- 1The Second Clinical Medical College, Shandong University of Traditional Chinese Medicine, Jinan, Shandong, China
- 2Department of Neurosciences, Affiliated Hospital of Jining Medical University, Jining, Shandong, China
- 3Cheeloo College of Medicine, Shandong University, Jinan, Shandon, China
- 4Clinical Medical College, Jining Medical University, Jining, Shandong, China
- 5Postdoctoral Mobile Station of Shandong University of Traditional Chinese Medicine, Jinan, Shandong, China
Disorders of the central nervous system (CNS) constitute a significant global health concern at the moment. Most CNS disorders are characterized by severe neuronal damage with excessive production of reactive oxygen species, which induces high levels of oxidative stress and intense inflammatory responses in the affected tissues, thus aggravating disease pathology. Notably, the blood–brain barrier makes it difficult to deliver many drugs and biologics to the CNS, which creates great difficulties in the diagnosis and treatment of CNS disorders. Recent research on polydopamine nanotechnology has led to the discovery of many promising properties; it shows strong scavenging ability for reactive oxygen species, prevents activation of pro-inflammatory microglia, and its repair function can reduce brain damage and protect neurons. Moreover, polydopamine nanotechnology can improve the blood–brain barrier permeability of biologics and reduce their neurotoxicity. It is therefore a promising candidate in the treatment of CNS disorders associated with oxidative stress. In the present paper, we review the functionality of polydopamine nanotechnology as well as the potential and recent advances of polydopamine-based nanosystems in the diagnosis and treatment of various CNS disorders, including Alzheimer’s disease, Parkinson’s disease, stroke, spinal cord injury, and glioma. Finally, we predict how polydopamine nanoparticles may guide future therapeutic strategies to address CNS disorders such as epilepsy, which currently have no cure.
1 Introduction
As populations age in many countries, the World Health Organization predicts a gradual increase in the number of cases of central nervous system (CNS) disorders (Waris et al., 2022), which are estimated to overtake cancer in 2040 to become the second most cause of mortality after cardiovascular disease (Gammon, 2014). CNS disorders can be categorized into two groups: (Waris et al., 2022): acute brain injuries, including stroke, cerebral ischemia, brain injury, and epilepsy; and (Gammon, 2014) chronic neurodegenerative disorders, including Alzheimer’s disease (AD), Parkinson’s disease (PD), and Huntington’s disease (Nguyen et al., 2021). These diseases lead to irreversible damage and loss of function, and severely affect patients’ quality of life and place a burden on families and society (Zhang W. et al., 2021). Unfortunately, most CNS disorders lack effective diagnostic and treatment approaches to delay or reverse the progression of the disease (Ying et al., 2023). There is therefore an urgent need to further explore and understand these disorders and find appropriate diagnostic and therapeutic approaches.
The production of polydopamine (PDA), an insoluble biopolymer with a dark brownish-black color, results from the autoxidation of its monomer, dopamine (DA) (Batul et al., 2017), a significant catechol neurotransmitter that plays important roles in the brain, including neurotransmission and hormone release control (Figure 1) (Brisch et al., 2014). Moreover, DA plays a role in the neurobiology, motor control, and symptoms of schizophrenia, PD, and attention deficit hyperactivity disorder (Marsden, 2006; Vocabulary, 2021). PDA is the main pigment of natural melanin (or true melanin) (Zheng et al., 2020). Thus, PDA exhibits many of the promising optical, electrical, and biomedical properties of natural melanin, including acting as an antioxidant and photoprotectant and providing immunity against pathogens.
PDA has garnered the interest of numerous scientific groups over the past few years, especially as a material that shows promise for creating multifunctional nanostructures. Nanomaterials have unique particle sizes (diameters less than 200 nm) and can target different molecules, surface charges, topologies, softness, and shapes. PDA-derived nanostructures reportedly have excellent properties for various biomedical applications (Chen et al., 2015; Wang et al., 2020). PDA nanoparticles (PDANPs) have gained much attention in recent years because of their particular physical and chemical properties, and especially their antioxidant activity. Notably, PDANPs have great scavenging capacity for reactive oxygen species (ROS), which combined with their fully organic nature and their ability to be degraded and excreted by living organisms makes them promising treatments for oxidative stress-associated CNS disorders (Figure 2) (Liu et al., 2014).
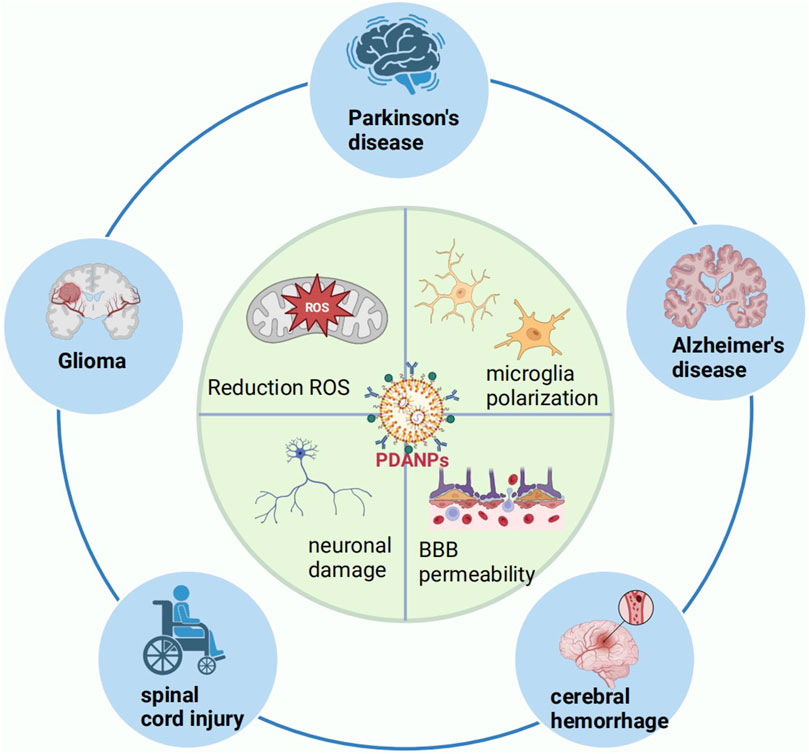
Figure 2. Schematic diagram of the use of PDANPs in the CNS. BBB, blood–brain barrier; CNS, central nervous system; PDANPs, polydopamine nanoparticles; ROS, reactive oxygen species.
2 Overview of PDA nanotechnology
2.1 Preparation of PDA
PDA is obtained via three common methods: enzymatic oxidation, electropolymerization, and solution oxidation. Of these methods, solution oxidation is currently the most generally used because of the simplicity of its polymerization process, which does not require complex instrumentation (Liu et al., 2014). In this method, the monomer DA of PDA oxidizes and undergoes self-polymerization in an alkaline environment (pH > 7.5) using dissolved oxygen in the solution as an oxidant. The transformation of a colorless solution in an alkaline environment to a brown color in the presence of oxygen indicates the conversion of DA to PDA. Another method to produce PDA is enzymatic oxidation (Kobayashi and Makino, 2009), while electropolymerization refers to the direct deposition of DA monomers as PDA on electrodes via electrochemical methods (Figure 3) (Tian et al., 2023). While PDA can be produced by polymerization under simple, mild conditions, the underlying mechanism remains unclear because of the complex redox reactions that are involved, in addition to the production of a series of intermediates.
2.2 Physicochemical properties of PDA
2.2.1 Adhesive and chemical properties
One important characteristic of PDA is the abundance of functional groups, including imines, amines, and catechols, in its chemical structure (Tran et al., 2019). PDA is extremely reactive due to these functional groups, and it may stick to the surface of a wide range of inorganic and organic materials, including metals, oxides, ceramics, semiconductors, and polymers (Li et al., 2024). Therefore, PDA can be utilized as a starting material for loading other proteins or noble metals, as well as for covalently modifying desired molecules, resulting in different hybrid materials and nanocomposites on both organic and inorganic substrates, including PDANPs, hollow capsules, core@shell nanocomposites, and various PDA coatings (Figure 4) (Madhurakkat Perikamana et al., 2015; Batul et al., 2017; Mrówczyński, 2018). The catechol structure of PDA can also bind to almost all kinds of transition metals and radioisotopes, which allows PDA to be used as an imaging contrast or for cancer radioisotope therapy (Mrówczyński, 2018). Furthermore, the phenolic-rich PDA can act as a free radical scavenger to remove ROS produced during inflammatory responses, and the rich aromatic ring structure can generate a large number of π-electrons; Adsorbed carriers are possible on the PDA’s surface through π–π stacking interactions (Zhao et al., 2018). PDANPs are therefore a promising biomedical nanomaterial (Cheng et al., 2019).
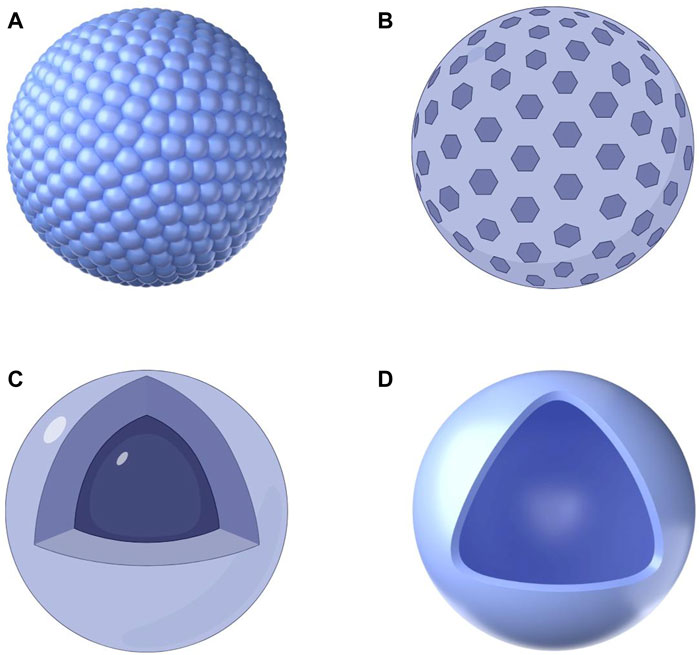
Figure 4. Schematic diagram of four common PDA-based nanoparticles. (A) PDA nanospheres; (B) Mesoporous PDA nanoparticles; (C) PDA-coated nanoparticles; (D) PDA nanocapsules.
2.2.2 Photothermal conversion capacity
Photothermal therapy is an emerging technology that has attracted much attention in recent years. Until now, a variety of photothermal conversion materials have been developed for photothermal therapy; their photothermal conversion efficiencies can be relatively high (Liu et al., 2019). As a near-infrared (NIR) light-responsive material, compared to certain other documented photothermal therapy species, PDA exhibits a significantly better photothermal conversion efficiency. It can effectively absorb NIR light energy and convert it into heat, making it an ideal photothermal therapeutic agent for tumor treatment and sterilization (Ding et al., 2020). Materials having absorptions in the NIR region (650–900 nm) are more appealing for therapeutically relevant uses in photothermal therapy because they provide deeper light penetration and exhibit comparatively low biological tissue scattering/absorption (Shanmugam et al., 2014).
2.2.3 Biocompatibility
Ensuring the excellent biocompatibility of materials is important for biomedical applications. Fortunately, PDA surface modification can effectively improve the biocompatibility of materials without affecting the viability of most mammalian cells. Guan et al. evaluated data from mice who were intravenously administered surface-modified PDA for 1 month and observed no significant organ inflammation or lesions; all blood markers were within normal limits (Gao et al., 2022). These findings indicate that PDA has good biocompatibility and low cytotoxicity.
2.2.4 Biodegradability
Drug-delivered materials need to be biodegraded in a timely manner while functioning in the body, otherwise, long-term retention can easily cause serious adverse reactions. Langer et al. reported that PDA is almost completely degraded at 8 weeks after entry into organisms (Bettinger et al., 2009). PDA nanoparticles lose UV absorption in the presence of hydrogen peroxide (H2O2) and are accompanied by color fading, whereas reduced nicotinamide adenine dinucleotide phosphate (NADPH) oxidase is widely present in phagocytes and in many organs and is capable of generating H2O2, an endogenous molecule, which suggests that PDA can be biodegraded in vivo (Liu et al., 2013; Cinato et al., 2024).
2.2.5 Other characteristics
The spectrogram of PDA has a broadband absorption, from ultraviolet to NIR regions, and has no distinct luminescent region; it thus exhibits weak fluorescence and strong fluorescence bursting ability (Cheng et al., 2019). In addition, PDA has properties such as paramagnetism, electrical conductivity, reducibility, and thermal stabilization. These capabilities increase its clinical utility (Hwang and Kim, 2014) and mean that PDA is not limited to being used as a coating material; it can be studied and applied in many different fields (Kwon and Bettinger, 2018).
3 Functions of PDANPs
3.1 PDANPs effectively scavenge ROS
ROS are essential to inflammation because they support the translation and release of cytokines, the polarization and activation of microglia, and the removal of injured tissue (von Leden et al., 2017). CNS disorders are often accompanied by ROS overproduction, which then induces oxidative stress, leading to an imbalance between oxygen radicals and antioxidant defense responses. This imbalance can induce cell death or initiate apoptosis, ultimately leading to severe structural damage and loss of function in neural tissues, which exacerbates disease-related pathological changes and causes neuroinflammation and neuronal loss (von Leden et al., 2017; An et al., 2020) (Figure 5). For example, during spinal cord injury (SCI), large amounts of ROS are generated at the injury site, thus leading to neuronal cell death, focal axonal degeneration, neuropathic pain, and motor impairment (Ham and Leipzig, 2018). For the treatment of CNS diseases and the subsequent recovery of neurological functions, it is advantageous to eliminate excess ROS generated during CNS lesions (Liu et al., 2020).
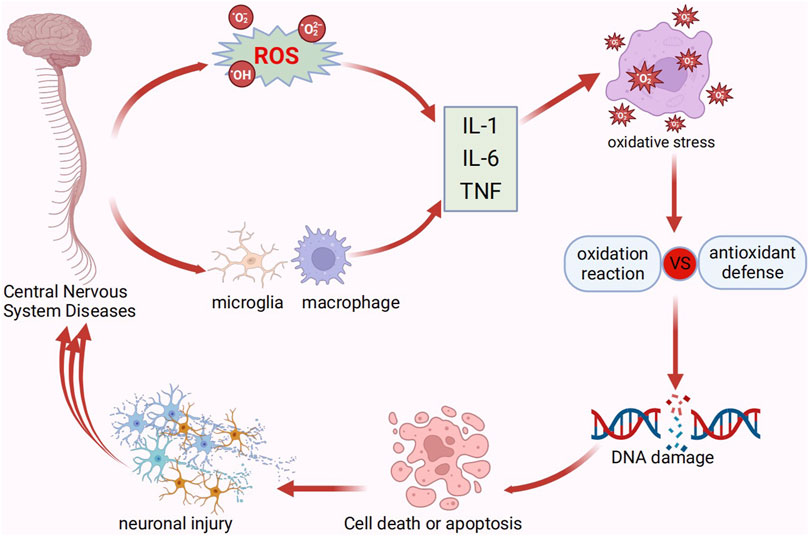
Figure 5. Pathological processes of central nervous system disorders. IL, interleukin; TNF, tumor necrosis factor.
Thanks to their large surface area effect, small size effect, and quantum size effect, PDA nanomaterials can immobilize natural enzymes or load antioxidant small molecules and deliver them to the target region. This can then reduce the secretion of pro-inflammatory cytokines and effectively adsorb the inflammatory chemotactic factors interleukin-8 and monocyte chemoattractant protein-1, which will decrease the migratory activity of neutrophils and macrophages to eliminate excess ROS and reduce oxidative stress (Zhao et al., 2018; An et al., 2020). In addition, PDA can reduce oxidative stress; it is rich in reducing functional groups consisting of catechols and imines, meaning that it is very good at scavenging various free radical substances and reducing ROS-induced inflammation (Jodko-Piórecka and Litwinienko, 2015; Bao et al., 2018). Recent research has demonstrated that ROS scavenging nanomaterials can be used as effective antioxidants or as therapeutic platforms for diseases of the central nervous system (Hu et al., 2017; Ballance et al., 2019).
3.2 PDANPs inhibit microglia and macrophage polarization
CNS trauma stimulates resident microglia and recruits peripheral monocytes to the damaged nervous system. Microglia are brain-residing macrophages that originate from primitive progenitor cells in the yolk sac (Ma et al., 2017). CNS macrophages, which are made up of activated microglia and blood-derived monocytes, adopt a functional phenotype that contributes to CNS repair and protection (Devanney et al., 2020). The M1 and M2 phenotypes are two opposing types of microglial activation in the CNS that are distinct from one another (Yang et al., 2018; Kwon and Koh, 2020). M1 microglia induce inflammation and neurotoxicity by releasing inflammatory mediators, and are predominant at the site of injury or in end-stage disease. In contrast, M2 microglia induce anti-inflammation and neuroprotection by releasing anti-inflammatory mediators, and are predominant in the immune resolution of disease and in the repair process (Tang and Le, 2016; Guo et al., 2022). The balancing of microglia M1/M2 polarization has great therapeutic promise for neurodegenerative diseases (Guo et al., 2022). During normal healing, classical pro-inflammatory M1 macrophages and alternatively activated M2a, M2b, and M2c macrophages are sequentially activated. This process helps the body go through the proliferative, remodeling, and inflammatory phases of repair (Gensel and Zhang, 2015). After ischemic stroke, activated microglia and peripherally infiltrating inflammatory cells in the brain lead to a complex and overactivated cerebral immune microenvironment as the result of compromised blood–brain barrier (BBB) integrity, which then produces neuronal death and significantly impedes neurological recovery (Jian et al., 2019). Furthermore, during the acute inflammatory phase of stroke, neutrophils and monocytes/macrophages may continuously migrate to ischemic regions of the brain and subsequently release ROS, thus exacerbating neuropathological damage (Tang et al., 2019).
Recent studies have reported that PDANPs can attenuate brain injury and protect neurons by preventing pro-inflammatory microglial activation or by affecting microglial phenotypic shifts, thus fully utilizing their reparative functions (Liu et al., 2021; Lou et al., 2021; Yan et al., 2023). Additionally, in AD, PDANPs can establish strong interactions with amyloid-β (Aβ) monomers/proto-fibers using their multiple recognition sites (e.g., catechol moiety, imine moiety, and indole/catechol π system) (Yin et al., 2023), which then alleviates Aβ-induced oxidative stress by effectively inhibiting Aβ aggregation, degrading Aβ protofibrils to alleviate neuroinflammation, and modulating the inflammatory microenvironment to promote microglial cell polarization toward an M2-like phenotype (Yan et al., 2021; Huang et al., 2023). Overall, the proposed nano-approach using PDA is a promising therapeutic strategy for CNS disorders.
3.3 PDANPs increase BBB penetration
The BBB is a specialized part of the vascular system (Kadry et al., 2020). Maintaining the exact regulation of CNS homeostasis, permitting appropriate neuronal function, and shielding neural tissue from toxins and pathogens all are dependent on its structural and functional integrity (Daneman and Prat, 2015). However, preventing the entry of toxins or pathogens into the CNS also markedly limits the brain uptake of many therapeutic and diagnostic compounds (Ceña and Játiva, 2018). The BBB frequently impedes the effective treatment of neurological disorders by preventing nearly all large-molecule medications and more than 98% of small-molecule medications from adequately penetrating the brain parenchyma (Zhao et al., 2023). While significant progress has been made in recent years, there are still major challenges in achieving controllable drug delivery to
The brain (Zhang et al., 2016; Tian et al., 2024). Thus, one of the most important challenges facing the treatment of CNS disorders is the accessibility of efficient brain-targeting technologies (Wong et al., 2012).
Research suggests that nanotechnology may be a useful tool for delivering therapeutic agents and diagnostic probes to the brain (Saeedi et al., 2019), because nanocarriers can be efficiently transported into various in vitro and in vivo BBB models via endocytosis and/or transcytosis. Furthermore, PDANPs have the potential to improve the therapeutic efficacy of many drugs by increasing their BBB permeability and reducing their neurotoxicity (Wong et al., 2012; Domínguez et al., 2014; Vashist et al., 2023). For example, in both in vivo and in vitro models of Parkinson’s disease, PDA can effectively penetrate the BBB to reach distal regions in the brain and be taken in by neuronal cells, and has good neuroprotective effects (Gao et al., 2022). Furthermore, nanoparticles reportedly enhance the neuroprotective effects of PDAs. For example, they can improve the targeting of effective therapeutic agents and their frequency of entry into the brain, and are even able to significantly improve memory and cognitive function in double transgenic amyloid precursor protein/presenilin 1 (APP/PS1) mice without any adverse effects (Wang Y. et al., 2022). In addition, because of the favorable photothermal effects of nanoscale PDA (Lu et al., 2021), it can contribute to effective BBB penetration under NIR irradiation (Wu D. et al., 2022). NIR irradiation with high spatial and temporal precision has been reported to produce focal interference, which enhances drug delivery into deep brain tissues in a non-invasive manner (Li et al., 2019). In summary, the use of PDANP approaches may effectively overcome the major drawbacks of conventional treatment.
3.4 PDANPs attenuate neuronal damage
Neurons are especially vulnerable to oxidative stress-induced injury because the brain’s high metabolic levels, which generate high levels of ROS and lead to neuronal loss of function and cell death, and because relatively low levels of antioxidant protective mechanisms are present at the neuronal level (Bélanger et al., 2011; Wojsiat et al., 2018).
PDANPs are fully organic, biocompatible, biodegradable, and antioxidant nanostructures that have very strong ROS scavenging abilities, thus acting as neuroprotective agents (Figure 6) (Liu et al., 2014; Martinelli et al., 2020). In addition, PDANPs can effectively mitigate cytotoxicity triggered by pathogenic substances, thus preventing cell membrane damage and cellular mechanical decline, inhibiting neuronal apoptosis, and exerting neuronal protection (Qin et al., 2022). Lipid-coated PDANPs have been investigated for their ability to counteract ROS-induced neuron-like cell damage and have the intrinsic ability to stimulate axon growth; they can also be used for the NIR-mediated fine-tuning of cell temperature and activity (Battaglini et al., 2020). Thus, PDANPs show strong superiority in the field of central nervous system (Table 1) and deserve further investigation.
4 PDANPs in CNS disorders
4.1 Applications in PD
PD is a common neurodegenerative disorder in older adults. It is primarily characterized by the loss of dopaminergic neurons in the substantia nigra and the localized deposition of cytoplasmic protofibrillar inclusions in the brain (as Lewy vesicles) (Li H. et al., 2021). Lewy vesicles are aggregates of α-synuclein phosphorylated on Ser 129 (Srivastava et al., 2020).
4.1.1 Applications in the treatment of PD
4.1.1.1 Neuroprotection through the inhibition of inflammation
PDA can reduce ROS production, inhibit cysteine-dependent and -non-dependent activation of apoptosis, and reduce the deposition of high-molecular-weight α-synuclein oligomers (Sardoiwala et al., 2020; Srivastava et al., 2020; Li H. et al., 2021). For example, PDANPs loaded with metformin reportedly modulate neuroprotective potential, reduce oxidative stress, prevent apoptosis, and show excellent biocompatibility for inhibiting α-synuclein phosphorylation (Sardoiwala et al., 2020). Another study prepared melatonin-enriched PDA nanostructures that exhibited efficient brain tissue retention, and prevented neuroblastoma cell death induced by both PD-associated stimuli and mitochondrial damage stimuli. Furthermore, this synergistic neuroprotective impact decreased the formation of cellular ROS, restored the potential of the mitochondrial membrane, and had anti-inflammatory properties (Srivastava et al., 2020). Wei Wang et al. co-synthesized PDA with selenocystine (SeCys) to prepare a nanocomposite (PDASeCys) with Glutathione peroxidase (GPx)-like activity. The results showed that the PDASeCys nanocomposite has the better free radical scavenging efficiency and protect neuronal cells from oxidative stress (Figure 7A) (Wang W. et al., 2022). Yumin Yang et al. develop a core-satellite-like nanoassembly (PDA-AFn (by integrating polydopamine nanoparticles and apoferritin)), not only elaborately regulate the iron homeostasis and redox microenvironment, but also utilize excessive reactive oxygen species (ROS) and iron ions in the damaged neurons to restore the function of the degenerated neurons (Figure 7B) (Yao et al., 2023).
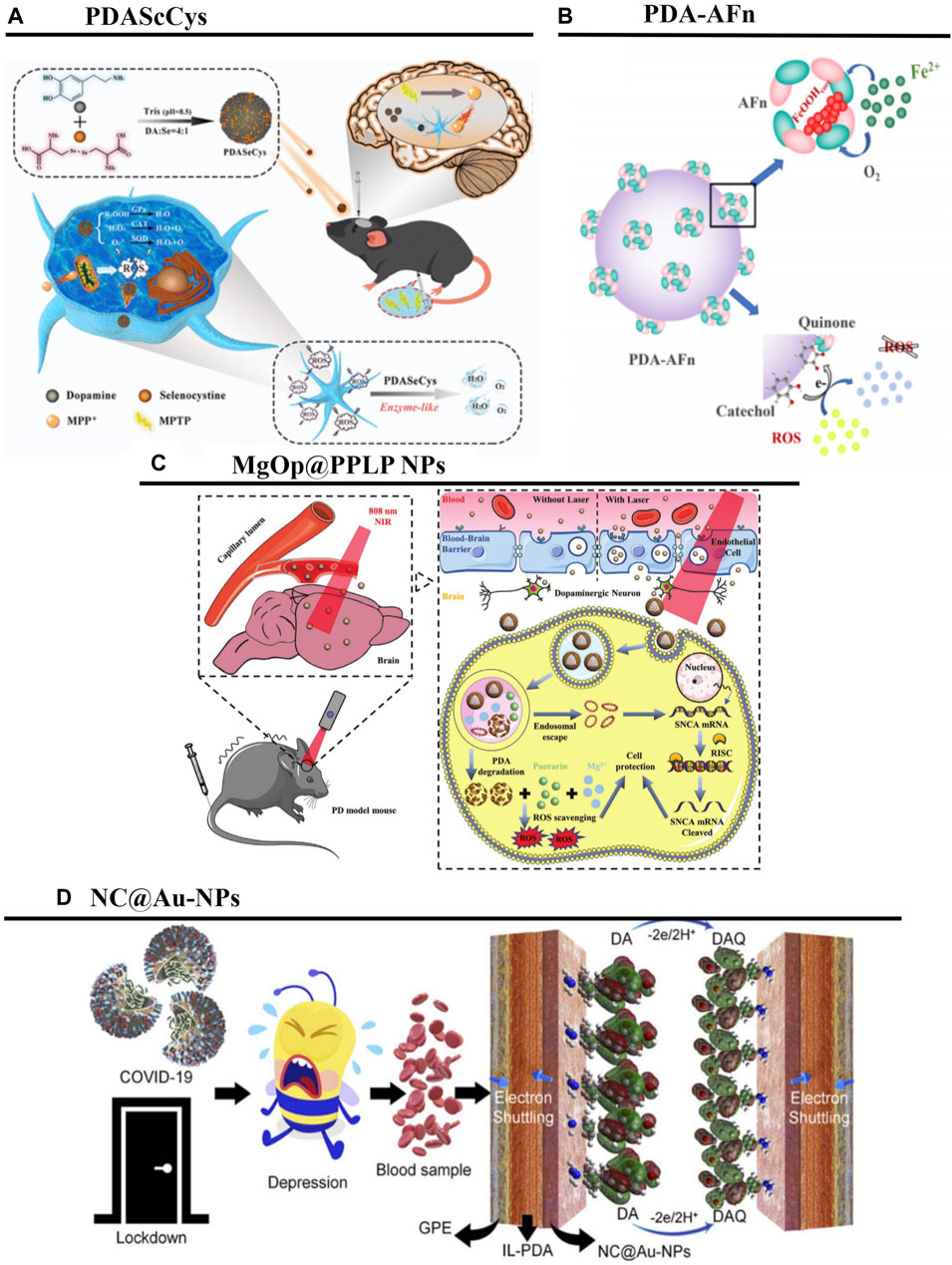
Figure 7. PDANPs’s applications in PD. (A) Schematic illustration of Polydopamine-Based Nanocomposite as a biomimetic antioxidant with a variety of enzymatic activities. Reproduced from Wei Wang et al. (Wang W. et al., 2022)Copyright 2022, ACS Appl Mater Interfaces. (B) Schematic of synthesis and main function of PDA-AFn. Reproduced from Ke Yao et al. (Yao et al., 2023)Copyright 2023, Nano Research. (C). Schematic illustration of the MgOp@PPLP NPs for the treatment of PD. Reproduced from Yifei Gao et al. (Gao et al., 2022)Copyright 2022, Adv Healthc Mater. (D) Schematic illustration showing the adsorption and oxidation of DA derived from the blood of COVID-19 quarantined depressed patients, at the surface of the designed NC@Au-NPs/PDA/IL electrode. Reproduced from Faria Shakeel et al. (Shakeel et al., 2022)Copyright 2022, RSC Advances.
4.1.1.2 Precision drug therapy through increased brain targeting
The BBB is a major limiting factor in PD treatment (Gao et al., 2022). A self-assembled poly (L-DOPA(OAc2))-based drug, NanoDOPA, has been developed for the treatment of MPTP-induced PD in a mouse model. The incorporation of L-DOPA into a self-assembled poly (L-DOPA(OAc)2) copolymer resulted in greater bioavailability of the drug in the bloodstream, improved pharmacokinetic values, led to more marked improvements of PD symptoms, and significantly inhibited levodopa-induced dyskinesia in mice (Ren et al., 2017). The results of this study are summarized in Table 1. Furthermore, no significant toxicity was observed in PDA nano-treated mice (Vong et al., 2020). These results suggest that self-assembled PDANPs are potential therapies for PD. Moreover, Guan et al. explored the hydrophilicity, brain targeting, and antioxidant properties of MgOp@PPLP nanoparticles, which had MgO nanoparticles as the substrate, PDA as the shell, were wrapped with anti-SNCA plasmid, and were surface-modified with molecules such as poly (ethylene glycol), lactoferrin, and geranylgeranyl. These authors reported that, when guided by the photothermal effect, these MgOp@PPLP particles exhibited excellent NIR radiation response, were able to penetrate the BBB and be taken up by neuronal cells (thus exerting gene therapy and antioxidant therapeutic effects), and showed good neuroprotective effects. The combination of biocompatible MgOp@PPLP nanoplatforms and non-invasive NIR radiation is therefore ideal for combatting neurodegenerative diseases (Figure 7C) (Gao et al., 2022).
4.1.2 Applications in the diagnosis of PD
PDANPs can be applied not only for PD treatment, but also for the high-precision monitoring of DA in patient blood or drug samples.
PD is mainly caused by disturbed DA levels; useful information regarding the treatment and prevention of PD can therefore be obtained via the precise and real-time monitoring of DA. It has been reported that, because of positively charged DA and negatively charged novel N-rich carbon-coated Au nanoparticles (NC@Au-NPs) exhibit a high electrostatic affinity, NC@AuNPs have rapid electro-oxidation efficacy on DA, thus providing a powerful tool for the diagnosis of PD (Figure 7D) (Shakeel et al., 2022). In addition, surface-enhanced Raman spectroscopy is a very promising technique for the sensitive detection of DA. To detect DA using label-free surface-enhanced Raman spectroscopic methods, reduction via citrates (c-AgNPs) must be used; stable AgNP-PDA material can be obtained using reduction via hydroxylamine (h-AgNPs) and 488 nm laser excitation (Badillo-Ramírez et al., 2021).
4.2 Applications in AD
Around 60%–70% of dementia cases worldwide are thought to be caused by AD, the most prevalent neurodegenerative disease (Leng and Edison, 2021). The two primary pathological features of AD are the accumulation of Amyloid-β (Aβ) plaques and hyperphosphorylated tau (p-tau) protein, which form neurofibrillary tangles (Lin et al., 2023). The complex pathogenesis leads to severe neuronal damage, which in turn exacerbates Aβ aggregation and promotes AD progression (Huang et al., 2023). Aβ is the most important pathological component of AD. The design and development of multifunctional agents capable of efficiently identifying and eliminating Aβ aggregates is a possible approach towards the treatment of AD.
4.2.1 Applications in diagnosing AD
PDANPs have excellent visible-light activity (Lu et al., 2022). For the quantitative detection of Aβ, a ZnO@PDA/Au nanocomposites-based ultrasensitive photoelectrochemical sensor has been constructed. In this sensing system, the PDA film is used both as a sensitizer for charge separation and for antibody conjugation; the localized surface plasmon resonance effect of the AuNPs further enhances the charge transfer efficiency and photoelectrochemical activity in visible light. Because of their stability, reproducibility, and good selectivity, these nanocomposites provide reliable signals for the early detection of AD (Figure 8A) (He et al., 2021). In addition, it has been reported that Aβ can be sensitively detected using silica nanoparticles as a carrier for the in situ growth of PDA and the further assembly of AuNPs and secondary antibody (Ab2), to generate a secondary antibody complex (SiO2@PDA-AuNPs-Ab2) (Xie et al., 2023). The core-shell nanocomposite CeONP-Res-PCM@ZIF-8/PDA/Apt, in which cerium nanoparticles (CeONPs), resveratrol (Res), and PCM (tetradecanol) are embedded into a zeolitic imidazolate framework (ZIF)-8/PDA matrix using an aqueous-based mild method, has also been demonstrated as a fluorescence sensing platform for Aβ detection and intracellular imaging (Figure 8B) (Yan et al., 2021).
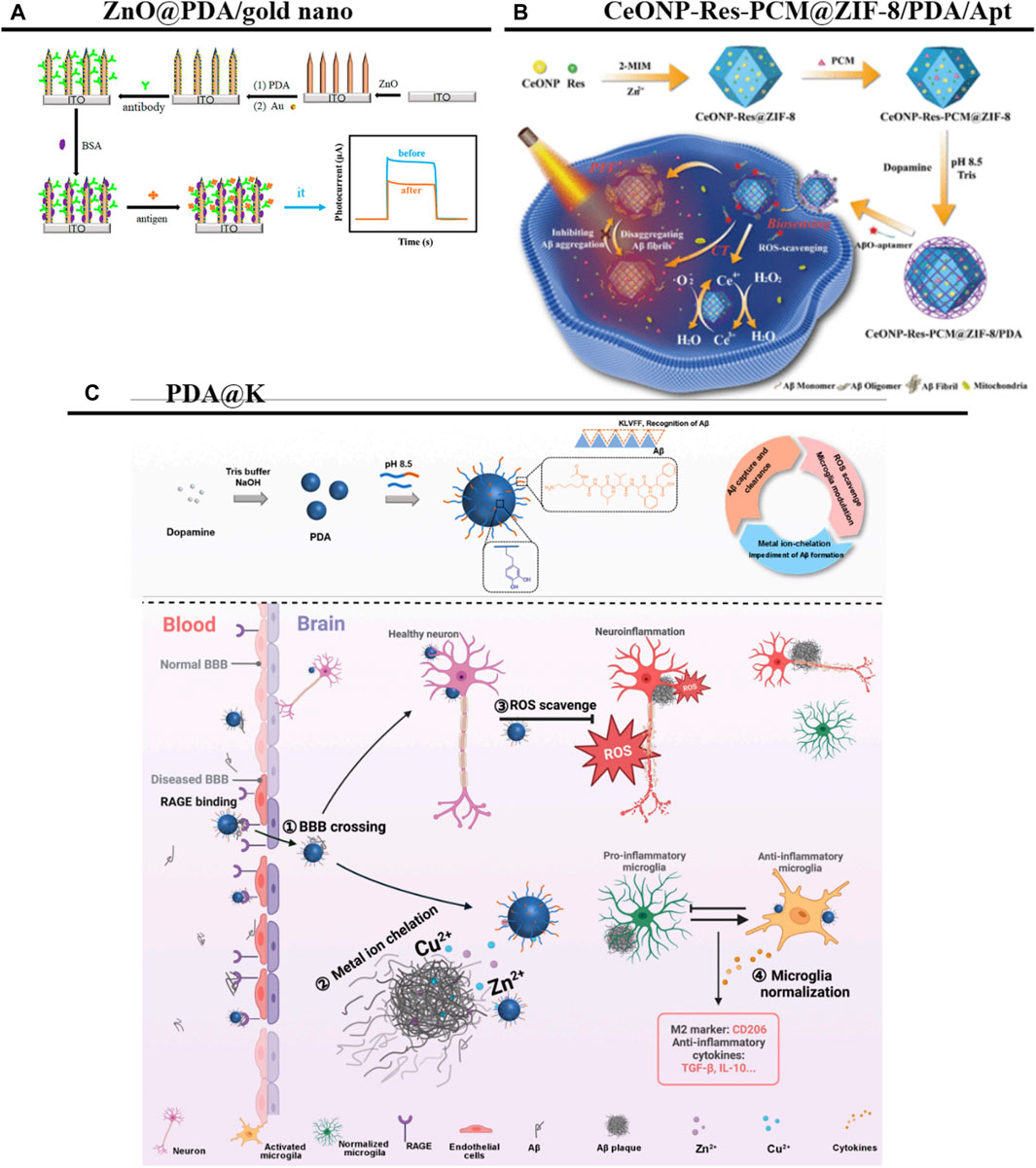
Figure 8. PDANPs’s applications in AD. (A) Schematic illustration of the stepwise fabrication process of the ZnO@PDA/Au PEC immunosensor. Reproduced from Guangli He et al. (He et al., 2021)Copyright 2021, Front Bioeng Biotechnol. (B) Schematic illustration of the ability of CeONP-Res-PCM@ZIF-8/PDA/Apt as the fluorescent sensing platform for AβO detection and intracellular imaging was demonstrated. Reproduced from Xueyan Yan et al. (Yan et al., 2021)Copyright 2021, Anal Chem. (C) Schematic diagram of the strategy. Reproduced from Qianqian Huang et al. (Huang et al., 2023)Copyright 2023, Research (Wash D C).
4.2.2 Applications in the treatment of AD
4.2.2.1 Neuroprotection through the reduction of Aβ aggregation
During AD onset, high concentrations of Aβ peptides in the CNS trigger microglial activation as well as an immune response against the Aβ aggregation. There is thus an urgent need for nanotechnology as a therapeutic strategy to reduce Aβ aggregation (Tiwari et al., 2019). To this end, one research group has constructed a multifunctional melanin-like metal ion chelator and neuroinflammatory modulator (named PDA@K) for the targeted therapy of AD. In their platform, PDANPs—with their strong metal ion chelating and ROS scavenging effects—are coated with Aβ pentapeptide fragment KLVFF peptides to provide a high affinity for Aβ. This elevated affinity enhances the crossing of the pathologic BBB via Aβ hitchhiking, and the treatment is reportedly able to alleviate Aβ aggregation at the lesion site and reduce neuroinflammation. In addition, modulating the inflammatory microenvironment promotes the polarization of microglia toward an M2-like phenotype, thus restoring their key functions of neuronal protection and plaque removal (Figure 8C) (Huang et al., 2023). Researchers have also designed aptamer-conjugated DA-coated gold (Au@PDA-Apt) NPs for targeting Aβ1–40 peptides. These Au@PDA-Apt NPs strongly inhibit the fibrillization of Aβ1–40 monomers and disassemble mature Aβ1–40 fibers, which effectively prevents Aβ1–40 aggregation-induced cell membrane damage (and the associated decrease in cellular mechanical properties), inhibits neuronal apoptosis, and exerts neuronal protective effects (Qin et al., 2022). These neuronal protective effects may occur via the inhibition of neuronal apoptosis (Martinelli et al., 2020). Furthermore, researchers recently developed a step-by-step metal-phenol coordination method and rationally designed a neuroprotective enhancer, K8@Fe-Rh/PDANPs, that can promote mitochondrial biogenesis and ultimately inhibit neuronal apoptosis by activating the sirtuin one/peroxisome proliferation-activated receptor gamma coactivator 1-alpha pathway. Using this method, rhubarbic acid and dopamine were able to be effectively coupled together to enhance their therapeutic effects on an AD mouse model, improving cognitive function in APP/PS1 mice without any adverse effects (Yin et al., 2023).
4.2.2.2 Therapeutic effects through Adjuvant mesenchymal stem cells (MSCs)
Transplantation of human umbilical cord-derived MSCs (hUC-MSCs) is a promising treatment for AD. However, in vitro cultured hUC-MSCs are prone to replicative senescence, which limits their application (Ma S. et al., 2022). Notably, the labeling of hUC-MSCs with superparamagnetic nanoparticles consisting of magnetic Fe3O4 and PDA shells (Fe3O4@PDA) to enhance the targeting of hUC-MSCs and their entry efficiency into the brain can improve memory and cognitive abilities in AD model mice by producing excess neuroprotective factors. The Fe3O4@PDA encapsulation of hUC-MSCs and its modulatory effects may thus be considered a viable therapy for AD (Wang Y. et al., 2022). Taken together, these studies suggest a new nano-platform for Aβ-targeted AD therapy.
4.3 Applications in SCI
SCI can result in permanent nerve damage and a loss of function at the site of injury and subsequent body parts, with severe consequences for the physical, economic, and psychosocial wellbeing of patients and their caregivers (Ahuja et al., 2017). Because of the complex pathomechanisms of SCI, there are not many effective therapeutic approaches for nerve regeneration and functional recovery (Shank et al., 2019).
4.3.1 Therapeutic effects through the sustained release of effective drugs
Drug delivery systems with high drug contents can minimize excipient usage, reduce side effects, improve efficacy, and/or promote patient compliance (Figure 9A) (Li W. et al., 2022). A mesoporous PDANP (mPDANP) that can be used for the combination therapy of SCI has been reported to inhibit ROS overproduction induced by aberrantly activated mammalian target rapamycin, and to simultaneously eliminate generated ROS. This mPDANP, known as mPDA@Rapa, is synthesized with a hydrophobic benzene ring structure and mesoporous cavities for the effective encapsulation and long-term release of rapamycin. Notably, mPDA@Rapa showed good therapeutic effects on SCI model rats, as evidenced by smaller injury cavities, more coordinated hindlimb movements, and increased neurogenesis and tissue regeneration (Shi H. et al., 2022). The results are summarized in Table 1.
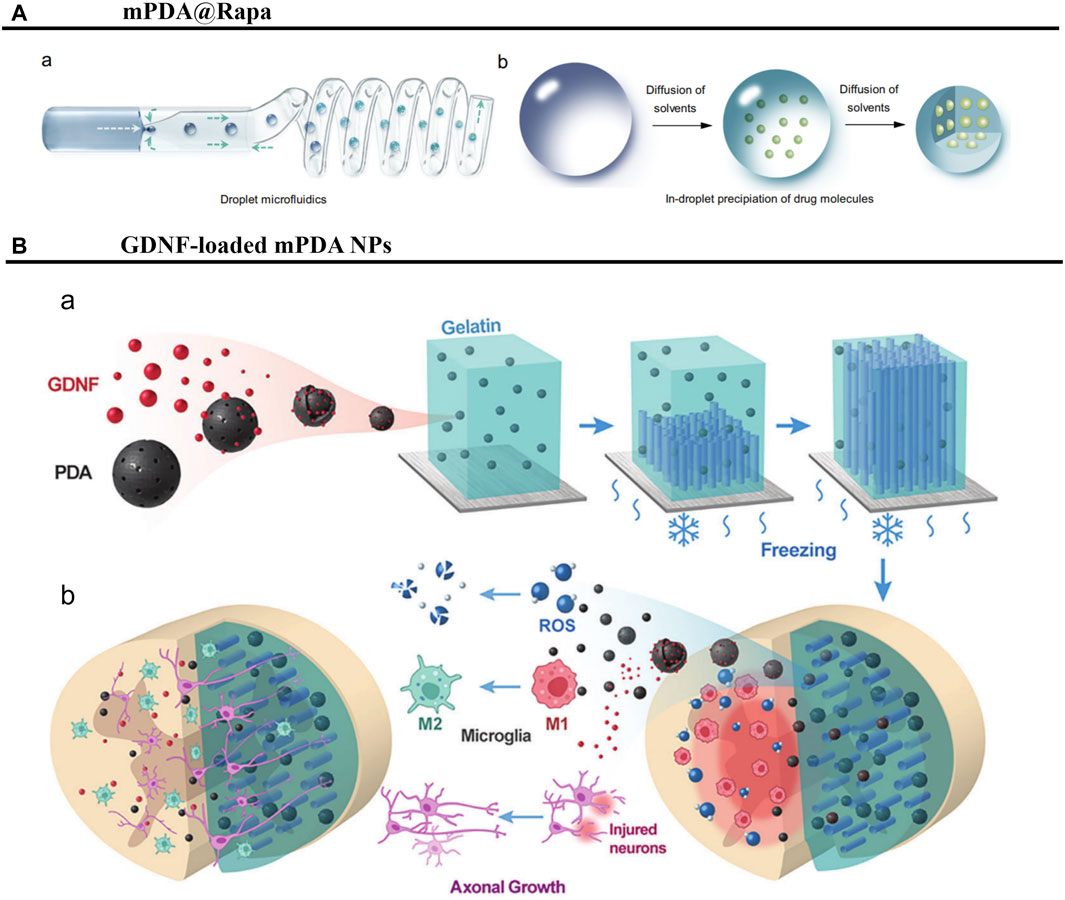
Figure 9. PDANPs’s applications in SCI. (A) Schematic illustration of preparation of nano-in-micro structured microspheres. (A) The preparation of high drug-loaded microspheres by droplet microfluidics. (B) The structural evolution of the droplets during solidification. Reproduced from Wei Li et al. (Li W. et al., 2022)Copyright 2022, Nat Commun. (B) Schematic illustration of study design. (A) Fabrication of GDNF-loaded PDA/Gelatin scaffold with liquid nitrogen freezing method. (B) Scaffolds were implanted at the mouse T9-10 spinal cord hemisection site. Reproduced from Jinjin Ma et al. (Ma et al., 2023)Copyright 2023, Adv Heaithc Mater.
4.3.2 Therapeutic effects for spinal cord repair
Mitochondrial function is significantly impaired after SCI, leading to neuronal apoptosis and impeding functional recovery. Repair of SCI thus remains a formidable clinical challenge that requires innovative therapies (Li Y. et al., 2022). In one study, anisotropic scaffolds based on glial cell-derived neurotrophic factor (GDNF)-loaded PDANPs were developed for spinal cord repair. The mPDANPs in the scaffolds were able to effectively scavenge ROS and promote microglia M2 polarization, thereby inhibiting the inflammatory response at the injury site and providing a favorable microenvironment for neuronal survival. In addition, GDNF encapsulated in mPDANPs was able to promote the regeneration of motor axons in corticospinal tracts and the recovery of their motor functions (Figure 9B) (Ma et al., 2023). In summary, PDANPs have potential applications in SCI and are expected to be an effective material for treating these injuries.
4.4 Applications in stroke
Ischemic stroke is a lethal cerebral disease that currently threatens human health and survival, with high morbidity and mortality. A multitude of cell types and biological pathways are involved in the complex but crucial pathogenic process known as neuroinflammation following an ischemic stroke (Iadecola and Anrather, 2011). The main goal of most interventions involves achieving reperfusion to salvage damaged neurons in the ischemic penumbra (Shi et al., 2018; Phipps and Cronin, 2020). However, although studies have suggested that some neuroprotective drugs can attenuate oxidative stress and inflammatory responses in stroke patients, the application of nanotechnology is greatly restricted in the treatment of central nervous system disorders due to physiological CNS barriers (Ruan et al., 2023), no single antioxidant has been reliably clinically validated and confirmed to be safe and effective for protecting the ischemic brain from oxidative damage (Li C. et al., 2021). Initial stages of oxidative stress can be mitigated by the nanosystem’s tailored mesostructure and inherent ability to scavenge free radicals (Wu et al., 2023). The investigation of safe and effective therapies to improve drug bioavailability and reduce toxic side effects is therefore of great importance.
4.4.1 Combined applications with MSCs for the treatment of stroke
MSCs could potentially be able to ameliorate cerebral infarction and decrease its consequences. Furthermore, the amount of functional MSCs transplanted and the ability of MSCs to target cerebral lesions are typically taken into account when determining the therapeutic efficacy of MSCs (Yun et al., 2018). Strategies that enhance MSC targeting may therefore improve therapeutic efficacy.
Magnetic navigation can increase the localization of MSCs to brain lesion sites and reduce lesion volume (Reeves and Weaver, 2014). It has been reported that iron oxide@PDA-labeled MSCs can significantly inhibit the polarization of M1 microglia, thus attenuating brain damage and protecting neurons by blocking pro-inflammatory microglial activation. This iron oxide@PDA-labeled MSC approach reduce the toxicity of the nanoparticles and greatly improve their superparamagnetism (physiological stability and biocompatibility of Fe3O4 with the nuclei) (Ge et al., 2016), overcomes the major drawbacks of conventional MSC therapies for the treatment of cerebral infarction, and thus represents a potential new avenue for cerebral infarction therapy (Yan et al., 2023).
It has been reported that a versatile immunosuppressive nanoparticle (VIN) with an engineering CXCL12 biomimetic decoy incorporated can control an overactive brain immune environment. Among other effects, the shell of VIN (a CXCR4-overexpressing MSC membrane) enhances nanoparticle homing to cerebral ischemic foci, and the loaded A151 (a cyclic GMP-AMP synthase [cGAS] inhibitor, telomerase repeat sequence) inhibits the cGAS–STING pathway in microglia, thus leading to the polarization of microglia to an M2-like phenotype. Importantly, A151 can be efficiently loaded onto PDA nanospheres (the core of VIN) via Zn2+ bridging (Zandieh and Liu, 2020). At the site of inflammation, PDA can then be oxidized by ROS, and the Zn2+ complex disappears, allowing the controlled release of A151. VIN integrates inflammatory eosinophilia, peripheral inflammatory cell filtration, the brain-resident activation of microglia polarization, and ROS clearance. Furthermore, it has a very good therapeutic effect, improving mortality, reducing infarct volume, and preserving neuronal neurogenic function (Figure 10A) (Shi J. et al., 2022).
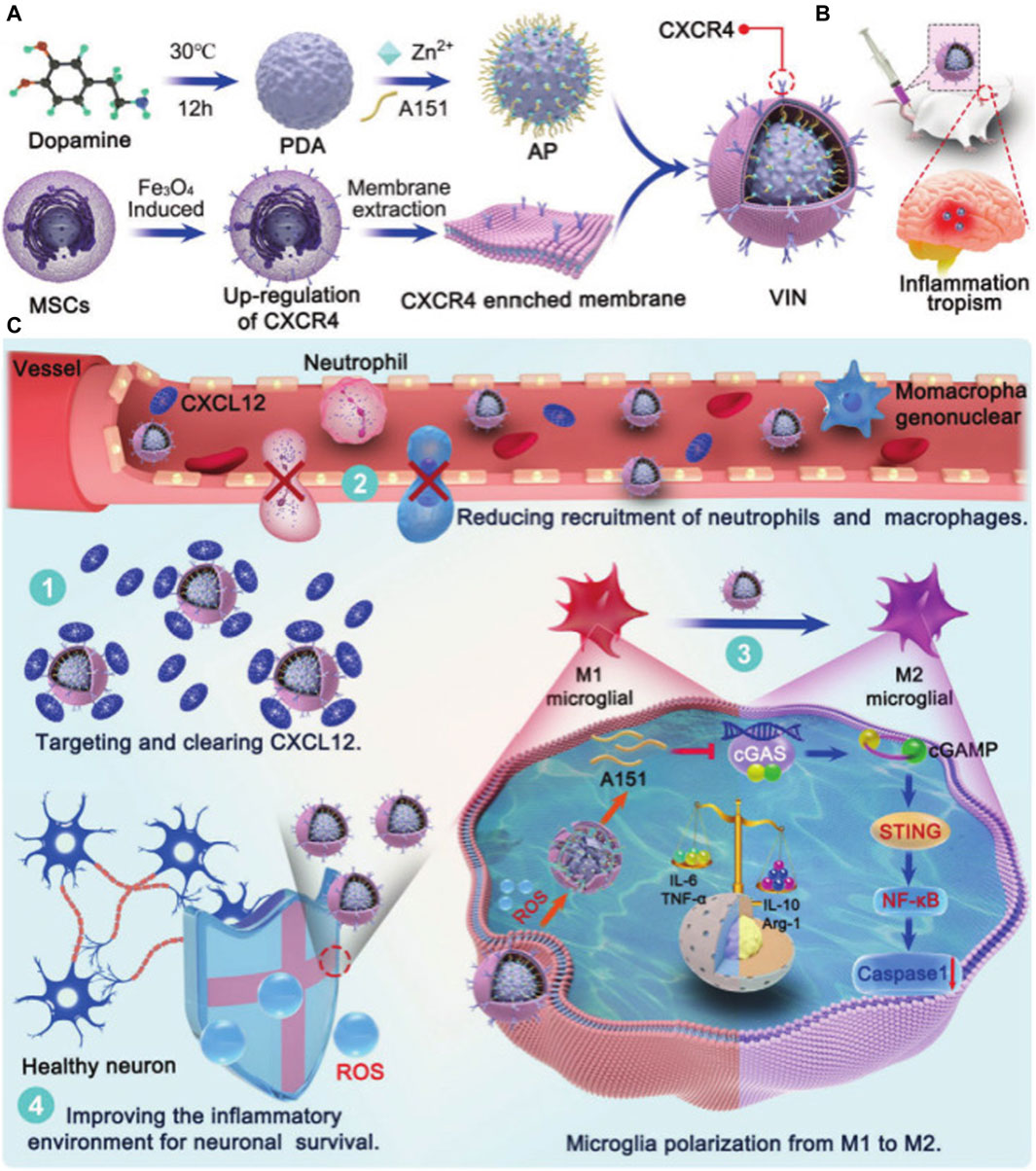
Figure 10. PDANPs’s applications in Stroke. Schematic illustration of engineering CXCL12 biomimetic decoy-integrated versatile immunosuppressive nanoparticle for ischemic stroke therapy with management of overactivated brain immune microenvironment. (A) Schematic illustration of VIN formation. (B) CXCR4-enriched VIN exhibited inflammation tropism to ischemic cerebrum after intravenous injection. (C) 1) The membrane vesicles overexpressed CXCR4 served as a “nanodecoy” for inflammatory driving factor (CXCL12) deletion. 2) The VIN efficiently cut off CXCR4-CXCL12 axis, reducing the infiltration of neutrophils and mononuclear macrophages. 3) A151 released in response to ROS, could inhibit the cGAS-STING pathway in microglia, leading to microglia polarization toward an anti-inflammatory M2-like phenotype. 4) VIN exhibited effective ROS scavenging in inflammatory microenvironment and protected neuronal cells from free radical-induced apoptosis. Reproduced from Jinjin Shi et al. (Shi J. et al., 2022)Copyright 2023, Small Methods.
PDANPs can make man-made melanin nanoparticles, which have strong antioxidant activity. These melanin nanoparticles can enhance the neuroprotective effects of MSCs against hypoxic–ischemic injury by inhibiting apoptosis and improving antioxidant defense. This evidence supports the combined application of melanin nanoparticles and MSCs in the treatment of ischemic stroke (Tang et al., 2022).
4.4.2 Improving the Safety of stenting
Stent placement is important for the prevention and treatment of ischemic stroke; however, its associated complications limit its use (Shi et al., 2023). One study has reported a biofunctional stent that effectively prolongs the release period of paclitaxel by encapsulating mesoporous silica nanoparticles loaded with paclitaxel in electrospun polylactic acid fibers. In addition, vascular endothelial growth factor was attached to the membrane surface by reacting with PDA for rapid release. This approach enhances the biosafety of covered stent placement for stroke treatment by promoting stent endothelialization at an early stage and inhibiting stenosis caused by smooth muscle proliferation in the long term (Zhang et al., 2019).
4.5 Applications in gliomas
Neurogliomas are the most prevalent primary malignant tumors in the CNS, and patients have a very poor prognosis (Ostrom et al., 2022). However, despite the fact that treating gliomas is still a major challenge for scientists and medical professionals, quick advancements in nanomedicine present promising prospects for long-term glioma treatment (Li T. et al., 2022).
4.5.1 Combination of radiotherapy and the delivery of chemotherapeutic agents
PDANPs offer unique diagnostic and therapeutic integration advantages in the field of combination therapy because they are able to simultaneously load metal ion contrast agents, chemotherapeutic drugs, photothermal agents, or photosensitizers on the surface, which are then combined with the photothermal conversion ability of PDAs (Dong et al., 2016). Not only can this approach improve drug penetration and delivery rates, but it can also reduce edge effects and resistance to radiation therapy, increase control in environments with different pHs, and enhance anti-tumor activity (Wu H. et al., 2022).
Radiotherapy and chemotherapy are the standard protocols for glioma treatment; however, gliomas tend to be resistant to radiotherapy and chemotherapy (Sanai and Berger, 2018). This problem was addressed by a research team who created a pifthrin-µ-loaded PDA-AuNP composite platform to combine photothermal and radiotherapy treatments, and simultaneously upregulate the pro-apoptotic unfolded protein response of gliomas after treatment, which significantly improved treatment efficacy. The AuNPs at the core of the nanoplatforms can also be used for computed tomography imaging, while the PDA layer on its surface can be used for magnetic resonance T1-weighted imaging. Thus, after tail vein injection of the nanoplatforms, computed tomography/magnetic resonance dual-modality imaging of tumors was able to guide radiotherapy and photothermal therapy, thus achieving diagnostic and therapeutic integration (Zhu et al., 2020).
Chemotherapy is the first-line treatment for brain tumors, but its efficacy is hampered by low drug targeting in the brain and tumor hypoxia-related drug resistance (Tan et al., 2020). Chemotherapeutic drugs can be loaded into PDA-based nanoparticles to precisely target tumor tissues and combine with the photothermal effect of PDA, thus improving the effectiveness and reduced toxicity of chemotherapy for cancer cells, thereby improving patient survival and prognosis (Zhao et al., 2020; Cao et al., 2021; Pu et al., 2021; Ma X. et al., 2022).
4.5.2 Improvement of effective drug uptake rate
The BBB can prevent drug and imaging agent access to the brain, and is thus an important obstacle to both the diagnosis and treatment of gliomas (Furtado et al., 2018). It has been reported that curcumin-loaded hemolysin (CUR-ZpD-G23) nanoparticles (NPs) functionalized with decameric peptide (G23) may penetrate through the blood-brain barrier and target glioma cells. Compared with free curcumin, the NPs enhanced curcumin uptake by glioma cells and had strong penetration into three-dimensional tumor spheroids. Moreover, functionalization of the NPs by G23 facilitated BBB penetration and the infiltration of tumor spheroids. Notably, these NPs have also been reported to significantly inhibit proliferation and engraftment and induce cell death (Zhang H. et al., 2021).
5 Opportunities and future prospectives
The characteristics of PDANPs determine their functions and roles, with unique advantages, and are currently a hot research topic in various fields worldwide. Although the important potential therapeutic role of PDANPs in the CNS has been confirmed by many, the underlying mechanisms remain unclear and require more practical and theoretical support. Moreover, the potential value of PDANPs remains to be further researched and explored in CNS diseases, which are dominated by inflammatory mechanisms. Although there has been some evidence that polydopamine nanometers can cross or bypass the blood-brain barrier, and some methods have been explored, due to the lack of preclinical models that can faithfully replicate the intricate structure of the human brain poses constraints on drug development and validation, resulting in only a limited number of successful preclinical studies, the scarcity of human clinical studies highlights the challenges of translation, resulting in significant progress in research but only in the nascent stage, which brings great challenges to the diagnosis and treatment of central nervous system diseases in the future.
Epilepsy is a chronic CNS disease in which brain dysfunction is caused by abnormal neuronal discharges. There are currently nearly 10 million people living with epilepsy in China, and long-term medication is the main treatment method. Unfortunately, despite great advances in medical science, existing therapeutic measures are often unable to achieve the expected results because of their inability to effectively cross the BBB. Furthermore, many therapies cause oxidative damage to endothelial cells, thus leading to a large amount of neuronal damage, and about 30% of patients develop drug-resistant epilepsy. PDANPs may be useful for overcoming these therapeutic deficiencies, as well as for diagnosing or controlling seizures, thus opening the door for research into epilepsy-related treatments.
A nano-engineered drug delivery system using polypyrrole-PDA nanomaterials for synergistic brain-targeted delivery and the on-demand release of antiepileptic drugs has been previously reported. The incorporation of PDA enables this drug delivery system to have relatively high conductivity and sensitivity, and synergistic targeting can be used with active peptide targeting and NIR photostimulation. When a seizure occurs, the nano-delivery system rapidly releases the drug within 30 s to increase the local drug concentration; it continuously responds to epileptic electrical signals to inhibit persistent seizures, and thus provides new ideas for the design of epileptic drug delivery systems. Of note, the application of PDA nanotechnology is also expected to reduce seizure severity by decreasing neuronal damage via the reduction of excessive ROS production and microglial polarization. In addition, effective drug release may be increased by optimizing the targeting mechanism to increase BBB permeability. Together with its satisfactory neuro-compatibility and biosafety, PDA nanotechnology may therefore become a safe and effective strategy to improve the effective therapeutic index of epilepsy.
Author contributions
SR: Writing–original draft. XX: Writing–review and editing. JL: Writing–review and editing. SL: Writing–review and editing. XW: Writing–review and editing. RL: Writing–review and editing. Q-xK: Writing–review and editing.
Funding
The author(s) declare that financial support was received for the research, authorship, and/or publication of this article. This article was supported by the Key Research and Development Plan of Jining City (grant no. 2023YXNS227), The present study was supported by the Key Research and Development Plan of Jining City (grant no. 2023YXNS061).
Conflict of interest
The authors declare that the research was conducted in the absence of any commercial or financial relationships that could be construed as a potential conflict of interest.
Publisher’s note
All claims expressed in this article are solely those of the authors and do not necessarily represent those of their affiliated organizations, or those of the publisher, the editors and the reviewers. Any product that may be evaluated in this article, or claim that may be made by its manufacturer, is not guaranteed or endorsed by the publisher.
References
Ahuja, C. S., Nori, S., Tetreault, L., Wilson, J., Kwon, B., Harrop, J., et al. (2017). Traumatic spinal cord injury-repair and regeneration. Neurosurgery 80 (3s), S9–s22. doi:10.1093/neuros/nyw080
An, Z., Yan, J., Zhang, Y., and Pei, R. (2020). Applications of nanomaterials for scavenging reactive oxygen species in the treatment of central nervous system diseases. J. Mater Chem. B 8 (38), 8748–8767. doi:10.1039/d0tb01380c
Badillo-Ramírez, I., Saniger, J. M., Popp, J., and Cialla-May, D. (2021). SERS characterization of dopamine and in situ dopamine polymerization on silver nanoparticles. Phys. Chem. Chem. Phys. 23 (21), 12158–12170. doi:10.1039/d1cp00966d
Ballance, W. C., Qin, E. C., Chung, H. J., Gillette, M. U., and Kong, H. (2019). Reactive oxygen species-responsive drug delivery systems for the treatment of neurodegenerative diseases. Biomaterials 217, 119292. doi:10.1016/j.biomaterials.2019.119292
Bao, X., Zhao, J., Sun, J., Hu, M., and Yang, X. (2018). Polydopamine nanoparticles as efficient scavengers for reactive oxygen species in periodontal disease. ACS Nano 12 (9), 8882–8892. doi:10.1021/acsnano.8b04022
Battaglini, M., Marino, A., Carmignani, A., Tapeinos, C., Cauda, V., Ancona, A., et al. (2020). Polydopamine nanoparticles as an organic and biodegradable multitasking tool for neuroprotection and remote neuronal stimulation. ACS Appl. Mater Interfaces 12 (32), 35782–35798. doi:10.1021/acsami.0c05497
Batul, R., Tamanna, T., Khaliq, A., and Yu, A. (2017). Recent progress in the biomedical applications of polydopamine nanostructures. Biomater. Sci. 5 (7), 1204–1229. doi:10.1039/c7bm00187h
Bélanger, M., Allaman, I., and Magistretti, P. J. (2011). Brain energy metabolism: focus on astrocyte-neuron metabolic cooperation. Cell Metab. 14 (6), 724–738. doi:10.1016/j.cmet.2011.08.016
Bettinger, C. J., Bruggeman, J. P., Misra, A., Borenstein, J. T., and Langer, R. (2009). Biocompatibility of biodegradable semiconducting melanin films for nerve tissue engineering. Biomaterials 30 (17), 3050–3057. doi:10.1016/j.biomaterials.2009.02.018
Brisch, R., Saniotis, A., Wolf, R., Bielau, H., Bernstein, H. G., Steiner, J., et al. (2014). The role of dopamine in schizophrenia from a neurobiological and evolutionary perspective: old fashioned, but still in vogue. Front. Psychiatry 5, 47. doi:10.3389/fpsyt.2014.00047
Cao, H., Jiang, B., Yang, Y., Zhao, M., Sun, N., Xia, J., et al. (2021). Cell membrane covered polydopamine nanoparticles with two-photon absorption for precise photothermal therapy of cancer. J. Colloid Interface Sci. 604, 596–603. doi:10.1016/j.jcis.2021.07.004
Ceña, V., and Játiva, P. (2018). Nanoparticle crossing of blood-brain barrier: a road to new therapeutic approaches to central nervous system diseases. Nanomedicine (Lond). 13 (13), 1513–1516. doi:10.2217/nnm-2018-0139
Chen, X., Huang, Y., Yang, G., Li, J., Wang, T., Schulz, O. H., et al. (2015). Polydopamine integrated nanomaterials and their biomedical applications. Curr. Pharm. Des. 21 (29), 4262–4275. doi:10.2174/1381612821666150901103418
Cheng, W., Zeng, X., Chen, H., Li, Z., Zeng, W., Mei, L., et al. (2019). Versatile polydopamine platforms: synthesis and promising applications for surface modification and advanced nanomedicine. ACS Nano 13 (8), 8537–8565. doi:10.1021/acsnano.9b04436
Cinato, M., Andersson, L., Miljanovic, A., Laudette, M., Kunduzova, O., Borén, J., et al. (2024). Role of perilipins in oxidative stress-implications for cardiovascular disease. Antioxidants (Basel) 13 (2), 209. doi:10.3390/antiox13020209
Daneman, R., and Prat, A. (2015). The blood-brain barrier. Cold Spring Harb. Perspect. Biol. 7 (1), a020412. doi:10.1101/cshperspect.a020412
Devanney, N. A., Stewart, A. N., and Gensel, J. C. (2020). Microglia and macrophage metabolism in CNS injury and disease: the role of immunometabolism in neurodegeneration and neurotrauma. Exp. Neurol. 329, 113310. doi:10.1016/j.expneurol.2020.113310
Ding, F., Gao, X., Huang, X., Ge, H., Xie, M., Qian, J., et al. (2020). Polydopamine-coated nucleic acid nanogel for siRNA-mediated low-temperature photothermal therapy. Biomaterials 245, 119976. doi:10.1016/j.biomaterials.2020.119976
Domínguez, A., Suárez-Merino, B., and Goñi-de-Cerio, F. (2014). Nanoparticles and blood-brain barrier: the key to central nervous system diseases. J. Nanosci. Nanotechnol. 14 (1), 766–779. doi:10.1166/jnn.2014.9119
Dong, Z., Gong, H., Gao, M., Zhu, W., Sun, X., Feng, L., et al. (2016). Polydopamine nanoparticles as a versatile molecular loading platform to enable imaging-guided cancer combination therapy. Theranostics 6 (7), 1031–1042. doi:10.7150/thno.14431
Furtado, D., Björnmalm, M., Ayton, S., Bush, A. I., Kempe, K., and Caruso, F. (2018). Overcoming the blood-brain barrier: the role of nanomaterials in treating neurological diseases. Adv. Mater 30 (46), e1801362. doi:10.1002/adma.201801362
Gammon, K. (2014). Neurodegenerative disease: brain windfall. Nature 515 (7526), 299–300. doi:10.1038/nj7526-299a
Gao, Y., Cheng, Y., Chen, J., Lin, D., Liu, C., Zhang, L. K., et al. (2022). NIR-assisted MgO-based polydopamine nanoparticles for targeted treatment of Parkinson's disease through the blood-brain barrier. Adv. Healthc. Mater 11 (23), e2201655. doi:10.1002/adhm.202201655
Ge, R., Li, X., Lin, M., Wang, D., Li, S., Liu, S., et al. (2016). Fe3O4@polydopamine composite theranostic superparticles employing preassembled Fe3O4 nanoparticles as the core. ACS Appl. Mater Interfaces 8 (35), 22942–22952. doi:10.1021/acsami.6b07997
Gensel, J. C., and Zhang, B. (2015). Macrophage activation and its role in repair and pathology after spinal cord injury. Brain Res. 1619, 1–11. doi:10.1016/j.brainres.2014.12.045
Guo, S., Wang, H., and Yin, Y. (2022). Microglia polarization from M1 to M2 in neurodegenerative diseases. Front. Aging Neurosci. 14, 815347. doi:10.3389/fnagi.2022.815347
Ham, T. R., and Leipzig, N. D. (2018). Biomaterial strategies for limiting the impact of secondary events following spinal cord injury. Biomed. Mater 13 (2), 024105. doi:10.1088/1748-605x/aa9bbb
He, G., Zhou, Y., Li, M., Guo, Y., Yin, H., Yang, B., et al. (2021). Bioinspired synthesis of ZnO@polydopamine/Au for label-free photoelectrochemical immunoassay of amyloid-β protein. Front. Bioeng. Biotechnol. 9, 777344. doi:10.3389/fbioe.2021.777344
Hu, M., Korschelt, K., Daniel, P., Landfester, K., Tremel, W., and Bannwarth, M. B. (2017). Fibrous nanozyme dressings with catalase-like activity for H(2)O(2) reduction to promote wound healing. ACS Appl. Mater Interfaces 9 (43), 38024–38031. doi:10.1021/acsami.7b12212
Huang, Q., Jiang, C., Xia, X., Wang, Y., Yan, C., Wang, X., et al. (2023). Pathological BBB crossing melanin-like nanoparticles as metal-ion chelators and neuroinflammation regulators against alzheimer's disease. Res. (Wash D C) 6, 0180. doi:10.34133/research.0180
Hwang, S. R., and Kim, K. (2014). Nano-enabled delivery systems across the blood-brain barrier. Arch. Pharm. Res. 37 (1), 24–30. doi:10.1007/s12272-013-0272-6
Iadecola, C., and Anrather, J. (2011). The immunology of stroke: from mechanisms to translation. Nat. Med. 17 (7), 796–808. doi:10.1038/nm.2399
Jian, Z., Liu, R., Zhu, X., Smerin, D., Zhong, Y., Gu, L., et al. (2019). The involvement and therapy target of immune cells after ischemic stroke. Front. Immunol. 10, 2167. doi:10.3389/fimmu.2019.02167
Jodko-Piórecka, K., and Litwinienko, G. (2015). Antioxidant activity of dopamine and L-DOPA in lipid micelles and their cooperation with an analogue of α-tocopherol. Free Radic. Biol. Med. 83, 1–11. doi:10.1016/j.freeradbiomed.2015.02.006
Kadry, H., Noorani, B., and Cucullo, L. (2020). A blood-brain barrier overview on structure, function, impairment, and biomarkers of integrity. Fluids Barriers CNS 17 (1), 69. doi:10.1186/s12987-020-00230-3
Kobayashi, S., and Makino, A. (2009). Enzymatic polymer synthesis: an opportunity for green polymer chemistry. Chem. Rev. 109 (11), 5288–5353. doi:10.1021/cr900165z
Kwon, H. S., and Koh, S. H. (2020). Neuroinflammation in neurodegenerative disorders: the roles of microglia and astrocytes. Transl. Neurodegener. 9 (1), 42. doi:10.1186/s40035-020-00221-2
Kwon, I. S., and Bettinger, C. J. (2018). Polydopamine nanostructures as biomaterials for medical applications. J. Mater Chem. B 6 (43), 6895–6903. doi:10.1039/c8tb02310g
Leng, F., and Edison, P. (2021). Neuroinflammation and microglial activation in Alzheimer disease: where do we go from here? Nat. Rev. Neurol. 17 (3), 157–172. doi:10.1038/s41582-020-00435-y
Li, C., Zhao, Z., Luo, Y., Ning, T., Liu, P., Chen, Q., et al. (2021b). Macrophage-disguised manganese dioxide nanoparticles for neuroprotection by reducing oxidative stress and modulating inflammatory microenvironment in acute ischemic stroke. Adv. Sci. (Weinh) 8 (20), e2101526. doi:10.1002/advs.202101526
Li, H., Yin, D., Li, W., Tang, Q., Zou, L., and Peng, Q. (2021a). Polydopamine-based nanomaterials and their potentials in advanced drug delivery and therapy. Colloids Surf. B Biointerfaces 199, 111502. doi:10.1016/j.colsurfb.2020.111502
Li, J., Duan, H., and Pu, K. (2019). Nanotransducers for near-infrared photoregulation in biomedicine. Adv. Mater 31 (33), e1901607. doi:10.1002/adma.201901607
Li, J., Lv, Y., Chen, Z., Zhao, J., and Wang, S. (2024). Citric acid loaded hydrogel-coated stent for dissolving pancreatic duct calculi. Gels 10 (2), 125. doi:10.3390/gels10020125
Li, T., Li, J., Chen, Z., Zhang, S., Li, S., Wageh, S., et al. (2022c). Glioma diagnosis and therapy: current challenges and nanomaterial-based solutions. J. Control Release 352, 338–370. doi:10.1016/j.jconrel.2022.09.065
Li, W., Chen, J., Zhao, S., Huang, T., Ying, H., Trujillo, C., et al. (2022a). High drug-loaded microspheres enabled by controlled in-droplet precipitation promote functional recovery after spinal cord injury. Nat. Commun. 13 (1), 1262. doi:10.1038/s41467-022-28787-7
Li, Y., Yang, L., Hu, F., Xu, J., Ye, J., Liu, S., et al. (2022b). Novel thermosensitive hydrogel promotes spinal cord repair by regulating mitochondrial function. ACS Appl. Mater Interfaces 14 (22), 25155–25172. doi:10.1021/acsami.2c04341
Lin, R.-R., Huang, H.-F., and Tao, Q.-Q. (2023). Advancing the battle against Alzheimer's Disease: a focus ontargeting tau pathology by antisense oligonucleotide. Innovation Med. 1 (2), 100020. doi:10.59717/j.xinn-med.2023.100020
Liu, M., Yu, W., Zhang, F., Liu, T., Li, K., Lin, M., et al. (2021). Fe(3)O(4)@Polydopamine-Labeled MSCs targeting the spinal cord to treat neuropathic pain under the guidance of a magnetic field. Int. J. Nanomedicine 16, 3275–3292. doi:10.2147/ijn.s296398
Liu, T., Xiao, B., Xiang, F., Tan, J., Chen, Z., Zhang, X., et al. (2020). Ultrasmall copper-based nanoparticles for reactive oxygen species scavenging and alleviation of inflammation related diseases. Nat. Commun. 11 (1), 2788. doi:10.1038/s41467-020-16544-7
Liu, Y., Ai, K., Liu, J., Deng, M., He, Y., and Lu, L. (2013). Dopamine-melanin colloidal nanospheres: an efficient near-infrared photothermal therapeutic agent for in vivo cancer therapy. Adv. Mater 25 (9), 1353–1359. doi:10.1002/adma.201204683
Liu, Y., Ai, K., and Lu, L. (2014). Polydopamine and its derivative materials: synthesis and promising applications in energy, environmental, and biomedical fields. Chem. Rev. 114 (9), 5057–5115. doi:10.1021/cr400407a
Liu, Y., Bhattarai, P., Dai, Z., and Chen, X. (2019). Photothermal therapy and photoacoustic imaging via nanotheranostics in fighting cancer. Chem. Soc. Rev. 48 (7), 2053–2108. doi:10.1039/c8cs00618k
Lou, X., Hu, Y., Zhang, H., Liu, J., and Zhao, Y. (2021). Polydopamine nanoparticles attenuate retina ganglion cell degeneration and restore visual function after optic nerve injury. J. Nanobiotechnology 19 (1), 436. doi:10.1186/s12951-021-01199-3
Lu, J., Cai, L., Dai, Y., Liu, Y., Zuo, F., Ni, C., et al. (2021). Polydopamine-based nanoparticles for photothermal therapy/chemotherapy and their synergistic therapy with autophagy inhibitor to promote antitumor treatment. Chem. Rec. 21 (4), 781–796. doi:10.1002/tcr.202000170
Lu, X., Hou, X., Tang, H., Yi, X., and Wang, J. (2022). A high-quality CdSe/CdS/ZnS quantum-dot-based FRET aptasensor for the simultaneous detection of two different alzheimer's disease core biomarkers. Nanomater. (Basel) 12 (22), 4031. doi:10.3390/nano12224031
Ma, J., Li, J., Wang, X., Li, M., Teng, W., Tao, Z., et al. (2023). GDNF-loaded polydopamine nanoparticles-based anisotropic scaffolds promote spinal cord repair by modulating inhibitory microenvironment. Adv. Healthc. Mater 12 (8), e2202377. doi:10.1002/adhm.202202377
Ma, S., Zhou, X., Wang, Y., Li, Z., Wang, Y., Shi, J., et al. (2022a). MG53 protein rejuvenates hUC-MSCs and facilitates their therapeutic effects in AD mice by activating Nrf2 signaling pathway. Redox Biol. 53, 102325. doi:10.1016/j.redox.2022.102325
Ma, X., Wang, C., Dong, Z., Hu, C., and Feng, L. (2022b). Lipid-coated CaCO(3)-PDA nanoparticles as a versatile nanocarrier to enable pH-responsive dual modal imaging-guided combination cancer therapy. J. Mater Chem. B 10 (21), 4096–4104. doi:10.1039/d2tb00022a
Ma, Y., Wang, J., Wang, Y., and Yang, G. Y. (2017). The biphasic function of microglia in ischemic stroke. Prog. Neurobiol. 157, 247–272. doi:10.1016/j.pneurobio.2016.01.005
Madhurakkat Perikamana, S. K., Lee, J., Lee, Y. B., Shin, Y. M., Lee, E. J., Mikos, A. G., et al. (2015). Materials from mussel-inspired chemistry for cell and tissue engineering applications. Biomacromolecules 16 (9), 2541–2555. doi:10.1021/acs.biomac.5b00852
Marsden, C. A. (2006). Dopamine: the rewarding years. Br. J. Pharmacol. 147 (Suppl. 1), S136–S144. doi:10.1038/sj.bjp.0706473
Martinelli, C., Pucci, C., Battaglini, M., Marino, A., and Ciofani, G. (2020). Antioxidants and nanotechnology: promises and limits of potentially disruptive approaches in the treatment of central nervous system diseases. Adv. Healthc. Mater 9 (3), e1901589. doi:10.1002/adhm.201901589
Mrówczyński, R. (2018). Polydopamine-based multifunctional (Nano)materials for cancer therapy. ACS Appl. Mater Interfaces 10 (9), 7541–7561. doi:10.1021/acsami.7b08392
Nguyen, T. T., Dung Nguyen, T. T., Vo, T. K., Tran, N. M., Nguyen, M. K., Van Vo, T., et al. (2021). Nanotechnology-based drug delivery for central nervous system disorders. Biomed. Pharmacother. 143, 112117. doi:10.1016/j.biopha.2021.112117
Ostrom, Q. T., Patil, N., Cioffi, G., Waite, K., Kruchko, C., and Barnholtz-Sloan, J. S. (2022). Corrigendum to: CBTRUS statistical report: primary brain and other central nervous system tumors diagnosed in the United States in 2013-2017. Neuro Oncol. 24 (7), 1214. doi:10.1093/neuonc/noaa269
Phipps, M. S., and Cronin, C. A. (2020). Management of acute ischemic stroke. Bmj 368, l6983. doi:10.1136/bmj.l6983
Pu, Y., Zhu, Y., Qiao, Z., Xin, N., Chen, S., Sun, J., et al. (2021). A Gd-doped polydopamine (PDA)-based theranostic nanoplatform as a strong MR/PA dual-modal imaging agent for PTT/PDT synergistic therapy. J. Mater Chem. B 9 (7), 1846–1857. doi:10.1039/d0tb02725a
Qin, J., Guan, Y., Li, Z., Guo, X., Zhang, M., Wang, D., et al. (2022). Aptamer conjugated polydopamine-coated gold nanoparticles as a dual-action nanoplatform targeting β-amyloid peptide for Alzheimer's disease therapy. J. Mater Chem. B 10 (41), 8525–8534. doi:10.1039/d2tb01499h
Reeves, D. B., and Weaver, J. B. (2014). Approaches for modeling magnetic nanoparticle dynamics. Crit. Rev. Biomed. Eng. 42 (1), 85–93. doi:10.1615/critrevbiomedeng.2014010845
Ren, Y., Zhao, X., Liang, X., Ma, P. X., and Guo, B. (2017). Injectable hydrogel based on quaternized chitosan, gelatin and dopamine as localized drug delivery system to treat Parkinson's disease. Int. J. Biol. Macromol. 105 (Pt 1), 1079–1087. doi:10.1016/j.ijbiomac.2017.07.130
Ruan, H., Li, Y., Zheng, D., Deng, L., Chen, G., Zhang, X., et al. (2023). Engineered extracellular vesicles for ischemic stroke treatment. Innovation 4 (2), 100394. doi:10.1016/j.xinn.2023.100394
Saeedi, M., Eslamifar, M., Khezri, K., and Dizaj, S. M. (2019). Applications of nanotechnology in drug delivery to the central nervous system. Biomed. Pharmacother. 111, 666–675. doi:10.1016/j.biopha.2018.12.133
Sanai, N., and Berger, M. S. (2018). Surgical oncology for gliomas: the state of the art. Nat. Rev. Clin. Oncol. 15 (2), 112–125. doi:10.1038/nrclinonc.2017.171
Sardoiwala, M. N., Srivastava, A. K., Kaundal, B., Karmakar, S., and Choudhury, S. R. (2020). Recuperative effect of metformin loaded polydopamine nanoformulation promoting EZH2 mediated proteasomal degradation of phospho-α-synuclein in Parkinson's disease model. Nanomedicine. 24, 102088. doi:10.1016/j.nano.2019.102088
Shakeel, F., Fazal, M. W., Zulfiqar, A., Zafar, F., Akhtar, N., Ahmed, A., et al. (2022). Melamine-derived N-rich C-entrapped Au nanoparticles for sensitive and selective monitoring of dopamine in blood samples. RSC Adv. 12 (40), 26390–26399. doi:10.1039/d2ra02754b
Shank, C. D., Walters, B. C., and Hadley, M. N. (2019). Current topics in the management of acute traumatic spinal cord injury. Neurocrit Care 30 (2), 261–271. doi:10.1007/s12028-018-0537-5
Shanmugam, V., Selvakumar, S., and Yeh, C. S. (2014). Near-infrared light-responsive nanomaterials in cancer therapeutics. Chem. Soc. Rev. 43 (17), 6254–6287. doi:10.1039/c4cs00011k
Shi, H., Jin, L., Li, J., Liang, K., Li, X., Ye, Z., et al. (2022a). Mesoporous polydopamine nanoparticles for sustained release of rapamycin and reactive oxygen species scavenging to synergistically accelerate neurogenesis after spinal cord injury. J. Mater Chem. B 10 (33), 6351–6359. doi:10.1039/d2tb00841f
Shi, J., Yang, Y., Yin, N., Liu, C., Zhao, Y., Cheng, H., et al. (2022b). Engineering CXCL12 biomimetic decoy-integrated versatile immunosuppressive nanoparticle for ischemic stroke therapy with management of overactivated brain immune microenvironment. Small Methods 6 (1), e2101158. doi:10.1002/smtd.202101158
Shi, L., Rocha, M., Leak, R. K., Zhao, J., Bhatia, T. N., Mu, H., et al. (2018). A new era for stroke therapy: integrating neurovascular protection with optimal reperfusion. J. Cereb. Blood Flow. Metab. 38 (12), 2073–2091. doi:10.1177/0271678x18798162
Shi, T., Chen, S., Long, Y., and Gu, Z. (2023). Safety and efficacy of stenting for symptomatic intracranial artery stenosis: a systematic reveiw and meta-analysis. Front. Pharmacol. 14, 1122842. doi:10.3389/fphar.2023.1122842
Srivastava, A. K., Roy Choudhury, S., and Karmakar, S. (2020). Melatonin/polydopamine nanostructures for collective neuroprotection-based Parkinson's disease therapy. Biomater. Sci. 8 (5), 1345–1363. doi:10.1039/c9bm01602c
Tan, J., Duan, X., Zhang, F., Ban, X., Mao, J., Cao, M., et al. (2020). Theranostic nanomedicine for synergistic chemodynamic therapy and chemotherapy of orthotopic glioma. Adv. Sci. (Weinh) 7 (24), 2003036. doi:10.1002/advs.202003036
Tang, C., Luo, J., Yan, X., Huang, Q., Huang, Z., Luo, Q., et al. (2022). Melanin nanoparticles enhance the neuroprotection of mesenchymal stem cells against hypoxic-ischemic injury by inhibiting apoptosis and upregulating antioxidant defense. Cell Biol. Int. 46 (6), 933–946. doi:10.1002/cbin.11781
Tang, C., Wang, C., Zhang, Y., Xue, L., Li, Y., Ju, C., et al. (2019). Recognition, intervention, and monitoring of neutrophils in acute ischemic stroke. Nano Lett. 19 (7), 4470–4477. doi:10.1021/acs.nanolett.9b01282
Tang, Y., and Le, W. (2016). Differential roles of M1 and M2 microglia in neurodegenerative diseases. Mol. Neurobiol. 53 (2), 1181–1194. doi:10.1007/s12035-014-9070-5
Tian, L., Chen, C., Gong, J., Han, Q., Shi, Y., Li, M., et al. (2023). The convenience of polydopamine in designing SERS biosensors with a sustainable prospect for medical application. Sensors (Basel) 23 (10), 4641. doi:10.3390/s23104641
Tian, M., Ma, Z., and Yang, G. Z. (2024). Micro/nanosystems for controllable drug delivery to the brain. Innov. (Camb) 5 (1), 100548. doi:10.1016/j.xinn.2023.100548
Tiwari, S., Atluri, V., Kaushik, A., Yndart, A., and Nair, M. (2019). Alzheimer's disease: pathogenesis, diagnostics, and therapeutics. Int. J. Nanomedicine 14, 5541–5554. doi:10.2147/ijn.s200490
Tran, H. Q., Batul, R., Bhave, M., and Yu, A. (2019). Current advances in the utilization of polydopamine nanostructures in biomedical therapy. Biotechnol. J. 14 (12), e1900080. doi:10.1002/biot.201900080
Vashist, A., Manickam, P., Raymond, A. D., Arias, A. Y., Kolishetti, N., Vashist, A., et al. (2023). Recent advances in nanotherapeutics for neurological disorders. ACS Appl. Bio Mater 6 (7), 2614–2621. doi:10.1021/acsabm.3c00254
Vong, L. B., Sato, Y., Chonpathompikunlert, P., Tanasawet, S., Hutamekalin, P., and Nagasaki, Y. (2020). Self-assembled polydopamine nanoparticles improve treatment in Parkinson's disease model mice and suppress dopamine-induced dyskinesia. Acta Biomater. 109, 220–228. doi:10.1016/j.actbio.2020.03.021
von Leden, R. E., Yauger, Y. J., Khayrullina, G., and Byrnes, K. R. (2017). Central nervous system injury and nicotinamide adenine dinucleotide phosphate oxidase: oxidative stress and therapeutic targets. J. Neurotrauma 34 (4), 755–764. doi:10.1089/neu.2016.4486
Wang, W., Tang, Z., Zhang, Y., Wang, Q., Liang, Z., and Zeng, X. (2020). Mussel-inspired polydopamine: the bridge for targeting drug delivery system and synergistic cancer treatment. Macromol. Biosci. 20 (10), e2000222. doi:10.1002/mabi.202000222
Wang, W., Zheng, J., Zhou, H., Liu, Q., Jia, L., Zhang, X., et al. (2022b). Polydopamine-based nanocomposite as a biomimetic antioxidant with a variety of enzymatic activities for Parkinson's disease. ACS Appl. Mater Interfaces 14, 32901–32913. doi:10.1021/acsami.2c06981
Wang, Y., Jiang, J., Fu, X., Zhang, J., Song, J., Wang, Y., et al. (2022a). Fe(3)O(4)@polydopamine nanoparticle-loaded human umbilical cord mesenchymal stem cells improve the cognitive function in Alzheimer's disease mice by promoting hippocampal neurogenesis. Nanomedicine. 40, 102507. doi:10.1016/j.nano.2021.102507
Waris, A., Ali, A., Khan, A. U., Asim, M., Zamel, D., Fatima, K., et al. (2022). Applications of various types of nanomaterials for the treatment of neurological disorders. Nanomater. (Basel) 12 (13), 2140. doi:10.3390/nano12132140
Wojsiat, J., Zoltowska, K. M., Laskowska-Kaszub, K., and Wojda, U. (2018). Oxidant/antioxidant imbalance in alzheimer's disease: therapeutic and diagnostic prospects. Oxid. Med. Cell Longev. 2018, 6435861. doi:10.1155/2018/6435861
Wong, H. L., Wu, X. Y., and Bendayan, R. (2012). Nanotechnological advances for the delivery of CNS therapeutics. Adv. Drug Deliv. Rev. 64 (7), 686–700. doi:10.1016/j.addr.2011.10.007
Wu, D., Fei, F., Zhang, Q., Wang, X., Gong, Y., Chen, X., et al. (2022a). Nanoengineered on-demand drug delivery system improves efficacy of pharmacotherapy for epilepsy. Sci. Adv. 8 (2), eabm3381. doi:10.1126/sciadv.abm3381
Wu, D., Zhou, J., Zheng, Y., Zheng, Y., Zhang, Q., Zhou, Z., et al. (2023). Pathogenesis-adaptive polydopamine nanosystem for sequential therapy of ischemic stroke. Nat. Commun. 14 (1), 7147. doi:10.1038/s41467-023-43070-z
Wu, H., Wei, M., Xu, Y., Li, Y., Zhai, X., Su, P., et al. (2022b). PDA-based drug delivery nanosystems: a potential approach for glioma treatment. Int. J. Nanomedicine 17, 3751–3775. doi:10.2147/ijn.s378217
Xie, J., Yang, G., Tan, X., Yuan, R., and Chen, S. (2023). Coreactant-free electrochemiluminescence of polyfluorene nanoparticle coupling double quencher for β-amyloid1-42 detection. Talanta. 258, 124398. doi:10.1016/j.talanta.2023.124398
Yan, J., Liu, T., Li, Y., Zhang, J., Shi, B., Zhang, F., et al. (2023). Effects of magnetically targeted iron oxide@polydopamine-labeled human umbilical cord mesenchymal stem cells in cerebral infarction in mice. Aging (Albany NY) 15 (4), 1130–1142. doi:10.18632/aging.204540
Yan, X., Pan, Y., Ji, L., Gu, J., Hu, Y., Xia, Y., et al. (2021). Multifunctional metal–organic framework as a versatile nanoplatform for Aβ oligomer imaging and chemo-photothermal treatment in living cells. Anal. Chem. 93 (41), 13823–13834. doi:10.1021/acs.analchem.1c02459
Yang, J., Zhao, Y., Zhang, L., Fan, H., Qi, C., Zhang, K., et al. (2018). RIPK3/MLKL-Mediated neuronal necroptosis modulates the M1/M2 polarization of microglia/macrophages in the ischemic cortex. Cereb. Cortex 28 (7), 2622–2635. doi:10.1093/cercor/bhy089
Yao, K., Gan, J., Zhao, D., Li, M., Shen, X., Yang, Y., et al. (2023). A core-satellite-like nanoassembly reverses a decisive tyrosine hydrox ylase loss in degenerative dopaminergic neurons. Nano Res. 16 (7), 9835–9847. doi:10.1007/s12274-023-5729-4
Yin, Z., Zhang, Z., Gao, D., Luo, G., Ma, T., Wang, Y., et al. (2023). Stepwise coordination-driven metal-phenolic nanoparticle as a neuroprotection enhancer for alzheimer's disease therapy. ACS Appl. Mater Interfaces 15 (1), 524–540. doi:10.1021/acsami.2c18060
Ying, C., Zhang, J., Zhang, H., Gao, S., Guo, X., Lin, J., et al. (2023). Stem cells in central nervous system diseases: promising therapeutic strategies. Exp. Neurol. 369, 114543. doi:10.1016/j.expneurol.2023.114543
Yun, S., Shin, T. H., Lee, J. H., Cho, M. H., Kim, I. S., Kim, J. W., et al. (2018). Design of magnetically labeled cells (Mag-Cells) for in vivo control of stem cell migration and differentiation. Nano Lett. 18 (2), 838–845. doi:10.1021/acs.nanolett.7b04089
Zandieh, M., and Liu, J. (2020). Cooperative metal ion-mediated adsorption of spherical nucleic acids with a large hysteresis. Langmuir 36 (47), 14324–14332. doi:10.1021/acs.langmuir.0c02677
Zhang, H., van Os, W. L., Tian, X., Zu, G., Ribovski, L., Bron, R., et al. (2021b). Development of curcumin-loaded zein nanoparticles for transport across the blood-brain barrier and inhibition of glioblastoma cell growth. Biomater. Sci. 9 (21), 7092–7103. doi:10.1039/d0bm01536a
Zhang, T. T., Li, W., Meng, G., Wang, P., and Liao, W. (2016). Strategies for transporting nanoparticles across the blood-brain barrier. Biomater. Sci. 4 (2), 219–229. doi:10.1039/c5bm00383k
Zhang, W., Xiong, B. R., Zhang, L. Q., Huang, X., Yuan, X., Tian, Y. K., et al. (2021a). The role of the GABAergic system in diseases of the central nervous system. Neuroscience 470, 88–99. doi:10.1016/j.neuroscience.2021.06.037
Zhang, Y., Wang, J., Xiao, J., Fang, T., Hu, N., Li, M., et al. (2019). An electrospun fiber-covered stent with programmable dual drug release for endothelialization acceleration and lumen stenosis prevention. Acta Biomater. 94, 295–305. doi:10.1016/j.actbio.2019.06.008
Zhao, H., Zeng, Z., Liu, L., Chen, J., Zhou, H., Huang, L., et al. (2018). Polydopamine nanoparticles for the treatment of acute inflammation-induced injury. Nanoscale 10 (15), 6981–6991. doi:10.1039/c8nr00838h
Zhao, M., van Straten, D., Broekman, M. L. D., Préat, V., and Schiffelers, R. M. (2020). Nanocarrier-based drug combination therapy for glioblastoma. Theranostics 10 (3), 1355–1372. doi:10.7150/thno.38147
Zhao, X., Deng, M., Wang, J., Liu, B., Dong, Y., and Li, Z. (2023). Miniaturized neural implants for localized and controllable drug delivery in the brain. J. Mater Chem. B 11 (27), 6249–6264. doi:10.1039/d3tb00728f
Zheng, P., Ding, B., and Li, G. (2020). Polydopamine-incorporated nanoformulations for biomedical applications. Macromol. Biosci. 20 (12), e2000228. doi:10.1002/mabi.202000228
Keywords: polydopamine nano, reactive oxygen species, inflammatory response, blood-brain barrier, neurons, central nervous system, disease
Citation: Ren S, Xiao X, Lv J, Lv S, Wang X, Liu R and Kong Q-x (2024) Advances in the study of polydopamine nanotechnology in central nervous system disorders. Front. Mater. 11:1396397. doi: 10.3389/fmats.2024.1396397
Received: 05 March 2024; Accepted: 26 March 2024;
Published: 12 April 2024.
Edited by:
Benjamin Salas Valdez, Autonomous University of Baja California, MexicoReviewed by:
Jiulong Zhao, Naval Medical University, ChinaHuitong Ruan, Shanghai Jiao Tong University, China
Copyright © 2024 Ren, Xiao, Lv, Lv, Wang, Liu and Kong. This is an open-access article distributed under the terms of the Creative Commons Attribution License (CC BY). The use, distribution or reproduction in other forums is permitted, provided the original author(s) and the copyright owner(s) are credited and that the original publication in this journal is cited, in accordance with accepted academic practice. No use, distribution or reproduction is permitted which does not comply with these terms.
*Correspondence: Ruihan Liu, ruihanliu1987@163.com; Qing-xia Kong, kxdqy8@sohu.com