Microbiol. 80, 345. doi:10.1007/s00284-023-03452-4
Allen, A. D., Anderson, W. A., Ayorinde, F. O., and Eribo, B. E. (2010). Biosynthesis and characterization of copolymer poly(3HB-co-3HV) from saponified Jatropha curcas oil by Pseudomonas oleovorans. J. Ind. Microbiol. Biotechnol. 37, 849–856. doi:10.1007/s10295-010-0732-7
Alsafadi, D., and Al-Mashaqbeh, O. (2017). A one-stage cultivation process for the production of poly-3-(hydroxybutyrate-co-hydroxyvalerate) from olive mill wastewater by Haloferax mediterranei. New Biotechnol. 34, 47–53. doi:10.1016/j.nbt.2016.05.003
Alsafadi, D., Ibrahim, M. I., Alamry, K. A., Hussein, M. A., and Mansour, A. (2020). Utilizing the crop waste of date palm fruit to biosynthesize polyhydroxyalkanoate bioplastics with favorable properties. Sci. Total Environ. 737, 139716. doi:10.1016/j.scitotenv.2020.139716
Amabile, C., Abate, T., Muñoz, R., Chianese, S., and Musmarra, D. (2024). Production of poly(3-hydroxybutyrate) and poly(3-hydroxybutyrate-co-3-hydroxyvalerate) from methane and volatile fatty acids: properties, metabolic routes and current trend. Sci. Total Environ. 927, 172138. doi:10.1016/j.scitotenv.2024.172138
Amobonye, A., Bhagwat, P., Singh, S., and Pillai, S. (2021). Plastic biodegradation: frontline microbes and their enzymes. Sci. Total Environ. 759, 143536. doi:10.1016/j.scitotenv.2020.143536
Aramvash, A., Hajizadeh-Turchi, S., Moazzeni-zavareh, F., Gholami-Banadkuki, N., Malek-sabet, N., and Akbari-Shahabi, Z. (2016). Effective enhancement of hydroxyvalerate content of PHBV in Cupriavidus necator and its characterization. Int. J. Biol. Macromol. 87, 397–404. doi:10.1016/j.ijbiomac.2016.03.002
Aramvash, A., Moazzeni Zavareh, F., and Gholami Banadkuki, N. (2018). Comparison of different solvents for extraction of polyhydroxybutyrate from Cupriavidus necator. Eng. Life Sci. 18, 20–28. doi:10.1002/elsc.201700102
Arif, A., Azeem, F., Rasul, I., Siddique, M. H., Zubair, M., Muneer, F., et al. (2023). Bioplastics from waste biomass of marine and Poultry Industries. J. Biosci. 48, 11. doi:10.1007/s12038-023-00332-8
Ariffin, H., Nishida, H., Hassan, M. A., and Shirai, Y. (2010). Chemical recycling of polyhydroxyalkanoates as a method towards sustainable development. Biotechnol. J. 5, 484–492. doi:10.1002/biot.200900293
Atiwesh, G., Mikhael, A., Parrish, C. C., Banoub, J., and Le, T. A. T. (2021). Environmental impact of bioplastic use: a review. Heliyon 7 (9), e07918. doi:10.1016/j.heliyon.2021.e07918
Banerjee, R., and Ray, S. S. (2022). Sustainability and life cycle assessment of thermoplastic polymers for packaging: a review on fundamental principles and applications. Macromol. Mater. Eng. 307 (6), 2100794. doi:10.1002/mame.202100794
Banu, J. R., Ginni, G., Kavitha, S., Kannah, R. Y., Kumar, V., Kumar, S. A., et al. (2021). Polyhydroxyalkanoates synthesis using acidogenic fermentative effluents. Int. J. Biol. Macromol. 193, 2079–2092. doi:10.1016/j.ijbiomac.2021.11.040
Bhattacharyya, A., Jana, K., Haldar, S., Bhowmic, A., Mukhopadhyay, U. K., De, S., et al. (2015). Integration of poly-3-(hydroxybutyrate-co-hydroxyvalerate) production by Haloferax mediterranei through utilization of stillage from rice-based ethanol manufacture in India and its techno-economic analysis. World J. Microbiol. Biotechnol. 31, 717–727. doi:10.1007/s11274-015-1823-4
Bhattacharyya, A., Pramanik, A., Maji, S. K., Haldar, S., Mukhopadhyay, U. K., and Mukherjee, J. (2012). Utilization of vinasse for production of poly-3-(hydroxybutyrate-co-hydroxyvalerate) by Haloferax mediterranei. Amb. Express 2 (1), 34. doi:10.1186/2191-0855-2-34
Bhattacharyya, A., Saha, J., Haldar, S., Bhowmic, A., Mukhopadhyay, U. K., and Mukherjee, J. (2014). Production of poly-3- (hydroxybutyrate-co-hydroxyvalerate) by Haloferax mediterranei using rice-based ethanol stillage with simultaneous recovery and re-use of medium salts. Extremophiles 18, 463–470. doi:10.1007/s00792-013-0622-9
Binelli, A., Della Torre, C., Nigro, L., Riccardi, N., and Magni, S. (2022). A realistic approach for the assessment of plastic contamination and its ecotoxicological consequences: a case study in the metropolitan city of Milan (N. Italy). Sci. Total Environ. 806, 150574. doi:10.1016/j.scitotenv.2021.150574
Blackburn, K., and Green, D. (2022). The potential effects of microplastics on human health: what is known and what is unknown. Ambio 51, 518–530. doi:10.1007/s13280-021-01589-9
Blunt, W., Shah, P., Vasquez, V., Ye, M., Doyle, C., Liu, Y., et al. (2023). Biosynthesis and properties of polyhydroxyalkanoates synthesized from mixed C5 and C6 sugars obtained from hardwood hydrolysis. N. Biotechnol. 77, 40–49. doi:10.1016/j.nbt.2023.06.005
Bruckmoser, J., Pongratz, S., Stieglitz, L., and Rieger, B. (2023). Highly isoselective ring-opening polymerization of rac-β-Butyrolactone: access to synthetic poly(3-hydroxybutyrate) with polyolefin-like material properties. J. Am. Chem. Soc. 145, 11494–11498. doi:10.1021/jacs.3c02348
Cai, S., Cai, L., Liu, H., Liu, X., Han, J., Zhou, J., et al. (2012). Identification of the haloarchaeal phasin (PHAP) that functions in polyhydroxyalkanoate accumulation and granule formation in Haloferax mediterranei. Appl. Environ. Microbiol. 78 (6), 1946–1952. doi:10.1128/aem.07114-11
Cai, S., Cai, L., Zhao, D., Liu, G., Han, J., Zhou, J., et al. (2015). A novel DNA-binding protein, Phar, plays a central role in the regulation of polyhydroxyalkanoate accumulation and granule formation in the Haloarchaeon Haloferax mediterranei. Appl. Environ. Microbiol. 81 (1), 373–385. doi:10.1128/aem.02878-14
Cai, S., Wu, Y., Liu, R., Jia, H., Qiu, Y., Jiang, M., et al. (2022). Study on the production of high 3 HV content PHBV via an open fermentation with waste silkworm excrement as the carbon source by the haloarchaeon Haloferax mediterranei. Front. Microbiol. 13, 981605. doi:10.3389/fmicb.2022.981605
Cánovas, V., Garcia-Chumillas, S., Monzó, F., Simó-Cabrera, L., Fernándezayuso, C., Pire, C., et al. (2021). Analysis of polyhydroxyalkanoates granules in Haloferax mediterranei by double-fluorescence staining with nile red and SYBR green by confocal fluorescence microscopy. Polym. (Basel) 13, 1582. doi:10.3390/polym13101582
Capanoglu, E., Nemli, E., and Tomas-Barberan, F. (2022). Novel Approaches in the valorization of agricultural wastes and their applications. J. Agric. Food Chem. 70, 6787–6804. doi:10.1021/acs.jafc.1c07104
Carlozzi, P., Touloupakis, E., Filippi, S., Cinelli, P., Mezzetta, A., and Seggiani, M. (2022). Purple non-sulfur bacteria as cell factories to produce a copolymer as PHBV under light/dark cycle in a 4-L photobioreactor. J. Biotechnol. 356, 51–59. doi:10.1016/j.jbiotec.2022.07.008
Chen, C. W., Don, T. M., and Yen, H. F. (2006). Enzymatic extruded starch as a carbon source for the production of poly(3-hydroxybutyrate- co-3-hydroxyvalerate) by Haloferax mediterranei. Process Biochem. 41, 2289–2296. doi:10.1016/j.procbio.2006.05.026
Chen, Y., Chen, X. Y., Du, H. T., Zhang, X., Ma, Y. M., Chen, J. C., et al. (2019). Chromosome engineering of the TCA cycle in Halomonas bluephagenesis for production of copolymers of 3-hydroxybutyrate and 3-hydroxyvalerate (PHBV). Metab. Eng. 54, 69–82. doi:10.1016/j.ymben.2019.03.006
Ciftcioglu-Gozuacik, B., Ulutug, F. C., Denizli, A., Dizge, N., Karagunduz, A., and Keskinler, B. (2023). Simultaneous production of Poly (3-hydroxybutyrate-co-3-hydroxyvalerate) from recovered volatile fatty acid with treatment of leachate by pilot-scale mechanical vapor recompression. Bioresour. Technol. 388, 129743. doi:10.1016/j.biortech.2023.129743
Corre, Y.-M., Bruzaud, S., Audic, J.-L., and y Grohens, Y. (2012). Morphology and functional properties of commercial polyhydroxyalkanoates: a comprehensive and comparative study. Polym. Test. 31 (2), 226–235. doi:10.1016/j.polymertesting.2011.11.002
Costa, A., Encarnação, T., Tavares, R., Todo Bom, T., and Mateus, A. (2023). Bioplastics: innovation for green transition. Polymers 15 (3), 517. doi:10.3390/polym15030517
Costa, O. Y., Raaijmakers, J. M., and Kuramae, E. E. (2018). Microbial extracellular polymeric substances: ecological function and impact on soil aggregation. Front. Microbiol. 9, 1636. doi:10.3389/fmicb.2018.01636
Cristóbal, J., Matos, C. T., Aurambout, J. P., Manfredi, S., and Kavalov, B. (2016). Environmental sustainability assessment of bioeconomy value chains. Biomass Bioenergy 89, 159–171. doi:10.1016/j.biombioe.2016.02.002
Dalsasso, R. R., Pavan, F. A., Bordignon, S. E., de Aragão, G. M. F., and Poletto, P. (2019). Polyhydroxybutyrate (PHB) production by Cupriavidus necator from sugarcane vinasse and molasses as mixed substrate. Process Biochem. 85, 12–18. doi:10.1016/j.procbio.2019.07.007
Das, S. K., Eshkalak, S. K., Chinnappan, A., Ghosh, R., Jayathilaka, W. A. D. M., Baskar, C. y., et al. (2021). Plastic recycling of polyethylene terephthalate (PET) and polyhydroxybutyrate (PHB)—a comprehensive review. Mater. Circ. Econ. 3, 9. doi:10.1007/s42824-021-00025-3
Del Oso, M. S., Mauricio-Iglesias, M., and Hospido, A. (2021). Evaluation and optimization of the environmental performance of PHA downstream processing. Chem. Eng. J. 412, 127687. doi:10.1016/j.cej.2020.127687
Del Oso, M. S., Nair, R., Mauricio-Iglesias, M., and Hospido, A. (2023). Comparative life cycle analysis of PHA-based consumer items for daily use. Resour. Conservation Recycl. 199, 107242. doi:10.1016/j.resconrec.2023.107242
de Mello, A. F., Vandenberghe, L. P., Machado, C. M., Brehmer, M. S., de Oliveira, P. Z., Binod, P., et al. (2024). Polyhydroxyalkanoates production in biorefineries: a review on current status, challenges and opportunities. Bioresour. Technol. 393, 130078. doi:10.1016/j.biortech.2023.130078
Dhaini, A., Hardouin-Duparc, V., Alaaeddine, A., Carpentier, J.-F., and y Guillaume, S. M. (2023). Recent advances in polyhydroxyalkanoates degradation and chemical recycling. Prog. Polym. Sci. 149, 101781. doi:10.1016/j.progpolymsci.2023.101781
Diankristanti, P. A., Lin, Y. C., Yi, Y. C., and Ng, I. S. (2023). Polyhydroxyalkanoates bioproduction from bench to industry: thirty years of development towards sustainability. Bioresour. Technol. 130149, 130149. doi:10.1016/j.biortech.2023.130149
Di Bartolo, A., Infurna, G., and Dintcheva, N. T. (2021). A review of bioplastics and their adoption in the circular economy. Polymers 13 (8), 1229. doi:10.3390/polym13081229
Dietrich, K., Dumont, M. J., Del Rio, L. F., and Orsat, V. (2017). Producing PHAs in the bioeconomy — towards a sustainable bioplastic. Sustain Prod. Consum. 9, 58–70. doi:10.1016/j.spc.2016.09.001
Divyashree, M. S., Shamala, T. R., and Rastogi, N. K. (2009). Isolation of polyhydroxyalkanoate from hydrolyzed cells of Bacillus flexus using aqueous two-phase system containing polyethylene glycol and phosphate. Biotechnol. Bioprocess Eng. 14, 482–489. doi:10.1007/s12257-008-0119-z
Dong, X., and Robinson, J. R. (2020). The role of neutral donor ligands in the isoselective ring-opening polymerization of rac-β-butyrolactone. Chem. Sci. 11 (31), 8184–8195. doi:10.1039/d0sc03507f
Doudoroff, M., and Stanier, R. Y. (1959). Role of poly-β-hydroxybutyric acid in the assimilation of organic carbon by bacteria. Nature 183 (4673), 1440–1442. doi:10.1038/1831440a0
El-Taweel, S. H., Stoll, B., Höhne, G. W. H., Mansour, A. A., and y Seliger, H. (2004). Stress-strain behavior of blends of bacterial polyhydroxybutyrate. J. Appl. Polym. Sci. 94 (6), 2528–2537. doi:10.1002/app.21215
Eubeler, J. P., Bernhard, M., and Knepper, T. P. (2010). Environmental biodegradation of synthetic polymers II. Biodegradation of different polymer groups. Trac. Trends Anal. Chem. 29 (1), 84–100. doi:10.1016/j.trac.2009.09.005
European Bioplastics (2022) Bioplastics market. Available at: https://docs.european-bioplastics.org/publications/market_data/2022/Report_Bioplastics_Market_Data_2022_short_version.pdf (Accessed February 14, 2020).
European Commission (2020). European green deal. Available at: https://ec.europa.eu/commission/presscorner/api/files/attachment/869813/EGD_brochure_ES.pdf.pdf (Accessed February 14, 2020).
Ferre-Guell, A., and Winterburn, J. (2018). Biosynthesis and characterization of polyhydroxyalkanoates with controlled composition and microstructure. Biomacromolecules 19, 996–1005. doi:10.1021/acs.biomac.7b01788
Flemming, H. C. (2016). EPS—then and now. Microorganisms 4 (4), 41. doi:10.3390/microorganisms4040041
Flores-Sánchez, A., López-Cuellar, M. D. R., Pérez-Guevara, F., Figueroa López, U., Martín-Bufájer, J. M., and Vergara-Porras, B. (2017). Synthesis of Poly-(R-hydroxyalkanoates) by Cupriavidus necator ATCC 17699 using Mexican avocado (Persea americana) oil as a carbon source. Int. J. Polym. Sci. 2017, 1–10. doi:10.1155/2017/6942950
Fredi, G., and Dorigato, A. (2021). Recycling of bioplastic waste: a review. Ind. Eng. Pol. Adv. Res. 4, 159–177. doi:10.1016/j.aiepr.2021.06.006
Gabirondo, E., Sangroniz, A., Etxeberria, A., Torres-Giner, S., and Sardon, H. (2020). Poly(hydroxy acids) derived from the self-condensation of hydroxy acids: from polymerization to end-of-life options. Polym. Chem. 11, 4861–4874. doi:10.1039/d0py00088d
Gallin, C. F., Lee, W. W., and Byers, J. A. (2023). A simple, selective, and general catalyst for ring closing depolymerization of polyesters and polycarbonates for chemical recycling. Angew. Chem. 135 (25), e202303762. doi:10.1002/anie.202303762
Garcia, I. L., Dorado Perez, M. P., Lopez, J. A., Villar, M. A., Yanniotis, S., and Koutinas, A. (2011). “Design and techno-economic evaluation of microbial biopolymer production from food industry wastes and agricultural crops,” in ICEF11, international congress on engineering and food, food process engineering in a changing World. Atenas, Greece.
Ghosh, S., Coons, J., Yeager, C., Halley, P., Chemodanov, A., Belgorodsky, B., et al. (2022). Halophyte biorefinery for polyhydroxyalkanoates production from Ulva sp. hydrolysate with Haloferax mediterranei in pneumatically agitated bioreactors and ultrasound harvesting. Bioresour. Tech. 344, 125964. doi:10.1016/j.biortech.2021.125964
Ghosh, S., Gnaim, R., Greiserman, S., Fadeev, L., Gozin, M., and Golberg, A. (2019). Macroalgal biomass subcritical hydrolysates for the production of polyhydroxyalkanoate (PHA) by Haloferax mediterranei. Bioresour. Tech. 271, 166–173. doi:10.1016/j.biortech.2018.09.108
Ghosh, S., Greiserman, S., Chemodanov, A., Slegers, P. M., Belgorodsky, B., Epstein, M., et al. (2021). Polyhydroxyalkanoates and biochar from green macroalgal Ulva sp. biomass subcritical hydrolysates: process optimization and a priori economic and greenhouse emissions break-even analysis. Sci. Total Environ. 770, 145281. doi:10.1016/j.scitotenv.2021.145281
Giani, M., Garbayo, I., Vílchez, C., and Martínez-Espinosa, R. M. (2019). Haloarchaeal carotenoids: healthy novel compounds from extreme environments. Mar. drugs 17 (9), 524. doi:10.3390/md17090524
Giani, M., Gervasi, L., Loizzo, M. R., and Martínez-Espinosa, R. M. (2022). Carbon source influences antioxidant, antiglycemic, and antilipidemic activities of Haloferax mediterranei carotenoid extracts. Mar. Drugs 20 (11), 659. doi:10.3390/md20110659
Giani, M., Montero-Lobato, Z., Garbayo, I., Vílchez, C., Vega, J. M., and Martínez-Espinosa, R. M. (2021). Haloferax mediterranei cells as C50 carotenoid factories. Mar. Drugs 19 (2), 100. doi:10.3390/MD19020100
Godoy, M. S., de Miguel, S. R., and Prieto, M. A. (2023). Aerobic-anaerobic transition boosts poly(3-hydroxybutyrate-co-3-hydroxyvalerate) synthesis in Rhodospirillum rubrum: the key role of carbon dioxide. Microb. Cell. Fact. 22, 47. doi:10.1186/s12934-023-02045-x
González-Rojo, S., and Díez-Antolínez, R. (2023). Production of polyhydroxyalkanoates as a feasible alternative for an integrated multiproduct lignocellulosic biorefinery. Bioresour. Technol. 386, 129493. doi:10.1016/j.biortech.2023.129493
Goswami, L., Kushwaha, A., Napathorn, S. C., and Kim, B. S. (2023). Valorization of organic wastes using bioreactors for polyhydroxyalkanoate production: recent advancement, sustainable approaches, challenges, and future perspectives. Int. J. Biol. Macromol. 125743, 125743. doi:10.1016/j.ijbiomac.2023.125743
Guo, M., Stuckey, D. C., and Murphy, R. J. (2013). Is it possible to develop biopolymer production systems independent of fossil fuels? case study in energy profiling of polyhydroxybutyrate-valerate (PHBV). Green. Chem. 15, 706. doi:10.1039/c2gc36546d
Hagagy, N., Saddiq, A. A., Tag, H. M., Selim, S., AbdElgawad, H., and Martínez-Espinosa, R. M. (2022). Characterization of polyhydroxybutyrate, PHB, synthesized by newly isolated haloarchaea Halolamina spp. Molecules 27 (21), 7366. doi:10.3390/molecules27217366
Hahladakis, J. N., Velis, C. A., Weber, R., Iacovidou, E., and Purnell, P. (2018). An overview of chemical additives present in plastics: migration, release, fate and environmental impact during their use, disposal and recycling. J. Hazard Mater 344, 179–199. doi:10.1016/j.jhazmat.2017.10.014
Hammami, K., Souissi, Y., Souii, A., Ouertani, A., El-Hidri, D., Jabberi, M., et al. (2022). Extremophilic bacterium Halomonas desertis G11 as a cell factory for poly-3-hydroxybutyrate-co-3-hydroxyvalerate copolymer’s production. Front. Bioeng. Biotechnol. 10, 878843. doi:10.3389/fbioe.2022.878843
Han, J., Li, M., Hou, J., Wu, L., Zhou, J., and Xiang, H. (2010). Comparison of four phaC genes from Haloferax mediterranei and their function in different PHBV copolymer biosyntheses in Haloarcula hispanica. Saline Syst. 6, 9–10. doi:10.1186/1746-1448-6-9
Han, J., Wu, L. P., Hou, J., Zhao, D., and Xiang, H. (2015). Biosynthesis, characterization, and hemostasis potential of tailor-made poly (3-hydroxybutyrate-co-3-hydroxyvalerate) produced by Haloferax mediterranei. Biomacromolecules 16 (2), 578–588. doi:10.1021/bm5016267
Hathi, Z. J., Haque, M. A., Priya, A., Qin, Z., Huang, S., Lam, C. H., et al. (2022). Fermentative bioconversion of food waste into biopolymer poly(3-hydroxybutyrate-co-3-hydroxyvalerate) using Cupriavidus necator. Environ. Res. 215, 114323. doi:10.1016/j.envres.2022.114323
Hou, J., and Cui, H. L. (2018). In vitro antioxidant, antihemolytic, and anticancer activity of the carotenoids from halophilic archaea. Curr. Microbiol. 75, 266–271. doi:10.1007/s00284-017-1374-z
Huang, T. Y., Duan, K. J., Huang, S. Y., and Chen, C. W. (2006). Production of polyhydroxyalkanoates from inexpensive extruded rice bran and starch by Haloferax mediterranei. J. Ind. Microbiol. Biotech. 33 (8), 701–706. doi:10.1007/s10295-006-0098-z
Im, H., Dwidar, M., and Mitchell, R. J. (2018). Bdellovibrio bacteriovorus HD100, a predator of Gram-negative bacteria, benefits energetically from Staphylococcus aureus biofilms without predation. ISME J. 12, 2090–2095. doi:10.1038/s41396-018-0154-5
Jacquel, N., Lo, C.-W., Wei, Y.-H., Wu, H.-S., and Wang, S. S. (2008). Isolation and purification of bacterial poly(3-hydroxyalkanoates). Biochem. Eng. J. 39, 15–27. doi:10.1016/j.bej.2007.11.029
Jendrossek, D., Schirmer, A., and Schlegel, H. G. (1996). Biodegradation of polyhydroxyalkanoic acids. App Microbiol. Biotechnol. 46, 451–463. doi:10.1007/s002530050844
Kapritchkoff, F. M., Viotti, A. P., Alli, R. C. P., Zuccolo, M., Pradella, J. G. C., Maiorano, A. E., et al. (2006). Enzymatic recovery and purification of polyhydroxybutyrate produced by Ralstonia eutropha. J. Biotechnol. 122, 453–462. doi:10.1016/j.jbiotec.2005.09.009
Khamplod, T., Wongsirichot, P., and Winterburn, J. (2023). Production of polyhydroxyalkanoates from hydrolysed rapeseed meal by Haloferax mediterranei. Bioresour. Technol. 386, 129541. doi:10.1016/j.biortech.2023.129541
Khosravi-Darani, K., and Mozafari, M. R. (2009). Supercritical fluids technology in bioprocess industries: a review. J. Biochem. Technol. 2, 144–152.
Kim, S., and Dale, B. E. (2008). Energy and greenhouse gas profiles of polyhydroxybutyrates derived from corn grain: a life cycle perspective. Environ. Sci. Technol. 42 (20), 7690–7695. doi:10.1021/es8004199
Kobayashi, T., Hori, Y., Kakimoto, M. A., and Imai, Y. (1993). Synthesis of biodegradable polyesters by polycondensation of methyl (R)-3-hydroxybutyrate and methyl (R)-3-hydroxy-valerate. Die Makromol. Chem. Rapid Commun. 14 (12), 785–790. doi:10.1002/marc.1993.030141209
Koller, M. (2019). Polyhydroxyalkanoate biosynthesis at the edge of water activity-haloarchaea as biopolyester factories. Bioengineering 6 (2), 34. doi:10.3390/bioengineering6020034
Koller, M., Atlić, A., Gonzalez-Garcia, Y., Kutschera, C., and Braunegg, G. (2008). Polyhydroxyalkanoate (PHA) biosynthesis from whey lactose. Macromol. Symp. 272 (1), 87–92. doi:10.1002/masy.200851212
Koller, M., Chiellini, E., and Braunegg, G. (2015). Study on the production and re-use of poly (3-hydroxybutyrate-co-3-hydroxyvalerate) and extracellular polysaccharide by the archaeon Haloferax mediterranei strain DSM 1411. Chem. Biochem. Eng. Q. 29 (2), 87–98. doi:10.15255/CABEQ.2014.2058
Koller, M., Hesse, P., Bona, R., Kutschera, C., Atlić, A., and Braunegg, G. (2007). Potential of various archae- and eubacterial strains as industrial polyhydroxyalkanoate producers from whey. Macromol. Biosci. 7 (2), 218–226. doi:10.1002/mabi.200600211
Koller, M., Hesse, P., Fasl, H., Stelzer, F., and Braunegg, G. (2017). Study on the effect of levulinic acid on whey-based biosynthesis of poly (3-hydroxybutyrate-co-3-hydroxyvalerate) by Hydrogenophaga pseudoflava. App Food Biotechnol. 4 (2), 65–78. doi:10.22037/afb.v4i2.16337
Koller, M., and Rittmann, S. K. M. R. (2022). Haloarchaea as emerging big players in future polyhydroxyalkanoate bioproduction: review of trends and perspectives. Curr. Res. Biotechnol. 4, 377–391. doi:10.1016/j.crbiot.2022.09.002
Kumar, M., Rathour, R., Singh, R., Sun, Y., Pandey, A., Gnansounou, E., et al. (2020). Bacterial polyhydroxyalkanoates: opportunities, challenges, and prospects. J. Clean. Prod. 263, 121500. doi:10.1016/j.jclepro.2020.121500
Kumar, M., Singhal, A., Verma, P. K., and Thakur, I. S. (2017). Production and characterization of polyhydroxyalkanoate from lignin derivatives by Pandoraea sp. ISTKB. ACS omega 2 (12), 9156–9163. doi:10.1021/acsomega.7b01615
Kunasundari, B., and Sudesh, K. (2011). Isolation and recovery of microbial polyhydroxyalkanoates. EXPRESS Polym. Lett. 5, 620–634. doi:10.3144/expresspolymlett.2011.60
Kurian, N. S., and Das, B. (2021). Comparative analysis of various extraction processes based on economy, eco-friendly, purity and recovery of polyhydroxyalkanoate: a review. Int. J. Biol. Macromol. 183, 1881–1890. doi:10.1016/j.ijbiomac.2021.06.007
Lee, O. K., Kang, S. G., Choi, T. R., Yang, Y. H., and Lee, E. Y. (2023). Production and characterization of a biodegradable polymer, poly (3-hydroxybutyrate-co-3-hydroxyvalerate), using the type II methanotroph, Methylocystis sp. MJC1. Bioresour. Technol. 389, 129853. doi:10.1016/j.biortech.2023.129853
Lehnertz, M. S., Mensah, J. B., and Palkovits, R. (2022). Chemical recycling of polyhydroxybutyrate and polylactic acid over supported Ru catalysts. Green Chem. 24 (10), 3957–3963. doi:10.1039/d2gc00216g
Li, F., Yu, D., Lin, X., Liu, D., Xia, H., and Chen, S. (2012). Biodegradation of poly (ε-caprolactone)(PCL) by a new Penicillium oxalicum strain DSYD05-1. W J. Microbiol. Biotechnol. 28, 2929–2935. doi:10.1007/s11274-012-1103-5
Li, Y., and Strathmann, T. J. (2019). Kinetics and mechanism for hydrothermal conversion of polyhydroxybutyrate (PHB) for wastewater valorization. Green Chem. 21 (20), 5586–5597. doi:10.1039/c9gc02507c
Lin, L., Chen, J., Mitra, R., Gao, Q., Cheng, F., Xu, T., et al. (2021). Optimising PHBV biopolymer production in haloarchaea via CRISPRi-mediated redirection of carbon flux. Commun. Biol. 4 (1), 1007. doi:10.1038/s42003-021-02541-z
Liu, J., Zhao, Y., Diao, M., Wang, W., Hua, W., Wu, S., et al. (2019). Poly(3-hydroxybutyrate-co- 3-hydroxyvalerate) production by Rhodospirillum rubrum using a two-step culture strategy. J. Chem. 2019, 1–8. doi:10.1155/2019/8369179
Liwarska-Bizukojc, E. (2021). Effect of (bio) plastics on soil environment: a review. Sci. Total Environ. 795, 148889. doi:10.1016/j.scitotenv.2021.148889
Lu, Q., Han, J., Zhou, L., Zhou, J., and Xiang, H. (2008). Genetic and biochemical characterization of the poly (3-hydroxybutyrate-co-3-hydroxyvalerate) synthase in Haloferax mediterranei. J. Bacteriol. 190 (12), 4173–4180. doi:10.1128/JB.00134-08
Luangthongkam, P., Laycock, B., Evans, P., Lant, P., and Pratt, S. (2019). Thermophilic production of poly(3-hydroxybutyrate-co-3-hydrovalerate) by a mixed methane-utilizing culture. New Biotechnol. 53, 49–56. doi:10.1016/j.nbt.2019.06.008
Ma, W., Wang, J., Li, Y., Yin, L., and Wang, X. (2018). Poly(3-hydroxybutyrate-co-3- hydroxyvalerate) co-produced with l-isoleucine in Corynebacterium glutamicum WM001. Microb. Cell. Fact. 17, 93–12. doi:10.1186/s12934-018-0942-7
Main, P., Petersmann, S., Wild, N., Feuchter, M., Duretek, I., Edeleva, M., et al. (2023a). Impact of multiple reprocessing on properties of polyhydroxybutyrate and polypropylene. Polymers 15 (20), 4126. doi:10.3390/polym15204126
Mannina, G., Presti, D., Montiel-Jarillo, G., and Su´arez-Ojeda, M. E. (2019). Bioplastic recovery from wastewater: a new protocol for polyhydroxyalkanoates (PHA) extraction from mixed microbial cultures. Bioresour. Technol. 282, 361–369. doi:10.1016/j.biortech.2019.03.037
Martínez-Espinosa, R. M., Richardson, D. J., and Bonete, M. J. (2015). Characterisation of chlorate reduction in the haloarchaeon Haloferax mediterranei. Biochim. Biophys. Acta (BBA)-General Subj. 1850 (4), 587–594. doi:10.1016/j.bbagen.2014.12.011
Martínez Martínez, G., Pire, C., and Martínez-Espinosa, R. M. (2022). Hypersaline environments as natural sources of microbes with potential applications in biotechnology: the case of solar evaporation systems to produce salt in Alicante County (Spain). Curr. Res. Microb. Sci. 3, 100136. doi:10.1016/j.crmicr.2022.100136
Masood, F., Hasan, F., Ahmed, S., and Hameed, A. (2012). Biosynthesis and characterization of poly (3-hydroxybutyrate-co-3-hydroxyvalerate) from Bacillus cereus FA11 isolated from TNT-contaminated soil. Ann. Microbiol. 62, 1377–1384. doi:10.1007/s13213-011-0386-3
Melchiors, M., Keul, H., and Höcker, H. (1996). Depolymerization of poly [(R)-3-hydroxybutyrate] to cyclic oligomers and polymerization of the cyclic trimer: an example of thermodynamic recycling. Macromolecules 29 (20), 6442–6451. doi:10.1021/ma9604350
Meng, D., Miao, C., Liu, Y., Wang, F., Chen, L., Huang, Z., et al. (2022). Metabolic engineering for biosynthesis of poly(3-hydroxybutyrate-co-3-hydroxyvalerate) from glucose and propionic acid in recombinant Escherichia coli. Bioresour. Technol. 348, 126786. doi:10.1016/j.biortech.2022.126786
Miao, C., Meng, D., Liu, Y., Wang, F., Chen, L., Huang, Z., et al. (2021). Biosynthesis of poly(3-hydroxybutyrate-co-3-hydroxyvalerate) in metabolically recombinant Escherichia coli. Int. J. Biol. Macromol. 193, 956–964. doi:10.1016/j.ijbiomac.2021.10.183
Miscevic, D., Srirangan, K., Kefale, T., Kilpatrick, S., Chung, D. A., Moo-Young, M., et al. (2019). Heterologous production of 3-hydroxyvalerate in engineered Escherichia coli. Metab. Eng. 61, 141–151. doi:10.1016/j.ymben.2019.11.005
Montemurro, M., Salvatori, G., Alfano, S., Martinelli, A., Verni, M., Pontonio, E., et al. (2022). Exploitation of wasted bread as substrate for polyhydroxyalkanoates production through the use of Haloferax mediterranei and seawater. Front. Microbiol. 13, 1000962. doi:10.3389/fmicb.2022.1000962
Moopantakath, J., Imchen, M., Anju, V. T., Busi, S., Dyavaiah, M., Martínez-Espinosa, R. M., et al. (2023). Bioactive molecules from haloarchaea: scope and prospects for industrial and therapeutic applications. Front. Microbiol. 14, 1113540. doi:10.3389/fmicb.2023.1113540
Morya, R., Andrianantenaina, F. H., Pandey, A. K., Yoon, Y. H., and Kim, S. H. (2023). Polyhydroxyalkanoate production from rice straw hydrolysate: insights into feast-famine dynamics and microbial community shifts. Chemosphere 341, 139967. doi:10.1016/j.chemosphere.2023.139967
Mozejko-Ciesielska, J., Moraczewski, K., Czaplicki, S., and Singh, V. (2023). Production and characterization of polyhydroxyalkanoates by Halomonas alkaliantarctica utilizing dairy waste as feedstock. Sci. Rep. 13 (1), 22289. doi:10.1038/s41598-023-47489-8
Mukai, K., Yamada, K., and Doi, Y. (1993). Kinetics and mechanism of heterogeneous hydrolysis of poly [(R)-3-hydroxybutyrate] film by PHA depolymerases. Int. J. Biol. Macromol. 15 (6), 361–366. doi:10.1016/0141-8130(93)90054-p
Myung, J., Flanagan, J. C. A., Waymouth, R. M., and Criddle, C. S. (2024). Ethane-dependent synthesis of polyhydroxyalkanoates by the obligate methanotroph Methylocystis parvus OBBP. J. Chem. Eng. 483, 149210. doi:10.1016/j.cej.2024.149210
Myung, J., Galega, W. M., Van Nostrand, J. D., Yuan, T., Zhou, J., and Criddle, C. S. (2015). Long-term cultivation of a stable Methylocystis-dominated methanotrophic enrichment enabling tailored production of poly(3-hydroxybutyrate-co-3-hydroxyvalerate). Bioresour. Technol. 198, 811–818. doi:10.1016/j.biortech.2015.09.094
Nájera-Fernández, C., Zafrilla, B., Bonete, M. J., and Martínez-Espinosa, R. M. (2012). Role of the denitrifying Haloarchaea in the treatment of nitrite-brines. Int. Microbiol. 15, 111–119. doi:10.2436/20.1501.01.164
Nessi, S., Sinkko, T., Bulgheroni, C., Garcia-Gutierrez, P., Giuntoli, J., Konti, A., et al. (2021) Life Cycle Assessment (LCA) of alternative feedstocks for plastics production. Luxembourg: Publications Office of the European Union. doi:10.2760/655230
Novackova, I., Kucera, D., Porizka, J., Pernicova, I., Sedlacek, P., Koller, M., et al. (2019). Adaptation of Cupriavidus necator to levulinic acid for enhanced production of P (3HB- co -3HV) copolyesters. Biochem. Eng. J. 151, 107350. doi:10.1016/j.bej.2019.107350
Obruca, S., Marova, I., Snajdar, O., Mravcova, L., and Svoboda, Z. (2010). Production of poly(3-hydroxybutyrate-co-3-hydroxyvalerate) by Cupriavidus necator from waste rapeseed oil using propanol as a precursor of 3-hydroxyvalerate. Biotechnol. Lett. 32 (12), 1925–1932. doi:10.1007/s10529-010-0376-8
Obruca, S., Sedlacek, P., Koller, M., Kucera, D., and Pernicova, I. (2018). Involvement of polyhydroxyalkanoates in stress resistance of microbial cells: biotechnological consequences and applications. Biotechnol. Adv. 36, 856–870. doi:10.1016/j.biotechadv.2017.12.006
Obulisamy, P. K., and Mehariya, S. (2021). Polyhydroxyalkanoates from extremophiles: a review. Bioresour. Technol. 325, 124653. doi:10.1016/j.biortech.2020.124653
Ono, H., Ebisuya, S., Osanai, S., Tanaka, S., Tamura, M., Yoshida, M., et al. (2023). Dehydrative polycondensation of (R)-3-Hydroxybutyric acid with an acid/phosphine system: formation of OH/COOH telechelic biodegradable polyester. Chem. Lett. 52, 485–487. doi:10.1246/cl.230149
Oren, A. (2010). Industrial and environmental applications of halophilic microorganisms. Environ. Technol. 31 (8-9), 825–834. doi:10.1080/09593330903370026
Pacholak, A., Gao, Z. L., Gong, X. Y., Kaczorek, E., and Cui, Y. W. (2021). The metabolic pathways of polyhydroxyalkanoates and exopolysaccharides synthesized by Haloferax mediterranei in response to elevated salinity. J. Proteomics 232, 104065. doi:10.1016/j.jprot.2020.104065
Pais, J., Serafim, L. S., Freitas, F., and Reis, M. A. M. (2016). Conversion of cheese whey into poly(3-hydroxybutyrate-co-3-hydroxyvalerate) by Haloferax mediterranei. New Biotechnol. 33, 224–230. doi:10.1016/j.nbt.2015.06.001
Parroquin-Gonzalez, M., and Winterburn, J. (2023). Continuous bioreactor production of polyhydroxyalkanoates in Haloferax mediterranei. Front. Bioeng. Biotechnol. 11, 1220271. doi:10.3389/fbioe.2023.1220271
Patil, T. D., Ghosh, S., Agarwal, A., Patel, S. K. S., Tripathi, A. D., Mahato, D. K., et al. (2024). Production, optimization, scale up and characterization of polyhydoxyalkanoates copolymers utilizing dairy processing waste. Sci. Rep. 14, 1620. doi:10.1038/s41598-024-52098-0
Pawelzik, P., Carus, M., Hotchkiss, J., Narayan, R., Selke, S., Wellisch, M., et al. (2013). Critical aspects in the life cycle assessment (LCA) of bio-based materials - reviewing methodologies and deriving recommendations. Resour. Conserv. Recycl 73, 211–228. doi:10.1016/j.resconrec.2013.02.006
Pérez, V., Mota, C. R., Muñoz, R., and Lebrero, R. (2020). Polyhydroxyalkanoates (PHA) production from biogas in waste treatment facilities: assessing the potential impacts on economy, environment and society. Chemosphere 255, 126929. doi:10.1016/j.chemosphere.2020.126929
Pfeifer, K., Ergal, İ., Koller, M., Basen, M., Schuster, B., and Rittmann, S. K. M. R. (2021). Archaea biotechnology. Biotechnol. Adv. 47, 107668. doi:10.1016/j.biotechadv.2020.107668
Pinho, I., Amezcua, F., Rivera, J. M., Green-Ruiz, C., Piñón-Colin, T. de J., and Wakida, F. (2022). First report of plastic contamination in batoids: plastic ingestion by Haller’s Round Ray (Urobatis halleri) in the Gulf of California. Environ. Res. 211, 113077. doi:10.1016/j.envres.2022.113077
Plastics Europe (2022) Plastics-the facts 2022. Available at: https://plasticseurope.org/knowledge-hub/plastics-the-facts-2022/ (Accessed March 15, 2024).
Plastics strategy (2020) Environment. Available at: https://environment.ec.europa.eu/strategy/plastics-strategy_en (Accessed March 15, 2024).
Policastro, G., Panico, A., and Fabbricino, M. (2021). Improving biological production of poly(3-hydroxybutyrate-co-3-hydroxyvalerate) (PHBV) co-polymer: a critical review. Rev. Environ. Sci. Biotechnol. 20, 479–513. doi:10.1007/s11157-021-09575-z
Priya, A., Hathi, Z., Haque, M. A., Kumar, S., Kumar, A., Singh, E., et al. (2022). Effect of levulinic acid on production of polyhydroxyalkanoates from food waste by Haloferax mediterranei. Environ. Res. 214, 114001. doi:10.1016/j.envres.2022.114001
Raho, S., Carofiglio, V. E., Montemurro, M., Miceli, V., Centrone, D., Stufano, P., et al. (2020). Production of the polyhydroxyalkanoate PHBV from ricotta cheese exhausted whey by Haloferax mediterranei fermentation. Foods 9, 1459. doi:10.3390/foods9101459
Rasmussen, S. C. (2021). From Parkesine to celluloid: the birth of organic plastics. Angew. Chem. 133, 8090–8094. doi:10.1002/ange.202015095
Ren, Z. W., Wang, Z. Y., Ding, Y. W., Dao, J. W., Li, H. R., Ma, X., et al. (2023). Polyhydroxyalkanoates: the natural biopolyester for future medical innovations. Biomat Sci. 11, 6013–6034. doi:10.1039/d3bm01043k
Renard, E., Walls, M., Guérin, P., and Langlois, V. (2004). Hydrolytic degradation of blends of polyhydroxyalkanoates and functionalized polyhydroxyalkanoates. Polym. Degrad. Stab. 85 (2), 779–787. doi:10.1016/S0141-3910(04)00018-7
Rigouin, C., Lajus, S., Ocando, C., Borsenberger, V., Nicaud, J. M., Marty, A., et al. (2019). Production and characterization of two medium-chain-length polydroxyalkanoates by engineered strains of Yarrowia lipolytica. Microb. Cell. Fact. 18, 99–9. doi:10.1186/s12934-019-1140-y
Rivas, L. F., Casarin, S. A., Nepomuceno, N. C., Alencar, M. I., Agnelli, J. A. M., De Medeiros, E. S., et al. (2017). Reprocessability of PHB in extrusion: ATR-FTIR, tensile tests and thermal studies. Polymers 27, 122–128. doi:10.1590/0104-1428.2406
Rivas-Castillo, A. M., Valdez-Calderón, A., Angeles-Padilla, A. F., Figueroa-Ocampo, C. B., Carrillo-Ibarra, S., Quezada-Cruz, M., et al. (2024). PHB production by Bacillus megaterium strain MNSH1-9K-1 using low-cost media. Braz. J. Microbiol. 55, 245–254. doi:10.1007/s42770-023-01232-7
Rivera-Briso, A., and Serrano-Aroca, Á. (2018). Poly(3-hydroxybutyrate-co-3-hydroxyvalerate): enhancement strategies for advanced applications. Polymers 10, 732. doi:10.3390/polym10070732
Rodríguez-Cendal, A. I., Gómez-Seoane, I., de Toro-Santos, F. J., Fuentes-Boquete, I. M., Señarís-Rodríguez, J., and Díaz-Prado, S. M. (2023). Biomedical applications of the biopolymer poly (3-hydroxybutyrate-co-3-hydroxyvalerate) (PHBV): drug encapsulation and scaffold fabrication. Int. Jf Mol. Sci. 24 (14), 11674. doi:10.3390/ijms241411674
Rodríguez-Contreras, A., Calafell-Monfort, M., and y Marqués-Calvo, M. S. (2012). Enzymatic degradation of poly(3-hydroxybutyrate-co-4-hydroxybutyrate) by commercial lipases. Polym. Degrad. Stab. 97 (4), 597–604. doi:10.1016/j.polymdegradstab.2012.01.007
Rodriguez-Perez, S., Serrano, A., Pantión, A. A., and Alonso-Fariñas, B. (2018). Challenges of scaling-up PHA production from waste streams. A review. J. Environ. Manag. 205, 215–230. doi:10.1016/j.jenvman.2017.09.083
Roibás-Rozas, A., Oso, del, Zarroli, G., Mauricio-Iglesias, M., Mosquera-Corral, A., Fiore, S., et al. (2022) How can we validate the environmental profile of bioplastics? Towards the introduction of polyhydroxyalkanoates (PHA) in the value chains. Elsevier eBooks, 405–429. doi:10.1016/b978-0-323-85851-9.00010-9
Roohi, Z., and Kuddus, M. (2018). PHB (poly-β-hydroxybutyrate) and its enzymatic degradation. Pol. Adv. Technol. 29 (1), 30–40. doi:10.1002/pat.4126
Salem, R., ElDyasti, A., and Audette, G. F. (2021). Biomedical applications of biomolecules isolated from methanotrophic bacteria in wastewater treatment systems. Biomolecules 11 (8), 1217. doi:10.3390/biom11081217
Salgaonkar, B. B., Mani, K., and Bragança, J. M. (2018). Sustainable bioconversion of cassava waste to poly(3-hydroxybutyrate-co-3-hydroxyvalerate) by Halogeometricum borinquense strain E3. J. Pol. Environ. 27 (2), 299–308. doi:10.1007/s10924-018-1346-9
Sanchez, J. G., Tsuchii, A., and Tokiwa, Y. (2000). Degradation of polycaprolactone at 50° C by a thermotolerant Aspergillus sp. Biotechnol. Lett. 22, 849–853. doi:10.1023/a:1005603112688
Santiago, B., Sillero, L., Moreira, M. T., Feijoo, G., and González-García, S. (2023) Valorisation of agro-industrial waste: recent advances in the recovery of bioactive compounds and environmental perspectives. doi:10.1039/BK978183767009300001
Santos, M., Gangoiti, J., Keul, H., Möller, M., Serra, J. L., and Llama, M. J. (2013). Polyester hydrolytic and synthetic activity catalyzed by the medium-chain-length poly (3-hydroxyalkanoate) depolymerase from Streptomyces venezuelae SO1. Appl. Microbiol. Biotechnol. 97, 211–222. doi:10.1007/s00253-012-4210-1
Shah, A. A., Hasan, F., Hameed, A., and Ahmed, S. (2008). Biological degradation of plastics: a comprehensive review. BiotechnolAdvs 26 (3), 246–265. doi:10.1016/j.biotechadv.2007.12.005
Shahid, S., Razzaq, S., Farooq, R., and Nazli, Z. i. H. (2021). Polyhydroxyalkanoates: next generation natural biomolecules and a solution for the world’s future economy. I J. Biol. Macromol. 166, 297–321. doi:10.1016/j.ijbiomac.2020.10.187
Sheu, D. S., Chen, W. M., Yang, J. Y., and Chang, R. C. (2009). Thermophilic bacterium Caldimonas taiwanensis produces poly(3-hydroxybutyrate- co-3-hydroxyvalerate) from starch and valerate as carbon sources. Enzyme Microb. Technol. 44, 289–294. doi:10.1016/j.enzmictec.2009.01.004
Silva, R. R. A., Marques, C. S., Arruda, T. R., Teixeira, S. C., and de Oliveira, T. V. (2023). Biodegradation of polymers: stages, measurement, standards and prospects. Macromol 3, 371–399. doi:10.3390/macromol3020023
Simó-Cabrera, L., García-Chumillas, S., Hagagy, N., Saddiq, A., Tag, H., Selim, S., et al. (2021). Haloarchaea as cell factories to produce bioplastics. Mar. Drugs 19 (3), 159. doi:10.3390/MD19030159
Sinaei, N., Zare, D., and Azin, M. (2021). Production and characterization of poly 3-hydroxybutyrate-co-3-hydroxyvalerate in wheat starch wastewater and its potential for nanoparticle synthesis. Braz. J. Microbiol. 52, 561–573. doi:10.1007/s42770-021-00430-5
Smith, R. L., West, T. P., and Gibbons, W. R. (2008). Rhodospirillum rubrum: utilization of condensed corn solubles for poly-(3-hydroxybutyrate-co-3-hydroxyvalerate) production. J. App Microbiol. 104 (5), 1488–1494. doi:10.1111/j.1365-2672.2007.03685.x
Spierling, S., Knüpffer, E., Behnsen, H., Mudersbach, M., Krieg, H., Springer, S., et al. (2018). Bio-based plastics - a review of environmental, social and economic impact assessments. J. Clean. Prod. 185, 476–491. doi:10.1016/j.jclepro.2018.03.014
Suhazsini, P., Keshav, R., Narayanan, S., Chaudhuri, A., and Radha, P. (2020). A Study on the synthesis of poly (3-hydroxybutyrate-co-3-hydroxyvalerate) by Bacillus megaterium utilizing cheese whey permeate. J. Pol. Environ. 28, 1390–1405. doi:10.1007/s10924-020-01687-x
Tabone, M. D., Cregg, J. J., Beckman, E. J., and Landis, A. E. (2010). Sustainability metrics: life cycle assessment and green design in polymers. Environ. Sci. Technol. 44 (21), 8264–8269. doi:10.1021/es101640n
Tamer, I. M., Moo-Young, M., and Chisti, Y. (1998). Disruption of Alcaligenes latus for recovery of poly(β-hydroxybutyric acid): comparison of high-pressure homogenization, bead milling, and chemically induced lysis. Ind. Eng. Chem. Res. 37, 1807–1814. doi:10.1021/ie9707432
Tan, D., Wu, Q., Chen, J. C., and Chen, G. Q. (2014a). Engineering Halomonas TD01 for the low-cost production of polyhydroxyalkanoates. Metab. Eng. 26, 34–47. doi:10.1016/j.ymben.2014.09.001
Tan, G. Y. A., Chen, C. L., Li, L., Ge, L., Wang, L., Razaad, I. M. N., et al. (2014b). Start a research on biopolymer polyhydroxyalkanoate (PHA): a review. Polym. (Basel) 6, 706–754. doi:10.3390/polym6030706
Tang, X., and Chen, E. Y. X. (2018). Chemical synthesis of perfectly isotactic and high melting bacterial poly (3-hydroxybutyrate) from bio-sourced racemic cyclic diolide. Nat. Commun. 9 (1), 2345. doi:10.1038/s41467-018-04734-3
Tang, X., Westlie, A. H., Watson, E. M., and Chen, E. Y. X. (2019). Stereosequenced crystalline polyhydroxyalkanoates from diastereomeric monomer mixtures. Science 366 (6466), 754–758. doi:10.1126/science.aax8466
Tebaldi, M. L., Maia, A. L. C., Poletto, F., de Andrade, F. V., and Soares, D. C. F. (2019). Poly(-3-Hydroxybutyrate-Co-3-Hydroxyvalerate) (PHBV): current advances in synthesis methodologies, antitumor applications and biocompatibility. J. Drug Deliv. Sci. Technol. 51, 115–126. doi:10.1016/j.jddst.2019.02.007
Tian, L., Li, H., Song, X., Ma, L., and Li, Z. J. (2022). Production of polyhydroxyalkanoates by a novel strain of Photobacterium using soybean oil and corn starch. J. Environ. Chem. Eng. 10 (5), 108342. doi:10.1016/j.jece.2022.108342
Toh, P. S., Jau, M. H., Yew, S. P., Abed, R. M., and Sudesh, K. (2008). Comparison of polyhydroxyalkanoates biosynthesis, mobilization and the effects on cellular morphology in Spirulina platensis and Synechocystis sp. Uniwge. Trop. Life Sci. Res. 19 (2), 21–38.
Urtuvia, V., Ponce, B., Andler, R., Peña, C., and Diaz-Barrera, A. (2022). Extended batch cultures for poly (3-hydroxybutyrate-co-3-hydroxyvalerate)(PHBV) production by Azotobacter vinelandii OP growing at different aeration rates. 3 Biotech. 12 (11), 304. doi:10.1007/s13205-022-03380-3
Venâncio, C., Lopes, I., and Oliveira, M. (2022). Bioplastics: known effects and potential consequences to marine and estuarine ecosystem services. Chemosphere 309, 136810. doi:10.1016/j.chemosphere.2022.136810
Vu, D. H., Mahboubi, A., Root, A., Heinmaa, I., Taherzadeh, M. J., and Åkesson, D. (2022). Thorough investigation of the effects of cultivation factors on polyhydroalkanoates (PHAs) production by Cupriavidus necator from food waste-derived volatile fatty acids. Fermentation 8 (11), 605. doi:10.3390/fermentation8110605
Wagle, A. R., Dixit, Y. M., and Vakil, B. V. (2019). Scale up studies for polyhydroxyalkanoate production by a Bacillus flexus strain with industrial potential. Indian. J. Microbiol. 59, 383–386. doi:10.1007/s12088-019-00807-z
Walker, S., and Rothman, R. (2020). Life cycle assessment of bio-based and fossil-based plastic: a review. J. Clean. Prod. 261, 121158. doi:10.1016/j.jclepro.2020.121158
Wang, K., Chen, C., and Zhang, R. (2022). Process development of polyhydroxyalkanoates production by halophiles valorising food waste. Bioengineering 9 (11), 630. doi:10.3390/bioengineering9110630
Wang, K., and Zhang, R. (2021). Production of polyhydroxyalkanoates (PHA) by Haloferax mediterranei from food waste derived nutrients for biodegradable plastic applications. J. Microbiol. Biotechnol. 31 (2), 338–347. doi:10.4014/JMB.2008.08057
Wang, Y., Dao, J., and Chen, G. Q. (2019) 3.21 polyhydroxyalkanoate/polyhydroxybutyrate. Available at: https://www.sciencedirect.com/science/article/abs/pii/B9780444640468001634 (Accessed March 14, 2024).
Webb, J. S., Nixon, M., Eastwood, I. M., Greenhalgh, M., Robson, G. D., and Handley, P. S. (2000). Fungal colonization and biodeterioration of plasticized polyvinyl chloride. App Environ. Microbiol. 66 (8), 3194–3200. doi:10.1128/aem.66.8.3194-3200.2000
Westhues, S., Idel, J., and Klankermayer, J. (2018). Molecular catalyst systems as key enablers for tailored polyesters and polycarbonate recycling concepts. Sci. Adv. 4 (8), eaat9669. doi:10.1126/sciadv.aat9669
Westlie, A. H., Hesse, S. A., Tang, X., Quinn, E. C., Parker, C. R., Takacs, C. J., et al. (2023). All-polyhydroxyalkanoate triblock copolymers via a stereoselective-chemocatalytic route. ACS Macro Lett. 12 (5), 619–625. doi:10.1021/acsmacrolett.3c00162
Westlie, A. H., Quinn, E. C., Parker, C. R., and Chen, E. Y. X. (2022). Synthetic biodegradable polyhydroxyalkanoates (PHAs): recent advances and future challenges. Prog. Polym. Sci. 134, 101608. doi:10.1016/j.progpolymsci.2022.101608
Yang, H., Chen, J., Mitra, R., Xue, Q., Xiang, H., and Han, J. (2022). Replication origin deletion enhances Poly (3-Hydroxybutyrate-co-3-Hydroxyvalerate) synthesis in haloarchaea. Microbiol. Spectr. 10 (6), e0214922–22. doi:10.1128/spectrum.02149-22
Yang, S., Huang, Z., Jiang, Z., and Li, L. (2008). Partition and purification of a thermostable xylanase produced by Paecilomyces thermophila in solid-state fermentation using aqueous two-phase systems. Process Biochem. 43, 56–61. doi:10.1016/j.procbio.2007.10.013
Yang, X., Clénet, J., Xu, H., Odelius, K., and Hakkarainen, M. (2015). Two step extrusion process: from thermal recycling of PHB to plasticized PLA by reactive extrusion grafting of PHB degradation products onto PLA chains. Macromolecules 48 (8), 2509–2518. doi:10.1021/acs.macromol.5b00235
Yates, M. R., and Barlow, C. Y. (2013). Life cycle assessments of biodegradable, commercial biopolymers - a critical review. Resour. Conserv. Recycl 78, 54–66. doi:10.1016/j.resconrec.2013.06.010
Yin, J., Wang, H., Fu, X. Z., Gao, X., Wu, Q., and Chen, G. Q. (2015). Effects of chromosomal gene copy number and locations on polyhydroxyalkanoate synthesis by Escherichia coli and Halomonas sp. Appl. Microbiol. Biotechnol. 99, 5523–5534. doi:10.1007/s00253-015-6510-8
Yu, G. E., and Marchessault, R. H. (2000). Characterization of low molecular weight poly(β-hydroxybutyrate)s from alkaline and acid hydrolysis. Polymer 41 (3), 1087–1098. doi:10.1016/s0032-3861(99)00230-x
Yu, J., and Chen, L. X. L. (2006). Cost-effective recovery and purification of polyhydroxyalkanoates by selective dissolution of cell mass. Biotechnol. Prog. 22, 547–553. doi:10.1021/bp050362g
Yu, J., Plackett, D., and Chen, L. X. (2005). Kinetics and mechanism of the monomeric products from abiotic hydrolysis of poly [(R)-3-hydroxybutyrate] under acidic and alkaline conditions. Polym. Degrad. Stab. 89 (2), 289–299. doi:10.1016/j.polymdegradstab.2004.12.026
Yu, L., Dean, K., and Li, L. (2006). Polymer blends and composites from renewable resources. Prog. Polym. Sci. 31, 576–602. doi:10.1016/J.PROGPOLYMSCI.2006.03.002
Zaverl, M., Seydibeyoğlu, M. Ö., Misra, M., and Mohanty, A. (2012). Studies on recyclability of polyhydroxybutyrate-co-valerate bioplastic: multiple melt processing and performance evaluations. J. Appl. Polym. Sci. 125 (S2), E324–E331. doi:10.1002/app.36840
Zembouai, I., Bruzaud, S., Kaci, M., Benhamida, A., Corre, Y. M., and Grohens, Y. (2014). Mechanical recycling of poly (3-hydroxybutyrate-co-3-hydroxyvalerate)/polylactide based blends. J. Polym. Environ. 22, 449–459. doi:10.1007/s10924-014-0684-5
Zhang, G., Zheng, W., Bai, X., Xu, L., Li, K., Zhang, M., et al. (2024). Polyhydroxyalkanoates (PHAs) biological recovery approaches and protein-mediated secretion model hypothesis. J. Clean. Prod. 440, 140851. doi:10.1016/j.jclepro.2024.140851
Zhang, H., Kang, S., Li, J., Li, Z., Chang, J., Xu, Y., et al. (2021). Near zero-waste biofuel production from bioderived polyhydroxybutyrate. Fuel 286, 119405. doi:10.1016/j.fuel.2020.119405
Zhang, Y. Z., Liu, G. M., Weng, W. Q., Ding, J. Y., and Liu, S. J. (2015). Engineering of Ralstonia eutropha for the production of poly (3-hydroxybutyrate-co-3-hydroxyvalerate) from glucose. J. Biotechnol. 195, 82–88. doi:10.1016/j.jbiotec.2014.12.014
Zhang, Z., Shi, C., Scoti, M., Tang, X., and Chen, E. Y. X. (2022). Alternating isotactic polyhydroxyalkanoates via site-and stereoselective polymerization of unsymmetrical diolides. ACS J. 144 (43), 20016–20024. doi:10.1021/jacs.2c08791
Zher Neoh, S., Fey Chek, M., Tiang Tan, H., Linares-Pastén, J. A., Nandakumar, A., Hakoshima, T., et al. (2022). Polyhydroxyalkanoate synthase (PHAC): the key enzyme for biopolyester synthesis. CRBIOT 4, 87–101. doi:10.1016/j.crbiot.2022.01.002
Zhuo, Z., Zhang, C., Luo, Y., Wang, Y., Yao, Y., Yuan, D., et al. (2018). Stereo-selectivity switchable ROP of rac-β-butyrolactone initiated by salan-ligated rare-earth metal amide complexes: the key role of the substituents on ligand frameworks. Chem. Commun. 54 (85), 11998–12001. doi:10.1039/c8cc05469j
Zinn, M., Weilenmann, H. U., Hany, R., Schmid, M., and Eglin, T. H. (2023). Tailored synthesis of poly([R]-3-hydroxybutyrate-co-3-hydroxyvalerate) (PHB/HV) in Ralstonia eutropha DSM 428. Acta Biotechnol. 23, 309–316. doi:10.1002/abio.200390039
Zintl, M., Molnar, F., Urban, T., Bernhart, V., Preishuber-Pflügl, P., and Rieger, B. (2008). Variably isotactic poly(hydroxybutyrate) from racemic β-butyrolactone: microstructure control by achiral chromium(III) salophen complexes. Angew. Chem. Int. Ed. 47 (18), 3458–3460. doi:10.1002/anie.200703859
Keywords: poly (3-hydroxybutyrate-co-3-hydroxyvalerate), polyhydroxyalkanoates, microbial cell factories, circular economy, biopolymers, waste valorisation, recyclability
Citation: García-Chumillas S, Guerrero-Murcia T, Nicolás-Liza M, Monzó F, Simica A, Simó-Cabrera L and Martínez-Espinosa RM (2024) PHBV cycle of life using waste as a starting point: from production to recyclability. Front. Mater. 11:1405483. doi: 10.3389/fmats.2024.1405483
Received: 22 March 2024; Accepted: 16 May 2024;
Published: 03 June 2024.
Edited by:
Chi-Hui Tsou, Sichuan University of Science and Engineering, ChinaReviewed by:
Patrizia Cinelli, University of Pisa, ItalyCopyright © 2024 García-Chumillas, Guerrero-Murcia, Nicolás-Liza, Monzó, Simica, Simó-Cabrera and Martínez-Espinosa. This is an open-access article distributed under the terms of the Creative Commons Attribution License (CC BY). The use, distribution or reproduction in other forums is permitted, provided the original author(s) and the copyright owner(s) are credited and that the original publication in this journal is cited, in accordance with accepted academic practice. No use, distribution or reproduction is permitted which does not comply with these terms.
*Correspondence: Rosa María Martínez-Espinosa, cm9zYS5tYXJ0aW5lekB1YS5lcw==
Disclaimer: All claims expressed in this article are solely those of the authors and do not necessarily represent those of their affiliated organizations, or those of the publisher, the editors and the reviewers. Any product that may be evaluated in this article or claim that may be made by its manufacturer is not guaranteed or endorsed by the publisher.
Research integrity at Frontiers
Learn more about the work of our research integrity team to safeguard the quality of each article we publish.